- 1Genethon, Evry, France
- 2Integrare Research Unit UMR_S951, Université Paris-Saclay, University Evry, Inserm, Genethon, Evry, France
Lessons learned from decades-long practice in the transplantation of hematopoietic stem and progenitor cells (HSPCs) to treat severe inherited disorders or cancer, have set the stage for the current ex vivo gene therapies using autologous gene-modified hematopoietic stem and progenitor cells that have treated so far, hundreds of patients with monogenic disorders. With increased knowledge of hematopoietic stem and progenitor cell biology, improved modalities for patient conditioning and with the emergence of new gene editing technologies, a new era of hematopoietic stem and progenitor cell-based gene therapies is poised to emerge. Gene editing has the potential to restore physiological expression of a mutated gene, or to insert a functional gene in a precise locus with reduced off-target activity and toxicity. Advances in patient conditioning has reduced treatment toxicities and may improve the engraftment of gene-modified cells and specific progeny. Thanks to these improvements, new potential treatments of various blood- or immune disorders as well as other inherited diseases will continue to emerge. In the present review, the most recent advances in hematopoietic stem and progenitor cell gene editing will be reported, with a focus on how this approach could be a promising solution to treat non-blood-related inherited disorders and the mechanisms behind the therapeutic actions discussed.
1 Introduction
Hematopoietic stem and progenitor cells (HSPCs) are the only cells with the ability to self-renew throughout a person’s lifetime and give rise to all the blood components including leukocytes, platelets and erythrocytes. Rekers and others first explored hematopoietic stem cell transplantation (HSCT) in the fifties, with the demonstration that intravenously injected bone marrow cells prevent the death of irradiated mice by reestablishing blood cell production (Rekers et al., 1950). These studies on animal models were soon translated into clinical applications for patients, especially with the extensive work of the Nobel Prize winner, Dr. E. Donnall Thomas, on allogeneic HSCT (Thomas et al., 1957; Thomas et al., 1977; Thomas et al., 1979), together with the discovery of HLA histocompatibility between donors and recipients (Dausset and Brecy, 1957).
Thus, bone marrow transplantation from healthy HLA-compatible donor has been used to correct genetic disorders such as cytopenias hemoglobinopathies or primary immune deficiencies, by providing HSCs free of pathogenic mutations that can engraft into a myelosupressed host to reconstitute a functional blood and immune system. The procedure of HSC transplantation has also been radically improved by discovering that HSPCs are not only found in high concentrations in the bone marrow, but also in peripheral blood after mobilization (Szade et al., 2018). Although allogeneic HSCT revolutionized medicine even with a HLA match-donor (with the exception of syngeneic twins) and an adapted conditioning protocol, there is always a substantial risk for graft-versus-host-disease (GvHD) in treated patients. GvHD occurs when immune competent T cells in the donated tissue recognize the recipient as non-self. The resulting immune response activates donor T cells to gain cytolytic capacity then attack the recipient to eliminate foreign antigen(s)-bearing cells (Zeiser and Blazar, 2017). . However, as some patients do not have a HLA-compatible donor, the concept of autologous HSCT using ex-vivo gene-modified HSPC has been developed by scientists over the years. The first successful attempt of gene therapy using autologous HSCT was performed using gamma retroviral vectors (RVs) in X-linked severe combined immunodeficiency (SCID-X1) and adenosine deaminase deficiency (ADA-SCID) (Bordignon et al., 1995; Cavazzana-Calvo et al., 2000). Unfortunately, the excitement from the promising early results was dampened by the presence of genotoxic events in some of the patients treated with these vectors (Hacein-Bey-Abina et al., 2003; Howe et al., 2008; Cavazzana et al., 2019). Scientists quickly reacted with the development of vectors that allowed robust gene correction in HSPCs, while decreasing insertional mutation events (Galy, 2017). Self-inactivating lentiviral vectors (SIN-LVs) derived from the human immunodeficiency virus (Zufferey et al., 1998) were proved to be more efficient and safer than RVs, and are nowadays the vectors of choice for HSPCs gene therapy, showing long-term benefits in treated patients (Eichler et al., 2017; Kohn et al., 2021; Magnani et al., 2022; Magrin et al., 2022).
More recently, the field of gene and cell therapy has been rattled by the discovery of specific nucleases able to precisely cut genomic DNA. Three main platforms using this strategy exist: Zinc-finger nucleases (ZFNs), Transcription activator-like effector nucleases (TALENs) and Clusters of regularly interspaced short palindromic repeats (CRISPR)/Cas9 nucleases which became the most exploited interface over the past decade. All these nuclease-based approaches rely on the intrinsic ability of the cells to repair double strand breaks (DSBs). . Additionally, the field of gene editing has also seen other tools emerge lately, such as prime editing or base editing (BE), which exploit the specific DNA recognition capacity of CRISPR/Cas9 but use different enzymes to modify the DNA. Most of these tools have already been adopted to gene-modify HSPCs ex vivo in an attempt to treat inherited hematopoietic disorders and some studies already led to clinical trials for β-thalassemia and severe sickle cell anemia (Frangoul et al., 2021).
This review will focus on the latest progress for HSPCs gene editing and transplantation, in terms of cells mobilization, selection, culture conditions and the conditioning of patients. We will also give an overview of all the gene-editing tools available and how to efficiently and safely deliver them into HSPCs. And most importantly, we will discuss how gene edited HSPCs could be used not only for blood- or immune-related genetic disorders, but as a vehicle to bring functional proteins to affected tissues in other types of inherited multisystemic disorders.
2 Advances in HSPC mobilization, culture and patients conditioning
HSPCs have the ability to reconstitute a new hematopoietic system when transplanted into an immunodepleted patient (Wilkinson et al., 2020). Advances in term of HSPC mobilization and culture together with patient conditioning have been essential to make HSCT safer and more efficient.
2.1 Advances in mobilization
HSPCs can be harvested in two ways, by BM aspiration from the pelvic crest or by leukapheresis after induced mobilization into the peripheral circulation. Mobilization is preferred because it is a less stressful procedure and allows for a generally higher stem cell yield, reduced graft failure rates, and faster reconstitution (Gertz, 2010). The recombinant human G-CSF (rh-G-CSF) is the cytokine of choice used for mobilization (Bendall and Bradstock, 2014). This treatment results in a 50–100 fold expansion of the circulating HSPC pool (Holig et al., 2009). In cancer patients, or when higher concentrations of collected HSPCs are necessary, the use of chemotherapy in combination with G-CSF is usually preferred. However, there are major disadvantages linked to the use of chemotherapy, such as toxicity, bone marrow damage and higher costs (Hubel, 2019).
Of note, there are certain conditions where G-CSF cannot be used to mobilize HSPCs, such as sickle cell disease (Fitzhugh et al., 2009). In this particular case, G-CSF can be substituted by the small molecule AMD3100 (Plerixafor) (Esrick et al., 2018). Interestingly, when Plerixafor is combined with G-CSF treatment; it triples the concentration of isolated CD34+ cells compared to the administration of G-CSF alone (Liles et al., 2005). Besides the treatments described above, multiple compounds able to increase the number of circulating HSPCs have been evaluated in preclinical models: sphingosine-1-phosphate (Juarez et al., 2012), inducers of MMP-9 (Pelus et al., 2004), or inhibitors of EGFR (Ryan et al., 2010) and Cdc42 (Liu et al., 2019). It has also been reported that treatment with cobalt protoporphyrin (CoPP) induces an endogenous stimulation of cytokines, reaching a better HSPCs mobilization than treatment with G-CSF alone (Szade et al., 2019). In 2018, Hoggatt and others demonstrated that GROβ, a CXCR2 agonist, was able to induce a rapid HSPCs mobilization (within minutes) when used together with the CXCR4 antagonist AMD3100, resulting in a higher engraftment capacity of the isolated cells with respect to those mobilized via G-CSF (Hoggatt et al., 2018).
2.2 HSPC transplantation without genotoxic conditioning
To ensure the engraftment of transplanted HSPCs, the standard procedure requires a genotoxic conditioning to ablate the endogenous HSPCs and immune system cells, providing the conditions and the “space” that allow for the creation of a donor HSPCs niche and to remove the diseased HSC and progeny. The chemotherapeutic drug commonly used in these settings is busulfan, an alkylating agent with myelosuppressive effects (Bernardo and Aiuti, 2016). However, in spite of low-intensity conditioning regimens which have been developed, there are still negative side effects of busulfan potentially at long term (i.e. organ toxicity, infertility and risk of secondary malignancy), therefore efforts to develop alternative, safer and more specific conditioning regimes have been made (Chanut et al., 2021). The use of specific antibodies against antigens specifically expressed by HSPCs has been the main focus for novel approaches to ablate the resident HSPCs niche. Of particular interest is a study by Czechowicz and others, which showed an almost total depletion of resident HSPCs in immunodeficient mice using ACK2, an antibody that blocks c-kit function (Czechowicz et al., 2007). Similar effects were achieved when using a combination of monoclonal antibodies against c-kit (CD-117) and CD47 (Chhabra et al., 2016), or with antibody–drug conjugates composed of anti-CD117 antibodies and saporin, a ribosome-inactivating protein (Czechowicz et al., 2019). Of note, anti-CD117 conditioning has been already used for allogeneic HSCT in a clinical trial for treating severe combined immunodeficiency (SCID) patients (Agarwal et al., 2020).
Other non-genotoxic conditioning strategies that are of extreme interest have been developed. Pharmacological inhibition of Cdc42 efficiently mobilizes HSPCs from BM in mice, allowing for the engraftment of HSPCs with a better reconstitution potential compared to AMD3100-mediated conditioning (Liu et al., 2019). Interestingly, in a study by Taya and others, removal of dietary valine in mice allowed for host HSPCs depletion supporting efficient engraftment of the transplanted HSPCs (Taya et al., 2016).
2.3 Ex-vivo expansion of HSPCs
Increasing the number of transplanted corrected HSPCs is determinant for improving the engraftment success rate, especially in settings where there is no selective advantage over the non-corrected HSPCs. A concept confirmed by HSCT clinical trials for Wiskott Aldrich syndrome (Hacein-Bey Abina et al., 2015), or β-thalassemia, where a higher percentage of gene-corrected cells correlated with better outcomes (Thompson et al., 2018). Improving HSPCs viability, function and cell expansion potential, during ex vivo manipulation is also a determinant step for increasing engraftment success rates. The Cooke group, discovered a purine derivative named StemRegenin 1 (SR1) able to promote a 50-fold ex vivo expansion of CD34+ cells, resulting in a 17-fold increase of engrafted cells in immunodeficient mice (Boitano et al., 2010). Another chemical compound of interest is the pyrimidoindole derivative named UM171, which highly promotes human HSC self-renewal and ex vivo expansion (Fares et al., 2014; Cohen et al., 2020). HSPCs ex vivo expansion via three-dimensional culture proved to be also an effective strategy to increase primitive CD34+ cells expansion, maintaining a high self-renewal potential and allowing for long-term reconstitution in immunocompromised mice (Bai et al., 2019).
3 Gene editing tools
Targetable nucleases can be programmed to induce changes in a specific sequence throughout the genome in a very specific fashion, making them useable gene-editing tools. Examples of these enzymes that have been successfully engineered for use as gene-editing tools in mammalian cells include ZFN, TALENs, and CRISPR/Cas9 system. After the generation of a DSB at a specific locus, the endogenous DSB-repair machinery of the cell can be exploited to insert a new sequence using a donor DNA template. The current genome editing toolbox also includes methods that do not rely on endogenous repair mechanisms, such as prime editors and base editors, which can modify the host’s sequence without induction of DSBs. The intrinsic features of each editing tool may represent strengths or weaknesses depending on the desired application and must be carefully considered when choosing the gene therapy strategy. A description of the currently available gene editing tools and possible advantages and disadvantages in the design of a therapeutic treatment will be briefly discussed in this section.
3.1 Zinc-finger nucleases
ZFNs are composed of two zinc-finger domains fused with FokI endonuclease that can be engineered to target a specific genomic region (Kim et al., 1996; Smith et al., 2000). Recognition of the sequence by an individual Zinc finger monomer does not activate the nuclease activity, the DSB is only generated when both zinc-finger domains bind the target sequence and dimerization occurs. Although this feature confers ZFNs a high specificity, the risk for off-target effects is still present (Pattanayak et al., 2011). However, strategies to reduce ZFN off-target effects have been developed, (Liu et al., 2015a; Miller et al., 2019), and despite the design difficulties, ZFNs remain a highly specific tool, depending on the target region (Gabriel et al., 2011; Isalan, 2011).
3.2 TALEN
Transcription activator-like proteins (TALEs) are composed by repetitive amino acid (aa) sequences that acts as transcription factors (Bogdanove and Voytas, 2011). TALE nucleases (TALENs) have been engineered as gene editing tools by the fusion of a modular TALE repeats with a FokI domain. Same as ZFNs, dimerization of two TALEN monomers is necessary for FokI activation and DSB generation (Boch et al., 2009; Moscou and Bogdanove, 2009; Cermak et al., 2011; Li et al., 2011; Miller et al., 2011; Gaj et al., 2013). TALENs have also shown a low off-target effects rate in human pluripotent stem cell clones (Veres et al., 2014). However, since TALEs are repetitive sequences that can undergo homologous recombination, their delivery via lentiviral vectors is challenging. Additionally, TALENs have affinity to sequences with low G content and the target sequence must begin with a T, substantially restraining the availability of targeting sites (Bogdanove and Voytas, 2011).
