- 1Bio Environmental Science and Technology (BEST) Lab, Ghent University Global Campus, Yeonsu-gu, Republic of Korea
- 2Department of Biology, Phycology Research Group, Ghent University, Ghent, Belgium
- 3Department of Plant Biotechnology and Bioinformatics, Ghent University, Ghent, Belgium
- 4VIB-UGent Center for Plant Systems Biology, Ghent, Belgium
This minireview examines the current state and challenges of genome editing in macroalgae. Despite the ecological and economic significance of this group of organisms, genome editing has seen limited applications. While CRISPR functionality has been established in two brown (Ectocarpus species 7 and Saccharina japonica) and one green seaweed (Ulva prolifera), these studies are limited to proof-of-concept demonstrations. All studies also (co)-targeted ADENINE PHOSPHORIBOSYL TRANSFERASE to enrich for mutants, due to the relatively low editing efficiencies. To advance the field, there should be a focus on advancing auxiliary technologies, particularly stable transformation, so that novel editing reagents can be screened for their efficiency. More work is also needed on understanding DNA repair in these organisms, as this is tightly linked with the editing outcomes. Developing efficient genome editing tools for macroalgae will unlock the ability to characterize their genes, which is largely uncharted terrain. Moreover, given their economic importance, genome editing will also impact breeding campaigns to develop strains that have better yields, produce more commercially valuable compounds, and show improved resilience to the impacts of global change.
1 Introduction
Multicellular marine macroalgae are typically classified into the red (Rhodophyta), brown (Phaeophyta) and green (Chlorophyta) seaweeds (Littler and Littler, 2011; Pereira, 2021). Their shared designation as seaweeds, however, belies the profound evolutionary divergence among these groups, as the lineages of photosynthetic organisms have split at the root of the eukaryotic tree ∼2 billion years ago (Strassert et al., 2021). The ecological and economic significance of these organisms cannot be overstated. As primary producers, seaweeds play a pivotal role in marine ecosystems. Furthermore, wild harvested or cultured individuals produce food, feed, fuel, and useful chemicals. This versatility underpins a robust seaweed farming industry, which boasts an estimated global value of 14.7 billion USD (Cai et al., 2021). On the other hand, certain seaweed genera are noted for their less favorable effects, including biofouling and massive blooming (Coates et al., 2015).
Despite their importance, seaweed research has only recently entered the era of genomics and molecular biology. Since the first seaweed nuclear genome of Ectocarpus species 7 was reported (Cock et al., 2010), many representatives of different seaweed groups have been sequenced (reviewed in Stock et al. (2024); Table 1). Nevertheless, these genomes remain a black box. When Blaby-Haas and Merchant (2019) assessed about 100 genomes of micro- and macroalgae, they found that over 50% of the genes were of unknown function. Although genome data is crucial for understanding seaweed biology, functional characterization of genes in these diverse species remains largely unexplored. To bridge this gap, one approach to perform functional analysis of genes is studying mutants that occur either naturally or by induction (Table 1). In green algae, mutations have been found that affect cell division, vegetative development, or result in sterility (Bryhni, 1974; Kakinuma et al., 2006; Jongma et al., 2013; Oertel et al., 2015; Gao et al., 2017). Fast growing, differently pigmented, or high monospore-producing mutants were isolated from a number of different red algae genera (Kursar et al., 1983; Patwary and Meer, 1983; Niwa et al., 1993; Yan et al., 2000; Plastino et al., 2004; Cornish et al., 2013; Lee and Choi, 2018; Marchi and Plastino, 2020; Sano et al., 2020). In brown algae, researchers have found mutants with impaired life cycles, abnormal cell differentiation or with defects in cell elongation and higher growth rates (Peters et al., 2008; Coelho et al., 2011; Le Bail et al., 2011; Hirano et al., 2020; Sato et al., 2021). It is worth noting that for most of these mutants the causative mutations remain unknown, underscoring the need for reverse genetics tools in macroalgae research.
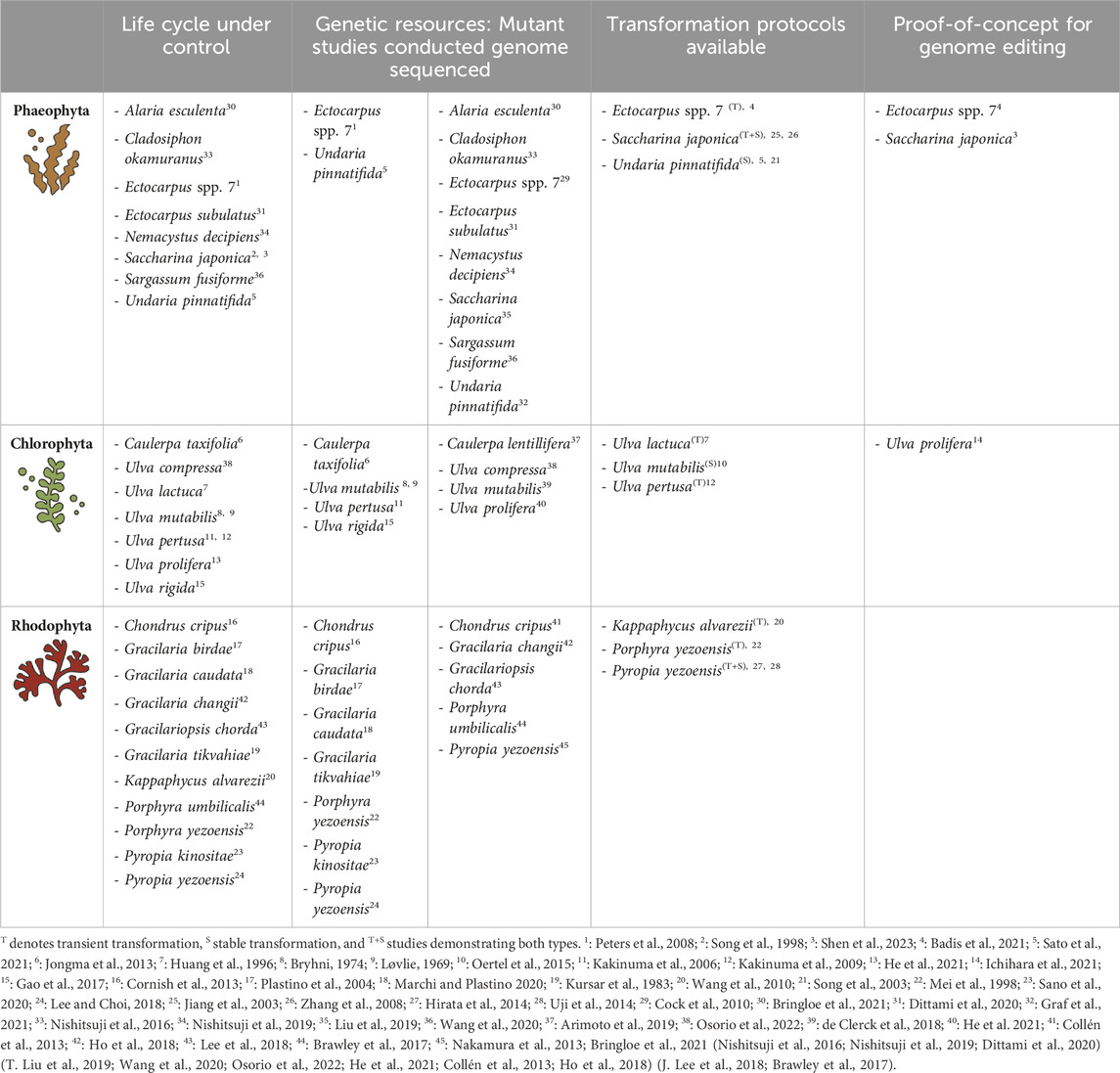
TABLE 1. Status of macroalgae research. This table provides a non-exhaustive overview of key macroalgae research domains discussed in this review, together with selected species. Superscript numbers (1–45) link to references detailed below table.
