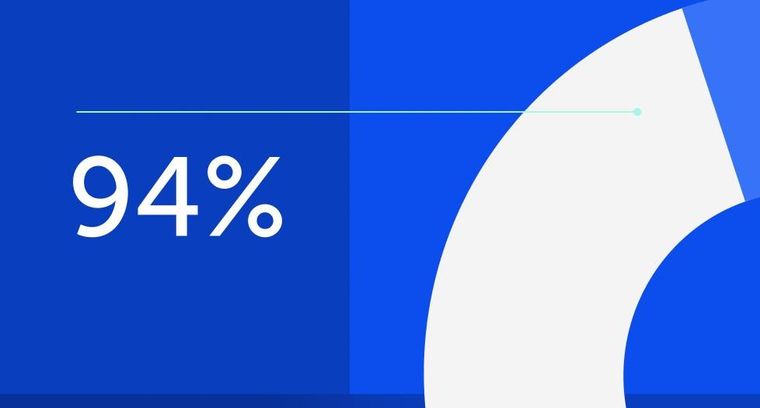
94% of researchers rate our articles as excellent or good
Learn more about the work of our research integrity team to safeguard the quality of each article we publish.
Find out more
MINI REVIEW article
Front. Genome Ed., 02 February 2022
Sec. Genome Editing in Plants
Volume 4 - 2022 | https://doi.org/10.3389/fgeed.2022.823486
This article is part of the Research TopicInsights in Genome Editing in Plants: 2021View all 10 articles
Hemp (Cannabis sativa L.) is a multipurpose crop with many important uses including medicine, fibre, food and biocomposites. This plant is currently gaining prominence and acceptance for its valuable applications. Hemp is grown as a cash crop for its novel cannabinoids which are estimated to be a multibillion-dollar downstream market. Hemp cultivation can play a major role in carbon sequestration with good CO2 to biomass conversion in low input systems and can also improve soil health and promote phytoremediation. The recent advent of genome editing tools to produce non-transgenic genome-edited crops with no trace of foreign genetic material has the potential to overcome regulatory hurdles faced by genetically modified crops. The use of Artificial Intelligence - mediated trait discovery platforms are revolutionizing the agricultural industry to produce desirable crops with unprecedented accuracy and speed. However, genome editing tools to improve the beneficial properties of hemp have not yet been deployed. Recent availability of high-quality Cannabis genome sequences from several strains (cannabidiol and tetrahydrocannabinol balanced and CBD/THC rich strains) have paved the way for improving the production of valuable bioactive molecules for the welfare of humankind and the environment. In this context, the article focuses on exploiting advanced genome editing tools to produce non-transgenic hemp to improve the most industrially desirable traits. The challenges, opportunities and interdisciplinary approaches that can be adopted from existing technologies in other plant species are highlighted.
Cultivation of hemp (Cannabis sativa) has increased globally in recent years and is a profitable enterprise that generates a range of useful products such as bioactive cannabinoids, seed, seed oil, fibre, textiles, construction materials and biocomposites. Archaeological evidence from Western China dating from 500 BCE suggests Cannabis was used for ceremonial purposes by ancient Chinese cultures during burial ceremonies (Ren et al., 2019). The medicinal properties of cannabinoids are extensively documented and renewed interest in these compounds in recent decades has driven growth in the health product and medical markets. The classification of Cannabis is typically determined by plant chemistry. In Europe, hemp was defined as Cannabis sativa plants containing less than 0.2% of the intoxicating cannabinoid Δ9-tetrahydrocannabinol (THC), but recent changes to laws and the adoption of the new Common Agricultural Policy have increased this to less than 0.3%. This figure is less than 0.3% in North America and Asia (Russo, 2017; Hammami et al., 2021). Drug-type Cannabis plants are grown for their high levels of the intoxicating THC and are commonly referred to as marijuana. Cannabis is a reservoir for a range of valuable secondary metabolites including cannabinoids and terpenes. Cannabinoids that have documented medical properties include cannabidiol (CBD), cannabigerol (CBG), cannabinol (CBN), cannabichromene (CBC), cannabidiolic acid (CBDA), cannabidivarin (CBDV), cannabicyclol (CBL), cannabivarin (CBV), cannabichromevarin (CBCV) and cannabielsoin (CBE). Cannabinoids accumulate in the secretory cavity of the hair-like glandular trichomes which are found in greatest abundance on the female inflorescences (Livingston et al., 2020). CBD is one of the most prominent non-intoxicating cannabinoids that has potential in treatment of various medical conditions including epilepsy, chronic pain, autism and post-traumatic stress disorder. Currently, medical Cannabis is legal in more than 50 countries including China, Australia, Germany, Israel, Canada and most of the U.S. The medical Cannabis market is rapidly growing from $3.5 billion at retail prices in 2019 to an estimated $20.2 billion during 2020–2025 (Aliekperova et al., 2020). Hemp is one of the earliest documented fibre crops used by humans with claims of domestication as early as 12,000 years ago (Ren et al., 2021). Hemp fibre is a strong, durable material with good insulative properties. It is used to make clothing, textiles, building materials and polymers. Hemp-based bioplastics have shown potential and could be superior in some respects to traditional polymers. They also offer a more sustainable, greener alternative to petroleum-based plastics (Fike, 2016). Materials such as Hempcrete® offer a means of carbon-negative building methods which can reduce net greenhouse gas emissions (Ip and Miller, 2012). Industrial hemp is an excellent carbon sink. Finnan and Styles (2013) found that hemp is comparable to the energy crops miscanthus and short-rotation coppice willow in net greenhouse gas emission abatement, and superior to sugar beet and oil seed rape. A comparative study carried out in Sweden demonstrated how hemp had similar biomass energy yield to maize and sugar beet (Prade et al., 2011). Hemp also has potential as a break crop between the planting of two food crop cycles and could play an important role in sustainable farming. This