3.3 CRISPR/Cas
CRISPR/Cas is a naturally occurring genome editing system that bacteria and archaea use as an immune defense against invasive nucleic acids. (Barrangou et al., 2007; Brouns et al., 2008; Sorek et al., 2013). Later, the system was adapted as a gene editing tool by customization of a guide RNA (gRNA) for recognition of the desired genomic sequence and the coupling with high efficient Cas proteins (Jinek et al., 2012). The most commonly used Cas proteins are the SpCas9 (from Streptococcus pyogenes) and the Cas12/CpfI (from Acidaminococcus and Lachnospiraceae) (Cong et al., 2013; Mali et al., 2013; Zetsche et al., 2015). The presence of a protospacer adjacent motif (PAM) sequence immediately downstream of the target site is necessary for effective recognition and cleavage (Hsu et al., 2013; Sternberg et al., 2014). However, CRISPR/Cas9 variants where the PAM sequence presence is dispensable have been developed (Walton et al., 2020; Xie et al., 2022). Addition of a stable hairpin in the gRNA sequence also appears to allow efficient editing regardless of PAM presence in the sequence composition (Riesenberg et al., 2022). For more details about new CRISPR/Cas based tools, please refer to the review by Hu and Li (Hu and Li, 2022).
3.3.1 Base editing
Base editors (BEs) are fusion proteins composed of a catalytically impaired Cas9 (deadCas9 or dCas9) and a single-stranded DNA deaminase, which are able to introduce single-nucleotide variants in the DNA of living cells, without generating DSBs (Komor et al., 2016; Gaudelli et al., 2017; Anzalone et al., 2020). Gene editing strategies that generate DSBs present with a risk for genotoxic events (Kosicki et al., 2018; Cullot et al., 2019; Blattner et al., 2020). Base editing represents a safer option because modification of the DNA sequence occurs without inducing DSBs. Additionally, BE works at any stage of the cell cycle, opposite to gene editing techniques relying on DSBs repair, which are more effective on dividing cells (Zhang et al., 2017). On the other hand, the action of BEs is restricted to the change of a single nucleotide. Although this approach could theoretically treat all diseases characterized by a single point mutation, it is limited by PAM sequence preferences and is restricted by the width of the editing. It is also characterized by high precision, with BEs that can discriminate between the target base and multiple bystander bases within a narrow active window (Jeong et al., 2020). Recent improved versions of classicals BEs are described in the review by Reshetnikov et al. (2022).
3.3.2 Prime editing
Prime editors (PEs) are protein complexes consisting of a PAM-independent Cas9 nickase, a reverse transcriptase and a prime editing gRNA (pegRNA) (Anzalone et al., 2019). PEs present with less off-target activity compared to CRISPR/Cas9, albeit with lower editing efficiency (Liu et al., 2021; Chen et al., 2021; Gao et al., 2022). However, PE-mediated correction of pathologic phenotype has been demonstrated (Zhang H. et al., 2022a; Zhang H. et al., 2022b). Different strategies to improve PEs have been developed, either stabilizing the pegRNA (Liu et al., 2021; Zhang G. et al., 2022), or manipulating repair pathways (Chen et al., 2021; Ferreira da Silva et al., 2022), or a combination of both (Chen et al., 2021; Jiang and Yao, 2022). Furthermore, by inserting specific same-sense mutations in the reverse transcription template of pegRNA or by altering the pegRNA secondary structure, efficiency could be significantly improved (Li et al., 2022).
4 Methods of delivery of the gene editing machinery into HSPCs
The main challenge of delivering of gene editing tools together with the corrective DNA template into HSPCs ex vivo or in vivo is obtaining HSPCs editing in the most efficient and safe manner to procure HSPC’s progeny with the corrected DNA information. To avoid toxicity and off-target activity, the enzyme needs to be optimized to create a specific DSB in the shortest possible period. The choice of carrier depends on the repair pathways the cells will be using during the editing process, the level of editing that needs to be achieved, the toxicity tolerance of the treated cells and, in the case of homology-directed based approaches, the size of donor template (Table 1).
4.1 Physical delivery of gene editing tools into HSPCs
Mobilization and isolation of HSPCs allowing their ex vivo manipulation offers a clear advantage for genetic manipulations. Compared to DNA vectors, mRNA increases the transfection efficiency of inactive cells, does not present the risk of insertional mutagenesis in the host’s genome, allows for a faster protein production with higher expression levels control, and does not contain additional exogenous sequences, such as antibiotic resistances or viruses-derived promoters (Guan and Rosenecker, 2017; Baptista et al., 2021). A lot of efforts have also been deployed to improve stability and translational efficiency of the mRNA filament (Korner and Wahle, 1997; Jemielity et al., 2003; Gustafsson et al., 2004; Kwon et al., 2018). as well as reducing the potential immunogenicity of transcribed mRNAin vitro (Triana-Alonso et al., 1995; Wu et al., 2020) (Kariko et al., 2008; Andries et al., 2015) (Mu et al., 2018) (Vaidyanathan et al., 2018) in vitro (Nelson et al., 2020). Delivery of transcribed mRNA coding for ZFN (DiGiusto et al., 2016; Conway et al., 2019), TALEN (Lux et al., 2019) and CRISPR-Cas9 (Hendel et al., 2015) into HSPCs showed great promises in terms of efficacy and safety. Finally, nucleases can also be delivered directly as proteins (Liu et al., 2015b), providing rapid action and fast turnover, hence reducing off-target effects. Nowadays, the gold standard to efficiently and safely perform CRISPR-Cas9 gene editing in HSPCs is nucleofecting both, the mRNA (guide-RNA) and the nuclease (Cas9) as pre-assembled ribonucleotide-protein complexes (RNP) (Gundry et al., 2016; Vakulskas et al., 2018; Lattanzi et al., 2019; Rocca et al., 2020; Bloomer et al., 2021). In addition, transient genome editing, reduces off-target effects, insertional mutagenesis, and immune responses, besides allowing for high editing efficiencies (Chandrasekaran et al., 2018).
4.2 Chemical delivery into HSPCs
Chemical delivery of gene editing tools is only of interest for in vivo delivery into HSPCs (not achievable by electroporation). In some cases, the patient’s condition might be too severe to consider a myeloablative treatment followed by HSCT, or the opposite, a condition too mild to consider such an invasive procedure. A chemical approach could also significantly reduce costs of HSPC-based therapies, making it more accessible to less privileged populations.
Recently, a c-kit nanoparticle that enables a partial in vivo targeting of HSPCs has been developed (Cannon et al., 2021). Another promising approach is the use of lipid nanoparticles (LNPs) for mRNA delivery to target cells in vivo (Mukai et al., 2022). LNPs are known to have high affinity for the hepatic tissue, but their surface can easily be crosslinked to antibodies to redirect their cellular specificity for the desired in vivo targeting (Rosenblum et al., 2020; Tombacz et al., 2021). The Dahlman laboratory developed a method using barcoding and bioinformatics to study biodistribution of LNPs in vivo and selected a LNP named BM1 able to target bone marrow endothelial cells (Sago et al., 2018a; Sago et al., 2018b). Finally, it has been reported by Weissman and collaborators that a CD90-targeting LNPs can transfect about 4% of CD34+ cells from bone marrow stem cell preparations (Cannon et al., 2021).
4.3 Viral and “viral-like” delivery into HSPCs
Different viral platforms that can be used to bring nucleases and donor template into HSPCs: Adenoviral vectors (AdVs), adeno-associated viral vectors (AAVs) and lentiviral vectors (LVs). The choice of the viral vector type depends on the size of the genetic material to carry and on the virus ability to transduce either quiescent or dividing cells.
AdVs are double-stranded DNA viruses capable of packaging up to 37 kb of DNAExtensive studies trying to optimize helper-dependent AdVs for transducing CD34+ have been performed not only because of their large packaging capacity, but also for the low cost for vector manufacturing, the episomal expression and the absence of viral gene expression (Li and Lieber, 2019). Based on the observation that HIV patients had non-detectable viral levels after HSCT with donor cells homozygous for CCR5delta32 (Hutter et al., 2009; Gupta et al., 2019), the targeting of HSPCs using AdVs able to inactivate CCR5 using ZNF or CRISPR/Cas9 have been the focus of several studies in the context of HIV-1/AIDS therapies (Li et al., 2013; Xu et al., 2017). In a recent study, the Lieber laboratory reported a novel approach to treat β-Hemoglobinopathies in CD46/β-YAC transgenic mice using AdVs and a base editing approach (Li et al., 2021).
AAVs have the advantages of being non-pathogenic, to efficiently infect non-dividing cells and, they do not integrate in the host genome. The main limitation of AAVs is their low packaging capacity (4.8 kb). However, this can be improved with the development of dual-AAV donor vector systems (Bak and Porteus, 2017). Serotype 6 is the AAV with the higher tropism for HSPCs (Yang et al., 2020). Currently, most of gene editing approaches for therapeutic protein expression need the usage of both gene editing tools and AAV6 (Rai et al., 2020; Brault et al., 2021; Cromer et al., 2021; Wilkinson et al., 2021).
LVs possess several advantages for HSPC manipulation: a large cargo capacity (∼10 kb), a stable integration into host genome, they can infect both dividing and non-dividing cells, no expression of viral proteins after transduction, the ability to deliver complex genetic elements, and a safer integration site profile (Sakuma et al., 2012). Researchers applied several genetic modifications over the years to make LVs safer for gene therapy, leading to the development of self-inactivating LVs (SIN-LVs) (Miyoshi et al., 1998; Zufferey et al., 1998) and integrative-deficient LVs (IDLVs) (Philippe et al., 2006; Yanez-Munoz et al., 2006). IDLVs have been successfully used for ZFNs delivery to the target cells and the corrective DNA template to HSPCs in the context of PIDs (Lombardo et al., 2007; Abdul-Razak et al., 2018).
Although AAV6 and IDLVs are powerful platforms to deliver DNA donor templates, both can persist in targeted tissue for long periods and may lead to unwanted effects (Cousin et al., 2019; Bolt et al., 2021). As an alternative, single-stranded oligodeoxynucleotides (ssODNs) can be used (short oligos <200bp with 30-60bp homology harms). They showed great potential for CRISPR/Cas9/HDR in HPSCs (Antony et al., 2018; Romero et al., 2019), having though the disadvantage of only permitting small insertion changes (point mutations or short fragments), however the technology is improving extremely fast (Roth et al., 2018).
To deliver gene-editing tools in vivo, a research group developed a viral-like approach named nanoblades, based on HIV or murine leukemia virus. The strategy consists in generating virus-like particles with the Gag protein fused to Cas9 and gRNA expression cassettes (Mangeot et al., 2019). After, these particles are pseudotyped with glycoproteins to specifically target HSPCs (Mangeot et al., 2019; Gutierrez-Guerrero et al., 2021). In 2019, the Atala laboratory developed a system able to package up to 100 copies of Staphylococcus aureus Cas9 (SaCas9) mRNA in each LV-like bionanoparticle (LVLP) (Lu et al., 2019). Finally, the Liu laboratory reported the development of DNA-free virus-like particles (eVLPs) able to efficiently package and deliver base editor or Cas9 ribonucleoproteins to different cell types using different glycoproteins (Banskota et al., 2022).
5 Methods to improve HDR-mediated gene editing in HSPCs
DNA DSBs generated by editing nucleases can be repaired via different pathways in mammalian cells depending on the cell cycle phase but also on the DNA end structures induced by specific programmable nucleases (i.e., blunt or cohesive ends). Quiescent HSPCs rely mainly on the NHEJ pathway to repair DSBs, because it is the only one operating during the G0/G1 phases of the cell cycle. However, this pathway is not homology dependent and can lead to sequence modifications at the repair junctions. On the other hand, MMEJ is active during the S/G2 phases of the cell cycle but can generate even larger indels with respect to NHEJ. In presence of a DNA template, targeted integration is possible with both NHEJ and MMEJ, but these are still not error-free alternative pathways. Single-strand annealing (SSA) and homologous recombination (HR) also occur during S/G2. . HR is considered error-free and consequently it is the DBS repair pathway of choice for gene editing. However, as mentioned earlier, HSPCs are quiescent cells and HR frequency is very low in these cells. In this chapter, we are going to review the most recent strategies developed to trick this biological system and increase homologous directed repair (HDR) frequency in HSPCs.
5.1 Choice of DNA donor template
The choice of delivery method of the donor DNA template alone (i.e. IDLVs, AAV6 or ssODNs) has been shown to influence HDR frequency in HSPCs. Several studies showed that AAV6 allows for higher HDR rates in HSPCs, independently of the nuclease used (Wang et al., 2015; Dever et al., 2016; Schiroli et al., 2017; Kuo et al., 2018; Pavel-Dinu et al., 2019; Rai et al., 2020; Bijlani et al., 2021). It is known that ssODNs are the preferred substrates for the single-stranded template repair (SSTR) pathway, rather than the conventional HDR and preferably used to correct few nucleotides (Richardson et al., 2018). Pattabhi and others showed that even though AAV6 were more efficient in vitro with respect to ssODN, a higher proportion of ssODN-modified cells persisted in vivo (Pattabhi et al., 2019).
5.2 Addition of molecules
Salisbury-Ruf and others have recently reviewed an extensive list of molecules used to modulate HDR in mammalian cells (Salisbury-Ruf and Larochelle, 2021). Briefly, they classified these modulators in five categories: inhibitors of NHEJ proteins, promoters of HDR, modulators of the cell cycle, molecules targeting chromatin structure, and molecules with undefined mechanisms (Salisbury-Ruf and Larochelle, 2021). Ex vivoex vivoin vivo. Interestingly, when using IDLVs to bring the donor DNA template, transduction in presence of the immunosuppressive molecule cyclosporine H considerably enhances HDR efficiency. Cyclosporine H inhibits the interferon-induced transmembrane protein 3 (IFITM3), responsible for hampering VSV glycoprotein-mediated vector entry (Petrillo et al., 2018).