A major bottleneck for macroalgal genetic research is the lack of protocols for generating transgenic organisms (Table 1). Already in 1998, Song and co-workers reported the stable transformation of the brown seaweed Laminaria japonica, but few reports have been published after that. It is only in recent years that there has been a renewed interest in optimizing transformation protocols. One recently published cloning toolkit was designed to stably express nuclear genes in the green seaweed Ulva mutabilis/compressa (Blomme et al., 2021). This toolkit allows to overexpress transgenes and tagged endogenous genes. In the red seaweed P. yezoensis, overexpression of endogenous genes has proven to be feasible by Zheng et al. (2022). Another report by Cao et al. (2022), optimized a biolistic protocol to allow a high-efficiency stable transformation in this species. However, it should be noted that only reporter genes were used in this study. Despite these successes, only a transient expression of transgenes is typically achieved in macroalgae (reviewed in Mikami (2013)). Consequently, there is a paucity of reports describing successful stable transformation in macroalgae. Hurdles in stable transgene expression in macroalgae include the identification of a selection system, overcoming transgene silencing and the identification of regulatory sequences (Stock et al., 2024).
2 Genome editing in macroalgae: The current state
At least on paper, seaweeds are attractive organisms for genome engineering. Typically, they exhibit advantageous characteristics such as a multicellular haploid life stage, relatively small genomes with few duplication events, and the production of prodigious amounts of (a)sexual spores/gametes (Stock et al., 2024). Although not all life cycles in this very diverse group of organisms are easy to complete in the lab (Table 1), some life cycles are also relatively short. Ulva mutabilis, for example, has a cycle that can be completed in less than 2 months, putting it on par with the plant model Arabidopsis thaliana (Krämer, 2015). Despite these favorable traits, successful reports of genome editing in macroalgae are very scarce, much like stable transformation protocols.
Homologous recombination is a commonly used system for gene editing in animals, bacteria, and fungi (Aguilera and Carreira, 2021). However, there are no reports of this approach being functional in macroalgae, with the sole exception being a plastid gene expression system in Pyropia yezoensis. This system, however, does not permit alterations of the nuclear genome (Kong et al., 2017). More recent additions to the bioengineer’s toolbox such as Meganucleases, ZFNs (Zinc Finger Nucleases), TALENs (Transcription Activator-Like Effector Nucleases) and CRISPR (Clustered Regularly Interspaced Short Palindromic Repeats) systems have been applied in about 10 microalgae genera (Sizova et al., 2013; Daboussi et al., 2014; Greiner et al., 2017; Jeong et al., 2023), but only CRISPR has been tested in macroalgae.
Three reports have demonstrated the successful application CRISPR technology in macroalgae: the brown algae Ectocarpus species 7 (Badis et al., 2021) and Saccharina japonica (Shen et al., 2023), as well as in the green seaweed Ulva prolifera (Ichihara et al., 2022) (Table 1). All these studies made use of CRISPR-Cas9 ribonucleoproteins (RNPs), which consist of preassembled Cas9 protein-gRNA complexes. This demonstrates that a functional stable transformation system is not a prerequisite to successfully make use of CRISPR in seaweeds. Indeed, this DNA-free method may even offer advantages in terms of efficiency, as well as in compliance with legislative and regulatory requirements (Woo et al., 2015). Additionally, there is no need to optimize transgene expression which is often a bottleneck in macroalgae (Blomme et al., 2021). ADENINE PHOSPHORIBOSYL TRANSFERASE (APT) was selected as the primary target in all three studies. This conserved gene is involved in the salvage pathway of adenine, and the enzyme encoded by this gene uses adenine or analogues thereof as its substrate. When the analogue 2-fluoroadenine (2-FA) is applied, it is converted into toxic nucleotides in wild type cells, but not in cells containing a knockout of this gene (Schaff, 1994). The Ectocarpus species 7 study (Badis et al., 2021) went one step further, and utilized a co-targeting approach to enrich for modifications at a second locus (Kim et al., 2014; Mikkelsen and Bak, 2023). This approach led to the isolation of double mutants for three different secondary loci at frequencies between 0% and 100% of the 2-FA resistant population. This stark difference in editing outcomes may be accounted for by differences in gRNA efficiency, which are generally not easy to predict in silico (Konstantakos et al., 2022). Nevertheless, this study clearly shows the potential of using APT as a selectable marker to enrich for mutants in a target of interest. With biolistically transformed gametes, the editing efficiency was approximately 2 × 10−5 for single mutants and 2.5 × 10−6 for double mutants under the most favorable experimental conditions reported. When microinjecting unilocular sporangia—which typically develop a minimum of 100 haploid spores—higher transformation efficiencies of approximately 4%–7% for single mutants and 0.3% for double mutants were observed. Despite these rather low efficiencies, double mutants could be generated in 80% of the experiments. In S. japonica (Shen et al., 2023), the reported APT editing efficiencies were remarkably high, 8.6% and 4.5% for the microinjected female and male gametophytes, respectively. The higher efficiency in female gametophytes was attributed to their larger cell size which potentially minimizes injection damage. The U. prolifera study (Ichihara et al., 2022) made use of polyethylene glycol (PEG)-mediated transfection of gametes and reported APT mutation efficiencies between 1.6 × 10−1 and 3.0 × 10−3, and due to the massive number of initial gametes (1.0 × 106), successful CRISPR events could be detected in all experiments. All three studies reported small indel mutations (<10 bp) as the primary editing outcome. Additionally, in the S. japonica study (Shen et al., 2023) two instances of larger deletions were observed among 35 mutants, one being 35 base pairs and the other 60 base pairs in length. Notably, the U. prolifera study (Ichihara et al., 2022) also identified substitution mutations. For one specific gRNA, substitutions–with no accompanying indels–were observed in as many as 16% of the mutants (2 out of 12 individuals).
Taken together, these publications demonstrate the successful application of genome editing in both brown and green seaweed species. It is important to note, however, that these reports were limited to proof-of-concept studies. Further research is needed to increase the mutation efficiencies and broaden the applicability of targeted mutagenesis systems in seaweeds.
3 Charting the way forward
Indeed, there are still some shortcomings that need to be addressed to make genome editing a viable technology in seaweeds. As previously noted, genome editing has been successfully applied in only a select few species (Table 1). To broaden the applicability of this technology (Figure 1A), more genomes of seaweed species will need to become available (Figure 1B). Ideally, these species should have life cycles that can be completed in laboratory settings (Figure 1C), allowing genetic transformation protocols to be developed (Figure 1D). Another limitation is that in all studies published until now, APT was either the sole gene that was targeted or was used to enrich mutants at a second locus (Figure 1E). While a knockout in APT is not lethal, it is likely that there will be an effect on the fitness of these organisms (Liu et al., 2023). Genetic interactions with other genes can also not be excluded, which may be an issue for functional characterization of other loci. A major challenge is that the reported mutation efficiencies are very low, except for S. japonica. Even in this species, the creation of double or higher order mutants may be difficult to achieve using the published protocol. In general, some kind of selection will remain necessary in absence of a drastic increase in editing efficiencies.