strategy can reduce soil pathogens, improve soil structure, and enrich soils if crop residues are ploughed in. Studies have shown how food crops such as wheat (Gorchs et al., 2017) and soybean (Liu et al., 2012) benefit from increased yield after hemp breaks crops over continuous systems. This accounted for yield increases of 37–48% in wheat monocultures and 9.1–10.8% in soybean monocultures. Hemp has documented nematicidal properties also and some of these yield gains can be attributed to suppression of these parasites (Adesina et al., 2020). Demand for hemp seed, oil and press-cake (remains of seed once pressed for oil) has contributed to the increased cultivation of hemp in the US (Adesina et al., 2020). As a food source hemp-derived protein has high nutritional value and excellent digestibility. The seed contains all the essential amino acids required by humans. There are also reported health benefits from consumption of hemp-derived protein including decreasing hypersensitivity and cholesterol (Shen et al., 2021). Overall, the cultivation of hemp has clear benefits and there is a growing market for hemp-derived products. Maximising the potential of this plant calls for more high-performing cultivars. Developing new cultivars through traditional breeding can often take a lot of time and labour. However, molecular breeding strategies such as marker assisted selection have refined the process of breeding, but these strategies are not well developed in this species. The recent development and adoption of genome editing technologies such as CRISPR (clustered regularly interspaced short palindromic repeats) offers a means to improve hemp varieties in a more precise and less time-consuming way. Many hemp varieties don’t self-pollinate, and this prevents using this strategy to obtain homozygous plants. Gene-editing technology allows breeders to modify genes on both alleles to achieve homozygous lines in one generation (Deguchi et al., 2020). Potential targets for gene-editing in hemp include genes controlling cannabinoid production and accumulation, fibre deposition, disease susceptibility and seed oil quality. This review discusses the opportunities for improving hemp with gene editing technology, and the potential challenges and opportunities in adopting these technologies.
The many different uses of Cannabis motivate the development of high performing cultivars with improved cannabinoid production, fibre accumulation, disease resistance and food quality. The growing demand for cannabinoids means there is an opportunity to develop high-yielding cultivars using novel methods. However, more research is needed to understand potential trade-offs when applying this technology. A knockout of the THC acid synthase gene via genome editing is a way to derive THC-free, high-CBD plants which would have huge value in countries with strict laws on THC levels. A patent filed by Canopy Growth Corporation details overexpression of genes regulating trichome development (e.g. GLABROUS INFLORESCENCE STEMS (GIS)) produced trichomes in greater density and abundance, and had a ten-fold increase in THC production over unmodified plants (Roscow, 2019). Using non-transgenic genome editing technology, the target gene(s) can be overexpressed by editing the respective regulatory (enhancer/promoter) elements upstream of a gene. Genome editing has been successfully applied to other important medicinal plant species such as Dendrobium officinale, Papaver somniferum, Dioscorea zingiberensis and Salvia miltiorrhiza (Alagoz et al., 2016; Kui et al., 2017; Feng et al., 2018; Zhou et al., 2018) and there is huge scope to modulate metabolite production via CRISPR/Cas9. Fibre quality of cultivated hemp plants can be improved by upregulating the expression of genes involved in the formation of bast fibres (phloem fibres). Several well-known transcription factors including NST1, MYB46 and WILM1 control secondary cell wall deposition and bast fibre development in hemp hypocotyls. The genes SND2, VND1 and NST1 are master regulators of secondary cell wall development (Behr et al., 2016). Hemp is susceptible to a range of diseases that can lead to loss in yield and decrease the overall value of the crop. Common pathogens of hemp include fungi, oomycetes, viruses, nematodes, and bacteria. Genome editing technologies offer a way to generate disease resistant varieties with greater precision and in a faster time frame than traditional breeding methods. Targeting resistance (R) and susceptibility (S) genes are one way to increase a plant’s resistance. A recent study has identified a powdery mildew resistance (R) gene in a Cannabis sativa cultivar, designated PM1, that confers resistance to the pathogen Golovinomyces ambrosiae (Mihalyov and Garfinkel, 2021). Improving food quality of seed and seed oil is also possible. Targeting FAD2 genes which are involved in converting oleic acid to linoleic acid and linolenic acid offer a reliable target to upregulate oleic acid production. Mutagenesis studies on the hemp cultivar Finola have shown increased oil quality (high oleic content) and shelf life through targeted mutations of fatty desaturase genes CsFAD2 and CsFAD3 (Bielecka et al., 2014). Genome editing of Brassica napus fatty acid desaturase gene 2 (FAD2) using CRISPR/Cas9 has been successfully demonstrated, producing high oleic acid content seed. Back-crossing of the progeny of one transformed line showed the mutation was inheritable and no transgenic DNA was inherited (Okuzaki et al., 2018).
