- 1Queensland Alliance for Agriculture and Food Innovation, University of Queensland, Brisbane, QLD, Australia
- 2ARC Centre for Plant Success in Nature and Agriculture, University of Queensland, Brisbane, QLD, Australia
Rice, a staple food worldwide and a model crop, could benefit from the introduction of novel genetics from wild relatives. Wild rice in the AA genome group closely related to domesticated rice is found across the tropical world. Due to their locality outside the range of domesticated rice, Australian wild rice populations are a potential source of unique traits for rice breeding. These rice species provide a diverse gene pool for improvement that could be utilized for desirable traits such as stress resistance, disease tolerance, and nutritional qualities. However, they remain poorly characterized. The CRISPR/Cas system has revolutionized gene editing and has improved our understanding of gene functions. Coupled with the increasing availability of genomic information on the species, genes in Australian wild rice could be modified through genome editing technologies to produce new domesticates. Alternatively, beneficial alleles from these rice species could be incorporated into cultivated rice to improve critical traits. Here, we summarize the beneficial traits in Australian wild rice, the available genomic information and the potential of gene editing to discover and understand the functions of novel alleles. Moreover, we discuss the potential domestication of these wild rice species for health and economic benefits to rice production globally.
Introduction
The CRISPR-Cas system has quickly gained popularity as a strong and widely used tool for genome editing as compared to traditional inefficient and laborious random mutagenesis and screening methods (Ma X. et al., 2015; McCarty et al., 2020). The introduction of genome edits, like substitutions, insertions, and deletions, using the CRISPR-Cas 9 system, can speed up the breeding of plants including rice (Romero and Gatica-Arias, 2019). Australian wild rice represents an untapped source of important alleles that are missing from the rice gene pool (Henry et al., 2010). To ensure rice food security, it is necessary to increase productivity which relies on continuous genetic improvement (Brar and Khush, 2018; Henry, 2019). The wild rice species have higher drought, salinity, lodging, disease, and insect resistance than the most tolerant or resistant rice genotype. Additionally, they have unique traits such as acid soil tolerance, shade tolerance, high micronutrient content and are not only known to tolerate biotic and abiotic stress but also to exhibit extraordinary growth and development traits, such as profuse tillering and the existence of a salt gland that might be transferred to cultivated rice, increasing production and profitability (Henry, 2018; Moner and Henry, 2018).
The primary gene pool of rice comprises the Oryza A-genome species that are easily interfertile with rice (Wambugu et al., 2015). Previous research indicates two separate and unique perennial wild populations in tropical Australia (Brozynska et al., 2017; Moner et al., 2020), an O. rufipogon-like population, that has been referred to as Taxa-A, and O. meridionalis, including both perennial and annual forms and sometimes, referred to as Taxa-B. Genome analysis suggests that the O. meridionalis populations diverged from the lineage that became O. sativa approximately 3 Mya, while the Australian O. rufipogon like populations diverged approximately 1.6 Mya. The phylogenetic relationships between these species have been studied using both chloroplast and nuclear genome sequences (Wambugu et al., 2015; Brozynska et al., 2017). Taxa A (O. rufipogon-like taxa) has a chloroplast that is more similar to that of O. meridionalis and a nuclear genome that is more similar to that of O. rufipogon from Asia (Brozynska et al., 2017). A recent analysis of these taxa has confirmed that there is ongoing reticulate evolution, with rare hybrid plants being found in the wild (Hasan et al., 2022). O. meridionalis is the most distant species in the AA genome group that includes domesticated rice making it a significant resource for improving rice and studying rice evolution. In addition to being a source of slowly digestible starch and higher amylose content, its photosynthetic traits and abiotic stress tolerance make it an excellent candidate for use in the rice improvement (Scafaro et al., 2009; Tikapunya et al., 2017). Until recently, Australian wild rice was generally undisturbed by the impact of rice domestication, resulting in the persistence of wild Oryza in vast populations across a large area of northern Australia (Henry et al., 2010). These Australian Oryza may be critical in adapting rice to rapidly changing climate conditions and altering consumer preferences and needs. Moreover, recent data reveals that, even though the rice was first domesticated in Asia, Australian wild rice populations have provided genes to the domestication of rice (Huang et al., 2012; Fujino et al., 2019).
Seed shattering is a significant drawback affecting yield loss in both taxa of Australian wild rice. Gene editing using CRISPR-Cas to induce loss of function in shattering genes could allow rapid production of potentially new wild rice cultivars (Bohra et al., 2021). Advancement in genome and transcriptome sequencing has been a major contributor to improving gene target identification. The genomes of many wild rice species have been sequenced allowing the discovery of the genes responsible for desirable characteristics. The availability of these genetic resources is highly beneficial in supporting molecular breeding by horizontal transfer of key traits from wild species to cultivated rice.
In this review, we will discuss genome editing and how it has been used to capture diversity in rice (Figure 1). Furthermore, we will discuss how the function of novel alleles have been identified in domesticated rice using CRISPR/cas9 and how these studies can guide the identification of useful alleles in wild rice (especially in the Australian species) with the potential of being used in rice breeding.
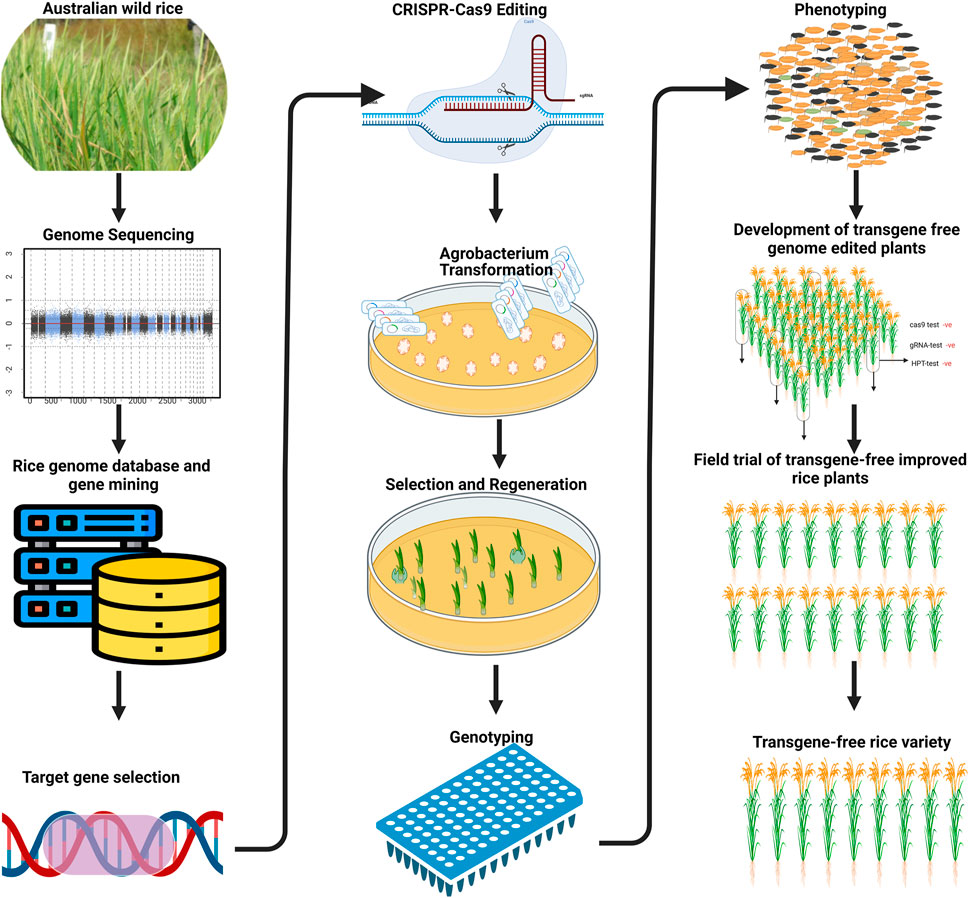
FIGURE 1. Schematic diagram shows the denovo domestication of Australian wild rice through genome editing.