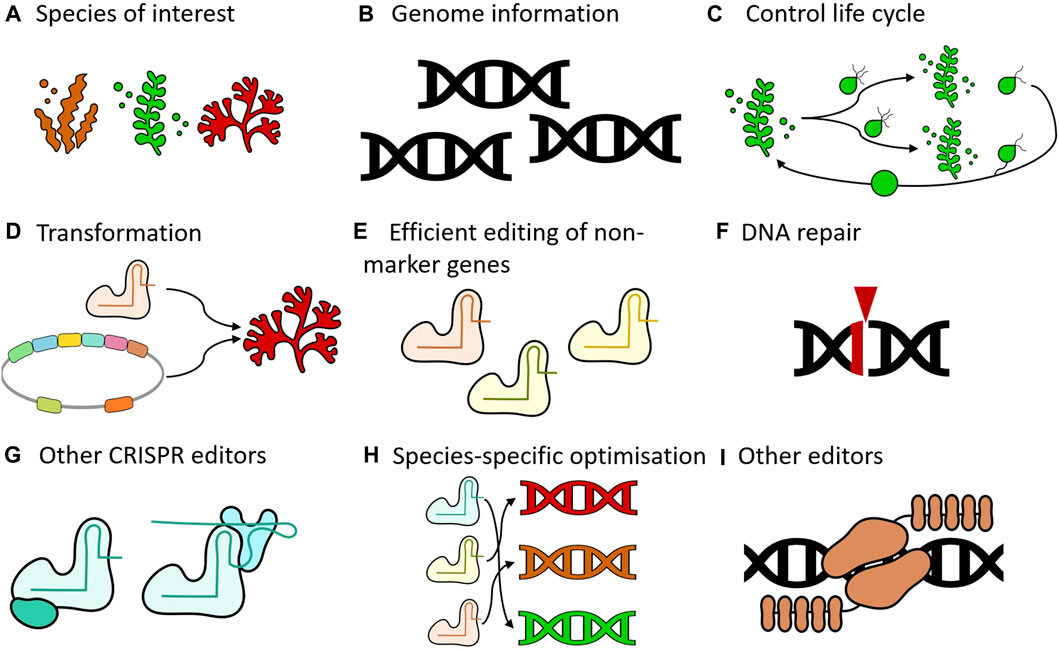
FIGURE 1. Key areas for advancing seaweed genome editing. (A) Species of interest. Expansion of the range of species that are amenable to genome editing would be desirable. Genome editing has not been demonstrated in any red seaweed, for example. (B) Genome information. Having access to genomic information of target species is critical for implementing genome editing technology as this will not only inform on the design of the reagents but will also contribute to avoiding off-target effects. (C) Control life cycle. Completing the full life cycle under laboratory conditions is desirable to advance supporting technologies (e.g., genetic transformation) and genome editing. (D) Transformation. Various methods can be utilized to introduce reagents into organisms, including electroporation, microinjection, transfection, and Agrobacterium-mediated transformation. The establishment of functional systems, whether transient or stable, is essential for genome editing. (E) Efficient editing of non-marker genes. All published studies targeted ADENINE PHOSPHORIBOSYL TRANSFERASE (APT) as the only gene or to enrich mutants at a second locus. Future efforts should focus on developing methods to generate mutants without the need for such enrichment processes. (F) DNA repair. Nucleases create double-stranded breaks in the DNA, which subsequently activate repair mechanisms. Gaining deeper insights into the specific repair processes active in the target species will guide the selection of appropriate genome editing strategies. (G) Other CRISPR editors. CRISPR mediated knock-outs are a very powerful tool, but other modalities such as base editing and prime editing can also be explored. Depending on the repair mechanisms that are active, these systems may be more efficient than conventional CRISPR systems (H) Species-specific optimization. Genome editing tools do not operate with the same efficiency across different organisms. Species-specific optimization can be beneficial to address this variability. (I) Other editors can also be used. This includes systems that already exist (e.g., TALENs), as well as systems that are yet to be developed.
To improve the efficiency of genome editing in seaweed, several approaches may be utilized. We will focus here on CRISPR systems, as these systems are currently unparalleled in knocking out genes in a variety of organisms. A first important consideration is that these nucleases will create double-stranded breaks, which will then need to be repaired by the cell. Therefore, the importance of the DNA repair mechanism is paramount (Figure 1F). This is a largely uncharted water, as these mechanisms have not been studied in detail in macroalgae. As previously discussed, genome editing results showed significant variation among the three seaweed species tested to date. Notably, U. prolifera exhibited a high incidence of substitution mutations, which is extremely rare in plants or animals (Hwang et al., 2020; Zhang et al., 2020). A lot of work on DNA repair has been done in other organisms, with many published protocols that can be leveraged here as well (Bjergbæk, 2016; Zentout et al., 2021; van de Kooij and van Attikum, 2022). In mammalian systems the successful redirection of DNA repair pathways has already been demonstrated with chemical inhibitors (Maruyama et al., 2015), cell cycle synchronization (Lin et al., 2014), Homology-directed repair (HDR) template modifications (Cruz-Becerra and Kadonaga, 2020; Schubert et al., 2021), modulation of regulatory factors (Canny et al., 2018; Charpentier et al., 2018; Jayavaradhan et al., 2019), and engineered Cas9 variants (Chauhan et al., 2023). In this respect, having a functional DNA based - either stable or transient - transformation system would be a definite advantage. The ribonucleoproteins (RNPs) technology that was used in all studies to date depends on the availability of a recombinant Cas9 protein. While the standard purified Cas9 protein is commercially available, creating new variants or fusions would require the challenging and expensive task of cloning and purifying new proteins. Using a DNA based system would also allow for other CRISPR systems to be easily tested, such as base editors (Figure 1G). Gaillochet et al. (2023) for example, utilized a DNA-based high-throughput platform to optimize base editors for rapid implementation in plants. This would have been very difficult indeed if a protein-based platform was used instead. Base editors are an interesting technology, not only because they avoid the induction of double-stranded breaks, but also because they rely on other DNA repair mechanisms (Gu et al., 2021). In bacteria, for example, these chromosomal breaks are typically lethal to the cell, and here base editors offer a very efficient alternative to achieve genome editing (Cui and Bikard, 2016; Zheng et al., 2018; Rodrigues et al., 2021). Another variant in genome editing technology are prime editors. In this approach, a nickase Cas9 is fused to a reverse transcriptase, enabling the incorporation of specific changes into the DNA guided by a prime editing gRNA. Prime editors are generally less efficient than base editors in all systems tested to date. However, prime editors offer more flexibility with regards to the possible genomic changes and make use of yet other repair systems (Chen and Liu, 2023). Given the variability in editing efficiencies across different organisms, further experimentation will be necessary to identify suitable systems for different groups of macroalgae (Figure 1H).
Here we focused on CRISPR based genome editing, but that does not mean that other technologies should be dismissed. TALENs, for example, have advantages in specificity and IP landscape compared to CRISPR (Cloney, 2016; Bhardwaj and Nain, 2021). As the genome editing field is developing at a rapid pace, new tools are also continuously being developed, which could offer benefits in terms of efficiency, adaptability, and precision (Figure 1I).
4 Navigating the applications of gene edited seaweeds
Seaweeds are economically important organisms. Processed red seaweed species in the genus Pyropia have a market value of about 2 billion USD in 2017 (San et al., 2023). Cultivars have been developed for economically important species such as S. japonica, Kappaphycus alvarezii, Ulva ssp., and Gracilaria spp. (Levy and Friedlander, 1990; Hayashi et al., 2007; Su et al., 2020; Lawton et al., 2021). Cultivars have been generated using a variety of techniques, including traditional selection, heavy ion radiation, and ethyl methanesulfonate (EMS) mutagenesis (Niwa et al., 2011; Park and Hwang, 2014; Lee and Choi, 2018; Hwang and Park, 2020; Kong et al., 2023). Nevertheless, whereas land plants which have been cultivated and gradually domesticated for more than 12,000 years (Purugganan, 2019), the earliest record of deliberate seaweed cultivation dates back to about 400 years ago in Korea (Hwang and Park, 2020). Modern seaweed cultivation has only started in the 1940s and currently still relies on relatively few species and cultivars (47 certified cultivars in 2019; Hwang et al., 2019). Breeding campaigns take a significant amount of time, partly because organisms obtained by mutation breeding methods are often burdened by background mutations that need to be removed by extensive backcrossing (Holme et al., 2019). Taken together, a substantial genetic potential is still untapped in a diverse group of organisms with clear commercial value.