Genome Editing by CRISPR/Cas is revolutionizing plant biology and agriculture in developing improved crops with novel traits. CRISPR/Cas technology allows for sequence specific editing of the target genome, thereby allowing for precise control over gene modifications and associated traits, in a low cost and straightforward manner. This level of control over DNA sequence change is unprecedented. It is a vast improvement over previous genome modification tools and opens new doors for exciting developments in the fields of medicine and agriculture. Agrobacterium-mediated CRISPR transformation is being widely used for targeted crop improvement to develop gene knockouts, knock-ins, transcriptional regulation, and epigenetic changes in the genome to achieve novel traits. However, agrobacterium-mediated transformation may pose a bottleneck for regulatory approval because of the introduction of external plasmid DNA into the plant genome. The newest next-generation genome editing technology encompasses modifying/editing the DNA bases by direct delivery of CRISPR/Cas ribonucleoprotein (RNP) complexes into plant tissue, such as protoplasts, embryos or in-vitro grown calli (Woo et al., 2015; Malnoy et al., 2016; Osakabe et al., 2018). The transformed plant tissue is grown in a suitable media to regenerate entire plants followed by screening for the genome edited plant lines (Figure 1). This approach eliminates the opportunity for plasmid encoded DNA elements to integrate into the plant genome, thereby mimicking natural mutations. In addition to introducing mutations and deleting entire fragments of DNA elements, CRISPR/Cas technology is also being used to introduce a specific DNA fragment to a precise location in the genome. A specific donor DNA is included together with Cas9 and sgRNA which spans the flanking regions of the target site with the donor DNA element in between. The presence of this single-stranded DNA triggers the Homology Directed Repair (HDR) mechanism wherein the donor DNA is used as a template by the DNA repair machinery to repair the cut target site, and consequently the target DNA sequence gets introduced to the target genome (Chen et al., 2019). This new generation of precision methods has several applications in hemp breeding such as gene knockout/knock-in, base editing, gene- and genome-wide screening, modifying gene regulation, and developing virus resistant plants, as demonstrated in different recalcitrant species such as wheat, maize and grape. These strategies have been clearly detailed in the review article by Chen et al. (2019) by providing specific examples.
FIGURE 1. A schematic overview of non-transgenic genome editing technology applicable for Cannabis improvement. Cannabis protoplasts isolated from in-vitro grown plants are transfected with a mixture of Cas9 and sgRNA followed by agarose or alginate embedding and plant regeneration.
The availability of cannabis genome sequences (Braich et al., 2020; Gao et al., 2020) and growing number of RNA-sequencing datasets (Massimino., 2017; Braich et al., 2019; Braich et al., 2019, 2019; Zager et al., 2019; Livingston et al., 2020; Livingston et al., 2020, 2020) allows for the use of big data analysis methods for greater understanding of Cannabis biology beyond the expression levels of genes. A systems-biology approach uses information-rich complex datasets to provide meaningful results by extrapolating the relationship between individual biomolecules. Biomolecules (e.g. genes, transcription factors, metabolites, promoters) are represented as nodes and the connections between them as edges in this in-silico molecular network. Two molecules (nodes) connected by an edge would mean a possible interaction in terms of physical interaction, biosynthesis, regulation and/or co-expression between them. Once the networks are developed, the dynamics of the interactions can be studied with a focus on the hubs that can be central to a biological function of interest (Breitling, 2010). This integrated approach is quite useful to make sense of the vast amounts of datasets produced by holistic studies and will provide a combined biological insight (emergent behaviour) that isolated experiments simply cannot. New genes participating in defense response pathways are predicted and validated using a systems biology approach in Arabidopsis thaliana (Windram et al., 2012). This emphasizes the potential of interdisciplinarity in biological research. Furthermore, combining the metabolomics datasets to develop network models using machine learning has been successful in predicting metabolic pathways in tomato (Toubiana et al., 2019). Applying these established methods in hemp will speed-up the understanding of molecular processes and metabolite accumulations in the context of improving desirable traits in hemp such as higher CBD production.
Another application of computational methods is the use of Artificial Intelligence (AI) to identify single nucleotide polymorphisms (SNPs) associated with important agricultural traits in Genome Wide Association Studies (GWAS). By using the available genomic sequences from different varieties of a certain crop species, these deep learning-based prediction methods can identify SNPs associated with the trait of interest. The machine learning algorithms are first trained with a combination of data including genotypic, phenotypic, agronomic practices and environmental data before it is used on a test dataset for predicting SNPs (Wang et al., 2020; Mieth et al., 2021). This is just one of the applications of AI and deep learning to accelerate knowledge discovery. The review article by Wang et al. (2020) provides a good overview of its various applications in plant research and agriculture. GWAS studies have been carried out on hemp with respect to fibre quality (Petit et al., 2020a) and flowering time and sex determination (Petit et al., 2020b). Hesami et al. (2021a) applied machine learning algorithms in silico to predict off-target gRNA activity in modifying centromeric histone H3 (CENH3) genes in Cannabis. Of the three machine learning algorithms used, the Random Forest (RF) had the highest precision. These predictive models offer a powerful tool in designing effective genome-editing protocols in Cannabis. Interdisciplinary approaches will accelerate the knowledge-discovery and will be valuable to understand cannabinoid biology and genetics, given imperfect genome sequence and annotations, recalcitrance for transformation and the lack of standard protocols/procedures for Cannabis.