5.3 Expression of proteins
Addition of proteins that influences gene integration at the DSB locus towards HDR in HSPCs have also been investigated. In 2016, Gutschner and others developed a strategy to increase HDR by directly synchronizing the expression of Cas9 during the S/G2 phase fusing Cas9 to the N-terminal region of human Geminin (Gutschner et al., 2016). This hGemCas9 construct has also been evaluated in HSPCs by D. Khon and others for targeting of the HBB gene. In this study a 4-fold increase in HDR/NHEJ ratio in primary human HSPCs in vitro was obtained and a significant improvement in HDR/NHEJ ratio in vivo (Lomova et al., 2019). In 2019, Schiroli and others showed that transient expression of the dominant-negative P53 mutant protein GSE56 during editing increased hematopoietic repopulation (Schiroli et al., 2019). In 2020, the same group combined GSE56 with expression of the protein E4orf6/7, which recruits the cell cycle controller E2F on its target genes. They showed that with this combined strategy, HDR editing efficiencies of up to 50% in the long-term graft were obtained, without perturbing the function of edited HSPCs (Ferrari et al., 2020). In 2021, De Ravin and others showed that i53, an engineered ubiquitin variant with a high affinity to the tandem Tudor domain of 52BP1, effectively reduces NHEJ repair, and promotes HDR in CD34+ HSPCs (De Ravin et al., 2021). Finally, other proteins tested in non-HSPCs cellular models showed great potential in increasing HDR efficiency, such as CtIP (Charpentier et al., 2018), AUNIP (Lou et al., 2017) or Pold-3 (Reint et al., 2021).
5.4 Additional strategies: Selection of corrected cells and bypassing HDR
Increasing HDR is not the only way to obtain an enriched population of edited cells. Dever and others were able to achieve 90% HBB-edited HSPCs in long-term graft by embedding a reporter cassette in the HDR template (Dever et al., 2016). Another research group designed a robust co-selection strategy using CRISPR–Cas9 and CRISPR-Cpf1 to generate dominant cellular resistance to ouabain and simultaneously edit a second locus of interest (Agudelo et al., 2017). Another possibility to improve editing frequency in HSPCs is the exploitation of the NHEJ pathway to bypass the inefficiencies of HDR-mediated approaches (Bloomer et al., 2021) (Roman-Rodriguez et al., 2019).
6 HSPC gene editing in multisystemic disorders
Nowadays, the portfolio of gene editing tools available and all the progress made in HSPCs processing methods provided great insights for the development of less toxic and more precise novel applications. As a result, during the last decade, multitudes of preclinical studies combining autologous HSCT and gene editing tools for treatment of various disorders were conducted. Diseases due to hematopoietic-derived cells/tissue alterations are the main target for this kind of therapy, and studies targeting blood and immune disorders have been extensively reviewed elsewhere (Jensen et al., 2019; Antoniou et al., 2021; Ferrari et al., 2021; Rai et al., 2021). In this part of the present manuscript, the focus will be on the ability of HSPC-derived cells in communicating with their surrounding environment to receive and pass on information through different mechanisms, such as direct cellular contact and/or secretion of proteins. We will review these different ways of communication and how researchers take advantage of them to develop therapeutic approaches for multisystemic genetic disorders using gene-editing HSPC (Figure 1).
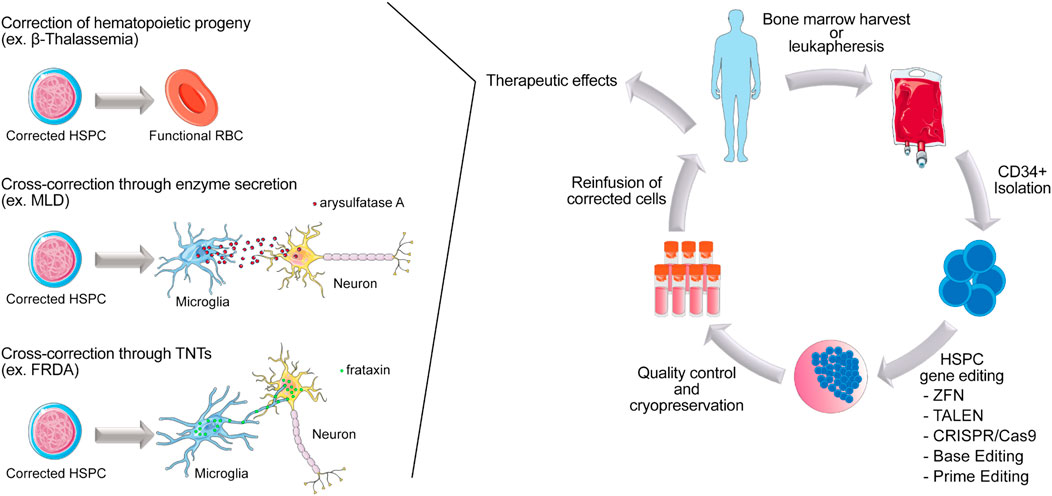
FIGURE 1. Scheme of autologous HSPC gene editing in patients affected by inherited genetic disorders. HSPCs are collected from the patient from the bone marrow or peripheral blood. CD34+ are isolated and gene modified ex vivo, using gene editing tools. After a short period of culture, cells are cryopreserved before being reinfused in patients. Once engrafted, corrected HSPCs can give rise to functional blood and immune cells in the context of blood related disorders such as Beta-thalassemia. Corrected HSPCs can also cross the blood brain barrier and differentiate into microglia to perform tissue cross correction by either enzyme secretion (ex. MLD) or protein transfer via TNTs (ex. FRDA).
6.1 Tissue repair mediated by secretion of therapeutic proteins from gene corrected HSPC-derived cells to diseased tissues
From different successful attempts to cure non-hematologic genetic disorders by allogeneic HSCT, it has been demonstrated that HSPC-derived cells are able to secrete curative proteins. This is particularly true in the context of congenital metabolic diseases (Steward and Jarisch, 2005). In 2004, Biffi and others. demonstrated that the progeny of ex vivo LV-transduced HSPCs transplanted in a mouse model for the neurodegenerative disorder metachromatic leukodystrophy (MLD) were present in the central and peripheral nervous system, where these cells expressed and secreted arylsulfatase A, rescuing the neurodegenerative phenotype. They showed that ex vivo gene therapy had a significantly higher therapeutic impact compared to allogeneic transplantation of WT HSPCs, indicating a critical role for enzyme overexpression in the HSPCs progeny (Biffi et al., 2004). In 2006, the same group showed that efficacy of this approach correlated with the overexpression of arylsulfatase A in the microglia progeny (Biffi et al., 2006). Similarly, in 2010, Visigalli and others showed that autologous HSCT could rescue the mucopolysaccharidosis type I (MPS-I) phenotype in a mouse model for the disease. In this study, lentiviral-based HSCT resulted more effective with respect to allogeneic HSCT, thanks to supranormal enzyme activity (α-l-iduronidase) in the lentiviral transduced hematopoietic system of the transplanted mice, allowing for enzyme delivery to the brain and skeleton and disease correction (Visigalli et al., 2010a). In 2020, Piras and others obtained similar results in a murine model for Pompe Disease. They observed phenotypic correction of heart and muscle function in mice transplanted with lentiviral-based gene modified HSPCs overexpressing the acid alpha-glucosidase enzyme (GAA) (Piras et al., 2020). Numerous studies have demonstrated similar results when autologous HSPCs were transduced with lentivirus to obtain an elevated production of the enzyme of interest in the progeny. In these settings, HSPC-derived cells become a “vehicle” that secretes the functional enzyme in concentrations high enough to compensate the complete absence of enzymes that is normally produced by other cell types (Yoshimitsu et al., 2007; Sergijenko et al., 2013; Adhikari et al., 2021; Dahl et al., 2021).
Although autologous HSCT became one of the most valuable options for patients with particular congenital metabolic diseases (Tucci et al., 2021), risks associated with the use of lentiviral vectors still remain, as previously discussed. Similar to other inherited disorders, also in congenital metabolic diseases gene editing at the specific genomic location has been investigated as an option to overcome these potential risks. In 2019, the Porteus laboratory presented an efficient ex vivo genome editing approach using CRISPR-Cas9, targeting α-l-iduronidase to the CCR5 safe harbor locus in human HSPCs. The modified cells secrete supra-endogenous enzyme levels, maintain long-term repopulation and multi-lineage differentiation potential and can improve biochemical and phenotypic abnormalities in an immunocompromised mouse model of MPS-I (Gomez-Ospina et al., 2019). The exact same approach, but overexpressing the glucocerebrosidase, was used in 2020 by Scharenberg and others as a potential treatment for Gaucher disease. In this study the authors also used a monocyte/macrophage promoter to restrict expression to this carrier cell type (Scharenberg et al., 2020). In a very interesting study, Pavani and others developed a system to highly express therapeutic enzymes in HSPC-derived erythroblasts. The genes for α-globin expression are present in four copies per cell and loss of up to three α-globin alleles is mostly asymptomatic, making this locus a promising candidate for knock-in (KI) in HSPCs using AAV6 and CRISPR/Cas9. Exploiting this notion, they demonstrated that KI of three different human transgenes encoding for α-l-iduronidase (Hurler syndrome), or α-galactosidase (Fabry disease), or lysosomal acid lipase (Wolman disease) at the α-globin locus was possible and resulted in a substantial increase of enzyme expression upon erythroid differentiation (Pavani et al., 2020). Finally, Anthony and others proposed a mutation-agnostic HSPC gene therapy using CRISPR-Cas9 and AAV6 repair template as a prospective treatment option for MLD. In this fascinating study, enzyme activity in HSPC’ patients was restored to levels similar to healthy adults, after gene editing (Antony et al., 2022).
6.2 Tissue repair mediated by protein transfer from gene corrected HSPC-derived cells to diseased tissues via tunneling nanotubes
Tunneling nanotubes (TNTs) have first been described in vitro as highly sensitive nanotubular de novo formed structures that create complex networks and facilitate the selective transfer of membrane vesicles and organelles between cells (Rustom et al., 2004). Later, the presence of TNTs have also been reported in vivo in bone marrow-derived MHC class II(+) cells of the corneal stroma (Chinnery et al., 2008), between human monocyte-derived macrophages (Onfelt et al., 2006) and between T cells as a novel route for HIV-1 transmission (Sowinski et al., 2011). After extensive studies, TNTs are currently defined as cytoplasmic bridges between two close or distant cells. They form gap-like junctions between connected cells and mediate the exchange of cytoplasmic proteins, cellular organelles, lipids, nucleic acids, microRNA, ions and several other components (Lou et al., 2012; Jackson et al., 2016; Drab et al., 2019). Only thicker TNTs containing microtubules (>7-µm diameter) allow for transfer of organelles like mitochondria (Eugenin et al., 2009; Jackson et al., 2016). They facilitate short and long-distance cell-to-cell direct communication (up to 300 µm) (Dubois et al., 2020). TNTs are able to polymerize and depolymerize rapidly (30–60 s), property that makes them fluid and transient structures (Gerdes et al., 2013). TNTs are involved in several pathologies (Tiwari et al., 2021) and generate from different cell types, including multiple HSPCs-derived immune cells (Onfelt et al., 2004).
One of the most powerful features of HSPC-derived cells is the ability to cross the blood brain barrier, hence facilitating the success of the treatment in neuromuscular diseases (Lampron et al., 2012). In 2017, we demonstrated that allogeneic healthy HSCT could rescue the phenotype in a mouse model for Friedreich’s ataxia (FRDA), a neurodegenerative disorder caused by GAA triplets expansion in the FXN gene (Rocca et al., 2017). Our data showed that engrafted HSPC-derived microglia/macrophages were able to transfer the functional mitochondrial frataxin protein to neurons, muscle fibers and cardiomyocytes of the transplanted FRDA mice, preventing the development of motor defects (Rocca et al., 2017). In the same study, we also evidenced the mechanism by which HSPC-derived microglia/macrophages cross-correct affected tissues. These cells transfer mitochondria containing the functional frataxin to neighboring diseased cells by direct contact via TNTs (Rocca et al., 2017). In a more recent manuscript, we provided the foundation for clinical translation of autologous transplantation of gene-corrected HSPCs as a treatment for FRDA (Rocca et al., 2020). Using RNP composed of two gRNAs surrounding the pathologic GAA(n) region and the Cas9 protein, we efficiently removed the pathologic intronic GAA expansion in CD34+ cells isolated from FRDA patients’ peripheral blood, and demonstrated that gene correction restored frataxin expression, allowed for normal hematopoietic differentiation and mitochondrial function in treated cells, in vitro and in vivo (Rocca et al., 2020).
The ability of HSPCs to engraft tissues and differentiate into macrophages/microglia to transfer a functional protein via TNTs have been used as a therapeutic approach also in other genetic disorders. The Cherqui laboratory has pioneered the use of HSPCs to transfer lysosomal proteins via TNTs in the lysosomal storage disorder Cystinosis (Harrison et al., 2013; Naphade et al., 2015; Rocca et al., 2015; Gaide Chevronnay et al., 2016; Rocca and Cherqui, 2019). The Devuyst laboratory successfully applied this approach in a mouse model of Dent disease (Gabriel et al., 2017). Overall, these results open new perspectives for the development of novel gene-modified HSPCs-based therapies for genetic disorders in which the dysfunctional protein is expressed in cellular organelles like lysosomes or mitochondria.
6.3 The strange case of X-linked adrenoleukodystrophy
X-linked adrenoleukodystrophy or X-ALD is a severe genetic demyelinating disease caused by a deficiency of ALD protein, an adenosine triphosphate-binding cassette transporter encoded by the ABCD1 gene. Although the mechanism of rescue is unclear, allogeneic and autologous lentiviral-based HSCT have shown to be effective in the treatment of X-ALD when performed at an early stage of disease (Cartier et al., 2009; Cartier and Aubourg, 2010; Cartier et al., 2012; Eichler et al., 2017). In X-ALD, the mutated gene ABCD1 encodes a peroxisomal membrane protein that cannot be secreted. The invasion of macrophages and/or cross-trafficking of monocytes have been suggested as a possible mechanism. Theory based also on the fact that inflammatory demyelination and microglial cell death “create space” for long-term repopulation of the brain parenchyma with residential macrophages/microglia derived from HSPCs (Cartier and Aubourg, 2010; Varvel et al., 2012; Hohsfield et al., 2020). On the other hand, Yamada and others demonstrated that cell-to-cell contact between healthy microglial cells and ALD fibroblasts was necessary for a phenotypic rescue in vitro, suggesting that cell replacement might not be the only mechanism (Yamada et al., 2004).