Gene Editing of Rice
Genome editing tools have broadened the range of options for rice research and improvement, giving scientists innovative ways to make new varieties that are more productive and better for the environment. The small size of the rice genome, high efficiency of transformation, abundance of genetic resources, and genomic synteny with other cereals provides an excellent model system for the study of functional genomics. In recent years, rice has been used to evaluate the efficacy of several genome editing methods, as well as to explore gene functions and their potential in the rice improvement (Li et al., 2012; Feng et al., 2013; Zafar et al., 2020) as briefly discussed below and highlighted in Table 1. CRISPR/Cas9-mediated editing of the bsr-k1 gene produced higher-yielding rice plants resistant to leaf blast and bacterial leaf blight (Zhou et al., 2018). When Bsr-d1, Pi21, and ERF922 were mutated using CRISPER/Cas-9 in all single and triple mutants of TGMS rice line (Indica thermosensitive genic male sterile) and longke638S (LK638S) were more resistant to rice blast than the wild type (Zhou et al., 2021). To find new sources of RTD (rice tungro disease) resistance, a CRISPR/Cas9 system was used to create mutations in the eIF4G gene in the RTSV-susceptible variety IR64, which is grown all over tropical Asia. eIF4G alleles with mutations in the SVLFPNLAGKS (mostly NL) close to the YVV residues were the only ones that were identified resistant (Macovei et al., 2018). Overexpression of OsAAP3 in transgenic plants resulted in reduced bud outgrowth and rice tillering while OsAAP3 RNAi slightly reduced the transport of amino acids, with lower concentrations of Arg, Lys, Asp, and Thr, but increased the number of bud outgrowth, tillers, grain production, and nitrogen usage efficiency (NUE). OsAAP3 promoter sequences differed in Japonica and Indica rice, and expression was higher in Japonica, which had fewer tillers. CRISPR technology was used to create OsAAP3 knockout lines in Japonica ZH11 and KY131 resulting in an increased grain yield (Lu et al., 2018).
Recent Advances in Editing Technology
The CRISPR-Cas9 system is mainly confined to genome editing at canonical NGG protospacer adjacent motif (PAM) sites. These sites are extremely important for nuclease identification, cleavage and efficient editing. Cas9 orthologs with changed PAM specificities have been discovered such as SaCas9 (Staphylococcus aureus) and Cas9-VQR (D1135V/R1335Q/T1337R) (Kleinstiver et al., 2015; Hu et al., 2016). Cas9-VQR has been designed to cleaves the sites containing a NGA PAM, however its editing efficiency was found to be insufficient in rice. To boost the VQR variant’s editing efficiency, the sgRNA structure was changed and significantly increased the editing efficiency (Hu et al., 2018). The CRISPR-SaCas9 toolkit was recently refined in rice by adding three important mutations (E782K/N968K/R105H) to improve the editing efficiency (Qin et al., 2019; Zafar et al., 2020). The editing efficiency of SaCas9 in the PDS and DL genes was determined via Agrobacterium-mediated transformation of Japonica rice. After mutagenesis, 34 out of 53 lines (64.2%) and 28 out of 36 (77.8%) lines had targeted mutations in the PDS/T1 and DL/T1 areas, respectively (Qin et al., 2019).
Cas9 with extended PAM SpCas9 (xCas9) and Cas9-NG (Cas9-NG) have also been tried in rice (Zhong et al., 2019) with xCas9 technology showing a better outcome in the rice genome editing (Wang J. et al., 2019; Endo et al., 2019). These enzymes can detect NG and GAA PAMs. The Cas9-NG also detects non-canonical PAM sites such as NCGAA and NG in addition to NCG (Ren et al., 2019; Zhong et al., 2019). These findings have broadened the breadth of rice genome editing.
Base editing is a novel approach to genome editing that enables irreversible base alterations at target loci without the use of double-stranded breaks or homology guided repair. (Hua et al., 2019). The combination of Cas9 nickase and cytidine deaminase enzymes allows for the creation of C to T or G to A substitutions anywhere in the genome (Komor et al., 2016; Mishra et al., 2018). For instance, the substitution of C-to-T in the OsALS gene resulted in an amino acid change at position 96 from alanine to valine conferred herbicide tolerance in Oryza sativa L (cv. Nipponbare) (Sun et al., 2016; Shimatani et al., 2017) (Table 1).
The tandem use of adenine and cytosine base editors in rice also shows their potential for use in the rice improvement (Hua et al., 2018). Human APOBEC3A and Cas9 nickase were used together to improve the efficiency of base editors (Zong et al., 2018). This fusion protein effectively converts cytidine to thymidine, allowing for larger editing frames, from 5 to 17 nucleotides in rice (Zong et al., 2018). Other recent examples of better base editing toolkits include (ABE)-nCas9 tool, SpCas9-NGv1, and ABE-P1S (Hao et al., 2019; Negishi et al., 2019; Hua et al., 2020). Although base editing is a highly effective method for inducing point mutation with high efficiency, base editors can’t generate exact indels, transversions, insertions, or avoid other mutations (Lin et al., 2020).
In contrast, prime editors have the ability to insert any of the 12 conceivable transition and transversion mutations as well as minor indels into the genome. Prime editing is a revolutionary method of genome editing (Anzalone et al., 2019). Instead of using a donor repair template, prime editing installs the desired modifications directly into the pegRNA sequence. Over the last few years, several attempts have been made to develop a reliable primary editing system in rice, with some success in creating herbicide-tolerant cultivars of rice (Li et al., 2020). Base and prime editing could contribute to domesticating Australian wild rice and significantly improving cultivated rice to overcome food security challenges.
Applications of Gene Editing to Wild Rice Relatives
CRISPR-Cas technology allows for rapid de novo domestication of wild plant relatives. Traditional domestication requires considerable cross-breeding and selection of naturally occurring genetic alterations. Groundcherry (Physalis pruinosa) and wild tomato were recently de novo domesticated by utilizing genome editing (Li T. et al., 2018; Lemmon et al., 2018; Zhu and Zhu, 2021). Yu et al., 2021 outlined a de novo domestication strategy for Oryza alta, an allotetraploid rice with high biomass that is widely adapted to the environment (Yu et al., 2021). Yu et al., 2021 knocked out genes associated with seed shattering and awn length (qSH1 and An-1 orthologues), resulting in a considerably lower seed shattering rate and shorter awn length. To improve additional traits, they edited several orthologues of rice genes semi-dwarf stature (SD1), grain length and size (GS3), heading date (Ghd7, DTH7), and ideal plant architecture (IPA1) in O. alta. This remarkable study introduced a new era of rapid domestication of crops with desired traits by applying precise genome editing technologies. To domesticate a wild crop relative, it must have a well-sequenced genome and be amenable to tissue culture and transformation. The capacity to induce callus and regenerate plantlets is frequently a bottleneck to build a plant genetic transformation system. Only a few plant species, including a few Oryza sativa cultivars, have adequate and robust transformation procedures, several hurdles remain in applying genome editing to rice wild relatives.