Micropropagation of Cannabis tissues in a disease-free aseptic environment is an important step towards a successful transformation protocol. Some varieties of Cannabis are recalcitrant to in vitro culture and transformation. An optimal strategy may be to transform more amenable varieties and backcross these into elite lines, which is still time and labor intensive. Adhikary et al. (2021) mentions that the Cannabis industries have been developing tissue culture and micropropagation techniques over the last 2 decades and are held as a trade secret to preserve competitive advantage with other commercial entities. Optimizing micropropagation protocols for non-meristematic tissues is important for genome editing applications. Factors including plant growth regulators (PGRs), type of light, carbohydrate sources, additives, temperature and genotype influence micropropagation success (Hesami et al., 2021b). Zhang et al. (2021) found that Cannabis embryo hypocotyls of immature grains collected 15 days after anthesis exhibited the greatest regeneration rate and were also more amenable to agrobacterium transformation. The authors used G41sg vector to deliver sgRNA targeting phytoene desaturase gene (CsPDS1) generating albino plants. This is the first published report of successful gene editing in Cannabis sativa, which paves the way for further developments in non-transgenic genome editing technology. Regenerating transfected protoplast cells into complete plants is also challenging. The first report of DNA-free (or non-transgenic) genome editing described the successful regeneration of genome edited lettuce protoplasts into complete plants (Woo et al., 2015). The authors incubated preassembled complexes of purified Cas9 protein and guide RNA with plant protoplasts in the presence of polyethylene glycol (PEG), a standard and widely used transfection method. Interestingly, RNA-guided mutations were detected as early as 24 h, suggesting the quick Cas9 activity even before the cell cycle was completed. The transfected protoplasts were mixed with a 1:1 solution of 50% B5 medium and 2.4% agarose to make agarose embeddings plated on 6-well plates (Woo et al., 2015). For regenerating plants from genome edited grapevine protoplasts the authors embedded the protoplasts in alginate disks and stimulated the formation of mini-calli in NN-based cultivation medium (Nitsch and Nitsch, 1969) optimized for regeneration (Scintilla et al., 2021). Beard et al. (2021) demonstrated PEG mediated transient transformation of Cannabis sativa protoplasts with a p35S:GFP expression cassette and achieved a transformation efficiency of up to 31%, thus demonstrating the viability of protoplast transformation in this species. Table 1 lists the published transformation and regeneration technologies for non-transgenic genome editing in different plants. Effective protoplast culture protocols provide a platform for whole plant regeneration, and a platform to test sgRNAs in optimizing CRISPR protocols.
TABLE 1. Protoplast transformation and regeneration technologies in different species applicable for Cannabis improvement.
In another approach, researchers used immature embryos from wheat and maize to bombard the mixture of either CRISPR/Cas RNPs or DNA/RNA elements encoding Cas proteins with sgRNAs coated on microparticles (Svitashev et al., 2016; Zhang et al., 2016; Liang et al., 2017, 2018). The embryos were transferred to callusing media, and then to shoot and root regeneration media for complete plant development. This procedure can be applied to develop non-transgenic Cannabis plants by using an embryo extraction protocol (Soler et al., 2016). The following biolistic transformation and whole plant regeneration method needs optimization in Cannabis.
Co-transformation of developmental regulator genes in combination with the target genes of interest have proven to increase or induce callus formation in recalcitrant varieties of sorghum, maize and wheat (Che et al., 2021; Hoerster et al., 2020; Nalapalli et al., 2021). In Cannabis, co-transformation of native homologs of developmental regulators in combinations increased shoot regeneration efficiency up to 1.7-fold with CsGRF3–CsGIF1 chimera and all chimeras containing CsWUS4 (Zhang et al., 2021). WUSCHEL (WUS) is essential for de novo establishment of the shoot stem cell niche (Zhang et al., 2017) and co-transfecting WUS into protoplasts could induce the formation of calli and subsequently shoots.
The current legal status of gene-edited crops in the European Union as genetically modified organisms (GMOs) prevents the full deployment of these technologies in C. sativa. A move toward social acceptance of gene-edited crops requires a raising of public awareness and a clear distinction between transgenic and non-transgenic plants. Highlighting the use of random mutagenesis by chemical and radiological means for the last century in developing new crop varieties offers utility in advocating the use of targeted mutagenesis technologies. Strictly speaking, DNA-free, gene-edited crops are equivalent to crop varieties derived through random mutagenesis, which include many important food crop species (e.g. bananas, barley). The caveat being that targeted mutagenesis is not random and offers greater control and specificity and reduces the incidence of deleterious mutations and the impact of mutation load (Jung and Till, 2021). The ruling of the Court of Justice of the European Union in 2018 on genome editing groups this new technology with GMOs as outlined in directive 2001/18. The distinction of gene-edited crops as genetically modified organisms (GMOs) within the EU also excludes them from organic certification. This may serve as a hurdle in the public acceptance of these crops as healthy, safe and nutritious. This contentious decision has been challenged by the European Federation of Biotechnology (EFB). They argue that the Site Directed nuclease 1 (SDN1) format of gene editing is fundamentally different from the genetic engineering outlined in directive 2001/18. Safety concerns of introducing foreign DNA and causing off-target mutations are avoided in SDN1 as no foreign DNA is introduced and whole genome sequencing of the transformed organism can investigate any potentially dangerous mutations (Hjort et al., 2021). The recent refinement of CRISPR methods circumvents the issue of introducing transgenes into gene-edited crops, where Agrobacterium plasmid DNA is not used, and nucleases are delivered directly into the cells (Ishii, 2018). Even though hemp has large genetic diversity and traditional breeding still offers utility to improve varieties, application of these new plant breeding technologies allows highly specific changes in markedly shorter timeframes. In producing new allelic variation in crop species, CRISPR is the most powerful tool available to breeders, and should be exploited for its full potential.
DS, BP, CK, OK, RH and RB conceptualized the article. DS, RH and RB wrote the manuscript. BP, CK and OK revised the manuscript, provided critical insights and improved the manuscript. All authors approved the manuscript for publication.