Although, gene editing in X-ALD HSPCs has not been investigated yet, the correction of an ALD patient-derived iPSC model using ssODN and the CRISPR/Cas9 system has been reported in a recent study, where the cell line exhibited normal iPSC pluripotency marker expression following genome editing (Sik Jung et al., 2022).
6.4 Challenges of autologous HSCT in treating non-blood disorders
Treating non-blood genetic diseases with genetically modified autologous HSPCs could present with additional challenges. For inherited blood disorders, gene therapy correct the expression of a protein that is naturally expressed in the hematopoietic line. On the contrary, in non-blood genetic disorders HSPC derived cells are used as vehicles to bring a functional protein that in physiological settings is produced by other cell types. With this approach, the integration of the transgene and the expression of a “non-physiological” protein in the HSPCs could lead to several issues: difficulties to control the expression of the protein, impact on the transplanted HSPCs niche itself and/or on the HSPC differentiation potential into blood cells. This is particularly true for metabolic disorders where high levels of enzymes are needed to achieve efficacy. A good example has been provided by Visigalli and others, which demonstrated that a supraphysiologic galactocerebrosidase activity, an enzyme that is dysfunctional in the lysosomal storage disorder globoid cell leukodystrophy, is associated with functional abnormalities affecting HSPCs and their niche (Visigalli et al., 2010b). However, most of these issues are theoretically avoided when using gene editing to restore expression of a dysfunctional protein. In this case, either the gene is corrected but still under the control of its own promoter, or a transgene is integrated under the control of an existing promoter, hence limiting the impact on the HSPCs physiology.
7 Final note
A lot of progress in the field of HSPC-based gene editing has been made in the last decade. Thanks to the significant amount of data obtained from studies and clinical trials involving HSPC in gene therapy, scientists have strong basis to improve existing methods and develop novel approaches more rapidly and safely. In this manuscript, we reviewed some advancements made in HSPC mobilization, culture and patient conditioning. We discussed how gene editing tools have become more and more specific and the various methods to safely deliver them. Most importantly, we also described the range of applications for this approach. HSPCs can be edited ex-vivo not only to replace a specific hematopoietic derived cell type or to re-create a diseased hematopoietic system, but also to use them as a vehicle to deliver a missing or dysfunctional protein in an inherited pathological setting. The various limitations associated to editing-based HSCT has already been extensively reviewed elsewhere (Ferrari et al., 2021; Koniali et al., 2021). However, it is important to mention that the main obstacles associated to gene editing of HSPCs for clinical applications are: a) The risks associated to off-target activity generated by the enzymes and consequently the need to develop more sensitivity and complementary techniques to detect them ex-vivo but also in the long-last graft in patients. b) The problems associated to the DSB toxicity. c) The issues associated with immunogenicity, although this is more a concern for in vivo applications. Finally, another main concern for clinical applications is the cost and access to this type of therapy.
Author contributions
VB, JA, AG and SS wrote the manuscript. CR conceived, organized, supervised, and wrote the manuscript. All authors contributed to the article and approved the submitted version.
Funding
The authors acknowledge funding from AFM/telethon.
Acknowledgments
Parts of Figure 1 were drawn by using pictures from Servier Medical Art. Servier Medical Art by Servier is licensed under a Creative Commons Attribution 3.0 Unported License (https://creativecommons.org/licenses/by/3.0/).
Conflict of interest
Authors VB, JA, AG, SS and CR were employed by the company Genethon.
Publisher’s note
All claims expressed in this article are solely those of the authors and do not necessarily represent those of their affiliated organizations, or those of the publisher, the editors and the reviewers. Any product that may be evaluated in this article, or claim that may be made by its manufacturer, is not guaranteed or endorsed by the publisher.
References
Abdul-Razak, H. H., Rocca, C. J., Howe, S. J., Alonso-Ferrero, M. E., Wang, J., Gabriel, R., et al. (2018). Molecular evidence of genome editing in a mouse model of immunodeficiency. Sci. Rep. 8, 8214. doi:10.1038/s41598-018-26439-9
Adhikari, A., Copping, N. A., Beegle, J., Cameron, D. L., Deng, P., O'geen, H., et al. (2021). Functional rescue in an Angelman syndrome model following treatment with lentivector transduced hematopoietic stem cells. Hum. Mol. Genet. 30, 1067–1083. doi:10.1093/hmg/ddab104
Agarwal, R., Weinberg, K. I., Kwon, H. S., Le, A., Long-Boyle, J. R., and Kohn, D. B. (2020). First report of non-genotoxic conditioning with JSP191 (anti-CD117) and hematopoietic stem cell transplantation in a newly diagnosed patient with severe combined immune deficiency. Blood 136, 10. doi:10.1182/blood-2020-137762
Agudelo, D., Duringer, A., Bozoyan, L., Huard, C. C., Carter, S., Loehr, J., et al. (2017). Marker-free coselection for CRISPR-driven genome editing in human cells. Nat. Methods 14, 615–620. doi:10.1038/nmeth.4265
Andries, O., Mc Cafferty, S., De Smedt, S. C., Weiss, R., Sanders, N. N., and Kitada, T. (2015). N(1)-methylpseudouridine-incorporated mRNA outperforms pseudouridine-incorporated mRNA by providing enhanced protein expression and reduced immunogenicity in mammalian cell lines and mice. J. Control Release 217, 337–344. doi:10.1016/j.jconrel.2015.08.051
Antoniou, P., Miccio, A., and Brusson, M. (2021). Base and prime editing technologies for blood disorders. Front. Genome Ed. 3, 618406. doi:10.3389/fgeed.2021.618406
Antony, J. S., Daniel-Moreno, A., Lamsfus-Calle, A., Raju, J., Kaftancioglu, M., Urena-Bailen, G., et al. (2022). A mutation-agnostic hematopoietic stem cell gene therapy for metachromatic leukodystrophy. CRISPR J. 5, 66–79. doi:10.1089/crispr.2021.0075
Antony, J. S., Latifi, N., Haque, A., Lamsfus-Calle, A., Daniel-Moreno, A., Graeter, S., et al. (2018). Gene correction of HBB mutations in CD34(+) hematopoietic stem cells using Cas9 mRNA and ssODN donors. Mol. Cell. Pediatr. 5, 9. doi:10.1186/s40348-018-0086-1
Anzalone, A. V., Koblan, L. W., and Liu, D. R. (2020). Genome editing with CRISPR-Cas nucleases, base editors, transposases and prime editors. Nat. Biotechnol. 38, 824–844. doi:10.1038/s41587-020-0561-9
Anzalone, A. V., Randolph, P. B., Davis, J. R., Sousa, A. A., Koblan, L. W., Levy, J. M., et al. (2019). Search-and-replace genome editing without double-strand breaks or donor DNA. Nature 576, 149–157. doi:10.1038/s41586-019-1711-4
Bai, T., Li, J., Sinclair, A., Imren, S., Merriam, F., Sun, F., et al. (2019). Expansion of primitive human hematopoietic stem cells by culture in a zwitterionic hydrogel. Nat. Med. 25, 1566–1575. doi:10.1038/s41591-019-0601-5
Bak, R. O., and Porteus, M. H. (2017). CRISPR-mediated integration of large gene cassettes using AAV donor vectors. Cell. Rep. 20, 750–756. doi:10.1016/j.celrep.2017.06.064
Banskota, S., Raguram, A., Suh, S., Du, S. W., Davis, J. R., Choi, E. H., et al. (2022). Engineered virus-like particles for efficient in vivo delivery of therapeutic proteins. Cell. 185, 250–265.e16. doi:10.1016/j.cell.2021.12.021
Baptista, B., Carapito, R., Laroui, N., Pichon, C., and Sousa, F. (2021). mRNA, a revolution in biomedicine. Pharmaceutics 13, 2090. doi:10.3390/pharmaceutics13122090
Barrangou, R., Fremaux, C., Deveau, H., Richards, M., Boyaval, P., Moineau, S., et al. (2007). CRISPR provides acquired resistance against viruses in prokaryotes. Science 315, 1709–1712. doi:10.1126/science.1138140
Bendall, L. J., and Bradstock, K. F. (2014). G-CSF: From granulopoietic stimulant to bone marrow stem cell mobilizing agent. Cytokine Growth Factor Rev. 25, 355–367. doi:10.1016/j.cytogfr.2014.07.011
Bernardo, M. E., and Aiuti, A. (2016). The role of conditioning in hematopoietic stem-cell gene therapy. Hum. Gene Ther. 27, 741–748. doi:10.1089/hum.2016.103
Biffi, A., Capotondo, A., Fasano, S., Del Carro, U., Marchesini, S., Azuma, H., et al. (2006). Gene therapy of metachromatic leukodystrophy reverses neurological damage and deficits in mice. J. Clin. Invest. 116, 3070–3082. doi:10.1172/JCI28873
Biffi, A., De Palma, M., Quattrini, A., Del Carro, U., Amadio, S., Visigalli, I., et al. (2004). Correction of metachromatic leukodystrophy in the mouse model by transplantation of genetically modified hematopoietic stem cells. J. Clin. Invest. 113, 1118–1129. doi:10.1172/JCI19205
Bijlani, S., Pang, K. M., Sivanandam, V., Singh, A., and Chatterjee, S. (2021). The role of recombinant AAV in precise genome editing. Front. Genome Ed. 3, 799722. doi:10.3389/fgeed.2021.799722
Blattner, G., Cavazza, A., Thrasher, A. J., and Turchiano, G. (2020). Gene editing and genotoxicity: Targeting the off-targets. Front. Genome Ed. 2, 613252. doi:10.3389/fgeed.2020.613252
Bloomer, H., Smith, R. H., Hakami, W., and Larochelle, A. (2021). Genome editing in human hematopoietic stem and progenitor cells via CRISPR-Cas9-mediated homology-independent targeted integration. Mol. Ther. 29, 1611–1624. doi:10.1016/j.ymthe.2020.12.010
Boch, J., Scholze, H., Schornack, S., Landgraf, A., Hahn, S., Kay, S., et al. (2009). Breaking the code of DNA binding specificity of TAL-type III effectors. Science 326, 1509–1512. doi:10.1126/science.1178811
Bogdanove, A. J., and Voytas, D. F. (2011). TAL effectors: Customizable proteins for DNA targeting. Science 333, 1843–1846. doi:10.1126/science.1204094
Boitano, A. E., Wang, J., Romeo, R., Bouchez, L. C., Parker, A. E., Sutton, S. E., et al. (2010). Aryl hydrocarbon receptor antagonists promote the expansion of human hematopoietic stem cells. Science 329, 1345–1348. doi:10.1126/science.1191536
Bolt, M. W., Brady, J. T., Whiteley, L. O., and Khan, K. N. (2021). Development challenges associated with rAAV-based gene therapies. J. Toxicol. Sci. 46, 57–68. doi:10.2131/jts.46.57
Bordignon, C., Notarangelo, L. D., Nobili, N., Ferrari, G., Casorati, G., Panina, P., et al. (1995). Gene therapy in peripheral blood lymphocytes and bone marrow for ADA- immunodeficient patients. Science 270, 470–475. doi:10.1126/science.270.5235.470
Brault, J., Liu, T., Bello, E., Liu, S., Sweeney, C. L., Meis, R. J., et al. (2021). CRISPR-targeted MAGT1 insertion restores XMEN patient hematopoietic stem cells and lymphocytes. Blood 138, 2768–2780. doi:10.1182/blood.2021011192
Brouns, S. J., Jore, M. M., Lundgren, M., Westra, E. R., Slijkhuis, R. J., Snijders, A. P., et al. (2008). Small CRISPR RNAs guide antiviral defense in prokaryotes. Science 321, 960–964. doi:10.1126/science.1159689
Cannon, P., Asokan, A., Czechowicz, A., Hammond, P., Kohn, D. B., Lieber, A., et al. (2021). Safe and effective in vivo targeting and gene editing in hematopoietic stem cells: Strategies for accelerating development. Hum. Gene Ther. 32, 31–42. doi:10.1089/hum.2020.263
Cartier, N., and Aubourg, P. (2010). Hematopoietic stem cell transplantation and hematopoietic stem cell gene therapy in X-linked adrenoleukodystrophy. Brain Pathol. 20, 857–862. doi:10.1111/j.1750-3639.2010.00394.x
Cartier, N., Hacein-Bey-Abina, S., Bartholomae, C. C., Bougneres, P., Schmidt, M., Kalle, C. V., et al. (2012). Lentiviral hematopoietic cell gene therapy for X-linked adrenoleukodystrophy. Methods Enzymol. 507, 187–198. doi:10.1016/B978-0-12-386509-0.00010-7
Cartier, N., Hacein-Bey-Abina, S., Bartholomae, C. C., Veres, G., Schmidt, M., Kutschera, I., et al. (2009). Hematopoietic stem cell gene therapy with a lentiviral vector in X-linked adrenoleukodystrophy. Science 326, 818–823. doi:10.1126/science.1171242
Cavazzana, M., Bushman, F. D., Miccio, A., Andre-Schmutz, I., and Six, E. (2019). Gene therapy targeting haematopoietic stem cells for inherited diseases: Progress and challenges. Nat. Rev. Drug Discov. 18, 447–462. doi:10.1038/s41573-019-0020-9
Cavazzana-Calvo, M., Hacein-Bey, S., De Saint Basile, G., Gross, F., Yvon, E., Nusbaum, P., et al. (2000). Gene therapy of human severe combined immunodeficiency (SCID)-X1 disease. Science 288, 669–672. doi:10.1126/science.288.5466.669
Cermak, T., Doyle, E. L., Christian, M., Wang, L., Zhang, Y., Schmidt, C., et al. (2011). Efficient design and assembly of custom TALEN and other TAL effector-based constructs for DNA targeting. Nucleic Acids Res. 39, e82. doi:10.1093/nar/gkr218
Chandrasekaran, A. P., Song, M., Kim, K. S., and Ramakrishna, S. (2018). Different methods of delivering CRISPR/Cas9 into cells. Prog. Mol. Biol. Transl. Sci. 159, 157–176. doi:10.1016/bs.pmbts.2018.05.001
Chanut, F. J. A., Sanvito, F., Ferrari, G., Visigalli, I., Carriglio, N., Hernandez, R. J., et al. (2021). Conditioning regimens in long-term pre-clinical studies to support development of ex vivo gene therapy: Review of nonproliferative and proliferative changes. Hum. Gene Ther. 32, 66–76. doi:10.1089/hum.2020.135