Australian Wild Rice
Henry et al., 2010 reported four Australian wild relatives Oryza rufipogon like population (Taxa-A), Oryza meridionalis like population (Taxa-B), Oryza officinalis, and Oryza australiensis (Henry et al., 2010; Brozynska et al., 2017). The characterization of unique wild rice species in Australia, via genetic and morphological investigation, has led to the discovery of novel Oryza gene pools (Waters et al., 2012; Sotowa et al., 2013; Brozynska et al., 2014). The AA genome species of most interest have been described above but the much more divergent O. australiensis is also of potential value in rice improvement. Oryza australiensis, the only known member of the E genome in the genus Oryza has unique characteristics such as an underground rhizome that a prospective source of novel genes for rice development because it allows plants to survive during the dry season (Henry et al., 2010). The relationships of Oryza australiensis with other species in the Oryza genus suggested that it may be useful in understanding the evolution of the Oryza genus. Oryza australiensis has a large and poorly characterised, with a high proportion of repeated sequences, making it challenging to study (Henry, 2018). In addition, the species shows outstanding grain properties, which suggests that it might potentially be used as a crop if domesticated (Tikapunya et al., 2016).
Genomic sequencing of these novel Australian wild rice species has been reported (Brozynska et al., 2017) but improved genome sequences are required to facilitate genome editing of rice to transfer their desirable traits.
Potential Applications to Introgression of Genes From Australian Wild Rice
Biotechnological and genomic breakthroughs in rice genomic studies have created new prospects for improving rice germplasm with unique genetic features and better knowledge of rice gene activity. High-yielding improved rice varieties have been developed by applying traditional breeding procedures and manipulating the rice (Oryza sativa) gene pool resulting in better quality features. The cultivated rice gene pool has little genetic diversity hence interspecific hybridization could play a role in introducing economically important agronomic traits from wild to cultivated rice. However, due to incompatible obstacles, including pre-and postfertilization barriers, seed shattering, hybrid sterility, poor grain properties, and linkage drag, gene transfer from wild to domesticated species is challenging (Brar and Khush, 2018). Interspecific hybridization has enabled researchers to get and measure the genetic diversity of aliens from different Oryza genomes. Wild rice species have provided functional genes that make plants resistant to bacterial blight, tungro, brown planthoppers and acidic soils (Table 2).
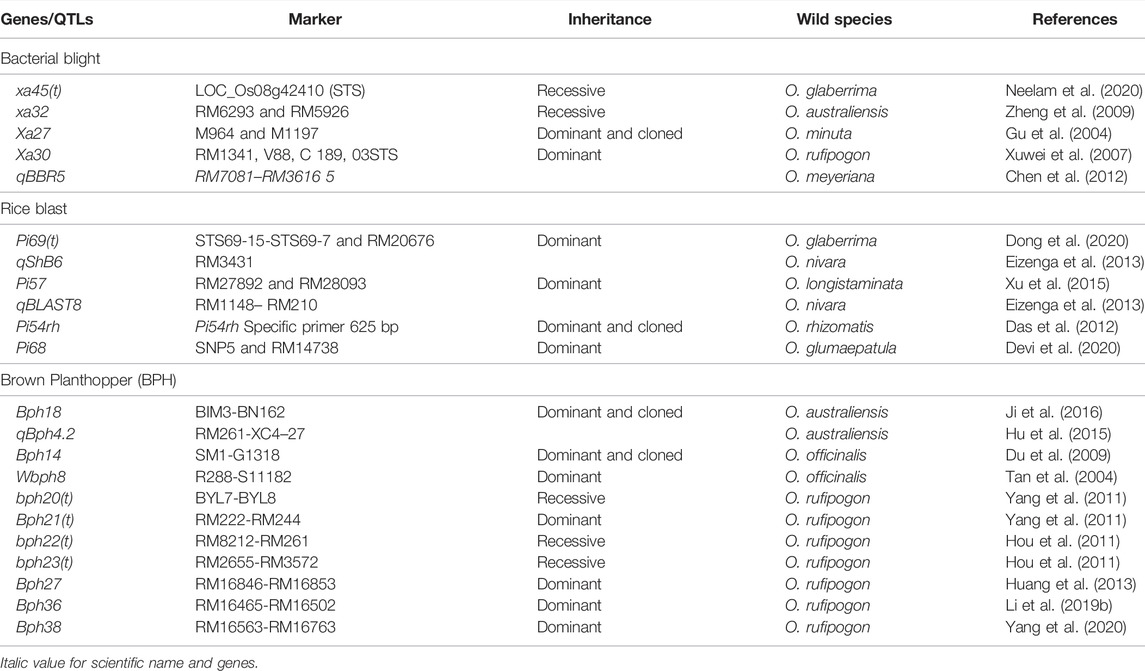
TABLE 2. List of the key biotic stress resistance genes and QTLs identified within wild rice species.
To capture useful genetic diversity, screening and phenotyping of many different accessions are very important. For example, only one O. nivara accession (IRGC101508) from India proved resistant to grassy stunt virus out of 6,000 cultivated and wild rice accessions examined.
Potential Applications to the Domestication of Australian Wild Rice
Population growth and climate change threaten global agriculture productivity. To feed 10 billion people by 2050 is a massive challenge. To meet the world’s food needs and increase crop yields quickly, existing methods of domesticating crops are insufficient. Together with a deeper understanding of domestication’s genetic foundation, provided by pangenomes, recent advancements in gene editing technologies open the intriguing probability of developing novel crops by modifying few genes in wild species. Using a new platform for domestication, it may be possible to convert crop wild relatives quickly and precisely into economically desirable crops while keeping some of the beneficial resilience and nutritional properties that have been lost during domestication and breeding.
Australian wild rice has many unique and novel traits that can feed the future population. Australian wild rice domestication can potentially be achieved by following and optimizing the de novo route highlighted by Li’s group; the development of a high-performance transformation system, putting together and annotating a high-quality reference genome, and editing several genes that are important for domestication, e.g., shattering, awn length, panicle architecture and nutritional benefits to improve a variety of features. In this way, genome engineering might be used to generate nutritionally and climate-smart crops from the start in a wide range of crops currently used for human consumption, food production, animal feed, or biofuel.
