- 1Department of Hematology and Oncology, University Children’s Hospital, University of Tübingen, Tübingen, Germany
- 2Abu Dhabi Stem Cells Center, Abu Dhabi, United Arab Emirates
Blood disorders are a group of diseases including hematological neoplasms, clotting disorders and orphan immune deficiency diseases that affects human health. Current improvements in genome editing based therapeutics demonstrated preclinical and clinical proof to treat different blood disorders. Genome editing components such as Cas nucleases, guide RNAs and base editors are supplied in the form of either a plasmid, an mRNA, or a ribonucleoprotein complex. The most common delivery vehicles for such components include viral vectors (e.g., AAVs and RV), non-viral vectors (e.g., LNPs and polymers) and physical delivery methods (e.g., electroporation and microinjection). Each of the delivery vehicles specified above has its own advantages and disadvantages and the development of a safe transferring method for ex vivo and in vivo application of genome editing components is still a big challenge. Moreover, the delivery of genome editing payload to the target blood cells possess key challenges to provide a possible cure for patients with inherited monogenic blood diseases and hematological neoplastic tumors. Here, we critically review and summarize the progress and challenges related to the delivery of genome editing elements to relevant blood cells in an ex vivo or in vivo setting. In addition, we have attempted to provide a future clinical perspective of genome editing to treat blood disorders with possible clinical grade improvements in delivery methods.
Introduction
Genome editing technologies have been extensively used in scientific research with the aim of genome modification. Zinc-finger nucleases (ZFNs), transcription activator-like effector nucleases (TALENs), and meganucleases (MegNs) are the previously developed approaches for targeted genetic editing (Gaj et al., 2013; Alagoz and Kherad, 2020; Khalil, 2020). The main disadvantages of ZFNs are low targeting efficacy and reduced specificity, while MegNs have low design flexibility. TALENs have shown to be highly efficient and specific. It is rather their design, assembly and construction that are cumbersome and that have limited their use, than their performance (Xu et al., 2020; Siva et al., 2021). Juillerat et al. modified the TALE scaffold by implementing non-conventional repeat variable diresidue (ncRVDs) and could improve TALEN-mediated specificity to target HBB locus and reduce off-site targeting (Juillerat et al., 2015). The subsequent development of clustered regularly interspaced short palindromic repeats-CRISPR-associated protein 9 (CRISPR-Cas9) as a powerful genome editing tool initiated notable improvement in the field of gene therapy due to its versatility and ease of use.
CRISPR-Cas system is originated from the microbial immune system and its application is more convenient and flexible than other engineered nucleases (Adli, 2018; Siva et al., 2021). Target-specific single guide RNA (sgRNA) and Cas endonuclease are the two main components of the CRISPR-Cas system. sgRNA is composed of custom-designed short CRISPR RNAs (crRNAs) and the scaffold so called trans-activating crRNA sequence (tracrRNA) (Yip, 2020). According to a recently published classification, CRISPR-Cas system has 2 classes, 6 types and 33 subtypes (Makarova et al., 2020), among them type II CRISPR-Cas system is the most frequently used type which uses Streptococcus pyogenes Cas9 endonuclease (Rodríguez-Rodríguez et al., 2019). During the editing process, sgRNA makes a complex with Cas9 directing it to the target site. After recognition of the protospacer-adjacent motif (PAM), Cas9, creates a double strand break (DSB) in the target site (Rodríguez-Rodríguez et al., 2019; Zhao et al., 2021). The induced DSBs can be repaired through two main DNA repair pathways in the cells: non-homologous end-joining (NHEJ) which is preferred for making gene knockout and homology-directed repair (HDR) pathway which is important for knock in strategy (Klaver-Flores et al., 2020). Base and prime editing are the recently developed CRISPR-Cas based genome editing mechanism in which DNA can be edited in the target site without the generation of DSBs, avoiding in this way potential genomic rearrangements (Kantor et al., 2020; Antoniou et al., 2021).