This research was carried out with funding from the European Union's Horizon 2020 Research and innovation Programme under the Marie Skłodowska-Curie Co-funding of regional, national and international programmes Grant agreement No: 847402 and an Irish Research Council 2021 Government of Ireland Postgraduate Scholarship.
Authors CK and OK are employed by Plantedit Pvt Ltd. whereas the author RB is employed through a Marie Skłodowska-Curie and Career-Fit Plus Fellowship.
The remaining authors declare that the research was conducted in the absence of any commercial or financial relationships that could be construed as a potential conflict of interest.
All claims expressed in this article are solely those of the authors and do not necessarily represent those of their affiliated organizations, or those of the publisher, the editors and the reviewers. Any product that may be evaluated in this article, or claim that may be made by its manufacturer, is not guaranteed or endorsed by the publisher.
Adesina, I., Bhowmik, A., Sharma, H., and Shahbazi, A. (2020). A Review on the Current State of Knowledge of Growing Conditions, Agronomic Soil Health Practices and Utilities of Hemp in the United States. Agriculture 10 (4), 129. doi:10.3390/agriculture10040129
Adhikary, D., Kulkarni, M., El-Mezawy, A., Mobini, S., Elhiti, M., Gjuric, R., et al. (2021). Medical Cannabis and Industrial Hemp Tissue Culture: Present Status and Future Potential. Front. Plant Sci. 12, 275. doi:10.3389/fpls.2021.627240
Alagoz, Y., Gurkok, T., Zhang, B., and Unver, T. (2016). Manipulating the Biosynthesis of Bioactive Compound Alkaloids for Next-Generation Metabolic Engineering in Opium Poppy Using CRISPR-Cas 9 Genome Editing Technology. Sci. Rep. 6 (1), 1–9. doi:10.1038/srep30910
Aliekperova, N., Kosyachenko, К., and Kaniura, O. (2020). Perspectives on Formation of Medical Cannabis Market in Ukraine Based on Holistic Approach. J. Cannabis Res. 2 (1), 1–19. doi:10.1186/s42238-020-00044-y
Barceló, M., Wallin, A., Medina, J. J., Gil-Ariza, D. J., López-Casado, G., Juarez, J., et al. (2019). Isolation and Culture of Strawberry Protoplasts and Field Evaluation of Regenerated Plants. Scientia Horticulturae 256, 108552. doi:10.1016/j.scienta.2019.108552
Beard, K. M., Boling, A. W. H., and Bargmann, B. O. R. (2021). Protoplast Isolation, Transient Transformation, and Flow-Cytometric Analysis of Reporter-Gene Activation in Cannabis Sativa L. Ind. Crops Prod. 164, 113360. doi:10.1016/j.indcrop.2021.113360
Behr, M., Legay, S., Žižková, E., Motyka, V., Dobrev, P. I., Hausman, J.-F., et al. (2016). Studying Secondary Growth and Bast Fiber Development: the Hemp Hypocotyl Peeks behind the wall. Front. Plant Sci. 7, 1733. doi:10.3389/fpls.2016.01733
Bielecka, M., Kaminski, F., Adams, I., Poulson, H., Sloan, R., Li, Y., et al. (2014). Targeted Mutation of Δ12 and Δ15 Desaturase Genes in Hemp Produce Major Alterations in Seed Fatty Acid Composition Including a High Oleic Hemp Oil. Plant Biotechnol. J. 12 (5), 613–623. doi:10.1111/pbi.12167
Braich, S., Baillie, R. C., Jewell, L. S., Spangenberg, G. C., and Cogan, N. O. I. (2019). Generation of a Comprehensive Transcriptome Atlas and Transcriptome Dynamics in Medicinal Cannabis. Sci. Rep. 9 (1), 1–12. doi:10.1038/s41598-019-53023-6
Braich, S., Baillie, R. C., Spangenberg, G. C., and Cogan, N. O. I. (2020). A New and Improved Genome Sequence of Cannabis Sativa. bioRxiv. doi:10.1101/2020.12.13.422592
Che, P., Wu, E., Simon, M. K., Anand, A., Lowe, K., Gao, H., et al. (2021). Wuschel2 Enables Highly Efficient CRISPR/Cas-targeted Genome Editing during Rapid De Novo Shoot Regeneration in Sorghum. bioRxiv. doi:10.1101/2021.06.21.449302
Chen, K., Wang, Y., Zhang, R., Zhang, H., and Gao, C. (2019). CRISPR/Cas Genome Editing and Precision Plant Breeding in Agriculture. Annu. Rev. Plant Biol. 70, 667–697. doi:10.1146/annurev-arplant-050718-100049
Deguchi, M., Kane, S., Potlakayala, S., George, H., Proano, R., Sheri, V., et al. (2020). Metabolic Engineering Strategies of Industrial Hemp (Cannabis Sativa L.): A Brief Review of the Advances and Challenges. Front. Plant Sci. 11, 580621. doi:10.3389/fpls.2020.580621