Future Prospects
Traditionally, domestication of wild plants into commercial crops took hundreds or even thousands of years, but newly emerging genome editing technologies enable this to be accomplished in a few generations (Van Tassel et al., 2020). As a result, effective genome editing techniques are critical for accelerating the speed of domestication. Only the O. sativa subspecies japonica and indica have been successfully transformed using Agrobacterium-mediated transformation systems (Hiei et al., 1994). To determine the most promising starting material, priority must be given to callus induction and regeneration capacities with suitable biomass traits and stress tolerance etc. During the domestication, traits that were good for farming instead of natural growth were chosen and improved, such as grain size, hull colour, erect growth, shattering, pericarp colour and awn etc (Chen et al., 2019). Many traits of Australian wild rice species are similar to those of the wild ancestors of the present cultivars because they are closely related. Identifying the wild rice homologs of the domestication-related genes from domesticated rice is the first step, for example qSH1gene homolog for seed shattering, Bh4 homolog gene for hull colour, An-1 and An-2 for awn length, Rc for pericarp colour, OsLG1 for panicle shape, and GW5 for grain width. Editing these homologs genes by utilising a CRISPR/Cas9-mutagenesis technique may genuinely achieve quick domestication of Australian wild rice.
Most crop improvements have involved targeted editing and transformation, which require the efficient transformation and precise large-scale genome editing system. For example, RNA viral vectors, may infect plants and deliver gene-editing reagents to the germline, inducing hundreds to thousands of different mutations. Using developmental regulators, altered somatic cells can generate meristems that produce seed-bearing branches, boosting productivity and minimizing timeframes (Nasti and Voytas, 2021). These and other techniques will allow faster breeding, domestication of Australian wild rice, and metabolic reengineering than previously conceivable. So, developing an efficient transformation and genome editing system for Australian wild rice is very important.
Furthermore, Australian wild rices have beneficial traits including biotic and abiotic stress tolerance that can be used in breeding programs for improved yield. Studies on the loss or gain of function of the genes associated with these traits need to be conducted to definitively understand their mechanisms and potentially edit them into cultivated rice varieties.
Author Contributions
MA write the draft, PO read carefully and give suggestions, AF technically helps and give the suggestions, RH give the outlines and idea of this review and technically improved with many revisions.
Funding
The Australian Research Council provided support for much of the research reviewed here.
Conflict of Interest
The authors declare that the research was conducted in the absence of any commercial or financial relationships that could be construed as a potential conflict of interest.
Publisher’s Note
All claims expressed in this article are solely those of the authors and do not necessarily represent those of their affiliated organizations, or those of the publisher, the editors and the reviewers. Any product that may be evaluated in this article, or claim that may be made by its manufacturer, is not guaranteed or endorsed by the publisher.
References
Abe, K., Araki, E., Suzuki, Y., Toki, S., and Saika, H. (2018). Production of High Oleic/low Linoleic rice by Genome Editing. Plant Physiol. Biochem. 131, 58–62. doi:10.1016/j.plaphy.2018.04.033
Anzalone, A. V., Randolph, P. B., Davis, J. R., Sousa, A. A., Koblan, L. W., Levy, J. M., et al. (2019). Search-and-replace Genome Editing without Double-Strand Breaks or Donor DNA. Nature 576 (7785), 149–157. doi:10.1038/s41586-019-1711-4
Ashokkumar, S., Jaganathan, D., Ramanathan, V., Rahman, H., Palaniswamy, R., Kambale, R., et al. (2020). Creation of Novel Alleles of Fragrance Gene OsBADH2 in rice through CRISPR/Cas9 Mediated Gene Editing. PLoS One 15 (8), e0237018. doi:10.1371/journal.pone.0237018
Bohra, A., Kilian, B., Sivasankar, S., Caccamo, M., Mba, C., McCouch, S. R., et al. (2022). Reap the Crop Wild Relatives for Breeding Future Crops. Trends Biotechnol. 40, 412–431. doi:10.1016/j.tibtech.2021.08.009
Brar, D. S., and Khush, G. S. (2018). “Wild Relatives of Rice: A Valuable Genetic Resource for Genomics and Breeding Research,” in The Wild Oryza Genomes (Springer), 1–25. doi:10.1007/978-3-319-71997-9_1
Brozynska, M., Copetti, D., Furtado, A., Wing, R. A., Crayn, D., Fox, G., et al. (2017). Sequencing of Australian Wild rice Genomes Reveals Ancestral Relationships with Domesticated rice. Plant Biotechnol. J. 15 (6), 765–774. doi:10.1111/pbi.12674
Brozynska, M., Omar, E. S., Furtado, A., Crayn, D., Simon, B., Ishikawa, R., et al. (2014). Chloroplast Genome of Novel Rice Germplasm Identified in Northern Australia. Trop. Plant Biol. 7 (3-4), 111–120. doi:10.1007/s12042-014-9142-8
Butt, H., Eid, A., Momin, A. A., Bazin, J., Crespi, M., Arold, S. T., et al. (2019). CRISPR Directed Evolution of the Spliceosome for Resistance to Splicing Inhibitors. Genome Biol. 20 (1), 73. doi:10.1186/s13059-019-1680-9
Chen, E., Huang, X., Tian, Z., Wing, R. A., and Han, B. (2019). The Genomics ofOryzaSpecies Provides Insights into Rice Domestication and Heterosis. Annu. Rev. Plant Biol. 70, 639–665. doi:10.1146/annurev-arplant-050718-100320
Chen, L.-N., Yang, Y., Yan, C.-Q., Wang, X.-M., Yu, C.-L., Zhou, J., et al. (2012). Identification of Quantitative Trait Loci for Bacterial Blight Resistance Derived from Oryza Meyeriana and Agronomic Traits in Recombinant Inbred Lines of Oryza Sativa. J. Phytopathology 160 (9), 461–468. doi:10.1111/j.1439-0434.2012.01931.x
Das, A., Soubam, D., Singh, P. K., Thakur, S., Singh, N. K., and Sharma, T. R. (2012). A Novel Blast Resistance Gene, Pi54rh Cloned from Wild Species of rice, Oryza Rhizomatis Confers Broad Spectrum Resistance to Magnaporthe Oryzae. Funct. Integr. Genomics 12 (2), 215–228. doi:10.1007/s10142-012-0284-1