The CRISPR-Cas system has various important applications in medicine including identifying genes involved in different diseases, developing disease models, establishing diagnostic and therapeutic approaches, cancer immunotherapy and drug screening (Antony et al., 2018b; Ureña-Bailén et al., 2019; Sun et al., 2020; Asmamaw and Zawdie, 2021). One of the proven applications of CRISPR-Cas in human medicine is its therapeutic potential in blood disorders (Zhang and McCarty, 2016; Daniel-Moreno et al., 2019). Blood disorders include various diseases with abnormalities in different stages of hematopoiesis such as Fanconi anemia, amegakaryocytic thrombocytopenia, β-hemoglobinopathies, hemophilia and cancer (Daniel-Moreno et al., 2019). A critical step in CRISPR-Cas based gene therapy for blood cells is choosing the appropriate delivery strategy to transfer the CRISPR components into the cells. Safety and specificity are two major concerns of applying CRISPR-Cas therapeutics in target cells, particularly in translational medicine. It is crucial that the selected delivery system can efficiently transfer the editing tools to the target cells and lead to high editing efficiency and low off target effects (Lino et al., 2018). This issue is more critical in hematopoietic stem and progenitor cells (HSPCs) because an inaccurate delivery system can introduce genotoxicity and impact their stem cell properties. (Cannon et al., 2021). In this review, we discuss the progress of CRISPR-Cas based gene therapy in blood disorders focusing on the pros and cons of different methods for the delivery of CRISPR components into the blood cells.
CRISPR-Cas9 components
Cas9 and sgRNA
CRISPR-Cas9 system can be delivered into the cells in three common forms: DNA, RNA and protein (Figure 1). In the DNA format method, one or two plasmids have to be introduced in the nucleus of cells to encode for Cas9 protein and sgRNA (Shalaby et al., 2020). This strategy can increase cellular toxicity in HSPCs due to persistent plasmid expression and induction of undesired mutations (Seema Rani Padhiary, 2021). Lattanzi et al. (2019) showed that using CRISPR-Cas9 plasmids to target hereditary persistence of fetal hemoglobin (HPFH) like region in HSPCs leads to a high frequency of genomic rearrangements (about 30%) and reactivation of γ-globin gene expression but also induce significant cell toxicity. In the RNA form, Cas9-encoding mRNA and the sgRNA can be delivered to the cell at the same time. In this method, low stability of RNA may result in low editing efficiency (Hendel et al., 2015). On the other hand, there is no risk of genomic insertional mutagenesis and the transient expression favors less off-target activity. Since Cas9 mRNA does not need to enter the nucleus, the editing process is also accelerated (Antony et al., 2018a; Behr et al., 2021). Transferring Cas9 protein and gRNA as an RNP complex can solve molecular stability issues, providing high editing efficiency and low toxicity due to immediate gene editing (González-Romero et al., 2019; Yip, 2020).
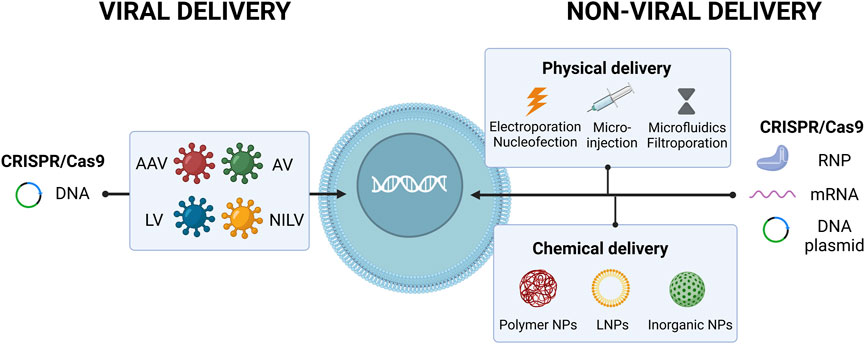
FIGURE 1. Schematic diagrams of CRISPR-Cas delivery formats and methods. CRISPR-Cas system can be delivered into the cells in DNA, RNA or RNP format. Delivery strategies of CRISPR-Cas9 system are categorized into three groups: physical delivery, chemical delivery and viral vectors.