Feng, S., Song, W., Fu, R., Zhang, H., Xu, A., and Li, J. (2018). Application of the CRISPR/Cas9 System in Dioscorea Zingiberensis. Plant Cel Tiss Organ. Cult 135 (1), 133–141. doi:10.1007/s11240-018-1450-5
Fike, J. (2016). Industrial Hemp: Renewed Opportunities for an Ancient Crop. Crit. Rev. Plant Sci. 35 (5-6), 406–424. doi:10.1080/07352689.2016.1257842
Finnan, J., and Styles, D. (2013). Hemp: a More Sustainable Annual Energy Crop for Climate and Energy Policy. Energy Policy 58, 152–162. doi:10.1016/j.enpol.2013.02.046
Gorchs, G., Lloveras, J., Serrano, L., and Cela, S. (2017). Hemp Yields and its Rotation Effects on Wheat under Rainfed Mediterranean Conditions. Agron.j. 109 (4), 1551–1560. doi:10.2134/agronj2016.11.0676
Gao, S., Wang, B., Xie, S., Xu, X., Zhang, J., Pei, L., et al. (2020). A High-Quality Reference Genome of Wild Cannabis sativa. Hortic. Res. 7 (1), 1–11. doi:10.1038/s41438-020-0295-3
Hammami, N., Privé, J.-P., Joly, D. L., and Moreau, G. (2021). Associations between Cannabinoids and Growth Stages of Twelve Industrial Hemp Cultivars Grown Outdoors in Atlantic Canada. Ind. Crops Prod. 172, 113997. doi:10.1016/j.indcrop.2021.113997
Hesami, M., Baiton, A., Alizadeh, M., Pepe, M., Torkamaneh, D., and Jones, A. M. P. (2021b). Advances and Perspectives in Tissue Culture and Genetic Engineering of Cannabis. Ijms 22 (11), 5671. doi:10.3390/ijms22115671
Hesami, M., Yoosefzadeh Najafabadi, M., Adamek, K., Torkamaneh, D., and Jones, A. M. P. (2021a). Synergizing Off-Target Predictions for In Silico Insights of CENH3 Knockout in Cannabis through CRISPR/CAS. Molecules 26 (7), 2053. doi:10.3390/molecules26072053
Hjort, C., Cole, J., and Frébort, I. (2021). European Genome Editing Regulations: Threats to the European Bioeconomy and Unfit for Purpose. EFB Bioeconomy J. 1, 100001. doi:10.1016/j.bioeco.2021.100001
Hoerster, G., Wang, N., Ryan, L., Wu, E., Anand, A., McBride, K., et al. (2020). Use of Non-integrating Zm-Wus2 Vectors to Enhance maize Transformation. In Vitro Cell.Dev.Biol.-Plant 56 (3), 265–279. doi:10.1007/s11627-019-10042-2
Ip, K., and Miller, A. (2012). Life Cycle Greenhouse Gas Emissions of Hemp-Lime wall Constructions in the UK. Resour. Conservation Recycling 69, 1–9. doi:10.1016/j.resconrec.2012.09.001
Ishii, T. (2018). Crop Gene-Editing: Should We Bypass or Apply Existing GMO Policy? Trends Plant Science 23 (11), 947–950. doi:10.1016/j.tplants.2018.09.001
Jiang, W. Z., Henry, I. M., Lynagh, P. G., Comai, L., Cahoon, E. B., and Weeks, D. P. (2017). Significant Enhancement of Fatty Acid Composition in Seeds of the Allohexaploid, Camelina Sativa , Using CRISPR/Cas9 Gene Editing. Plant Biotechnol. J. 15 (5), 648–657. doi:10.1111/pbi.12663
Jung, C., and Till, B. (2021). Mutagenesis and Genome Editing in Crop Improvement: Perspectives for the Global Regulatory Landscape. Trends Plant Sci. 26, 1258–1269. doi:10.1016/j.tplants.2021.08.002
Kui, L., Chen, H., Zhang, W., He, S., Xiong, Z., Zhang, Y., et al. (2017). Building a Genetic Manipulation Tool Box for Orchid Biology: Identification of Constitutive Promoters and Application of CRISPR/Cas9 in the Orchid, Dendrobium Officinale. Front. Plant Sci. 7, 2036. doi:10.3389/fpls.2016.02036
Li, R., Li, R., Li, X., Fu, D., Zhu, B., Tian, H., et al. (2018). Multiplexed CRISPR/Cas9-mediated Metabolic Engineering of γ-aminobutyric Acid Levels in Solanum lycopersicum. Plant Biotechnol. J. 16 (2), 415–427. doi:10.1111/pbi.12781
Liang, Z., Chen, K., Li, T., Zhang, Y., Wang, Y., Zhao, Q., et al. (2017). Efficient DNA-free Genome Editing of Bread Wheat Using CRISPR/Cas9 Ribonucleoprotein Complexes. Nat. Commun. 8 (1), 1–5. doi:10.1038/ncomms14261
Liang, Z., Chen, K., Zhang, Y., Liu, J., Yin, K., Qiu, J.-L., et al. (2018). Genome Editing of Bread Wheat Using Biolistic Delivery of CRISPR/Cas9 In Vitro Transcripts or Ribonucleoproteins. Nat. Protoc. 13 (3), 413–430. doi:10.1038/nprot.2017.145