Devi, S. J. S. R., Singh, K., Umakanth, B., Vishalakshi, B., Rao, K. V. S., Suneel, B., et al. (2020). Identification and Characterization of a Large Effect QTL from Oryza Glumaepatula Revealed Pi68(t) as Putative Candidate Gene for Rice Blast Resistance. Rice 13 (1), 17. doi:10.1186/s12284-020-00378-4
Dong, L., Liu, S., Kyaing, M. S., Xu, P., Tharreau, D., Deng, W., et al. (2020). Identification and Fine Mapping of Pi69(t), a New Gene Conferring Broad-Spectrum Resistance against Magnaporthe Oryzae from Oryza Glaberrima Steud. Front. Plant Sci. 11, 1190. doi:10.3389/fpls.2020.01190
Du, B., Zhang, W., Liu, B., Hu, J., Wei, Z., Shi, Z., et al. (2009). Identification and Characterization of Bph14 , a Gene Conferring Resistance to Brown Planthopper in rice. Proc. Natl. Acad. Sci. U.S.A. 106 (52), 22163–22168. doi:10.1073/pnas.0912139106
Eizenga, G. C., Prasad, B., Jackson, A. K., and Jia, M. H. (2013). Identification of rice Sheath Blight and Blast Quantitative Trait Loci in Two Different O. Sativa/O. Nivara Advanced Backcross Populations. Mol. Breed. 31 (4), 889–907. doi:10.1007/s11032-013-9843-y
Endo, M., Mikami, M., Endo, A., Kaya, H., Itoh, T., Nishimasu, H., et al. (2019). Genome Editing in Plants by Engineered CRISPR-Cas9 Recognizing NG PAM. Nat. Plants 5 (1), 14–17. doi:10.1038/s41477-018-0321-8
Feng, Z., Zhang, B., Ding, W., Liu, X., Yang, D.-L., Wei, P., et al. (2013). Efficient Genome Editing in Plants Using a CRISPR/Cas System. Cell Res 23 (10), 1229–1232. doi:10.1038/cr.2013.114
Fujino, K., Hirayama, Y., Obara, M., and Ikegaya, T. (2019). Introgression of the Chromosomal Region With the Pi-cd Locus From Oryza meridionalis Into O. sativa L. During Rice Domestication. Theor. Appl. Genet. 132 (7), 1981–1990. doi:10.1007/s00122-019-03332-1
Gao, Q., Li, G., Sun, H., Xu, M., Wang, H., Ji, J., et al. (2020). Targeted Mutagenesis of the Rice FW 2.2-Like Gene Family Using the CRISPR/Cas9 System Reveals OsFWL4 as a Regulator of Tiller Number and Plant Yield in Rice. Ijms 21 (3), 809. doi:10.3390/ijms21030809
Gu, K., Tian, D., Yang, F., Wu, L., Sreekala, C., Wang, D., et al. (2004). High-resolution Genetic Mapping of Xa27(t), a New Bacterial Blight Resistance Gene in rice, Oryza Sativa L. Theor. Appl. Genet. 108 (5), 800–807. doi:10.1007/s00122-003-1491-x
Hao, L., Ruiying, Q., Xiaoshuang, L., Shengxiang, L., Rongfang, X., Jianbo, Y., et al. (2019). CRISPR/Cas9-Mediated Adenine Base Editing in Rice Genome. Rice Sci. 26 (2), 125–128. doi:10.1016/j.rsci.2018.07.002
Hasan, S., Furtado, A., and Henry, R. (2022). Reticulate Evolution in AA-Genome Wild Rice in Australia. Front. Plant Sci. 13. doi:10.3389/fpls.2022.767635
Henry, R. J. (2019). Australian Wild Rice Populations: A Key Resource for Global Food Security. Front. Plant Sci. 10, 1354. doi:10.3389/fpls.2019.01354
Henry, R. J. (2018). “Oryza Australiensis Domin,” in The Wild Oryza Genomes (Springer), 61–66. doi:10.1007/978-3-319-71997-9_5
Henry, R. J., Rice, N., Waters, D. L. E., Kasem, S., Ishikawa, R., Hao, Y., et al. (2010). Australian Oryza: Utility and Conservation. Rice 3 (4), 235–241. doi:10.1007/s12284-009-9034-y
Hiei, Y., Ohta, S., Komari, T., and Kumashiro, T. (1994). Efficient Transformation of rice (Oryza Sativa L.) Mediated by Agrobacterium and Sequence Analysis of the Boundaries of the T-DNA. Plant J. 6 (2), 271–282. doi:10.1046/j.1365-313X.1994.6020271.x
Hou, L.-y., Yu, P., Xu, Q., Yuan, X.-p., Yu, H.-y., Wang, Y.-p., et al. (2011). Genetic Analysis and Preliminary Mapping of Two Recessive Resistance Genes to Brown Planthopper, Nilaparvata Lugens Stål in Rice. Rice Sci. 18 (3), 238–242. doi:10.1016/S1672-6308(11)60033-4
Hu, J., Xiao, C., Cheng, M.-x., Gao, G.-j., Zhang, Q.-l., and He, Y.-q. (2015). A New Finely Mapped Oryza Australiensis-Derived QTL in rice Confers Resistance to Brown Planthopper. Gene 561 (1), 132–137. doi:10.1016/j.gene.2015.02.026
Hu, X., Cui, Y., Dong, G., Feng, A., Wang, D., Zhao, C., et al. (2019). Using CRISPR-Cas9 to Generate Semi-dwarf rice Lines in Elite Landraces. Sci. Rep. 9 (1), 19096. doi:10.1038/s41598-019-55757-9
Hu, X., Meng, X., Liu, Q., Li, J., and Wang, K. (2018). Increasing the Efficiency of CRISPR-Cas9-VQR Precise Genome Editing in rice. Plant Biotechnol. J. 16 (1), 292–297. doi:10.1111/pbi.12771
Hu, X., Wang, C., Fu, Y., Liu, Q., Jiao, X., and Wang, K. (2016). Expanding the Range of CRISPR/Cas9 Genome Editing in Rice. Mol. Plant 9 (6), 943–945. doi:10.1016/j.molp.2016.03.003
Hua, K., Tao, X., Liang, W., Zhang, Z., Gou, R., and Zhu, J. K. (2020). Simplified Adenine Base Editors Improve Adenine Base Editing Efficiency in rice. Plant Biotechnol. J. 18 (3), 770–778. doi:10.1111/pbi.13244
Hua, K., Tao, X., Yuan, F., Wang, D., and Zhu, J.-K. (2018). Precise A·T to G·C Base Editing in the Rice Genome. Mol. Plant 11 (4), 627–630. doi:10.1016/j.molp.2018.02.007
Hua, K., Tao, X., and Zhu, J.-K. (2019). Expanding the Base Editing Scope in rice by Using Cas9 Variants. Plant Biotechnol. J. 17 (2), 499–504. doi:10.1111/pbi.12993
Huang, D., Qiu, Y., Zhang, Y., Huang, F., Meng, J., Wei, S., et al. (2013). Fine Mapping and Characterization of BPH27, a Brown Planthopper Resistance Gene from Wild rice (Oryza Rufipogon Griff.). Theor. Appl. Genet. 126 (1), 219–229. doi:10.1007/s00122-012-1975-7
Huang, L., Li, Q., Zhang, C., Chu, R., Gu, Z., Tan, H., et al. (2020). Creating Novel Wx Alleles with fine‐tuned Amylose Levels and Improved Grain Quality in rice by Promoter Editing Using CRISPR/Cas9 System. Plant Biotechnol. J. 18 (11), 2164–2166. doi:10.1111/pbi.13391
Huang, L., Zhang, R., Huang, G., Li, Y., Melaku, G., Zhang, S., et al. (2018). Developing superior Alleles of Yield Genes in rice by Artificial Mutagenesis Using the CRISPR/Cas9 System. Crop J. 6 (5), 475–481. doi:10.1016/j.cj.2018.05.005
Huang, X., Kurata, N., Wei, X., Wang, Z. X., Wang, A., Zhao, Q., et al. (2012). A Map of Rice Genome Variation Reveals the Origin of Cultivated Rice. Nature 490 (7421), 497–501. doi:10.1038/nature11532