Donor template
When the aim of gene editing is to correct a mutation or insertion of a new sequence to the genome, the CRISPR system requires an additional component, the so-called donor DNA template or repair template. Donor templates can be delivered to the cells in plasmid form, synthetic double-stranded DNA oligonucleotide (dsODN), synthetic single-stranded DNA oligonucleotide (ssODN) or viral vectors (Behr et al., 2021). Design and production of dsODNs, ssODNs is faster, simpler and more cost effective than other formats (Romero et al., 2019). Although it is recommended to use the ssODN for the insertion of short sequences and plasmids for larger ones (Song and Stieger, 2017) the perfect donor template format is not clearly known yet. Various factors are involved in ODN design and can influence the efficiency of HDR including size, orientation, length of homology arms and symmetry. ssODNs are usually designed by ≥30 nucleotides homology arm at both sides of the Cas target site (Zhang et al., 2022). Efficiencies between performing HDR with shorter ODN to introduce smaller mutations or long HDR templates have challenges, especially in terms of viabilities because longer ssODNs can cause cell cytotoxicity (Okamoto et al., 2019). Moreover, it has been shown that using longer dsDNA to introduce larger sequences is less efficient due to their size which makes their transfer to the cells more difficult. Long dsODN also can negatively affect cell viability by induction of concatemers in eukaryotic cells (Ghanta et al., 2021). Romero et al. compared Adeno-associated virus type 6 (AAV6) and ssODN application as donor template for CRISPR-Cas9 mediated editing of the mutation responsible for sickle cell disease (SCD) in HBB gene. The result demonstrates that using AAV6 as donor template gives rise to higher HDR rates (between 50% and 60%) in vitro in comparison to ssODN. However, in vivo analysis in immunodeficient mouse xenografts showed similar frequency for AAV6 and ssODNs. Moreover, despite of low toxicity in vitro, AAV template negatively affect the in vivo engraftment of the HSPCs (Romero et al., 2019). In another study, Ferrari et al. (2022) compared template delivery between integrase defective lentiviral vector (IDLV) and AAV (ssAAV2/6 and other AAV genome forms), showing that delivery via IDLV in HSPCs mitigated genotoxic burden, thus confirming the intrinsic issues when using AAV. Nguyen et al. added truncated Cas9 target sequence (tCTSs) to the end of HDR template to facilitate transferring of the template to the nucleus through interaction with RNP and could increase knock-in efficiency (Nguyen et al., 2020). To improve this method by decreasing the cell toxicity of dsDNA, Shy et al. developed a hybrid HDR template by cooperating of a long ssDNA and short dsDNA including CTS on both sides. Applying this method in combination with HDR enhancing molecules in different genetic loci and various types of primary hematopoietic cells resulted in enhanced knock in efficiency and yield (Shy et al., 2022).
Delivery methods
The ability to deliver gene editing components safely and efficiently into the cells is a critical issue for successful gene therapy. In general, gene editing components such as nucleases and guide RNAs can be delivered into the cells through three strategies: physical delivery, chemical delivery and viral vectors (Figure 1). Each method has highlights and challenges. Choosing the appropriate delivery system depends on the status of the experiment (in vitro or in vivo), type of cargo (DNA, mRNA, or protein) and the targeted cell or organ type. Moreover, different technical challenges including efficiency, specificity, risk of insertional mutagenesis and immune response induction have to be considered in this context (Yang et al., 2021).
Physical delivery
Electroporation is an electro-physical, non-viral and fast method for the delivery of exogenous materials into the cells and tissues. In this method, an electric field is utilized to disturb the phospholipid bilayer of the membrane, thereby inducing the formation of temporary pores which allow the delivery of external molecules into cells (Bao et al., 2010; Yip, 2020). Electroporation is safer and more economical compared to viral methods, however, when it is not properly optimized, it can lead to cell death, especially in stress-sensitive cells. The major advantage of electroporation is its applicability for different types of cells including blood cells (Yip, 2020) although in case of using high intensity pulses may lead to changes in the properties of HSPCs (DiTommaso et al., 2018). Electroporation is widely in use for the delivery of CRISPR components into the blood cells ex vivo. In this strategy, after electroporation of the CRISPR components, ex vivo edited hematopoietic stem/progenitor cells of the patients are transplanted back. Vuelta et al. (2020) used electroporation for the delivery of the Cas9 RNP complex for disrupting BCR/ABL1 oncogene in leukemic stem cells. Transplantation of ex vivo edited cells restored normal hematopoiesis in NSG mice. Other preclinical studies also reported promising results after using electroporation for in vitro delivery of CRISPR for the treatment of blood disorders (Xie et al., 2014; Smith et al., 2015; DeWitt et al., 2016). Electroporation is an acceptable method in CRISPR-based cancer immunotherapy for in vitro and ex vivo manipulation of immune cells including T cells, B cells and natural killer (NK) cells (Naeimi Kararoudi et al., 2018; Wu et al., 2018; Afolabi et al., 2019; Greiner et al., 2019). More importantly, several clinical studies are ongoing using electroporation for CRISPR based gene editing in blood disorders (Table 1). CTX001, a CRISPR gene-edited therapy, for the treatment of β-thalassemia and sickle cell disease, is in phase 2/3 of clinical trial (NCT03655678 and NCT03745287). In these clinical trials, hematopoietic stem cells are electroporated with CRISPR-Cas9 to target the BCL11A gene and demonstrated to produce high levels of fetal hemoglobin (Frangoul et al., 2021). Nucleofection, a modified form of electroporation for direct delivery of nucleic acids into the nucleus of different cells, has also been proven to be an effective way of transfecting human CD34+ cells (von Levetzow et al., 2006; Antony et al., 2018b; Vaidyanathan et al., 2018).