Charpentier, M., Khedher, A. H. Y., Menoret, S., Brion, A., Lamribet, K., Dardillac, E., et al. (2018). CtIP fusion to Cas9 enhances transgene integration by homology-dependent repair. Nat. Commun. 9, 1133. doi:10.1038/s41467-018-03475-7
Chen, P. J., Hussmann, J. A., Yan, J., Knipping, F., Ravisankar, P., Chen, P. F., et al. (2021). Enhanced prime editing systems by manipulating cellular determinants of editing outcomes. Cell. 184, 5635–5652.e29. doi:10.1016/j.cell.2021.09.018
Chhabra, A., Ring, A. M., Weiskopf, K., Schnorr, P. J., Gordon, S., Le, A. C., et al. (2016). Hematopoietic stem cell transplantation in immunocompetent hosts without radiation or chemotherapy. Sci. Transl. Med. 8, 351ra105. doi:10.1126/scitranslmed.aae0501
Chinnery, H. R., Pearlman, E., and Mcmenamin, P. G. (2008). Cutting edge: Membrane nanotubes in vivo: A feature of MHC class II+ cells in the mouse cornea. J. Immunol. 180, 5779–5783. doi:10.4049/jimmunol.180.9.5779
Cohen, S., Roy, J., Lachance, S., Delisle, J. S., Marinier, A., Busque, L., et al. (2020). Hematopoietic stem cell transplantation using single um171-expanded cord blood: A single-arm, phase 1-2 safety and feasibility study. Lancet Haematol. 7, e134–e145. doi:10.1016/S2352-3026(19)30202-9
Cong, L., Ran, F. A., Cox, D., Lin, S., Barretto, R., Habib, N., et al. (2013). Multiplex genome engineering using CRISPR/Cas systems. Science 339, 819–823. doi:10.1126/science.1231143
Conway, A., Mendel, M., Kim, K., Mcgovern, K., Boyko, A., Zhang, L., et al. (2019). Non-viral delivery of zinc finger nuclease mRNA enables highly efficient in vivo genome editing of multiple therapeutic gene targets. Mol. Ther. 27, 866–877. doi:10.1016/j.ymthe.2019.03.003
Cousin, C., Oberkampf, M., Felix, T., Rosenbaum, P., Weil, R., Fabrega, S., et al. (2019). Persistence of integrase-deficient lentiviral vectors correlates with the induction of STING-independent CD8(+) T cell responses. Cell. Rep. 26, 1242–1257. e1247. doi:10.1016/j.celrep.2019.01.025
Cromer, M. K., Camarena, J., Martin, R. M., Lesch, B. J., Vakulskas, C. A., Bode, N. M., et al. (2021). Gene replacement of alpha-globin with beta-globin restores hemoglobin balance in beta-thalassemia-derived hematopoietic stem and progenitor cells. Nat. Med. 27, 677–687. doi:10.1038/s41591-021-01284-y
Cullot, G., Boutin, J., Toutain, J., Prat, F., Pennamen, P., Rooryck, C., et al. (2019). CRISPR-Cas9 genome editing induces megabase-scale chromosomal truncations. Nat. Commun. 10, 1136. doi:10.1038/s41467-019-09006-2
Czechowicz, A., Kraft, D., Weissman, I. L., and Bhattacharya, D. (2007). Efficient transplantation via antibody-based clearance of hematopoietic stem cell niches. Science 318, 1296–1299. doi:10.1126/science.1149726
Czechowicz, A., Palchaudhuri, R., Scheck, A., Hu, Y., Hoggatt, J., Saez, B., et al. (2019). Selective hematopoietic stem cell ablation using CD117-antibody-drug-conjugates enables safe and effective transplantation with immunity preservation. Nat. Commun. 10, 617. doi:10.1038/s41467-018-08201-x
Dahl, M., Smith, E. M. K., Warsi, S., Rothe, M., Ferraz, M. J., Aerts, J., et al. (2021). Correction of pathology in mice displaying Gaucher disease type 1 by a clinically-applicable lentiviral vector. Mol. Ther. Methods Clin. Dev. 20, 312–323. doi:10.1016/j.omtm.2020.11.018
Dausset, J., and Brecy, H. (1957). Identical nature of the leucocyte antigens detectable in monozygotic twins by means of immune iso-leuco-agglutinins. Nature 180, 1430. doi:10.1038/1801430a0
De Ravin, S. S., Brault, J., Meis, R. J., Liu, S., Li, L., Pavel-Dinu, M., et al. (2021). Enhanced homology-directed repair for highly efficient gene editing in hematopoietic stem/progenitor cells. Blood 137, 2598–2608. doi:10.1182/blood.2020008503
Dever, D. P., Bak, R. O., Reinisch, A., Camarena, J., Washington, G., Nicolas, C. E., et al. (2016). CRISPR/Cas9 beta-globin gene targeting in human haematopoietic stem cells. Nature 539, 384–389. doi:10.1038/nature20134
Digiusto, D. L., Cannon, P. M., Holmes, M. C., Li, L., Rao, A., Wang, J., et al. (2016). Preclinical development and qualification of ZFN-mediated CCR5 disruption in human hematopoietic stem/progenitor cells. Mol. Ther. Methods Clin. Dev. 3, 16067. doi:10.1038/mtm.2016.67
Drab, M., Stopar, D., Kralj-Iglic, V., and Iglic, A. (2019). Inception mechanisms of tunneling nanotubes. Cells 8, 626. doi:10.3390/cells8060626
Dubois, F., Benard, M., Jean-Jacques, B., Schapman, D., Roberge, H., Lebon, A., et al. (2020). Investigating tunneling nanotubes in cancer cells: Guidelines for structural and functional studies through cell imaging. Biomed. Res. Int. 2020, 2701345. doi:10.1155/2020/2701345
Eichler, F., Duncan, C., Musolino, P. L., Orchard, P. J., De Oliveira, S., Thrasher, A. J., et al. (2017). Hematopoietic stem-cell gene therapy for cerebral adrenoleukodystrophy. N. Engl. J. Med. 377, 1630–1638. doi:10.1056/NEJMoa1700554
Esrick, E. B., Manis, J. P., Daley, H., Baricordi, C., Trebeden-Negre, H., Pierciey, F. J., et al. (2018). Successful hematopoietic stem cell mobilization and apheresis collection using plerixafor alone in sickle cell patients. Blood Adv. 2, 2505–2512. doi:10.1182/bloodadvances.2018016725
Eugenin, E. A., Gaskill, P. J., and Berman, J. W. (2009). Tunneling nanotubes (TNT) are induced by HIV-infection of macrophages: A potential mechanism for intercellular HIV trafficking. Cell. Immunol. 254, 142–148. doi:10.1016/j.cellimm.2008.08.005
Fares, I., Chagraoui, J., Gareau, Y., Gingras, S., Ruel, R., Mayotte, N., et al. (2014). Cord blood expansion. Pyrimidoindole derivatives are agonists of human hematopoietic stem cell self-renewal. Science 345, 1509–1512. doi:10.1126/science.1256337
Ferrari, S., Jacob, A., Beretta, S., Unali, G., Albano, L., Vavassori, V., et al. (2020). Efficient gene editing of human long-term hematopoietic stem cells validated by clonal tracking. Nat. Biotechnol. 38, 1298–1308. doi:10.1038/s41587-020-0551-y
Ferrari, S., Vavassori, V., Canarutto, D., Jacob, A., Castiello, M. C., Javed, A. O., et al. (2021). Gene editing of hematopoietic stem cells: Hopes and hurdles toward clinical translation. Front. Genome Ed. 3, 618378. doi:10.3389/fgeed.2021.618378
Ferreira Da Silva, J., Oliveira, G. P., Arasa-Verge, E. A., Kagiou, C., Moretton, A., Timelthaler, G., et al. (2022). Prime editing efficiency and fidelity are enhanced in the absence of mismatch repair. Nat. Commun. 13, 760. doi:10.1038/s41467-022-28442-1
Fitzhugh, C. D., Hsieh, M. M., Bolan, C. D., Saenz, C., and Tisdale, J. F. (2009). Granulocyte colony-stimulating factor (G-CSF) administration in individuals with sickle cell disease: Time for a moratorium? Cytotherapy 11, 464–471. doi:10.1080/14653240902849788
Frangoul, H., Altshuler, D., Cappellini, M. D., Chen, Y. S., Domm, J., Eustace, B. K., et al. (2021). CRISPR-Cas9 gene editing for sickle cell disease and beta-thalassemia. N. Engl. J. Med. 384, 252–260. doi:10.1056/NEJMoa2031054
Gabriel, R., Lombardo, A., Arens, A., Miller, J. C., Genovese, P., Kaeppel, C., et al. (2011). An unbiased genome-wide analysis of zinc-finger nuclease specificity. Nat. Biotechnol. 29, 816–823. doi:10.1038/nbt.1948
Gabriel, S. S., Belge, H., Gassama, A., Debaix, H., Luciani, A., Fehr, T., et al. (2017). Bone marrow transplantation improves proximal tubule dysfunction in a mouse model of Dent disease. Kidney Int. 91, 842–855. doi:10.1016/j.kint.2016.11.016
Gaide Chevronnay, H. P., Janssens, V., Van Der Smissen, P., Rocca, C. J., Liao, X. H., Refetoff, S., et al. (2016). Hematopoietic stem cells transplantation can normalize thyroid function in a cystinosis mouse model. Endocrinology 157, 1363–1371. doi:10.1210/en.2015-1762
Gaj, T., Gersbach, C. A., and Barbas, C. F. (2013). ZFN, TALEN, and CRISPR/Cas-based methods for genome engineering. Trends Biotechnol. 31, 397–405. doi:10.1016/j.tibtech.2013.04.004
Galy, A. (2017). Major advances in the development of vectors for clinical gene therapy of hematopoietic stem cells from European groups over the last 25 years. Hum. Gene Ther. 28, 964–971. doi:10.1089/hum.2017.152
Gao, R., Fu, Z. C., Li, X., Wang, Y., Wei, J., Li, G., et al. (2022). Genomic and transcriptomic analyses of prime editing guide RNA-independent off-target effects by prime editors. CRISPR J. 5, 276–293. doi:10.1089/crispr.2021.0080
Gaudelli, N. M., Komor, A. C., Rees, H. A., Packer, M. S., Badran, A. H., Bryson, D. I., et al. (2017). Programmable base editing of A*T to G*C in genomic DNA without DNA cleavage. Nature 551, 464–471. doi:10.1038/nature24644
Gerdes, H. H., Rustom, A., and Wang, X. (2013). Tunneling nanotubes, an emerging intercellular communication route in development. Mech. Dev. 130, 381–387. doi:10.1016/j.mod.2012.11.006
Gertz, M. A. (2010). Current status of stem cell mobilization. Br. J. Haematol. 150, 647–662. doi:10.1111/j.1365-2141.2010.08313.x
Gomez-Ospina, N., Scharenberg, S. G., Mostrel, N., Bak, R. O., Mantri, S., Quadros, R. M., et al. (2019). Human genome-edited hematopoietic stem cells phenotypically correct Mucopolysaccharidosis type I. Nat. Commun. 10, 4045. doi:10.1038/s41467-019-11962-8
Guan, S., and Rosenecker, J. (2017). Nanotechnologies in delivery of mRNA therapeutics using nonviral vector-based delivery systems. Gene Ther. 24, 133–143. doi:10.1038/gt.2017.5
Gundry, M. C., Brunetti, L., Lin, A., Mayle, A. E., Kitano, A., Wagner, D., et al. (2016). Highly efficient genome editing of murine and human hematopoietic progenitor cells by CRISPR/Cas9. Cell. Rep. 17, 1453–1461. doi:10.1016/j.celrep.2016.09.092
Gupta, R. K., Abdul-Jawad, S., Mccoy, L. E., Mok, H. P., Peppa, D., Salgado, M., et al. (2019). HIV-1 remission following CCR5Δ32/Δ32 haematopoietic stem-cell transplantation. Nature 568, 244–248. doi:10.1038/s41586-019-1027-4
Gustafsson, C., Govindarajan, S., and Minshull, J. (2004). Codon bias and heterologous protein expression. Trends Biotechnol. 22, 346–353. doi:10.1016/j.tibtech.2004.04.006
Gutierrez-Guerrero, A., Abrey Recalde, M. J., Mangeot, P. E., Costa, C., Bernadin, O., Perian, S., et al. (2021). Baboon envelope pseudotyped "nanoblades" carrying cas9/gRNA complexes allow efficient genome editing in human T, B, and CD34(+) cells and knock-in of AAV6-encoded donor DNA in CD34(+) cells. Front. Genome Ed. 3, 604371. doi:10.3389/fgeed.2021.604371
Gutschner, T., Haemmerle, M., Genovese, G., Draetta, G. F., and Chin, L. (2016). Post-translational regulation of Cas9 during G1 enhances homology-directed repair. Cell. Rep. 14, 1555–1566. doi:10.1016/j.celrep.2016.01.019
Hacein-Bey Abina, S., Gaspar, H. B., Blondeau, J., Caccavelli, L., Charrier, S., Buckland, K., et al. (2015). Outcomes following gene therapy in patients with severe Wiskott-Aldrich syndrome. JAMA 313, 1550–1563. doi:10.1001/jama.2015.3253
Hacein-Bey-Abina, S., Von Kalle, C., Schmidt, M., Mccormack, M. P., Wulffraat, N., Leboulch, P., et al. (2003). LMO2-associated clonal T cell proliferation in two patients after gene therapy for SCID-X1. Science 302, 415–419. doi:10.1126/science.1088547
Harrison, F., Yeagy, B. A., Rocca, C. J., Kohn, D. B., Salomon, D. R., and Cherqui, S. (2013). Hematopoietic stem cell gene therapy for the multisystemic lysosomal storage disorder cystinosis. Mol. Ther. 21, 433–444. doi:10.1038/mt.2012.214
Hendel, A., Bak, R. O., Clark, J. T., Kennedy, A. B., Ryan, D. E., Roy, S., et al. (2015). Chemically modified guide RNAs enhance CRISPR-Cas genome editing in human primary cells. Nat. Biotechnol. 33, 985–989. doi:10.1038/nbt.3290
Hoggatt, J., Singh, P., Tate, T. A., Chou, B. K., Datari, S. R., Fukuda, S., et al. (2018). Rapid mobilization reveals a highly engraftable hematopoietic stem cell. Cell. 172, 191–204. doi:10.1016/j.cell.2017.11.003
Hohsfield, L. A., Najafi, A. R., Ghorbanian, Y., Soni, N., Hingco, E. E., Kim, S. J., et al. (2020). Effects of long-term and brain-wide colonization of peripheral bone marrow-derived myeloid cells in the CNS. J. Neuroinflammation 17, 279. doi:10.1186/s12974-020-01931-0
Holig, K., Kramer, M., Kroschinsky, F., Bornhauser, M., Mengling, T., Schmidt, A. H., et al. (2009). Safety and efficacy of hematopoietic stem cell collection from mobilized peripheral blood in unrelated volunteers: 12 years of single-center experience in 3928 donors. Blood 114, 3757–3763. doi:10.1182/blood-2009-04-218651
Howe, S. J., Mansour, M. R., Schwarzwaelder, K., Bartholomae, C., Hubank, M., Kempski, H., et al. (2008). Insertional mutagenesis combined with acquired somatic mutations causes leukemogenesis following gene therapy of SCID-X1 patients. J. Clin. Invest. 118, 3143–3150. doi:10.1172/JCI35798
Hsu, P. D., Scott, D. A., Weinstein, J. A., Ran, F. A., Konermann, S., Agarwala, V., et al. (2013). DNA targeting specificity of RNA-guided Cas9 nucleases. Nat. Biotechnol. 31, 827–832. doi:10.1038/nbt.2647
Hu, Y., and Li, W. (2022). Development and application of CRISPR-cas based tools. Front. Cell. Dev. Biol. 10, 834646. doi:10.3389/fcell.2022.834646
Hubel, K. (2019). “Mobilization and collection of HSC,” in The EBMT handbook: Hematopoietic stem cell transplantation and cellular therapies. Editors Th, E. Carreras, C. Dufour, M. Mohty, and N. Kroger (Cham (CH), 117–122.