Today, the increase in seaweed cultivation comes with a higher prevalence of poorly understood diseases and biofouling epiphytes, combined with abiotic challenges such as ocean acidification and increase in water temperature (Sugumaran et al., 2022). All these stressors impact yield negatively. As seaweed production can contribute to the United Nations sustainable development goals, establishing techniques like genome editing will be crucial to generate, e.g., disease-resistant cultivars (Valero et al., 2017; Hayashi et al., 2020; Sugumaran et al., 2022). Therefore, exploiting genome editing systems may offer a fast way to produce elite strains with desired characteristics. Developing de novo domesticated plants through genome editing is not a pipedream and has been successfully demonstrated multiple times already. In these instances, closely related domesticated species, such as tomato (Solanum lycopersicum) and rice (Oryza sativa), harbored known domestication-related genes (Yu and Li, 2022). Although these types of genetic resources are not as well developed in seaweed species, Genome-Wide Association Studies (GWAS) have the potential to identify interesting candidate genes. These investigations have already yielded genetic regions associated with various yield-related traits in red and brown seaweeds (Liu et al., 2010; Xu et al., 2012; Shan et al., 2015; Avia et al., 2017; Huang and Yan, 2019; Hwang et al., 2019). Traits of interest for cultivar improvement can be disease resistance and yield, but can include nutrient content, production of metabolites or macromolecules, and tolerance to environmental conditions.
5 Discussion
The advent of CRISPR technology has revolutionized the life sciences (van der Oost and Patinios, 2023). Nevertheless, not all fields have been able to reap the promises that this genome editing tool holds. One such field is phycology, which has seen only three published reports on seaweed genome editing to date, despite the ecological and economic importance of these organisms. One major bottleneck is the absence of robust transformation protocols for many seaweed species, preventing the screening of gene editing reagents as is commonly done in contemporary experimental setups. This underscores the need for the development of genetic tools specifically tailored to these organisms. In the Environmental Model Systems (EMS) Project (Faktorová et al., 2020), researchers attempted to optimize transformation protocols in 39 marine protist species, which included green microalgae. The results showed that after optimization exogenous DNA could be successfully delivered and expressed in over 50% of these species. Importantly, no single universally applicable protocol was identified for all species. It should be noted however, that this study was conducted by no less than 113 authors, and a similar collaborative effort will be needed to advance the macroalgae field. Smaller-scale efforts can still benefit from considerable progress made in unicellular model systems such as Chlamydomonas reinhardtii, Cyanidioschyzon merolae or Phaedactylum tricornutum. For example, both Ulva and Chlamydomonas transformant selection relies on the same bleomycin resistance gene (Oertel et al., 2015) and several transit peptides isolated from Chlamydomonas are functional in Ulva (Blomme et al., 2021).
Successful development of genome editing techniques in macroalgae will not only yield insights into the biology of these organisms themselves but will also provide valuable information for understanding the biology of other groups, particularly land plants. This includes insights into the evolution of multicellularity (Coates et al., 2015; De Clerck et al., 2018), organogenesis (Bogaert et al., 2013; 2023), and phytohormone pathways (Bogaert et al., 2022). Additionally, genome editing will also enable the generation of customized strains which can be used in aquaculture. Given the current challenges of higher-intensity cultivation coupled with global change and concomitant effects such as ocean acidification (Sugumaran et al., 2022), breeding new varieties will be an important strategy to future-proof this industry.
In summary, while genome editing in macroalgae is still in its early stages, its potential impact is significant. Future efforts in the field should focus on improving not only genome editing protocols, but also other supporting biotechnological techniques.
Author contributions
JDS: Conceptualization, Project administration, Supervision, Writing–original draft, Writing–review and editing. ECV: Writing–original draft, Writing–review and editing. HL: Funding acquisition, Writing–review and editing. JP: Funding acquisition, Writing–review and editing. JB: Funding acquisition, Supervision, Writing–original draft, Writing–review and editing.
Funding
The author(s) declare that financial support was received for the research, authorship, and/or publication of this article. EV and JB are supported by the Research Foundation - Flanders (FWO, research project G015623N). JP and HL are supported by the Basic Science Research Program of the National Research Foundation of Korea (NRF) funded by the Ministry of Education (RS-2023-00248364).
Conflict of interest
The authors declare that the research was conducted in the absence of any commercial or financial relationships that could be construed as a potential conflict of interest.
Publisher’s note
All claims expressed in this article are solely those of the authors and do not necessarily represent those of their affiliated organizations, or those of the publisher, the editors and the reviewers. Any product that may be evaluated in this article, or claim that may be made by its manufacturer, is not guaranteed or endorsed by the publisher.
References
A. Aguilera, and A. Carreira (Eds.). (2021). Homologous recombination. Humana New York, NY. doi:10.1007/978-1-0716-0644-5
Arimoto, A., Nishitsuji, K., Higa, Y., Arakaki, N., Hisata, K., Shinzato, C., et al. (2019). A siphonous macroalgal genome suggests convergent functions of homeobox genes in algae and land plants. DNA Research 26 (2), 183–192. doi:10.1093/dnares/dsz002
Avia, K., Coelho, S. M., Montecinos, G. J., Cormier, A., Lerck, F., Mauger, S., et al. (2017). High-density genetic map and identification of QTLs for responses to temperature and salinity stresses in the model brown alga Ectocarpus. Sci. Rep. 7 (1), 43241. doi:10.1038/srep43241
Badis, Y., Scornet, D., Harada, M., Caillard, C., Godfroy, O., Raphalen, M., et al. (2021). Targeted CRISPR-Cas9-based gene knockouts in the model brown alga Ectocarpus. New Phytol. 231(5), 2077–2091. doi:10.1111/nph.17525
Bhardwaj, A., and Nain, V. (2021). TALENs—an indispensable tool in the era of CRISPR: a mini review. J. Genet. Eng. Biotechnol. 19 (1), 125. doi:10.1186/s43141-021-00225-z
Blaby-Haas, C. E., and Merchant, S. S. (2019). Comparative and functional algal genomics. Annu. Rev. Plant Biol. 70 (1), 605–638. doi:10.1146/annurev-arplant-050718-095841
Blomme, J., Liu, X., Jacobs, T. B., and De Clerck, O. (2021). A molecular toolkit for the green seaweed Ulva mutabilis. Plant Physiol. 186 (3), 1442–1454. doi:10.1093/plphys/kiab185