Liu, X., Li, Y., Han, B., Zhang, Q., Zhou, K., Zhang, X., et al. (2012). Yield Response of Continuous Soybean to One-Season Crop Disturbance in a Previous Continuous Soybean Field in Northeast China. Field Crops Res. 138, 52–56. doi:10.1016/j.fcr.2012.09.012
Livingston, S. J., Quilichini, T. D., Booth, J. K., Wong, D. C. J., Rensing, K. H., Laflamme‐Yonkman, J., et al. (2020). Cannabis Glandular Trichomes Alter Morphology and Metabolite Content during Flower Maturation. Plant J. 101 (1), 37–56. doi:10.1111/tpj.14516
Malnoy, M., Viola, R., Jung, M.-H., Koo, O.-J., Kim, S., Kim, J.-S., et al. (2016). DNA-free Genetically Edited grapevine and Apple Protoplast Using CRISPR/Cas9 Ribonucleoproteins. Front. Plant Sci. 7, 1904. doi:10.3389/fpls.2016.01904
Massimino, L. (2017). In Silico gene Expression Profiling in Cannabis Sativa. F1000Res 6, 69. doi:10.12688/f1000research.10631.1
Mieth, B., Rozier, A., Rodriguez, J. A., Höhne, M. M. C., Görnitz, N., and Müller, K.-R. (2021). DeepCOMBI: Explainable Artificial Intelligence for the Analysis and Discovery in Genome-wide Association Studies. NAR genomics and bioinformatics 3 (3), lqab065. doi:10.1093/nargab/lqab065
Mihalyov, P. D., and Garfinkel, A. R. (2021). Discovery and Genetic Mapping of PM1, a Powdery Mildew Resistance Gene in Cannabis Sativa L. Front. Agron. 3, 66. doi:10.3389/fagro.2021.720215
Moon, K.-B., Park, J.-S., Park, S.-J., Lee, H.-J., Cho, H.-S., Min, S.-R., et al. (2021). A More Accessible, Time-Saving, and Efficient Method for In Vitro Plant Regeneration from Potato Protoplasts. Plants 10 (4), 781. doi:10.3390/plants10040781
Nalapalli, S., Tunc-Ozdemir, M., Sun, Y., Elumalai, S., and Que, Q. (2021). “Morphogenic Regulators and Their Application in Improving Plant Transformation,” in Rice Genome Engineering and Gene Editing. Editors A. Bandyopadhyay,, and R. Thilmony (New York, NY: Humana), 37–61. doi:10.1007/978-1-0716-1068-8_3
Nitsch, J. P., and Nitsch, C. (1969). Haploid Plants from Pollen Grains. Science 163 (3862), 85–87. doi:10.1126/science.163.3862.85
Okuzaki, A., Ogawa, T., Koizuka, C., Kaneko, K., Inaba, M., Imamura, J., et al. (2018). CRISPR/Cas9-mediated Genome Editing of the Fatty Acid Desaturase 2 Gene in Brassica Napus. Plant Physiol. Biochem. 131, 63–69. doi:10.1016/j.plaphy.2018.04.025
Osakabe, Y., Liang, Z., Ren, C., Nishitani, C., Osakabe, K., Wada, M., et al. (2018). CRISPR-Cas9-mediated Genome Editing in Apple and grapevine. Nat. Protoc. 13 (12), 2844–2863. doi:10.1038/s41596-018-0067-9
Pasternak, T., Paponov, I. A., and Kondratenko, S. (2021). Optimizing Protocols for Arabidopsis Shoot and Root Protoplast Cultivation. Plants 10 (2), 375. doi:10.3390/plants10020375
Petit, J., Salentijn, E. M. J., Paulo, M.-J., Denneboom, C., and Trindade, L. M. (2020b). Genetic Architecture of Flowering Time and Sex Determination in Hemp (Cannabis Sativa L.): A Genome-wide Association Study. Front. Plant Sci. 11, 1704. doi:10.3389/fpls.2020.569958
Petit, J., Salentijn, E. M. J., Paulo, M.-J., Denneboom, C., van Loo, E. N., and Trindade, L. M. (2020a). Elucidating the Genetic Architecture of Fiber Quality in Hemp (Cannabis Sativa L.) Using a Genome-wide Association Study. Front. Genet. 11, 1101. doi:10.3389/fgene.2020.566314
Prade, T., Svensson, S.-E., Andersson, A., and Mattsson, J. E. (2011). Biomass and Energy Yield of Industrial Hemp Grown for Biogas and Solid Fuel. Biomass and bioenergy 35 (7), 3040–3049. doi:10.1016/j.biombioe.2011.04.006
Ren, G., Zhang, X., Li, Y., Ridout, K., Serrano-Serrano, M. L., Yang, Y., et al. (2021). Large-scale Whole-Genome Resequencing Unravels the Domestication History of Cannabis Sativa. Sci. Adv. 7 (29), eabg2286. doi:10.1126/sciadv.abg2286
Ren, M., Tang, Z., Wu, X., Spengler, R., Jiang, H., Yang, Y., et al. (2019). The Origins of Cannabis Smoking: Chemical Residue Evidence from the First Millennium BCE in the Pamirs. Sci. Adv. 5 (6), eaaw1391. doi:10.1126/sciadv.aaw1391
Roscow, R. F. (2019). “Canopy Growth Corp,” Enhanced Cannabis Plants and Methods of Making and Using the Same. U.S. Patent Application 16/093,066.