Ji, H., Kim, S.-R., Kim, Y.-H., Suh, J.-P., Park, H.-M., Sreenivasulu, N., et al. (2016). Map-based Cloning and Characterization of the BPH18 Gene from Wild Rice Conferring Resistance to Brown Planthopper (BPH) Insect Pest. Sci. Rep. 6, 34376. doi:10.1038/srep34376
Jiang, W., Zhou, H., Bi, H., Fromm, M., Yang, B., and Weeks, D. P. (2013). Demonstration of CRISPR/Cas9/sgRNA-mediated Targeted Gene Modification in Arabidopsis, Tobacco, Sorghum and rice. Nucleic Acids Res. 41 (20), e188. doi:10.1093/nar/gkt780
Khanday, I., Skinner, D., Yang, B., Mercier, R., and Sundaresan, V. (2019). A Male-Expressed rice Embryogenic Trigger Redirected for Asexual Propagation through Seeds. Nature 565 (7737), 91–95. doi:10.1038/s41586-018-0785-8
Kleinstiver, B. P., Prew, M. S., Tsai, S. Q., Topkar, V. V., Nguyen, N. T., Zheng, Z., et al. (2015). Engineered CRISPR-Cas9 Nucleases with Altered PAM Specificities. Nature 523 (7561), 481–485. doi:10.1038/nature14592
Komor, A. C., Kim, Y. B., Packer, M. S., Zuris, J. A., and Liu, D. R. (2016). Programmable Editing of a Target Base in Genomic DNA without Double-Stranded DNA Cleavage. Nature 533 (7603), 420–424. doi:10.1038/nature17946
Lemmon, Z. H., Reem, N. T., Dalrymple, J., Soyk, S., Swartwood, K. E., Rodriguez-Leal, D., et al. (2018). Rapid Improvement of Domestication Traits in an Orphan Crop by Genome Editing. Nat. Plants 4 (10), 766–770. doi:10.1038/s41477-018-0259-x
Li, C., Zong, Y., Wang, Y., Jin, S., Zhang, D., Song, Q., et al. (2018a). Expanded Base Editing in rice and Wheat Using a Cas9-Adenosine Deaminase Fusion. Genome Biol. 19 (1), 59. doi:10.1186/s13059-018-1443-z
Li, H., Li, J., Chen, J., Yan, L., and Xia, L. (2020). Precise Modifications of Both Exogenous and Endogenous Genes in Rice by Prime Editing. Mol. Plant 13 (5), 671–674. doi:10.1016/j.molp.2020.03.011
Li, S., Li, J., He, Y., Xu, M., Zhang, J., Du, W., et al. (2019a). Precise Gene Replacement in rice by RNA Transcript-Templated Homologous Recombination. Nat. Biotechnol. 37 (4), 445–450. doi:10.1038/s41587-019-0065-7
Li, T., Liu, B., Spalding, M. H., Weeks, D. P., and Yang, B. (2012). High-efficiency TALEN-Based Gene Editing Produces Disease-Resistant rice. Nat. Biotechnol. 30 (5), 390–392. doi:10.1038/nbt.2199
Li, T., Yang, X., Yu, Y., Si, X., Zhai, X., Zhang, H., et al. (2018b). Domestication of Wild Tomato Is Accelerated by Genome Editing. Nat. Biotechnol. 36, 1160–1163. doi:10.1038/nbt.4273
Li, Z., Xue, Y., Zhou, H., Li, Y., Usman, B., Jiao, X., et al. (2019b). High-resolution Mapping and Breeding Application of a Novel Brown Planthopper Resistance Gene Derived from Wild rice (Oryza. Rufipogon Griff). Rice 12 (1), 41. doi:10.1186/s12284-019-0289-7
Lin, Q., Zong, Y., Xue, C., Wang, S., Jin, S., Zhu, Z., et al. (2020). Prime Genome Editing in rice and Wheat. Nat. Biotechnol. 38 (5), 582–585. doi:10.1038/s41587-020-0455-x
Lou, D., Wang, H., Liang, G., and Yu, D. (2017). OsSAPK2 Confers Abscisic Acid Sensitivity and Tolerance to Drought Stress in Rice. Front. Plant Sci. 8, 993. doi:10.3389/fpls.2017.00993
Lu, K., Wu, B., Wang, J., Zhu, W., Nie, H., Qian, J., et al. (2018). Blocking Amino Acid Transporter Os AAP 3 Improves Grain Yield by Promoting Outgrowth Buds and Increasing Tiller Number in rice. Plant Biotechnol. J. 16 (10), 1710–1722. doi:10.1111/pbi.12907
Ma, L., Zhu, F., Li, Z., Zhang, J., Li, X., Dong, J., et al. (2015a). TALEN-based Mutagenesis of Lipoxygenase LOX3 Enhances the Storage Tolerance of Rice (Oryza Sativa) Seeds. PLoS One 10 (12), e0143877. doi:10.1371/journal.pone.0143877
Ma, X., Zhang, Q., Zhu, Q., Liu, W., Chen, Y., Qiu, R., et al. (2015b). A Robust CRISPR/Cas9 System for Convenient, High-Efficiency Multiplex Genome Editing in Monocot and Dicot Plants. Mol. Plant 8 (8), 1274–1284. doi:10.1016/j.molp.2015.04.007
Macovei, A., Sevilla, N. R., Cantos, C., Jonson, G. B., Slamet‐Loedin, I., Čermák, T., et al. (2018). Novel Alleles of rice eIF4G Generated by CRISPR/Cas9‐targeted Mutagenesis Confer Resistance to Rice Tungro Spherical Virus. Plant Biotechnol. J. 16 (11), 1918–1927. doi:10.1111/pbi.12927
McCarty, N. S., Graham, A. E., Studená, L., and Ledesma-Amaro, R. (2020). Multiplexed CRISPR Technologies for Gene Editing and Transcriptional Regulation. Nat. Commun. 11 (1), 1281. doi:10.1038/s41467-020-15053-x
Miao, C., Xiao, L., Hua, K., Zou, C., Zhao, Y., Bressan, R. A., et al. (2018). Mutations in a Subfamily of Abscisic Acid Receptor Genes Promote rice Growth and Productivity. Proc. Natl. Acad. Sci. U.S.A. 115 (23), 6058–6063. doi:10.1073/pnas.1804774115
Mishra, R., Joshi, R. K., and Zhao, K. (2018). Genome Editing in Rice: Recent Advances, Challenges, and Future Implications. Front. Plant Sci. 9, 1361. doi:10.3389/fpls.2018.01361
Moner, A. M., Furtado, A., and Henry, R. J. (2020). Two Divergent Chloroplast Genome Sequence Clades Captured in the Domesticated rice Gene Pool May Have Significance for rice Production. BMC Plant Biol. 20 (1), 472. doi:10.1186/s12870-020-02689-6
Moner, A. M., and Henry, R. J. (2018). “Oryza Meridionalis N.Q.Ng,” in The Wild Oryza Genomes (Springer), 177–182. doi:10.1007/978-3-319-71997-9_16
Nasti, R. A., and Voytas, D. F. (2021). Attaining the Promise of Plant Gene Editing at Scale. Proc. Natl. Acad. Sci. U.S.A. 118 (22). doi:10.1073/pnas.2004846117
Neelam, K., Mahajan, R., Gupta, V., Bhatia, D., Gill, B. K., Komal, R., et al. (2020). High-resolution Genetic Mapping of a Novel Bacterial Blight Resistance Gene Xa-45(t) Identified from Oryza Glaberrima and Transferred to Oryza Sativa. Theor. Appl. Genet. 133 (3), 689–705. doi:10.1007/s00122-019-03501-2
Negishi, K., Kaya, H., Abe, K., Hara, N., Saika, H., and Toki, S. (2019). An Adenine Base Editor with Expanded Targeting Scope Using SpCas9‐ NG V1 in rice. Plant Biotechnol. J. 17 (8), 1476–1478. doi:10.1111/pbi.13120
Qin, R., Li, J., Li, H., Zhang, Y., Liu, X., Miao, Y., et al. (2019). Developing a Highly Efficient and Wildly adaptiveCRISPR‐SaCas9 Toolset for Plant Genome Editing. Plant Biotechnol. J. 17 (4), 706–708. doi:10.1111/pbi.13047