Bogaert, K. A., Arun, A., Coelho, S. M., and De Clerck, O. (2013). Brown algae as a model for plant organogenesis BT - plant organogenesis: methods and protocols (I. De Smet (Ed.); pp. 97–125). New York: Humana Press. doi:10.1007/978-1-62703-221-6_6
Bogaert, K. A., Blomme, J., Beeckman, T., and De Clerck, O. (2022). Auxin’s origin: do PILS hold the key? Trends Plant Sci. 27 (3), 227–236. doi:10.1016/j.tplants.2021.09.008
Bogaert, K. A., Zakka, E. E., Coelho, S. M., and De Clerck, O. (2023). Polarization of brown algal zygotes. Seminars Cell and Dev. Biol., 134, 90–102. doi:10.1016/j.semcdb.2022.03.008
Brawley, S. H., Blouin, N. A., Ficko-Blean, E., Wheeler, G. L., Lohr, M., Goodson, H. V., et al. (2017). Insights into the red algae and eukaryotic evolution from the genome of Porphyra umbilicalis (Bangiophyceae, Rhodophyta). Proc. Natl. Acad. Sci. 114 (31), E6361–E6370. doi:10.1073/pnas.1703088114
Bringloe, T. T., Zaparenkov, D., Starko, S., Grant, W. S., Vieira, C., Kawai, H., et al. (2021). Whole-genome sequencing reveals forgotten lineages and recurrent hybridizations within the kelp genus Alaria (Phaeophyceae). J. Phycol., 57(6), 1721–1738. doi:10.1111/jpy.13212
Bryhni, E. (1974). Genetic control of morphogenesis in the multicellular alga Ulva mutabilis: defect in cell wall production. Dev. Biol., 37(2), 273–279. doi:10.1016/0012-1606(74)90148-1
Cai, J., Lovatelli, A., Garrido Gamarro, E., Geehan, J., Lucente, D., Mair, G., et al. (2021). Seaweeds and microalgae: an overview for unlocking their potential in global aquaculture development. Fisheries: FAO. doi:10.4060/cb5670en%0D
Canny, M. D., Moatti, N., Wan, L. C. K., Fradet-Turcotte, A., Krasner, D., Mateos-Gomez, P. A., et al. (2018). Inhibition of 53BP1 favors homology-dependent DNA repair and increases CRISPR–Cas9 genome-editing efficiency. Nat. Biotechnol. 36 (1), 95–102. doi:10.1038/nbt.4021
Cao, X., Kong, F., Sun, B., Yin, J., Ren, H., Yue, H., et al. (2022). A toolbox for constructing a stable genetic transformation platform allowing foreign fragment integration in the genome of neopyropia yezoensis. Front. Mar. Sci. 9. doi:10.3389/fmars.2022.856790
Charpentier, M., Khedher, A. H. Y., Menoret, S., Brion, A., Lamribet, K., Dardillac, E., et al. (2018). CtIP fusion to Cas9 enhances transgene integration by homology-dependent repair. Nat. Commun. 9 (1), 1133. doi:10.1038/s41467-018-03475-7
Chauhan, V. P., Sharp, P. A., and Langer, R. (2023). Altered DNA repair pathway engagement by engineered CRISPR-Cas9 nucleases. Proc. Natl. Acad. Sci. 120 (11), e2300605120. doi:10.1073/pnas.2300605120
Chen, P. J., and Liu, D. R. (2023). Prime editing for precise and highly versatile genome manipulation. Nat. Rev. Genet. 24 (3), 161–177. doi:10.1038/s41576-022-00541-1
Cloney, R. (2016). Patent law and genome engineering: a short guide to a rapidly changing landscape. Mol. Ther. 24 (3), 419–421. doi:10.1038/mt.2016.31
Coates, J. C., Umm-E-Aiman, , and Charrier, B. (2015). Understanding “green” multicellularity: do seaweeds hold the key? Front. Plant Sci. 5, 737. doi:10.3389/fpls.2014.00737
Cock, J. M., Sterck, L., Rouzé, P., Scornet, D., Allen, A. E., Amoutzias, G., et al. (2010). The Ectocarpus genome and the independent evolution of multicellularity in brown algae. Nature 465 (7298), 617–621. doi:10.1038/nature09016
Coelho, S. M., Godfroy, O., Arun, A., Le Corguillé, G., Peters, A. F., and Cock, J. M. (2011). OUROBOROS is a master regulator of the gametophyte to sporophyte life cycle transition in the brown alga Ectocarpus. Proc. Natl. Acad. Sci. 108 (28), 11518–11523. doi:10.1073/pnas.1102274108
Collén, J., Porcel, B., Carré, W., Ball, S. G., Chaparro, C., Tonon, T., et al. (2013). Genome structure and metabolic features in the red seaweed Chondrus crispus shed light on evolution of the Archaeplastida. Proc. Natl. Acad. Sci. 110 (13), 5247–5252. doi:10.1073/pnas.1221259110
Cornish, M. L., O’Leary, S. J. B., and Garbary, D. J. (2013). Phycobilisome composition in Chondrus crispus (Gigartinales, Rhodophyta) from a wild type strain and its vegetatively derived green mutant. Algae 28 (1), 121–129. doi:10.4490/algae.2013.28.1.121
Cruz-Becerra, G., and Kadonaga, J. T. (2020). Enhancement of homology-directed repair with chromatin donor templates in cells. ELife 9, e55780. doi:10.7554/eLife.55780
Cui, L., and Bikard, D. (2016). Consequences of Cas9 cleavage in the chromosome of Escherichia coli. Nucleic Acids Res. 44 (9), 4243–4251. doi:10.1093/nar/gkw223
Daboussi, F., Leduc, S., Maréchal, A., Dubois, G., Guyot, V., Perez-Michaut, C., et al. (2014). Genome engineering empowers the diatom Phaeodactylum tricornutum for biotechnology. Nat. Commun. 5 (1), 3831. doi:10.1038/ncomms4831
De Clerck, O., Kao, S.-M., Bogaert, K. A., Blomme, J., Foflonker, F., Kwantes, M., et al. (2018). Insights into the evolution of multicellularity from the sea lettuce genome. Curr. Biol. 28 (18), 2921–2933. doi:10.1016/j.cub.2018.08.015
Dittami, S. M., Corre, E., Brillet-Guéguen, L., Lipinska, A. P., Pontoizeau, N., Aite, M., et al. (2020). The genome of Ectocarpus subulatus – a highly stress-tolerant brown alga. Mar. Genomics, 52, 100740. doi:10.1016/j.margen.2020.100740
Faktorová, D., Nisbet, R. E. R., Fernández Robledo, J. A., Casacuberta, E., Sudek, L., Allen, A. E., et al. (2020). Genetic tool development in marine protists: emerging model organisms for experimental cell biology. Nat. Methods 17 (5), 481–494. doi:10.1038/s41592-020-0796-x
Gaillochet, C., Peña Fernández, A., Goossens, V., D’Halluin, K., Drozdzecki, A., Shafie, M., et al. (2023). Systematic optimization of Cas12a base editors in wheat and maize using the ITER platform. Genome Biol. 24 (1), 6. doi:10.1186/s13059-022-02836-2
Gao, G., Clare, A. S., Rose, C., and Caldwell, G. S. (2017). Reproductive sterility increases the capacity to exploit the green seaweed Ulva rigida for commercial applications. Algal Res., 24, 64–71. doi:10.1016/j.algal.2017.03.008
Graf, L., Shin, Y., Yang, J. H., Choi, J. W., Hwang, I. K., Nelson, W., et al. (2021). A genome-wide investigation of the effect of farming and human-mediated introduction on the ubiquitous seaweed Undaria pinnatifida. Nature Ecology and Evolution 5 (3). doi:10.1038/s41559-020-01378-9
Greiner, A., Kelterborn, S., Evers, H., Kreimer, G., Sizova, I., and Hegemann, P. (2017). Targeting of photoreceptor genes in Chlamydomonas reinhardtii via zinc-finger nucleases and CRISPR/Cas9. Plant Cell 29 (10), 2498–2518. doi:10.1105/tpc.17.00659
Gu, S., Bodai, Z., Cowan, Q. T., and Komor, A. C. (2021). Base editors: expanding the types of DNA damage products harnessed for genome editing. Gene Genome Ed., 1, 100005. doi:10.1016/j.ggedit.2021.100005
Hayashi, L., Cantarino, d. J. S., and Critchley, A. T. (2020). Challenges to the future domestication of seaweeds as cultivated species: understanding their physiological processes for large-scale production. Adv. Botanical Res., In N. B. T.-A. in B. R. Bourgougnon (Ed.), Seaweeds Around the World: State of Art and Perspectives Academic Press, 95, 57–83. doi:10.1016/bs.abr.2019.11.010
Hayashi, L., de Paula, E. J., and Chow, F. (2007). Growth rate and carrageenan analyses in four strains of Kappaphycus alvarezii (Rhodophyta, Gigartinales) farmed in the subtropical waters of São Paulo State, Brazil. J. Appl. Phycol. 19 (5), 393–399. doi:10.1007/s10811-006-9135-6