Hutter, G., Nowak, D., Mossner, M., Ganepola, S., Mussig, A., Allers, K., et al. (2009). Long-term control of HIV by CCR5 Delta32/Delta32 stem-cell transplantation. N. Engl. J. Med. 360, 692–698. doi:10.1056/NEJMoa0802905
Isalan, M. (2011). Zinc-finger nucleases: How to play two good hands. Nat. Methods 9, 32–34. doi:10.1038/nmeth.1805
Jackson, M. V., Morrison, T. J., Doherty, D. F., Mcauley, D. F., Matthay, M. A., Kissenpfennig, A., et al. (2016). Mitochondrial transfer via tunneling nanotubes is an important mechanism by which mesenchymal stem cells enhance macrophage phagocytosis in the in vitro and in vivo models of ARDS. Stem Cells 34, 2210–2223. doi:10.1002/stem.2372
Jemielity, J., Fowler, T., Zuberek, J., Stepinski, J., Lewdorowicz, M., Niedzwiecka, A., et al. (2003). Novel "anti-reverse" cap analogs with superior translational properties. RNA 9, 1108–1122. doi:10.1261/rna.5430403
Jensen, T. I., Axelgaard, E., and Bak, R. O. (2019). Therapeutic gene editing in haematological disorders with CRISPR/Cas9. Br. J. Haematol. 185, 821–835. doi:10.1111/bjh.15851
Jeong, Y. K., Song, B., and Bae, S. (2020). Current status and challenges of DNA base editing tools. Mol. Ther. 28, 1938–1952. doi:10.1016/j.ymthe.2020.07.021
Jiang, L., and Yao, S. (2022). Enhancing prime editing via inhibition of mismatch repair pathway. Mol. Biomed. 3, 7. doi:10.1186/s43556-022-00072-5
Jinek, M., Chylinski, K., Fonfara, I., Hauer, M., Doudna, J. A., and Charpentier, E. (2012). A programmable dual-RNA-guided DNA endonuclease in adaptive bacterial immunity. Science 337, 816–821. doi:10.1126/science.1225829
Juarez, J. G., Harun, N., Thien, M., Welschinger, R., Baraz, R., Pena, A. D., et al. (2012). Sphingosine-1-phosphate facilitates trafficking of hematopoietic stem cells and their mobilization by CXCR4 antagonists in mice. Blood 119, 707–716. doi:10.1182/blood-2011-04-348904
Kariko, K., Muramatsu, H., Welsh, F. A., Ludwig, J., Kato, H., Akira, S., et al. (2008). Incorporation of pseudouridine into mRNA yields superior nonimmunogenic vector with increased translational capacity and biological stability. Mol. Ther. 16, 1833–1840. doi:10.1038/mt.2008.200
Kim, Y. G., Cha, J., and Chandrasegaran, S. (1996). Hybrid restriction enzymes: Zinc finger fusions to fok I cleavage domain. Proc. Natl. Acad. Sci. U. S. A. 93, 1156–1160. doi:10.1073/pnas.93.3.1156
Kohn, D. B., Booth, C., Shaw, K. L., Xu-Bayford, J., Garabedian, E., Trevisan, V., et al. (2021). Autologous ex vivo lentiviral gene therapy for adenosine deaminase deficiency. N. Engl. J. Med. 384, 2002–2013. doi:10.1056/NEJMoa2027675
Komor, A. C., Kim, Y. B., Packer, M. S., Zuris, J. A., and Liu, D. R. (2016). Programmable editing of a target base in genomic DNA without double-stranded DNA cleavage. Nature 533, 420–424. doi:10.1038/nature17946
Koniali, L., Lederer, C. W., and Kleanthous, M. (2021). Therapy development by genome editing of hematopoietic stem cells. Cells 10 (6), 1492. doi:10.3390/cells10061492
Korner, C. G., and Wahle, E. (1997). Poly(A) tail shortening by a mammalian poly(A)-specific 3'-exoribonuclease. J. Biol. Chem. 272, 10448–10456. doi:10.1074/jbc.272.16.10448
Kosicki, M., Tomberg, K., and Bradley, A. (2018). Repair of double-strand breaks induced by CRISPR-Cas9 leads to large deletions and complex rearrangements. Nat. Biotechnol. 36, 765–771. doi:10.1038/nbt.4192
Kuo, C. Y., Long, J. D., Campo-Fernandez, B., De Oliveira, S., Cooper, A. R., Romero, Z., et al. (2018). Site-specific gene editing of human hematopoietic stem cells for X-linked hyper-IgM syndrome. Cell. Rep. 23, 2606–2616. doi:10.1016/j.celrep.2018.04.103
Kwon, H., Kim, M., Seo, Y., Moon, Y. S., Lee, H. J., Lee, K., et al. (2018). Emergence of synthetic mRNA: In vitro synthesis of mRNA and its applications in regenerative medicine. Biomaterials 156, 172–193. doi:10.1016/j.biomaterials.2017.11.034
Lampron, A., Lessard, M., and Rivest, S. (2012). Effects of myeloablation, peripheral chimerism, and whole-body irradiation on the entry of bone marrow-derived cells into the brain. Cell. Transpl. 21, 1149–1159. doi:10.3727/096368911X593154
Lattanzi, A., Meneghini, V., Pavani, G., Amor, F., Ramadier, S., Felix, T., et al. (2019). Optimization of CRISPR/Cas9 delivery to human hematopoietic stem and progenitor cells for therapeutic genomic rearrangements. Mol. Ther. 27, 137–150. doi:10.1016/j.ymthe.2018.10.008
Li, C., Georgakopoulou, A., Mishra, A., Gil, S., Hawkins, R. D., Yannaki, E., et al. (2021). In vivo HSPC gene therapy with base editors allows for efficient reactivation of fetal gamma-globin in beta-YAC mice. Blood Adv. 5, 1122–1135. doi:10.1182/bloodadvances.2020003702
Li, C., and Lieber, A. (2019). Adenovirus vectors in hematopoietic stem cell genome editing. FEBS Lett. 593, 3623–3648. doi:10.1002/1873-3468.13668
Li, L., Krymskaya, L., Wang, J., Henley, J., Rao, A., Cao, L. F., et al. (2013). Genomic editing of the HIV-1 coreceptor CCR5 in adult hematopoietic stem and progenitor cells using zinc finger nucleases. Mol. Ther. 21, 1259–1269. doi:10.1038/mt.2013.65
Li, T., Huang, S., Jiang, W. Z., Wright, D., Spalding, M. H., Weeks, D. P., et al. (2011). TAL nucleases (TALNs): Hybrid proteins composed of TAL effectors and FokI DNA-cleavage domain. Nucleic Acids Res. 39, 359–372. doi:10.1093/nar/gkq704
Li, X., Zhou, L., Gao, B. Q., Li, G., Wang, X., Wang, Y., et al. (2022). Highly efficient prime editing by introducing same-sense mutations in pegRNA or stabilizing its structure. Nat. Commun. 13, 1669. doi:10.1038/s41467-022-29339-9
Liles, W. C., Rodger, E., Broxmeyer, H. E., Dehner, C., Badel, K., Calandra, G., et al. (2005). Augmented mobilization and collection of CD34+ hematopoietic cells from normal human volunteers stimulated with granulocyte-colony-stimulating factor by single-dose administration of AMD3100, a CXCR4 antagonist. Transfusion 45, 295–300. doi:10.1111/j.1537-2995.2005.04222.x
Liu, J., Gaj, T., Wallen, M. C., and Barbas, C. F. (2015a). Improved cell-penetrating zinc-finger nuclease proteins for precision genome engineering. Mol. Ther. Nucleic Acids 4, e232. doi:10.1038/mtna.2015.6
Liu, J., Gaj, T., Yang, Y., Wang, N., Shui, S., Kim, S., et al. (2015b). Efficient delivery of nuclease proteins for genome editing in human stem cells and primary cells. Nat. Protoc. 10, 1842–1859. doi:10.1038/nprot.2015.117
Liu, P., Liang, S. Q., Zheng, C., Mintzer, E., Zhao, Y. G., Ponnienselvan, K., et al. (2021). Improved prime editors enable pathogenic allele correction and cancer modelling in adult mice. Nat. Commun. 12, 2121. doi:10.1038/s41467-021-22295-w
Liu, W., Du, W., Shang, X., Wang, L., Evelyn, C., Florian, M. C., et al. (2019). Rational identification of a Cdc42 inhibitor presents a new regimen for long-term hematopoietic stem cell mobilization. Leukemia 33, 749–761. doi:10.1038/s41375-018-0251-5
Liu, Y., Yang, G., Huang, S., Li, X., Wang, X., Li, G., et al. (2021). Enhancing prime editing by Csy4-mediated processing of pegRNA. Cell. Res. 31, 1134–1136. doi:10.1038/s41422-021-00520-x
Lombardo, A., Genovese, P., Beausejour, C. M., Colleoni, S., Lee, Y. L., Kim, K. A., et al. (2007). Gene editing in human stem cells using zinc finger nucleases and integrase-defective lentiviral vector delivery. Nat. Biotechnol. 25, 1298–1306. doi:10.1038/nbt1353
Lomova, A., Clark, D. N., Campo-Fernandez, B., Flores-Bjurstrom, C., Kaufman, M. L., Fitz-Gibbon, S., et al. (2019). Improving gene editing outcomes in human hematopoietic stem and progenitor cells by temporal control of DNA repair. Stem Cells 37, 284–294. doi:10.1002/stem.2935
Lou, E., Fujisawa, S., Barlas, A., Romin, Y., Manova-Todorova, K., Moore, M. A., et al. (2012). Tunneling nanotubes: A new paradigm for studying intercellular communication and therapeutics in cancer. Commun. Integr. Biol. 5, 399–403. doi:10.4161/cib.20569
Lou, J., Chen, H., Han, J., He, H., Huen, M. S. Y., Feng, X. H., et al. (2017). AUNIP/C1orf135 directs DNA double-strand breaks towards the homologous recombination repair pathway. Nat. Commun. 8, 985. doi:10.1038/s41467-017-01151-w
Lu, B., Javidi-Parsijani, P., Makani, V., Mehraein-Ghomi, F., Sarhan, W. M., Sun, D., et al. (2019). Delivering SaCas9 mRNA by lentivirus-like bionanoparticles for transient expression and efficient genome editing. Nucleic Acids Res. 47, e44. doi:10.1093/nar/gkz093
Lux, C. T., Pattabhi, S., Berger, M., Nourigat, C., Flowers, D. A., Negre, O., et al. (2019). TALEN-mediated gene editing of HBG in human hematopoietic stem cells leads to therapeutic fetal hemoglobin induction. Mol. Ther. Methods Clin. Dev. 12, 175–183. doi:10.1016/j.omtm.2018.12.008
Magnani, A., Semeraro, M., Adam, F., Booth, C., Dupre, L., Morris, E. C., et al. (2022). Long-term safety and efficacy of lentiviral hematopoietic stem/progenitor cell gene therapy for Wiskott-Aldrich syndrome. Nat. Med. 28, 71–80. doi:10.1038/s41591-021-01641-x
Magrin, E., Semeraro, M., Hebert, N., Joseph, L., Magnani, A., Chalumeau, A., et al. (2022). Long-term outcomes of lentiviral gene therapy for the beta-hemoglobinopathies: The HGB-205 trial. Nat. Med. 28, 81–88. doi:10.1038/s41591-021-01650-w
Mali, P., Yang, L., Esvelt, K. M., Aach, J., Guell, M., Dicarlo, J. E., et al. (2013). RNA-guided human genome engineering via Cas9. Science 339, 823–826. doi:10.1126/science.1232033
Mangeot, P. E., Risson, V., Fusil, F., Marnef, A., Laurent, E., Blin, J., et al. (2019). Genome editing in primary cells and in vivo using viral-derived Nanoblades loaded with Cas9-sgRNA ribonucleoproteins. Nat. Commun. 10, 45. doi:10.1038/s41467-018-07845-z
Miller, J. C., Patil, D. P., Xia, D. F., Paine, C. B., Fauser, F., Richards, H. W., et al. (2019). Enhancing gene editing specificity by attenuating DNA cleavage kinetics. Nat. Biotechnol. 37, 945–952. doi:10.1038/s41587-019-0186-z
Miller, J. C., Tan, S., Qiao, G., Barlow, K. A., Wang, J., Xia, D. F., et al. (2011). A TALE nuclease architecture for efficient genome editing. Nat. Biotechnol. 29, 143–148. doi:10.1038/nbt.1755
Miyoshi, H., Blomer, U., Takahashi, M., Gage, F. H., and Verma, I. M. (1998). Development of a self-inactivating lentivirus vector. J. Virol. 72, 8150–8157. doi:10.1128/JVI.72.10.8150-8157.1998
Moscou, M. J., and Bogdanove, A. J. (2009). A simple cipher governs DNA recognition by TAL effectors. Science 326, 1501. doi:10.1126/science.1178817