Microinjection is another physical delivery method in which genome editing components can be directly injected into cells under a microscope using very small needles (Elaswad et al., 2018). This method is suitable for in vitro and ex vivo delivery of the CRISPR system and is used mainly for embryonic genome editing and the creation of transgenic animal models (Horii et al., 2014; Ma et al., 2014). Microinjection can be considered a potential method for CRISPR delivery in human blood stem/progenitor cells as delivery of macromolecules into the HSPCs without negative effect on cellular function is previously shown (Anderson et al., 1980; Davis et al., 2000). However, the processing of only one cell at a time can make the procedure more labor and time-consuming in comparison to other delivery methods.
The microfluidic device is a membrane deformation-based delivery system that uses physical constriction to change the shape of the cell and create transient pores in the cell membrane. Consequently, the crossing of a variety of biomolecules such as CRISPR components by passive diffusion is enabled (Zhang et al., 2021). Ma et al. (2017) developed a specific microfluidic chip for HSPCs. This Nano-Blade Chip (NB-Chip) is designed using silicon instead of polydimethylsiloxane (PDMS). Interestingly, using NB-Chip for transferring macro-molecules or plasmids into the HSPCs was more effective than electroporation in terms of longer persistence of HSPCs’ inherent pluripotency. They could deliver CRISPR in RNP complex format to the human HSPCs and disrupt CCAAT/enhancer-binding protein-α (C/EBPα/CEBPA) p42 in vitro.
Filtroporation is another approach for the delivery of CRISPR system in HSPCs. In this method, cells are forced to pass through microporous membranes to increase the permeability of the cells (Stewart et al., 2018; Zhang et al., 2021). Transmembrane internalization assisted by membrane filtration (TRIAMF) is based on the filter membrane cell permeabilization technique to deliver the RNPs to CD34+ HSPCs (Yen et al., 2018). Using this system, Yen et al. (2018) could induce indels in the γ-globin gene in HSPCs in vitro (44% for HBG2 and 33% for HBG1 site). Ex vivo TRIAMF/RNP treatment of HSPCs did not change the engraftment competency and multilineage potential in (SCID)/Il2rg−/− (NSG) mice.
Chemical delivery
Chemical vectors are the other alternative for the non-viral delivery of CRISPR components into the cells. These methods are safer than viral vectors and do not apply much stress on cells in comparison to physical delivery (Yip, 2020).
Lipid materials such as liposomes and lipid nanoparticles (LNPs) provide a safe and efficient delivery method for nucleic acids. Due to their negative charge, nucleic acids are not able to enter the cells through the membrane but their encapsulation into liposomes eases the way for crossing the membrane (Pensado et al., 2014). In a recent study, lipid nanoparticles are used for the delivery of Cas9 mRNA and guide RNA to target antithrombin in hemophilia mouse models (Han et al., 2022). Antithrombin is a thrombin inhibitor and anticoagulant encoded by the serpin family C member 1 (SERPINC1) gene. Reduction of antithrombin level is important for balancing coagulation and hemostasis in hemophilia (Sehgal et al., 2015). Han et al. (2022) could down-regulate the function of Serpinc1 gene by 70% using LNP-based delivery of the CRISPR-Cas9 editing system and improved thrombin generation in both hemophilia A and B mouse models without reported off target effects. Their results showed that unlike viral vectors, repeated in vivo application of LNPs is not problematic in terms of induction of immune response. Intellia Therapeutics in cooperation with Regeneron have developed a CRISPR-mediated treatment research program for hemophilia A and B. In this program, they use LNPs to insert a transgene in the liver of non-human primates to produce human Factor IX, which is necessary to treat hemophilia A and B (https://www.intelliatx.com/our-pipeline/). In another study, Ho et al. (2021) delivered bioreducible lipidoid-encapsulated Cas9-sgRNA into human leukemia stem cells (LSCs) to knock-out interleukin-1 receptor accessory protein (IL1RAP). It led to decreased clonogenicity of leukemia cells in vitro and reduced leukemic burden in vivo.