He, Y., Shen, S., Yu, D., Wang, Y., Yin, J., Wang, Z., et al. (2021). The Ulva prolifera genome reveals the mechanism of green tides. J. Oceanol. Limnol. 39 (4), 1458–1470. doi:10.1007/s00343-020-0212-5
Hirata, R., Uji, T., Fukuda, S., Mizuta, H., Fujiyama, A., Tabata, S., et al. (2014). Development of a nuclear transformation system with a codon-optimized selection marker and reporter genes in Pyropia yezoensis (Rhodophyta). Journal of Applied Phycology 26 (4), 1863–1868. doi:10.1007/s10811-013-0234-x
Hirano, T., Sato, Y., Ichinose, K., Yamada, M., Hayashi, Y., Fukunishi, N., et al. (2020). Mutant induction in gametophytes of Undaria pinnatifida (Phaeophyceae) by heavy ion beam irradiation. Phycol. Res., 68(1), 63–69. doi:10.1111/pre.12397
Ho, C.-L., Lee, W.-K., and Lim, E.-L. (2018). Unraveling the nuclear and chloroplast genomes of an agar producing red macroalga, Gracilaria changii (Rhodophyta, Gracilariales). Genomics, 110(2), 124–133. doi:10.1016/j.ygeno.2017.09.003
Holme, I. B., Gregersen, P. L., and Brinch-Pedersen, H. (2019). Induced genetic variation in crop plants by random or targeted mutagenesis: convergence and differences. Front. Plant Sci. 10, 1468. doi:10.3389/fpls.2019.01468
Huang, L., and Yan, X. (2019). Construction of a genetic linkage map in Pyropia yezoensis (Bangiales, Rhodophyta) and QTL analysis of several economic traits of blades. PLOS ONE 14 (3), e0209128. doi:10.1371/journal.pone.0209128
Huang, X., Weber, J. C., Hinson, T. K., Mathieson, A. C., and Minocha, S. C. (1996). Transient expression of the GUS reporter gene in the protoplasts and partially digested cells of Ulva lactuca L. (Chlorophyta). Botanica Marina 39 (1–6), 467–474. doi:10.1515/botm.1996.39.1-6.467
Hwang, E. K., and Park, C. S. (2020). Seaweed cultivation and utilization of Korea. Algae 35 (2), 107–121. doi:10.4490/algae.2020.35.5.15
Hwang, E. K., Yotsukura, N., Pang, S. J., Su, L., and Shan, T. F. (2019). Seaweed breeding programs and progress in eastern Asian countries. Phycologia 58 (5), 484–495. doi:10.1080/00318884.2019.1639436
Hwang, G.-H., Yu, J., Yang, S., Son, W. J., Lim, K., Kim, H. S., et al. (2020). CRISPR-sub: analysis of DNA substitution mutations caused by CRISPR-Cas9 in human cells. Comput. Struct. Biotechnol. J., 18, 1686–1694. doi:10.1016/j.csbj.2020.06.026
Ichihara, K., Yamazaki, T., and Kawano, S. (2021). Genome editing using a DNA-free clustered regularly interspaced short palindromic repeats-Cas9 system in green seaweed Ulva prolifera. Phycological. Research 70, 50–56. doi:10.1111/pre.12472
Ichihara, K., Yamazaki, T., and Kawano, S. (2022). Genome editing using a DNA-free clustered regularly interspaced short palindromic repeats-Cas9 system in green seaweed Ulva prolifera. Phycol. Res., 70(1), 50–56. doi:10.1111/pre.12472
Jayavaradhan, R., Pillis, D. M., Goodman, M., Zhang, F., Zhang, Y., Andreassen, P. R., et al. (2019). CRISPR-Cas9 fusion to dominant-negative 53BP1 enhances HDR and inhibits NHEJ specifically at Cas9 target sites. Nat. Commun. 10 (1), 2866. doi:10.1038/s41467-019-10735-7
Jeong, B., Jang, J., and Jin, E. (2023). Genome engineering via gene editing technologies in microalgae. Bioresour. Technol. 373, 128701. doi:10.1016/j.biortech.2023.128701
Jiang, P., Qin, S., and Tseng, C. K. (2003). Expression of the lacZ reporter gene in sporophytes of the seaweed Laminaria japonica (Phaeophyceae) by gametophyte-targeted transformation. Plant Cell Reports 21 (12), 1211–1216. doi:10.1007/s00299-003-0645-2
Jongma, D. N., Campo, D., Dattolo, E., D’Esposito, D., Duchi, A., Grewe, P., et al. (2013). Identity and origin of a slender Caulerpa taxifolia strain introduced into the Mediterranean Sea. Bot. Mar. 56(1), 27–39. doi:10.1515/bot-2012-0175
Kakinuma, M., Coury, D. A., Kuno, Y., Itoh, S., Kozawa, Y., Inagaki, E., et al. (2006). Physiological and biochemical responses to thermal and salinity stresses in a sterile mutant of Ulva pertusa (Ulvales, Chlorophyta). Mar. Biol. 149 (1), 97–106. doi:10.1007/s00227-005-0215-y
Kakinuma, M., Ikeda, M., Coury, D. A., Tominaga, H., Kobayashi, I., and Amano, H. (2009). Isolation and characterization of the rbcS genes from a sterile mutant of Ulva pertusa (Ulvales, Chlorophyta) and transient gene expression using the rbcS gene promoter. Fisheries Science 75 (4), 1015–1028. doi:10.1007/s12562-009-0101-5
Kim, H., Ishidate, T., Ghanta, K. S., Seth, M., Conte, D., Shirayama, M., et al. (2014). A Co-CRISPR strategy for efficient genome editing in Caenorhabditis elegans. Genetics 197 (4), 1069–1080. doi:10.1534/genetics.114.166389
Kong, F., Yin, J., Yu, X., Wang, J., Sun, B., Mao, Y., et al. (2023). Ethyl methanesulfonate mutant library construction in Neopyropia yezoensis to provide germplasm resources for next-generation genome-selection breeding. Front. Mar. Sci. 10. doi:10.3389/fmars.2023.1166370
Kong, F., Zhao, H., Liu, W., Li, N., and Mao, Y. (2017). Construction of plastid expression vector and development of genetic transformation system for the seaweed pyropia yezoensis. Mar. Biotechnol. 19 (2), 147–156. doi:10.1007/s10126-017-9736-x
Konstantakos, V., Nentidis, A., Krithara, A., and Paliouras, G. (2022). CRISPR–Cas9 gRNA efficiency prediction: an overview of predictive tools and the role of deep learning. Nucleic Acids Res. 50 (7), 3616–3637. doi:10.1093/nar/gkac192
Krämer, U. (2015). Planting molecular functions in an ecological context with Arabidopsis thaliana. ELife 4, e06100. doi:10.7554/eLife.06100
Kursar, T. A., van der Meer, J., and Alberte, R. S. (1983). Light-harvesting system of the red alga Gracilaria tikvahiae: I. Biochemical analyses of pigment mutations. Plant Physiol. 73 (2), 353–360. doi:10.1104/pp.73.2.353
Lawton, R. J., Sutherland, J. E., Glasson, C. R. K., and Magnusson, M. E. (2021). Selection of temperate Ulva species and cultivars for land-based cultivation and biomass applications. Algal Res. 56, 102320. doi:10.1016/j.algal.2021.102320
Le Bail, A., Billoud, B., Le Panse, S., Chenivesse, S., and Charrier, B. (2011). ETOILE regulates developmental patterning in the filamentous Brown alga Ectocarpus siliculosus. Plant Cell 23 (4), 1666–1678. doi:10.1105/tpc.110.081919
Lee, H.-J., and Choi, J. (2018). Isolation and characterization of a high-growth-rate strain in Pyropia yezoensis induced by ethyl methane sulfonate. J. Appl. Phycol. 30 (4), 2513–2522. doi:10.1007/s10811-018-1426-1
Lee, J., Yang, E. C., Graf, L., Yang, J. H., Qiu, H., Zelzion, U., et al. (2018). Analysis of the draft genome of the red seaweed gracilariopsis chorda provides insights into genome size evolution in rhodophyta. Mol. Biol. Evol. 35 (8), 1869–1886. doi:10.1093/molbev/msy081
Levy, I., and Friedlander, M. (1990). Strain selection in Gracilaria spp. I. Growth, pigment, and carbohydrates characterization of strains of G. Conferta and G. Verrucosa (rhodophyta, gigartinales). Bot. Mar. 33(4), 339–346. doi:10.1515/botm.1990.33.4.339
Lin, S., Staahl, B. T., Alla, R. K., and Doudna, J. A. (2014). Enhanced homology-directed human genome engineering by controlled timing of CRISPR/Cas9 delivery. ELife 3, e04766. doi:10.7554/eLife.04766
Littler, M. M., and Littler, D. S. (2011). Algae-macro BT - encyclopedia of modern coral reefs: structure, form and process (D. Hopley (Ed.). 30–38). Springer Netherlands. doi:10.1007/978-90-481-2639-2_36