Ren, B., Liu, L., Li, S., Kuang, Y., Wang, J., Zhang, D., et al. (2019). Cas9-NG Greatly Expands the Targeting Scope of the Genome-Editing Toolkit by Recognizing NG and Other Atypical PAMs in Rice. Mol. Plant 12 (7), 1015–1026. doi:10.1016/j.molp.2019.03.010
Romero, F. M., and Gatica-Arias, A. (2019). CRISPR/Cas9: Development and Application in Rice Breeding. Rice Sci. 26 (5), 265–281. doi:10.1016/j.rsci.2019.08.001
Santosh Kumar, V. V., Verma, R. K., Yadav, S. K., Yadav, P., Watts, A., Rao, M. V., et al. (2020). CRISPR-Cas9 Mediated Genome Editing of Drought and Salt Tolerance (OsDST) Gene in Indica Mega rice Cultivar MTU1010. Physiol. Mol. Biol. Plants 26 (6), 1099–1110. doi:10.1007/s12298-020-00819-w
Scafaro, A. P., Haynes, P. A., and Atwell, B. J. (2009). Physiological and Molecular Changes in Oryza Meridionalis Ng., a Heat-Tolerant Species of Wild rice. J. Exp. Bot. 61 (1), 191–202. doi:10.1093/jxb/erp294
Shan, Q., Zhang, Y., Chen, K., Zhang, K., and Gao, C. (2015). Creation of Fragrant rice by Targeted Knockout of theOsBADH2gene Using TALEN Technology. Plant Biotechnol. J. 13 (6), 791–800. doi:10.1111/pbi.12312
Shen, C., Que, Z., Xia, Y., Tang, N., Li, D., He, R., et al. (2017). Knock Out of the Annexin Gene OsAnn3 via CRISPR/Cas9-mediated Genome Editing Decreased Cold Tolerance in rice. J. Plant Biol. 60 (6), 539–547. doi:10.1007/s12374-016-0400-1
Shen, L., Wang, C., Fu, Y., Wang, J., Liu, Q., Zhang, X., et al. (2018). QTL Editing Confers Opposing Yield Performance in Different rice Varieties. J. Integr. Plant Biol. 60 (2), 89–93. doi:10.1111/jipb.12501
Shimatani, Z., Kashojiya, S., Takayama, M., Terada, R., Arazoe, T., Ishii, H., et al. (2017). Targeted Base Editing in rice and Tomato Using a CRISPR-Cas9 Cytidine Deaminase Fusion. Nat. Biotechnol. 35 (5), 441–443. doi:10.1038/nbt.3833
Sotowa, M., Ootsuka, K., Kobayashi, Y., Hao, Y., Tanaka, K., Ichitani, K., et al. (2013). Molecular Relationships between Australian Annual Wild rice, Oryza Meridionalis, and Two Related Perennial Forms. Rice 6 (1), 26. doi:10.1186/1939-8433-6-26
Sun, Y., Jiao, G., Liu, Z., Zhang, X., Li, J., Guo, X., et al. (2017). Generation of High-Amylose Rice through CRISPR/Cas9-Mediated Targeted Mutagenesis of Starch Branching Enzymes. Front. Plant Sci. 8, 298. doi:10.3389/fpls.2017.00298
Sun, Y., Zhang, X., Wu, C., He, Y., Ma, Y., Hou, H., et al. (2016). Engineering Herbicide-Resistant Rice Plants through CRISPR/Cas9-Mediated Homologous Recombination of Acetolactate Synthase. Mol. Plant 9 (4), 628–631. doi:10.1016/j.molp.2016.01.001
Tan, G. X., Weng, Q. M., Ren, X., Huang, Z., Zhu, L. L., and He, G. C. (2004). Two Whitebacked Planthopper Resistance Genes in rice Share the Same Loci with Those for Brown Planthopper Resistance. Heredity 92 (3), 212–217. doi:10.1038/sj.hdy.6800398
Tang, L., Mao, B., Li, Y., Lv, Q., Zhang, L., Chen, C., et al. (2017). Knockout of OsNramp5 Using the CRISPR/Cas9 System Produces Low Cd-Accumulating Indica rice without Compromising Yield. Sci. Rep. 7 (1), 14438. doi:10.1038/s41598-017-14832-9
Tikapunya, T., Fox, G., Furtado, A., and Henry, R. (2016). Grain Physical Characteristic of the Australian Wild Rices. Plant Genet. Resour. 15 (5), 409–420. doi:10.1017/s1479262116000083
Tikapunya, T., Zou, W., Yu, W., Powell, P. O., Fox, G. P., Furtado, A., et al. (2017). Molecular Structures and Properties of Starches of Australian Wild rice. Carbohydr. Polym. 172, 213–222. doi:10.1016/j.carbpol.2017.05.046
Usman, B., Nawaz, G., Zhao, N., Liao, S., Qin, B., Liu, F., et al. (2020a). Programmed Editing of Rice (Oryza Sativa L.) OsSPL16 Gene Using CRISPR/Cas9 Improves Grain Yield by Modulating the Expression of Pyruvate Enzymes and Cell Cycle Proteins. Ijms 22 (1), 249. doi:10.3390/ijms22010249
Usman, B., Nawaz, G., Zhao, N., Liu, Y., and Li, R. (2020b). Generation of High Yielding and Fragrant Rice (Oryza Sativa L.) Lines by CRISPR/Cas9 Targeted Mutagenesis of Three Homoeologs of Cytochrome P450 Gene Family and OsBADH2 and Transcriptome and Proteome Profiling of Revealed Changes Triggered by Mutations. Plants 9 (6), 788. doi:10.3390/plants9060788
Van Tassel, D. L., Tesdell, O., Schlautman, B., Rubin, M. J., DeHaan, L. R., Crews, T. E., et al. (2020). New Food Crop Domestication in the Age of Gene Editing: Genetic, Agronomic and Cultural Change Remain Co-evolutionarily Entangled. Front. Plant Sci. 11, 789. doi:10.3389/fpls.2020.00789
Wambugu, P. W., Brozynska, M., Furtado, A., Waters, D. L., and Henry, R. J. (2015). Relationships of Wild and Domesticated Rices (Oryza AA Genome Species) Based upon Whole Chloroplast Genome Sequences. Sci. Rep. 5, 13957. doi:10.1038/srep13957
Wang, C., Liu, Q., Shen, Y., Hua, Y., Wang, J., Lin, J., et al. (2019a). Clonal Seeds from Hybrid rice by Simultaneous Genome Engineering of Meiosis and Fertilization Genes. Nat. Biotechnol. 37 (3), 283–286. doi:10.1038/s41587-018-0003-0
Wang, F., Wang, C., Liu, P., Lei, C., Hao, W., Gao, Y., et al. (2016). Enhanced Rice Blast Resistance by CRISPR/Cas9-Targeted Mutagenesis of the ERF Transcription Factor Gene OsERF922. PLoS One 11 (4), e0154027. doi:10.1371/journal.pone.0154027
Wang, J., Meng, X., Hu, X., Sun, T., Li, J., Wang, K., et al. (2019b). xC As9 Expands the Scope of Genome Editing with Reduced Efficiency in rice. Plant Biotechnol. J. 17 (4), 709–711. doi:10.1111/pbi.13053
Waters, D. L. E., Nock, C. J., Ishikawa, R., Rice, N., and Henry, R. J. (2012). Chloroplast Genome Sequence Confirms Distinctness of Australian and Asian Wild rice. Ecol. Evol. 2 (1), 211–217. doi:10.1002/ece3.66
Xu, P., Dong, L., Zhou, J., Li, J., Zhang, Y., Hu, F., et al. (2015). Identification and Mapping of a Novel Blast Resistance Gene Pi57(t) in Oryza Longistaminata. Euphytica 205 (1), 95–102. doi:10.1007/s10681-015-1402-7
Xu, R., Yang, Y., Qin, R., Li, H., Qiu, C., Li, L., et al. (2016). Rapid Improvement of Grain Weight via Highly Efficient CRISPR/Cas9-mediated Multiplex Genome Editing in rice. J. Genet. Genomics 43 (8), 529–532. doi:10.1016/j.jgg.2016.07.003
Xuwei, J., Chunlian, W., and Qing, Y. (2007). Breeding of Near‐isogenic Line CBB30 and Molecular Mapping of Xa30(t), a New Resistance Gene to Bacterial Blight in rice. Scientia Agricultura Sinica 40, 1094–1100.