Mu, X., Greenwald, E., Ahmad, S., and Hur, S. (2018). An origin of the immunogenicity of in vitro transcribed RNA. Nucleic Acids Res. 46, 5239–5249. doi:10.1093/nar/gky177
Mukai, H., Ogawa, K., Kato, N., and Kawakami, S. (2022). Recent advances in lipid nanoparticles for delivery of nucleic acid, mRNA, and gene editing-based therapeutics. Drug Metab. Pharmacokinet. 44, 100450. doi:10.1016/j.dmpk.2022.100450
Naphade, S., Sharma, J., Gaide Chevronnay, H. P., Shook, M. A., Yeagy, B. A., Rocca, C. J., et al. (2015). Brief reports: Lysosomal cross-correction by hematopoietic stem cell-derived macrophages via tunneling nanotubes. Stem Cells 33, 301–309. doi:10.1002/stem.1835
Nelson, J., Sorensen, E. W., Mintri, S., Rabideau, A. E., Zheng, W., Besin, G., et al. (2020). Impact of mRNA chemistry and manufacturing process on innate immune activation. Sci. Adv. 6, eaaz6893. doi:10.1126/sciadv.aaz6893
Onfelt, B., Nedvetzki, S., Benninger, R. K., Purbhoo, M. A., Sowinski, S., Hume, A. N., et al. (2006). Structurally distinct membrane nanotubes between human macrophages support long-distance vesicular traffic or surfing of bacteria. J. Immunol. 177, 8476–8483. doi:10.4049/jimmunol.177.12.8476
Onfelt, B., Nedvetzki, S., Yanagi, K., and Davis, D. M. (2004). Cutting edge: Membrane nanotubes connect immune cells. J. Immunol. 173, 1511–1513. doi:10.4049/jimmunol.173.3.1511
Pattabhi, S., Lotti, S. N., Berger, M. P., Singh, S., Lux, C. T., Jacoby, K., et al. (2019). In vivo outcome of homology-directed repair at the HBB gene in HSC using alternative donor template delivery methods. Mol. Ther. Nucleic Acids 17, 277–288. doi:10.1016/j.omtn.2019.05.025
Pattanayak, V., Ramirez, C. L., Joung, J. K., and Liu, D. R. (2011). Revealing off-target cleavage specificities of zinc-finger nucleases by in vitro selection. Nat. Methods 8, 765–770. doi:10.1038/nmeth.1670
Pavani, G., Laurent, M., Fabiano, A., Cantelli, E., Sakkal, A., Corre, G., et al. (2020). Author correction: Ex vivo editing of human hematopoietic stem cells for erythroid expression of therapeutic proteins. Nat. Commun. 11, 4146. doi:10.1038/s41467-020-18036-0
Pavel-Dinu, M., Wiebking, V., Dejene, B. T., Srifa, W., Mantri, S., Nicolas, C. E., et al. (2019). Author Correction: Gene correction for SCID-X1 in long-term hematopoietic stem cells. Nat. Commun. 10, 5624. doi:10.1038/s41467-019-13620-5
Pelus, L. M., Bian, H., King, A. G., and Fukuda, S. (2004). Neutrophil-derived MMP-9 mediates synergistic mobilization of hematopoietic stem and progenitor cells by the combination of G-CSF and the chemokines GRObeta/CXCL2 and GRObetaT/CXCL2delta4. Blood 103, 110–119. doi:10.1182/blood-2003-04-1115
Petrillo, C., Thorne, L. G., Unali, G., Schiroli, G., Giordano, A. M. S., Piras, F., et al. (2018). Cyclosporine H overcomes innate immune restrictions to improve lentiviral transduction and gene editing in human hematopoietic stem cells. Cell. Stem Cell. 23, 820–832. doi:10.1016/j.stem.2018.10.008
Philippe, S., Sarkis, C., Barkats, M., Mammeri, H., Ladroue, C., Petit, C., et al. (2006). Lentiviral vectors with a defective integrase allow efficient and sustained transgene expression in vitro and in vivo. Proc. Natl. Acad. Sci. U. S. A. 103, 17684–17689. doi:10.1073/pnas.0606197103
Piras, G., Montiel-Equihua, C., Chan, Y. A., Wantuch, S., Stuckey, D., Burke, D., et al. (2020). Lentiviral hematopoietic stem cell gene therapy rescues clinical phenotypes in a murine model of Pompe disease. Mol. Ther. Methods Clin. Dev. 18, 558–570. doi:10.1016/j.omtm.2020.07.001
Rai, R., Romito, M., Rivers, E., Turchiano, G., Blattner, G., Vetharoy, W., et al. (2020). Targeted gene correction of human hematopoietic stem cells for the treatment of Wiskott - Aldrich Syndrome. Nat. Commun. 11, 4034. doi:10.1038/s41467-020-17626-2
Rai, R., Thrasher, A. J., and Cavazza, A. (2021). Gene editing for the treatment of primary immunodeficiency diseases. Hum. Gene Ther. 32, 43–51. doi:10.1089/hum.2020.185
Reint, G., Li, Z., Labun, K., Keskitalo, S., Soppa, I., Mamia, K., et al. (2021). Rapid genome editing by CRISPR-Cas9-POLD3 fusion. Elife 10, e75415. doi:10.7554/eLife.75415
Rekers, P. E., Coulter, M. P., and Warren, S. L. (1950). Effect of transplantation of bone marrow into irradiated animals. Archives Surg. 60, 635–667. doi:10.1001/archsurg.1950.01250010656001
Reshetnikov, V. V., Chirinskaite, A. V., Sopova, J. V., Ivanov, R. A., and Leonova, E. I. (2022). Translational potential of base-editing tools for gene therapy of monogenic diseases. Front. Bioeng. Biotechnol. 10, 942440. doi:10.3389/fbioe.2022.942440
Richardson, C. D., Kazane, K. R., Feng, S. J., Zelin, E., Bray, N. L., Schafer, A. J., et al. (2018). CRISPR-Cas9 genome editing in human cells occurs via the Fanconi anemia pathway. Nat. Genet. 50, 1132–1139. doi:10.1038/s41588-018-0174-0
Riesenberg, S., Helmbrecht, N., Kanis, P., Maricic, T., and Paabo, S. (2022). Improved gRNA secondary structures allow editing of target sites resistant to CRISPR-Cas9 cleavage. Nat. Commun. 13, 489. doi:10.1038/s41467-022-28137-7
Rocca, C. J., and Cherqui, S. (2019). Potential use of stem cells as a therapy for cystinosis. Pediatr. Nephrol. 34, 965–973. doi:10.1007/s00467-018-3974-7
Rocca, C. J., Goodman, S. M., Dulin, J. N., Haquang, J. H., Gertsman, I., Blondelle, J., et al. (2017). Transplantation of wild-type mouse hematopoietic stem and progenitor cells ameliorates deficits in a mouse model of Friedreich's ataxia. Sci. Transl. Med. 9, eaaj2347. doi:10.1126/scitranslmed.aaj2347
Rocca, C. J., Kreymerman, A., Ur, S. N., Frizzi, K. E., Naphade, S., Lau, A., et al. (2015). Treatment of inherited eye defects by systemic hematopoietic stem cell transplantation. Invest. Ophthalmol. Vis. Sci. 56, 7214–7223. doi:10.1167/iovs.15-17107
Rocca, C. J., Rainaldi, J. N., Sharma, J., Shi, Y., Haquang, J. H., Luebeck, J., et al. (2020). CRISPR-Cas9 gene editing of hematopoietic stem cells from patients with friedreich's ataxia. Mol. Ther. Methods Clin. Dev. 17, 1026–1036. doi:10.1016/j.omtm.2020.04.018
Roman-Rodriguez, F. J., Ugalde, L., Alvarez, L., Diez, B., Ramirez, M. J., Risueno, C., et al. (2019). NHEJ-mediated repair of CRISPR-cas9-induced DNA breaks efficiently corrects mutations in HSPCs from patients with fanconi anemia. Cell. Stem Cell. 25, 607–621. e607. doi:10.1016/j.stem.2019.08.016
Romero, Z., Lomova, A., Said, S., Miggelbrink, A., Kuo, C. Y., Campo-Fernandez, B., et al. (2019). Editing the sickle cell disease mutation in human hematopoietic stem cells: Comparison of endonucleases and homologous donor templates. Mol. Ther. 27, 1389–1406. doi:10.1016/j.ymthe.2019.05.014
Rosenblum, D., Gutkin, A., Kedmi, R., Ramishetti, S., Veiga, N., Jacobi, A. M., et al. (2020). CRISPR-Cas9 genome editing using targeted lipid nanoparticles for cancer therapy. Sci. Adv. 6, eabc9450. doi:10.1126/sciadv.abc9450
Roth, T. L., Puig-Saus, C., Yu, R., Shifrut, E., Carnevale, J., Li, P. J., et al. (2018). Reprogramming human T cell function and specificity with non-viral genome targeting. Nature 559, 405–409. doi:10.1038/s41586-018-0326-5
Rustom, A., Saffrich, R., Markovic, I., Walther, P., and Gerdes, H. H. (2004). Nanotubular highways for intercellular organelle transport. Science 303, 1007–1010. doi:10.1126/science.1093133
Ryan, M. A., Nattamai, K. J., Xing, E., Schleimer, D., Daria, D., Sengupta, A., et al. (2010). Pharmacological inhibition of EGFR signaling enhances G-CSF-induced hematopoietic stem cell mobilization. Nat. Med. 16, 1141–1146. doi:10.1038/nm.2217
Sago, C. D., Lokugamage, M. P., Islam, F. Z., Krupczak, B. R., Sato, M., and Dahlman, J. E. (2018a). Nanoparticles that deliver RNA to bone marrow identified by in vivo directed evolution. J. Am. Chem. Soc. 140, 17095–17105. doi:10.1021/jacs.8b08976
Sago, C. D., Lokugamage, M. P., Paunovska, K., Vanover, D. A., Monaco, C. M., Shah, N. N., et al. (2018b). High-throughput in vivo screen of functional mRNA delivery identifies nanoparticles for endothelial cell gene editing. Proc. Natl. Acad. Sci. U. S. A. 115, E9944–E9952. doi:10.1073/pnas.1811276115
Sakuma, T., Barry, M. A., and Ikeda, Y. (2012). Lentiviral vectors: Basic to translational. Biochem. J. 443, 603–618. doi:10.1042/BJ20120146
Salisbury-Ruf, C. T., and Larochelle, A. (2021). Advances and obstacles in homology-mediated gene editing of hematopoietic stem cells. J. Clin. Med. 10, 513. doi:10.3390/jcm10030513
Scharenberg, S. G., Poletto, E., Lucot, K. L., Colella, P., Sheikali, A., Montine, T. J., et al. (2020). Engineering monocyte/macrophage-specific glucocerebrosidase expression in human hematopoietic stem cells using genome editing. Nat. Commun. 11, 3327. doi:10.1038/s41467-020-17148-x
Schiroli, G., Conti, A., Ferrari, S., Della Volpe, L., Jacob, A., Albano, L., et al. (2019). Precise gene editing preserves hematopoietic stem cell function following transient p53-mediated DNA damage response. Cell. Stem Cell. 24, 551–565. e558. doi:10.1016/j.stem.2019.02.019
Schiroli, G., Ferrari, S., Conway, A., Jacob, A., Capo, V., Albano, L., et al. (2017). Preclinical modeling highlights the therapeutic potential of hematopoietic stem cell gene editing for correction of SCID-X1. Sci. Transl. Med. 9, eaan0820. doi:10.1126/scitranslmed.aan0820
Sergijenko, A., Langford-Smith, A., Liao, A. Y., Pickford, C. E., Mcdermott, J., Nowinski, G., et al. (2013). Myeloid/Microglial driven autologous hematopoietic stem cell gene therapy corrects a neuronopathic lysosomal disease. Mol. Ther. 21, 1938–1949. doi:10.1038/mt.2013.141
Sik Jung, E., Hun Kim, J., Chang, M. Y., Hong, W., Quan, Z., Hyun Kim, S., et al. (2022). Generation of mutation-corrected induced pluripotent stem cell lines derived from adrenoleukodystrophy patient by using homology directed repair. Stem Cell. Res. 59, 102664. doi:10.1016/j.scr.2022.102664
Smith, J., Bibikova, M., Whitby, F. G., Reddy, A. R., Chandrasegaran, S., and Carroll, D. (2000). Requirements for double-strand cleavage by chimeric restriction enzymes with zinc finger DNA-recognition domains. Nucleic Acids Res. 28, 3361–3369. doi:10.1093/nar/28.17.3361
Sorek, R., Lawrence, C. M., and Wiedenheft, B. (2013). CRISPR-mediated adaptive immune systems in bacteria and archaea. Annu. Rev. Biochem. 82, 237–266. doi:10.1146/annurev-biochem-072911-172315