Liu, F., Shao, Z., Zhang, H., Liu, J., Wang, X., and Duan, D. (2010). QTL mapping for frond length and width in Laminaria japonica aresch (laminarales, phaeophyta) using AFLP and SSR markers. Mar. Biotechnol. 12 (4), 386–394. doi:10.1007/s10126-009-9229-7
Liu, T., Wang, X., Wang, G., Jia, S., Liu, G., Shan, G., et al. (2019). Evolution of complex thallus alga: genome sequencing of Saccharina japonica. Front. Genet. 10, 378. doi:10.3389/fgene.2019.00378
Liu, Y., Wu, P., Li, B., Wang, W., and Zhu, B. (2023). Phosphoribosyltransferases and their roles in plant development and abiotic stress response. Int. J. Mol. Sci. 24 (14), 11828. doi:10.3390/ijms241411828
Løvlie, A. (1969). Cell size, nucleic acids, and synthetic efficiency in the wild type and a growth mutant of the multicellular alga Ulva mutabilis Føyn. Developmental Biology 20 (4), 349–367. doi:10.1016/0012-1606(69)90019-0
Marchi, F., and Plastino, E. M. (2020). Codominant inheritance of polymorphic color mutant and characterization of a bisexual mutant of Gracilaria caudata (Gracilariales, Rhodophyta). J. Appl. Phycol. 32 (6), 4385–4398. doi:10.1007/s10811-020-02199-w
Maruyama, T., Dougan, S. K., Truttmann, M. C., Bilate, A. M., Ingram, J. R., and Ploegh, H. L. (2015). Increasing the efficiency of precise genome editing with CRISPR-Cas9 by inhibition of nonhomologous end joining. Nat. Biotechnol. 33 (5), 538–542. doi:10.1038/nbt.3190
Mikami, K. (2013). Current advances in seaweed transformation (G. R. Baptista (Ed.); p. Ch. 13). IntechOpen. doi:10.5772/52978 Available at: https://www.intechopen.com/chapters/44174
Mei, K., Su-juan, W., Yao, L., Da-leng, S., and Cheng-kui, Z. (1998). Transient expression of exogenous gus gene in Porphyra yezoensis (Rhodophyta). Chinese Journal of Oceanology and Limnology 16 (1), 56–61. doi:10.1007/BF02849081
Mikkelsen, N. S., and Bak, R. O. (2023). Enrichment strategies to enhance genome editing. J. Biomed. Sci. 30 (1), 51. doi:10.1186/s12929-023-00943-1
Nakamura, Y., Sasaki, N., Kobayashi, M., Ojima, N., Yasuike, M., Shigenobu, Y., et al. (2013). The first symbiont-free genome sequence of marine red alga, susabi-nori (pyropia yezoensis). PLOS ONE 8 (3), e57122. doi:10.1371/journal.pone.0057122
Nishitsuji, K., Arimoto, A., Higa, Y., Mekaru, M., Kawamitsu, M., Satoh, N., et al. (2019). Draft genome of the brown alga, Nemacystus decipiens, Onna-1 strain: fusion of genes involved in the sulfated fucan biosynthesis pathway. Sci. Rep. 9 (1), 4607. doi:10.1038/s41598-019-40955-2
Nishitsuji, K., Arimoto, A., Iwai, K., Sudo, Y., Hisata, K., Fujie, M., et al. (2016). A draft genome of the brown alga, Cladosiphon okamuranus, S-strain: a platform for future studies of ‘mozuku’ biology. DNA Res. 23 (6), 561–570. doi:10.1093/dnares/dsw039
Niwa, K., Miuea, A., Shin, J.-A., and Aruga, Y. (1993). Characterization and genetic analysis of the violet type pigmentation mutant of porphyra yezoensis ueda (bangiales, rhodophyta). Algae 8 (2), 217–230. http://www.e-algae.org/journal/view.php?number=2010.
Niwa, K., Yamamoto, T., Furuita, H., and Abe, T. (2011). Mutation breeding in the marine crop Porphyra yezoensis (Bangiales, Rhodophyta): cultivation experiment of the artificial red mutant isolated by heavy-ion beam mutagenesis. Aquaculture, 314(1), 182–187. doi:10.1016/j.aquaculture.2011.02.007
Oertel, W., Wichard, T., and Weissgerber, A. (2015). Transformation of Ulva mutabilis (Chlorophyta) by vector plasmids integrating into the genome. J. Phycol., 51(5), 963–979. doi:10.1111/jpy.12336
Osorio, H., Tapia-Reyes, P., Espinoza, D., Laporte, D., González, A., Castro-Nallar, E., et al. (2022). The genome of the marine alga Ulva compressa (chlorophyta) reveals protein-coding genes with similarity to plants and green microalgae, but also to animal, bacterial, and fungal genes. Int. J. Mol. Sci. 23 (13), 7279. doi:10.3390/ijms23137279
Park, C. S., and Hwang, E. K. (2014). Isolation and evaluation of a strain of Pyropia yezoensis (Bangiales, Rhodophyta) resistant to red rot disease. J. Appl. Phycol. 26 (2), 811–817. doi:10.1007/s10811-013-0183-4
Patwary, M. U., and Meer, J. P. van der. (1983). Growth experiments on morphological mutants of Gracilaria tikvahiae (Rhodophyceae). Can. J. Bot. 61 (6), 1654–1659. doi:10.1139/b83-177
Peters, A. F., Scornet, D., Ratin, M., Charrier, B., Monnier, A., Merrien, Y., et al. (2008). Life-cycle-generation-specific developmental processes are modified in the immediate upright mutant of the brown alga Ectocarpus siliculosus. Development 135 (8), 1503–1512. doi:10.1242/dev.016303
Plastino, E. M., Ursi, S., and Fujii, M. T. (2004). Color inheritance, pigment characterization, and growth of a rare light green strain of Gracilaria birdiae (Gracilariales, Rhodophyta). Phycol. Res., 52(1), 45–52. doi:10.1111/j.1440-1835.2003.00324.x
Purugganan, M. D. (2019). Evolutionary insights into the nature of plant domestication. Curr. Biol., 29(14), R705–R714. doi:10.1016/j.cub.2019.05.053
Rodrigues, S. D., Karimi, M., Impens, L., Van Lerberge, E., Coussens, G., Aesaert, S., et al. (2021). Efficient CRISPR-mediated base editing in Agrobacterium spp. Proc. Natl. Acad. Sci. 118 (2), e2013338118. doi:10.1073/pnas.2013338118
San, M. H., Kawamura, Y., Kimura, K., Witharana, E. P., Shimogiri, T., Aye, S. S., et al. (2023). Characterization and organelle genome sequencing of Pyropia species from Myanmar. Sci. Rep. 13 (1), 15677. doi:10.1038/s41598-023-42262-3
Sano, F., Murata, K., and Niwa, K. (2020). Identification, growth, and pigment content of a spontaneous green mutant of Pyropia kinositae (Bangiales, Rhodophyta). J. Appl. Phycol. 32 (3), 1983–1994. doi:10.1007/s10811-020-02085-5
Sato, Y., Hirano, T., Hayashi, Y., Fukunishi, N., Abe, T., and Kawano, S. (2021). Screening for high-growth mutants in sporophytes of Undaria pinnatifida using heavy-ion beam irradiation. CYTOLOGIA 86 (4), 291–295. doi:10.1508/cytologia.86.291
Schaff, D. A. (1994). The adenine phosphoribosyltransferase (APRT) selectable marker system. Plant Sci., 101(1), 3–9. doi:10.1016/0168-9452(94)90159-7
Schubert, M., Thommandru, B., Woodley, J., Turk, R., Yan, S., Kurgan, G., et al. (2021). Improved methods and optimized design for CRISPR Cas9 and Cas12a homology-directed repair. Sci. Rep. 11, 19482. doi:10.1101/2021.04.07.438685
Shan, T., Pang, S., Li, J., Li, X., and Su, L. (2015). Construction of a high-density genetic map and mapping of a sex-linked locus for the brown alga Undaria pinnatifida (Phaeophyceae) based on large scale marker development by specific length amplified fragment (SLAF) sequencing. BMC Genomics 16 (1), 902. doi:10.1186/s12864-015-2184-y
Shen, Y., Motomura, T., Ichihara, K., Matsuda, Y., Yoshimura, K., Kosugi, C., et al. (2023). Application of CRISPR-Cas9 genome editing by microinjection of gametophytes of Saccharina japonica (Laminariales, Phaeophyceae). J. Appl. Phycol. 35 (3), 1431–1441. doi:10.1007/s10811-023-02940-1
Sizova, I., Greiner, A., Awasthi, M., Kateriya, S., and Hegemann, P. (2013). Nuclear gene targeting in Chlamydomonas using engineered zinc-finger nucleases. Plant J. 73(5), 873–882. doi:10.1111/tpj.12066
Song, Q., Daozhan, Y., Peng, J., Changying, T., and Chengkui, Z. (2003). Stable Expression of lacZ Reporter Gene in Seaweed Undaria pinnatifida. Gaojishu Tongxun 13 (7), 87–89.