Russo, E. B. (2017). Cannabidiol Claims and Misconceptions. Trends Pharmacological Sciences 38 (3), 198–201. doi:10.1016/j.tips.2016.12.004
Russo, E. B. (2019). The Case for the Entourage Effect and Conventional Breeding of Clinical Cannabis: No "Strain," No Gain. Front. Plant Sci. 9, 1969. doi:10.3389/fpls.2018.01969
Scintilla, S., Salvagnin, U., Giacomelli, L., Zeilmaker, T., Malnoy, M. A., van der Voort, J. R., et al. (2021). Regeneration of Plants from DNA-free Edited Grapevine Protoplasts. bioRxiv. doi:10.1101/2021.07.16.452503
Shen, P., Gao, Z., Fang, B., Rao, J., and Chen, B. (2021). Ferreting Out the Secrets of Industrial Hemp Protein as Emerging Functional Food Ingredients. Trends Food Sci. Technol. 112, 1–15. doi:10.1016/j.tifs.2021.03.022
Soler, S., Borràs, D., Vilanova, S., Sifres, A., Andújar, I., Figàs, M. R., et al. (2016). Use of Embryos Extracted from Individual Cannabis Sativa Seeds for Genetic Studies and Forensic Applications. J. Forensic Sci. 61 (2), 494–500. doi:10.1111/1556-4029.12995
Subburaj, S., Chung, S. J., Lee, C., Ryu, S.-M., Kim, D. H., Kim, J.-S., et al. (2016). Site-directed Mutagenesis in Petunia × Hybrida Protoplast System Using Direct Delivery of Purified Recombinant Cas9 Ribonucleoproteins. Plant Cel Rep 35 (7), 1535–1544. doi:10.1007/s00299-016-1937-7
Svitashev, S., Schwartz, C., Lenderts, B., Young, J. K., and Mark Cigan, A. (2016). Genome Editing in maize Directed by CRISPR-Cas9 Ribonucleoprotein Complexes. Nat. Commun. 7 (1), 1–7. doi:10.1038/ncomms13274
Toubiana, D., Puzis, R., Wen, L., Sikron, N., Kurmanbayeva, A., Soltabayeva, A., et al. (2019). Combined Network Analysis and Machine Learning Allows the Prediction of Metabolic Pathways from Tomato Metabolomics Data. Commun. Biol. 2 (1), 1–13. doi:10.1038/s42003-019-0440-4
Wang, H., Cimen, E., Singh, N., and Buckler, E. (2020). Deep Learning for Plant Genomics and Crop Improvement. Curr. Opin. Plant Biol. 54, 34–41. doi:10.1016/j.pbi.2019.12.010
Windram, O., Madhou, P., McHattie, S., Hill, C., Hickman, R., Cooke, E., et al. (2012). Arabidopsis Defense against Botrytis Cinerea: Chronology and Regulation Deciphered by High-Resolution Temporal Transcriptomic Analysis. The Plant Cell 24 (9), 3530–3557. doi:10.1105/tpc.112.102046
Woo, J. W., Kim, J., Kwon, S. I., Corvalán, C., Cho, S. W., Kim, H., et al. (2015). DNA-free Genome Editing in Plants with Preassembled CRISPR-Cas9 Ribonucleoproteins. Nat. Biotechnol. 33 (11), 1162–1164. doi:10.1038/nbt.3389
Zager, J. J., Lange, I., Srividya, N., Smith, A., and Lange, B. M. (2019). Gene Networks Underlying Cannabinoid and Terpenoid Accumulation in Cannabis. Plant Physiol. 180 (4), 1877–1897. doi:10.1104/pp.18.01506
Zhang, T.-Q., Lian, H., Zhou, C.-M., Xu, L., Jiao, Y., and Wang, J.-W. (2017). A Two-step Model for De Novo Activation of WUSCHEL during Plant Shoot Regeneration. Plant Cell 29 (5), 1073–1087. doi:10.1105/tpc.16.00863
Zhang, X., Xu, G., Cheng, C., Lei, L., Sun, J., Xu, Y., et al. (2021). Establishment of an Agrobacterium ‐mediated Genetic Transformation and CRISPR/Cas9‐mediated Targeted Mutagenesis in Hemp (Cannabis Sativa L.). Plant Biotechnol. J. 19, 1979–1987. doi:10.1111/pbi.13611
Zhang, Y., Liang, Z., Zong, Y., Wang, Y., Liu, J., Chen, K., et al. (2016). Efficient and Transgene-free Genome Editing in Wheat through Transient Expression of CRISPR/Cas9 DNA or RNA. Nat. Commun. 7 (1), 1–8. doi:10.1038/ncomms12617
Keywords: HEMP, non-transgenic, genome editing, cannabinoids, tissue culture, next generation technologies, artificial intelligence for crop improvement
Citation: Shiels D, Prestwich BD, Koo O, Kanchiswamy CN, O'Halloran R and Badmi R (2022) Hemp Genome Editing—Challenges and Opportunities. Front. Genome Ed. 4:823486. doi: 10.3389/fgeed.2022.823486
Received: 27 November 2021; Accepted: 05 January 2022;
Published: 02 February 2022.
Edited by:
Jimmy R. Botella, The University of Queensland, AustraliaReviewed by:
Ethan Budd Russo, Phytecs, United StatesCopyright © 2022 Shiels, Prestwich, Koo, Kanchiswamy, O'Halloran and Badmi. This is an open-access article distributed under the terms of the Creative Commons Attribution License (CC BY). The use, distribution or reproduction in other forums is permitted, provided the original author(s) and the copyright owner(s) are credited and that the original publication in this journal is cited, in accordance with accepted academic practice. No use, distribution or reproduction is permitted which does not comply with these terms.
*Correspondence: Raghuram Badmi, cmJhZG1pQHVjYy5pZQ==
Disclaimer: All claims expressed in this article are solely those of the authors and do not necessarily represent those of their affiliated organizations, or those of the publisher, the editors and the reviewers. Any product that may be evaluated in this article or claim that may be made by its manufacturer is not guaranteed or endorsed by the publisher.
Research integrity at Frontiers
Learn more about the work of our research integrity team to safeguard the quality of each article we publish.