Sowinski, S., Alakoskela, J. M., Jolly, C., and Davis, D. M. (2011). Optimized methods for imaging membrane nanotubes between T cells and trafficking of HIV-1. Methods 53, 27–33. doi:10.1016/j.ymeth.2010.04.002
Sternberg, S. H., Redding, S., Jinek, M., Greene, E. C., and Doudna, J. A. (2014). DNA interrogation by the CRISPR RNA-guided endonuclease Cas9. Nature 507, 62–67. doi:10.1038/nature13011
Steward, C. G., and Jarisch, A. (2005). Haemopoietic stem cell transplantation for genetic disorders. Arch. Dis. Child. 90, 1259–1263. doi:10.1136/adc.2005.074278
Szade, A., Szade, K., Nowak, W. N., Bukowska-Strakova, K., Muchova, L., Gonka, M., et al. (2019). Cobalt protoporphyrin IX increases endogenous G-CSF and mobilizes HSC and granulocytes to the blood. EMBO Mol. Med. 11, e09571. doi:10.15252/emmm.201809571
Szade, K., Gulati, G. S., Chan, C. K. F., Kao, K. S., Miyanishi, M., Marjon, K. D., et al. (2018). Where hematopoietic stem cells live: The bone marrow niche. Antioxid. Redox Signal 29, 191–204. doi:10.1089/ars.2017.7419
Taya, Y., Ota, Y., Wilkinson, A. C., Kanazawa, A., Watarai, H., Kasai, M., et al. (2016). Depleting dietary valine permits nonmyeloablative mouse hematopoietic stem cell transplantation. Science 354, 1152–1155. doi:10.1126/science.aag3145
Thomas, E. D., Buckner, C. D., Banaji, M., Clift, R. A., Fefer, A., Flournoy, N., et al. (1977). One hundred patients with acute leukemia treated by chemotherapy, total body irradiation, and allogeneic marrow transplantation. Blood 49, 511–533. doi:10.1182/blood.v49.4.511.bloodjournal494511
Thomas, E. D., Buckner, C. D., Clift, R. A., Fefer, A., Johnson, F. L., Neiman, P. E., et al. (1979). Marrow transplantation for acute nonlymphoblastic leukemia in first remission. N. Engl. J. Med. 301, 597–599. doi:10.1056/NEJM197909133011109
Thomas, E. D., Lochte, H. L., Lu, W. C., and Ferrebee, J. W. (1957). Intravenous infusion of bone marrow in patients receiving radiation and chemotherapy. N. Engl. J. Med. 257, 491–496. doi:10.1056/NEJM195709122571102
Thompson, A. A., Walters, M. C., Kwiatkowski, J., Rasko, J. E. J., Ribeil, J. A., Hongeng, S., et al. (2018). Gene therapy in patients with transfusion-dependent beta-thalassemia. N. Engl. J. Med. 378, 1479–1493. doi:10.1056/NEJMoa1705342
Tiwari, V., Koganti, R., Russell, G., Sharma, A., and Shukla, D. (2021). Role of tunneling nanotubes in viral infection, neurodegenerative disease, and cancer. Front. Immunol. 12, 680891. doi:10.3389/fimmu.2021.680891
Tombacz, I., Laczko, D., Shahnawaz, H., Muramatsu, H., Natesan, A., Yadegari, A., et al. (2021). Highly efficient CD4+ T cell targeting and genetic recombination using engineered CD4+ cell-homing mRNA-LNPs. Mol. Ther. 29, 3293–3304. doi:10.1016/j.ymthe.2021.06.004
Triana-Alonso, F. J., Dabrowski, M., Wadzack, J., and Nierhaus, K. H. (1995). Self-coded 3'-extension of run-off transcripts produces aberrant products during in vitro transcription with T7 RNA polymerase. J. Biol. Chem. 270, 6298–6307. doi:10.1074/jbc.270.11.6298
Tucci, F., Scaramuzza, S., Aiuti, A., and Mortellaro, A. (2021). Update on clinical ex vivo hematopoietic stem cell gene therapy for inherited monogenic diseases. Mol. Ther. 29, 489–504. doi:10.1016/j.ymthe.2020.11.020
Urnov, F. D., Miller, J. C., Lee, Y. L., Beausejour, C. M., Rock, J. M., Augustus, S., et al. (2005). Highly efficient endogenous human gene correction using designed zinc-finger nucleases. Nature 435 (7042), 646–651. doi:10.1038/nature03556
Vaidyanathan, S., Azizian, K. T., Haque, A., Henderson, J. M., Hendel, A., Shore, S., et al. (2018). Uridine depletion and chemical modification increase Cas9 mRNA activity and reduce immunogenicity without HPLC purification. Mol. Ther. Nucleic Acids 12, 530–542. doi:10.1016/j.omtn.2018.06.010
Vakulskas, C. A., Dever, D. P., Rettig, G. R., Turk, R., Jacobi, A. M., Collingwood, M. A., et al. (2018). A high-fidelity Cas9 mutant delivered as a ribonucleoprotein complex enables efficient gene editing in human hematopoietic stem and progenitor cells. Nat. Med. 24, 1216–1224. doi:10.1038/s41591-018-0137-0
Varvel, N. H., Grathwohl, S. A., Baumann, F., Liebig, C., Bosch, A., Brawek, B., et al. (2012). Microglial repopulation model reveals a robust homeostatic process for replacing CNS myeloid cells. Proc. Natl. Acad. Sci. U. S. A. 109, 18150–18155. doi:10.1073/pnas.1210150109
Veres, A., Gosis, B. S., Ding, Q., Collins, R., Ragavendran, A., Brand, H., et al. (2014). Low incidence of off-target mutations in individual CRISPR-Cas9 and TALEN targeted human stem cell clones detected by whole-genome sequencing. Cell. Stem Cell. 15, 27–30. doi:10.1016/j.stem.2014.04.020
Visigalli, I., Delai, S., Politi, L. S., Di Domenico, C., Cerri, F., Mrak, E., et al. (2010a). Gene therapy augments the efficacy of hematopoietic cell transplantation and fully corrects mucopolysaccharidosis type I phenotype in the mouse model. Blood 116, 5130–5139. doi:10.1182/blood-2010-04-278234
Visigalli, I., Ungari, S., Martino, S., Park, H., Cesani, M., Gentner, B., et al. (2010b). The galactocerebrosidase enzyme contributes to the maintenance of a functional hematopoietic stem cell niche. Blood 116, 1857–1866. doi:10.1182/blood-2009-12-256461
Walton, R. T., Christie, K. A., Whittaker, M. N., and Kleinstiver, B. P. (2020). Unconstrained genome targeting with near-PAMless engineered CRISPR-Cas9 variants. Science 368, 290–296. doi:10.1126/science.aba8853
Wang, J., Exline, C. M., Declercq, J. J., Llewellyn, G. N., Hayward, S. B., Li, P. W., et al. (2015). Homology-driven genome editing in hematopoietic stem and progenitor cells using ZFN mRNA and AAV6 donors. Nat. Biotechnol. 33, 1256–1263. doi:10.1038/nbt.3408
Wilkinson, A. C., Dever, D. P., Baik, R., Camarena, J., Hsu, I., Charlesworth, C. T., et al. (2021). Cas9-AAV6 gene correction of beta-globin in autologous HSCs improves sickle cell disease erythropoiesis in mice. Nat. Commun. 12, 686. doi:10.1038/s41467-021-20909-x
Wilkinson, A. C., Igarashi, K. J., and Nakauchi, H. (2020). Haematopoietic stem cell self-renewal in vivo and ex vivo. Nat. Rev. Genet. 21, 541–554. doi:10.1038/s41576-020-0241-0
Wu, M. Z., Asahara, H., Tzertzinis, G., and Roy, B. (2020). Synthesis of low immunogenicity RNA with high-temperature in vitro transcription. RNA 26, 345–360. doi:10.1261/rna.073858.119
Xie, L., Hu, Y., Li, L., Jiang, L., Jiao, Y., Wang, Y., et al. (2022). Expanding PAM recognition and enhancing base editing activity of Cas9 variants with non-PI domain mutations derived from xCas9. FEBS J. 289, 5899–5913. doi:10.1111/febs.16457
Xu, L., Yang, H., Gao, Y., Chen, Z., Xie, L., Liu, Y., et al. (2017). CRISPR/Cas9-Mediated CCR5 ablation in human hematopoietic stem/progenitor cells confers HIV-1 resistance in vivo. Mol. Ther. 25, 1782–1789. doi:10.1016/j.ymthe.2017.04.027
Yamada, T., Ohyagi, Y., Shinnoh, N., Kikuchi, H., Osoegawa, M., Ochi, H., et al. (2004). Therapeutic effects of normal cells on ABCD1 deficient cells in vitro and hematopoietic cell transplantation in the X-ALD mouse model. J. Neurol. Sci. 218, 91–97. doi:10.1016/j.jns.2003.11.006
Yanez-Munoz, R. J., Balaggan, K. S., Macneil, A., Howe, S. J., Schmidt, M., Smith, A. J., et al. (2006). Effective gene therapy with nonintegrating lentiviral vectors. Nat. Med. 12, 348–353. doi:10.1038/nm1365
Yang, H., Qing, K., Keeler, G. D., Yin, L., Mietzsch, M., Ling, C., et al. (2020). Enhanced transduction of human hematopoietic stem cells by AAV6 vectors: Implications in gene therapy and genome editing. Mol. Ther. Nucleic Acids 20, 451–458. doi:10.1016/j.omtn.2020.03.009
Yoshimitsu, M., Higuchi, K., Ramsubir, S., Nonaka, T., Rasaiah, V. I., Siatskas, C., et al. (2007). Efficient correction of Fabry mice and patient cells mediated by lentiviral transduction of hematopoietic stem/progenitor cells. Gene Ther. 14, 256–265. doi:10.1038/sj.gt.3302839
Zeiser, R., and Blazar, B. R. (2017). Acute graft-versus-host disease - biologic process, prevention, and therapy. N. Engl. J. Med. 377, 2167–2179. doi:10.1056/NEJMra1609337
Zetsche, B., Gootenberg, J. S., Abudayyeh, O. O., Slaymaker, I. M., Makarova, K. S., Essletzbichler, P., et al. (2015). Cpf1 is a single RNA-guided endonuclease of a class 2 CRISPR-Cas system. Cell. 163, 759–771. doi:10.1016/j.cell.2015.09.038
Zhang, G., Liu, Y., Huang, S., Qu, S., Cheng, D., Yao, Y., et al. (2022). Enhancement of prime editing via xrRNA motif-joined pegRNA. Nat. Commun. 13, 1856. doi:10.1038/s41467-022-29507-x
Zhang, H., Sun, R., Fei, J., Chen, H., and Lu, D. (2022a). Correction of beta-thalassemia IVS-II-654 mutation in a mouse model using prime editing. Int. J. Mol. Sci. 23, 5948. doi:10.3390/ijms23115948
Zhang, H., Zhou, Q., Chen, H., and Lu, D. (2022b). Prime editor 3 mediated beta-thalassemia mutations of the HBB gene in human erythroid progenitor cells. Int. J. Mol. Sci. 23, 5002. doi:10.3390/ijms23095002
Zhang, J. P., Li, X. L., Li, G. H., Chen, W., Arakaki, C., Botimer, G. D., et al. (2017). Efficient precise knockin with a double cut HDR donor after CRISPR/Cas9-mediated double-stranded DNA cleavage. Genome Biol. 18, 35. doi:10.1186/s13059-017-1164-8
Keywords: gene editing, hematopoietic stem and progenitor cells, inherited disorders, base editing, prime editing, ZFN, TALEN and CRISPR/Cas9
Citation: Buffa V, Alvarez Vargas JR, Galy A, Spinozzi S and Rocca CJ (2023) Hematopoietic stem and progenitors cells gene editing: Beyond blood disorders. Front. Genome Ed. 4:997142. doi: 10.3389/fgeed.2022.997142
Received: 18 July 2022; Accepted: 19 December 2022;
Published: 09 January 2023.
Edited by:
Sjaak Philipsen, Erasmus Medical Center, NetherlandsReviewed by:
Saravanabhavan Thangavel, Center for Stem Cell Research (CSCR), IndiaJosé Carlos Segovia Sanz, Medioambientales y Tecnológicas, Spain
Copyright © 2023 Buffa, Alvarez Vargas, Galy, Spinozzi and Rocca. This is an open-access article distributed under the terms of the Creative Commons Attribution License (CC BY). The use, distribution or reproduction in other forums is permitted, provided the original author(s) and the copyright owner(s) are credited and that the original publication in this journal is cited, in accordance with accepted academic practice. No use, distribution or reproduction is permitted which does not comply with these terms.
*Correspondence: Céline J. Rocca, Y2VsaW5lLnJvY2NhQGdtYWlsLmNvbQ==