Song, Q., Peng, J., Xi-hua, W., Cheng-kui, Z., and Tseng, C. K. (1998). A transformation model for Laminaria Japonica (Phaeophyta, Laminariales. Chinese Journal of Oceanology and Limnology 16, 50–55.
Stock, W., Blomme, J., Xiaojie, L., and De Clerck, O. (2024). Chapter 2–evolution, biology, and genetics of seaweeds (D. I. Hefft, and N. Adetunji (Eds.) 19–34). C. O. B. T.-A. of S. in F. Elsevier. doi:10.1016/B978-0-323-91803-9.00008-1
Strassert, J. F. H., Irisarri, I., Williams, T. A., and Burki, F. (2021). A molecular timescale for eukaryote evolution with implications for the origin of red algal-derived plastids. Nat. Commun. 12 (1), 1879. doi:10.1038/s41467-021-22044-z
Su, L., Shan, T. F., Li, J., Pang, S. J., Leng, X. F., Zhang, Y., et al. (2020). Aquaculture of the hybrid cultivars of Saccharina japonica: removing the obstacle of sori production by photoperiodic control. Aquaculture 519, 734917. doi:10.1016/j.aquaculture.2019.734917
Sugumaran, R., Padam, B. S., Yong, W. T., Saallah, S., Ahmed, K., and Yusof, N. A. (2022). A retrospective review of global commercial seaweed production—current challenges, biosecurity and mitigation measures and prospects. Int. J. Environ. Res. Public Health 19 (12), 7087. doi:10.3390/ijerph19127087
Uji, T., Hirata, R., Fukuda, S., Mizuta, H., and Saga, N. (2014). A Codon-Optimized Bacterial Antibiotic Gene Used as Selection Marker for Stable Nuclear Transformation in the Marine Red Alga Pyropia yezoensis. Marine Biotechnology 16 (3), 251–255. doi:10.1007/s10126-013-9549-5
Valero, M., Guillemin, M.-L., Destombe, C., Jacquemin, B., Gachon, C. M. M., Badis, Y., et al. (2017). Perspectives on domestication research for sustainable seaweed aquaculture. Perspect. Phycol. 4 (1), 33–46. doi:10.1127/pip/2017/0066
van de Kooij, B., and van Attikum, H. (2022). Genomic reporter constructs to monitor pathway-specific repair of DNA double-strand breaks. Front. Genet. 12, 809832. doi:10.3389/fgene.2021.809832
van der Oost, J., and Patinios, C. (2023). The genome editing revolution. Trends Biotechnol. 41 (3), 396–409. doi:10.1016/j.tibtech.2022.12.022
Wang, J., Jiang, P., Cui, Y., Deng, X., Li, F., and Liu, J. (2010). Genetic transformation in Kappaphycus alvarezii using micro-particle bombardment: A potential strategy for germplasm improvement. Aquaculture International 18 (6), 1027–1034. doi:10.1007/s10499-010-9320-0
Wang, S., Lin, L., Shi, Y., Qian, W., Li, N., Yan, X., et al. (2020). First draft genome assembly of the seaweed sargassum fusiforme. Front. Genet. 11, 590065. doi:10.3389/fgene.2020.590065
Woo, J. W., Kim, J., Kwon, S.Il, Corvalán, C., Cho, S. W., Kim, H., et al. (2015). DNA-free genome editing in plants with preassembled CRISPR-Cas9 ribonucleoproteins. Nat. Biotechnol. 33 (11), 1162–1164. doi:10.1038/nbt.3389
Xu, Y., Xie, C., Chen, C., Ji, D., and Gao, Y. (2012). Genetic analyses of six quantitative traits of a doubled haploid population of Porphyra haitanensis Chang et Zheng (Bangiales, Rhodophyta). J. Appl. Phycol. 24 (1), 89–96. doi:10.1007/s10811-011-9653-8
Yan, X.-H., Fujita, Y., and Aruga, Y. (2000). Induction and characterization of pigmentation mutants in Porphyra yezoensis (Bangiales, Rhodophyta). J. Appl. Phycol. 12 (1), 69–81. doi:10.1023/A:1008129119065
Yu, H., and Li, J. (2022). Breeding future crops to feed the world through de novo domestication. Nat. Commun. 13 (1), 1171. doi:10.1038/s41467-022-28732-8
Zhang, Y., Jiang, P., Gao, J., Liao, J., Sun, S., and Shen, Z. (2008). Recombinant expression of rt-PA gene (encoding Reteplase) in gametophytes of the seaweed Laminaria japonica (Laminariales, Phaeophyta). Science in China Series C: Life Sciences 51 (12), 1116–1120. doi:10.1007/s11427-008-0143-4
Zentout, S., Smith, R., Jacquier, M., and Huet, S. (2021). New methodologies to study DNA repair processes in space and time within living cells. Front. Cell Dev. Biol. 9, 730998. doi:10.3389/fcell.2021.730998
Zhang, N., Roberts, H. M., Van Eck, J., and Martin, G. B. (2020). Generation and molecular characterization of CRISPR/Cas9-Induced mutations in 63 immunity-associated genes in tomato reveals specificity and a range of gene modifications. Front. Plant Sci. 11, 10. doi:10.3389/fpls.2020.00010
Zheng, K., Wang, Y., Li, N., Jiang, F.-F., Wu, C.-X., Liu, F., et al. (2018). Highly efficient base editing in bacteria using a Cas9-cytidine deaminase fusion. Commun. Biol. 1 (1), 32. doi:10.1038/s42003-018-0035-5
Keywords: genome editing, CRISPR, macroalgae, seaweed biotechnology, seaweed breeding, reverse genetics
Citation: De Saeger J, Coulembier Vandelannoote E, Lee H, Park J and Blomme J (2024) Genome editing in macroalgae: advances and challenges. Front. Genome Ed. 6:1380682. doi: 10.3389/fgeed.2024.1380682
Received: 02 February 2024; Accepted: 13 February 2024;
Published: 06 March 2024.
Edited by:
Prasenjit Saha, Meiogenix Inc., United StatesReviewed by:
Rui Zhang, Chinese Academy of Sciences (CAS), ChinaCopyright © 2024 De Saeger, Coulembier Vandelannoote, Lee, Park and Blomme. This is an open-access article distributed under the terms of the Creative Commons Attribution License (CC BY). The use, distribution or reproduction in other forums is permitted, provided the original author(s) and the copyright owner(s) are credited and that the original publication in this journal is cited, in accordance with accepted academic practice. No use, distribution or reproduction is permitted which does not comply with these terms.
*Correspondence: Jonas De Saeger, Sm9uYXMuZGVzYWVnZXJAdWdlbnQuYmU=; Jonas Blomme, Sm9uYXMuYmxvbW1lQHVnZW50LmJl