Yang, L., Li, R. B., Li, Y. R., Huang, F. K., Chen, Y. Z., Huang, S. S., et al. (2011). Genetic Mapping of Bph20(t) and Bph21(t) Loci Conferring Brown Planthopper Resistance to Nilaparvata Lugens Stål in rice (Oryza Sativa L.). Euphytica 183 (2), 161–171. doi:10.1007/s10681-011-0437-7
Yang, M., Lin, J., Cheng, L., Zhou, H., Chen, S., Liu, F., et al. (2020). Identification of a Novel Planthopper Resistance Gene from Wild rice (Oryza Rufipogon Griff.). Crop J. 8 (6), 1057–1070. doi:10.1016/j.cj.2020.03.011
Yu, H., Lin, T., Meng, X., Du, H., Zhang, J., Liu, G., et al. (2021). A Route to De Novo Domestication of Wild Allotetraploid rice. Cell 184 (5), 1156–1170. e1114. doi:10.1016/j.cell.2021.01.013
Zafar, K., Sedeek, K. E. M., Rao, G. S., Khan, M. Z., Amin, I., Kamel, R., et al. (2020). Genome Editing Technologies for Rice Improvement: Progress, Prospects, and Safety Concerns. Front. Genome Ed. 2, 5. doi:10.3389/fgeed.2020.00005
Zeng, Y., Wen, J., Zhao, W., Wang, Q., and Huang, W. (2019). Rational Improvement of Rice Yield and Cold Tolerance by Editing the Three Genes OsPIN5b, GS3, and OsMYB30 with the CRISPR-Cas9 System. Front. Plant Sci. 10, 1663. doi:10.3389/fpls.2019.01663
Zhao, D.-S., Li, Q.-F., Zhang, C.-Q., Zhang, C., Yang, Q.-Q., Pan, L.-X., et al. (2018). GS9 Acts as a Transcriptional Activator to Regulate rice Grain Shape and Appearance Quality. Nat. Commun. 9 (1), 1240. doi:10.1038/s41467-018-03616-y
Zheng, C.-K., Wang, C.-L., Yu, Y.-J., Liang, Y.-T., and Zhao, K.-J. (2009). Identification and Molecular Mapping of Xa32(t), a Novel Resistance Gene for Bacterial Blight (Xanthomonas Oryzae Pv. Oryzae) in Rice. Acta Agronomica Sinica 35 (7), 1173–1180. doi:10.1016/S1875-2780(08)60089-9
Zhong, Z., Sretenovic, S., Ren, Q., Yang, L., Bao, Y., Qi, C., et al. (2019). Improving Plant Genome Editing with High-Fidelity xCas9 and Non-canonical PAM-Targeting Cas9-NG. Mol. Plant 12 (7), 1027–1036. doi:10.1016/j.molp.2019.03.011
Zhou, J., Peng, Z., Long, J., Sosso, D., Liu, B., Eom, J.-S., et al. (2015). Gene Targeting by the TAL Effector PthXo2 Reveals Cryptic Resistance Gene for Bacterial Blight of rice. Plant J. 82 (4), 632–643. doi:10.1111/tpj.12838
Zhou, X., Liao, H., Chern, M., Yin, J., Chen, Y., Wang, J., et al. (2018). Loss of Function of a rice TPR-Domain RNA-Binding Protein Confers Broad-Spectrum Disease Resistance. Proc. Natl. Acad. Sci. U.S.A. 115 (12), 3174–3179. doi:10.1073/pnas.1705927115
Zhou, Y., Xu, S., Jiang, N., Zhao, X., Bai, Z., Liu, J., et al. (2021). Engineering of rice Varieties with Enhanced Resistances to Both Blast and Bacterial Blight Diseases via CRISPR/Cas9. Plant Biotechnol. J. doi:10.1111/pbi.13766
Zhu, X.-G., and Zhu, J.-K. (2021). Precision Genome Editing Heralds Rapid De Novo Domestication for New Crops. Cell 184 (5), 1133–1134. doi:10.1016/j.cell.2021.02.004
Keywords: gene editing, australian wild rice, CRISPR-Cas9, genetic diversity, novel alleles
Citation: Abdullah M, Okemo P, Furtado A and Henry R (2022) Potential of Genome Editing to Capture Diversity From Australian Wild Rice Relatives. Front. Genome Ed. 4:875243. doi: 10.3389/fgeed.2022.875243
Received: 14 February 2022; Accepted: 25 March 2022;
Published: 27 April 2022.
Edited by:
Thorben Sprink, Julius Kühn-Institut, GermanyReviewed by:
Jaindra Nath Tripathi, International Institute of Tropical Agriculture (IITA), KenyaCopyright © 2022 Abdullah, Okemo, Furtado and Henry. This is an open-access article distributed under the terms of the Creative Commons Attribution License (CC BY). The use, distribution or reproduction in other forums is permitted, provided the original author(s) and the copyright owner(s) are credited and that the original publication in this journal is cited, in accordance with accepted academic practice. No use, distribution or reproduction is permitted which does not comply with these terms.
*Correspondence: Robert Henry, cm9iZXJ0LmhlbnJ5QHVxLmVkdS5hdQ==