Inorganic nanoparticles, in particular gold nanoparticles (AuNPs), are another interesting option for delivery of genetic materials into the cells. These kinds of nanoparticles can be adaptable to different sizes and chemical modifications and can be applicable in combining with lipids or polymers. Moreover, they are less toxic for cells than lipid and polymer nanocarriers (Ding et al., 2014; Behr et al., 2021). Shahbazi et al. (2019) developed a gold nanoparticles-based delivery for CRISPR gene editing system in HSPCs. The multilayered AuNP/CRISPR nanoformulation used by this group could be detected by confocal microscopy imaging 6h after treatment in HSPCs. They could also target γ-globin promoter on chromosome 11 and reach 12.1% total editing in this region. The result showed no impact on colony formation and xenograft analysis after infusion of ex vivo edited CD34+ HSPC into neonatal immune-deficient mice.
Polymer-based nanoparticles use the same strategy as LNPs for delivery of CRISPR components in different forms through the cell membrane. Polymeric NPs have high stability and capacity for cargo encapsulation (Zielińska et al., 2020; Piperno et al., 2021). El-Kharrag et al. (2022) compared the polymer-based nanoparticles delivery and electroporation of mRNA and nucleases into human granulocyte colony-stimulating factor (GCSF)-mobilized CD34+ cells with electroporation method. They found that despite similar efficiency, polymer NPs based delivery needs three times less reagents than electroporation. They also proposed PBAE-NPs as an efficient method for CRISPR-Cas9 gene editing in HSPCs in vivo.
Viral delivery
Different viral vectors have been used for the delivery of CRISPR components as natural delivery systems. Adeno-associated virus (AAV) is a parvovirus with no report of causing disease in human (Lau and Suh, 2017). Mitochondrial DNA and AAVS1 site on chromosome 19 are known as integration sites for AAV, although they are considered safe locations without the risk of tumorigenesis (Kaeppel et al., 2013; Xu et al., 2019). Besides natural AAVs, recombinant AAV vectors are also designed with the aim of increasing the transduction efficiency and decreasing immune response (Li and Samulski, 2020). Because of their safety and therapeutic potential, AAVs are attractive vehicles for in vivo delivery of gene editing elements into a wide range of cells. AAV-CRISPR mediated gene therapy is a promising approach for the treatment of blood disorders especially hemophilia. Different studies have assessed the efficiency of AAVs as a delivery method for gene editing in hemophilia A and B in vitro and in vivo (Chen et al., 2019; Gao et al., 2019; Zhang et al., 2019; Wang et al., 2020).
CRISPR components can be transferred to the cells using either single or dual viral vectors. Gao et al. (2019) compared these two methods for gene editing in hemophilia B cells (Huh7-cFIXmut cells). In one strategy, they transduced cells with adenovirus vector type 5 (HCAdV5) carrying CRISPR-Cas9 and single-stranded adeno-associated virus type 2 vector (ssAAV2) carrying modified donor. In the second strategy, they utilized a single HCAdV5 for the delivery of all components of repair. They found that single vector application is more efficient than two vectors. Although Wang et al. (2020) showed that dual AAV vectors (AAV8.SpCas9 and AAV8.sgRNA. donor vector) application in vivo, is a safe method to integrate partial human FIX (hFIX) in mouse albumin (mAlb) and enhance the coagulation in hemophilia B mice.
Chen et al. (2019) used two recombinant AAV8 vectors containing Cas9-gRNA and codon-optimized human B-domain-deleted human FVIII (BDD-F8) encoding sequence to insert BDD-F8 at liver-specific albumin (Alb) locus in hemophilia A mouse model. This treatment resulted in the improvement of the disease phenotype and increasing of FVIII protein and activity levels in mice liver without toxicity for 7 months. Different studies have employed AAVs as delivery method for the treatment of human immunodeficiencies such as chronic granulomatous disease (Sweeney et al., 2017) and human immunodeficiency virus (HIV) infection (Sather et al., 2015; Yin et al., 2017; Dash et al., 2019). Nahmad et al. (2022) succeeded in in vivo engineering of B cells using AAV vectors (dual AAV-DJ) for the delivery of CRISPR and the production of therapeutic antibodies against HIV in mice. Recently, Excision Bio Therapeutics has started a phase 1/2 clinical trial evaluating EBT-101, a CRISPR-Cas9 based therapy, using AAV9 for delivery through intravenous (IV) administration in aviremic HIV-1 infected adults (NCT05144386). AAV vectors are not the optimal delivery methods for all cell types because the viral genome can remain in the cells as an episome and AAV capsid proteins may lead to immune responses (Park et al., 2016; Chen et al., 2019).
Adenoviral vectors (AVs) have higher capacity (>8 kB) which makes possible a single transferring of Cas9 and sgRNAs in only one vector. AVs can be delivered into both dividing and non-dividing cells without integration in the host genome, nevertheless, there is a high risk of induction of immune response in cells after AVs transduction (Wilbie et al., 2019; Yip, 2020). Guan et al. (2016) compared two different strategies including naked DNA constructs and adenoviral vectors for the delivery of Cas9 component to hemophilia B mice models carrying F9 Y371D mutation. They reported that although using adenoviral delivery system leads to higher editing efficiency than naked DNA, it also shows severe hepatic toxicity. Helper-dependent Ad (HDAd) is the recombinant form of AVs in which all viral genome is deleted except the packaging sequence and cis-acting ITRs, contributing to high transgene capacity (Vetrini and Ng, 2010; Rosewell Shaw et al., 2022). HDAd expressing CRISPR-Cas9 is utilized to reactivate human γ-globin by disrupting the repressor binding site in γ-globin promoter in HSPCs and β-YAC/CD46–transgenic mice (Li et al., 2018). The result showed an increase of the HBG/HBB expression ratio ex vivo and in vivo without negative effect on hematopoiesis (Li et al., 2018). A subsequent study has utilized HSPCs transduction with HDAd vectors expressing CRISPR and globin donor ex vivo and in vivo and could reach stable levels of γ-globin expression (Li et al., 2019).
Retroviral vectors including gamma-retrovirus and lentivirus vectors are also used by scientists as gene therapy tools (Ghosh et al., 2020). Lentiviral vector systems are interesting especially for ex vivo gene editing because of their high capacity for carrying complex transgenes and their ability to express in both dividing and non-dividing cells (Lattanzi et al., 2019; Gutierrez-Guerrero et al., 2020; Dong and Kantor, 2021). Therefore, these vectors are considered efficient methods for the delivery of CRISPR components into the cells. However, long-term expression and high frequency of off target effects due to the integration of LVs into the genome is the biggest limitation of these vectors. Lentiviral based CRISPR delivery system is used for the disruption of different genes involved in blood disorders. Silencing of mucin 1 C-terminal subunit (MUC1-C), an oncogenic transmembrane protein, by CRISPR-Cas9 promotes the reduction of MYC oncoprotein expression and β-catenin levels in multiple myeloma (MM) cells (Daniel and Molta, 1989). Gene disruption in the HBG1/HBG2 promoter sequence by lentivirus expressing Cas9 and guide RNA increased HbF levels in CD34+ cells to simulate a natural benign condition that prevents the symptoms of HBB mutation in SCD and β-thalassemia (Traxler et al., 2016).
Using lentivirus for introducing edited ABL gene into leukemia xenograft mouse model showed significant inhibition of leukemia cell growth (Chen et al., 2020). Further on, Chen et al. (2020) transduced patient CML cells ex vivo with a lentivirus based CRISPR-Cas9 genome editing system and obtained more than 30.9% indel frequency without reporting off-target effects. They could show that the ABL-targeted CRISPR-Cas9 virus can lead to a high rate of apoptosis in CML cells. On the contrary, different studies have shown that gammaretroviral vectors-mediated gene therapy approaches in hematopoietic stem cells can result in serious side effects such as leukemogenic risks (Hacein-Bey-Abina et al., 2003a; Hacein-Bey-Abina et al., 2003b; Ott et al., 2006; Stein et al., 2010; Braun et al., 2014).
Due to the increasing concern about the inevitable risk of insertional mutagenesis, vector design has improved, and new generations of SIN gamma- and lentiviral vectors with inactivated LTRs have been developed to minimize the oncogenic potential and improve their use in clinical settings (Engelman et al., 1995; Hacein-Bey-Abina et al., 2014; Shaw and Cornetta, 2014; Daniel-Moreno et al., 2020). These modified viruses will harbor non-replicating episomes, which have certain limitations such as loss over time in rapid-dividing cells, lower transgene expression in comparison to traditional lentiviruses and risk of residual integration (Luis, 2020; Gurumoorthy et al., 2022). Nevertheless, integrase defective lentiviruses (IDLVs) can effectively transduce HSPCs and have proven to be effective donor template carriers in preclinical studies of X-linked severe combined immunodeficiency (X-SCID) therapy (Genovese et al., 2014) and CRISPR-Cas9 deliverer for the repair of patient-specific frameshift point mutations (CYBB) involved in chronic granulomatous disease (XCGD) (Sürün et al., 2018).
Discussion
In recent years, the emergence and development of the CRISPR-Cas system have made a great revolution in genome editing technology. Pre-clinical and clinical results of applying this technique for the treatment of different genetic disorders are very promising. On target and off target cutting, homology directed repair efficiency, proper guide RNA and donor template selection and suitable delivery method are critical considerations in CRISPR based gene editing (Lino et al., 2018). In this review, we summarized the state of art for the delivery methods of the CRISPR components into the cells with a focus on gene editing in blood cells. It has been shown that despite many attractive features and high gene editing efficiency, viral vectors have significant limitations. Recent findings concerning the integration of AVV vectors into the CRISPR induced DSBs sites have questioned the safety of these vectors for CRISPR-based gene therapy (Hanlon et al., 2019). The induction of immunogenicity and cellular toxicity by some types of adenovirus and the risk of insertional mutagenesis by lentiviral vectors is still challenging for using these vectors in gene editing (Bulcha et al., 2021). Physical methods are likely to be the most convenient method for ex vivo therapy development but are not feasible for treatments based on gene editing in vivo, where non-carriers such as lipid or polymer-based NPs seems to have a brighter future. Overall, we believe that the substantial progress and optimization in current delivery options will promote the CRISPR-Cas9 application for the treatment of blood disorders in the coming decades.
Author contributions
TMG wrote the main part of the manuscript, followed by the contributions of GU-B who created the figure and gave input to the manuscript. GU-B, YH, RS, and RH performed proofreading. MM and JA drafted the final version of the manuscript. All authors read and approved the final manuscript.
Funding
This study was financially supported by the research funding programs Fortüne Tübingen (Nos. 2485-0-0 and 2412-0-0), Clinician Scientist Program (No. 440-0-0), Stefan Morsch Stiftung, Förderverein für krebskranke Kinder Tübingen e.V. and the University Children’s Hospital of Tübingen. We acknowledge support by Open access publishing fund of University of Tübingen.
Conflict of interest
The authors declare that the research was conducted in the absence of any commercial or financial relationships that could be construed as a potential conflict of interest.
Publisher’s note
All claims expressed in this article are solely those of the authors and do not necessarily represent those of their affiliated organizations, or those of the publisher, the editors and the reviewers. Any product that may be evaluated in this article, or claim that may be made by its manufacturer, is not guaranteed or endorsed by the publisher.
References
Adli, M. (2018). The CRISPR tool kit for genome editing and beyond. Nat. Commun. 9 (1), 1911. doi:10.1038/s41467-018-04252-2
Afolabi, L. O., Adeshakin, A. O., Sani, M. M., Bi, J., and Wan, X. (2019). Genetic reprogramming for NK cell cancer immunotherapy with CRISPR/Cas9. Immunology 158 (2), 63–69. doi:10.1111/imm.13094
Alagoz, M., and Kherad, N. (2020). Advance genome editing technologies in the treatment of human diseases: CRISPR therapy (review). Int. J. Mol. Med. 46 (2), 521–534. doi:10.3892/ijmm.2020.4609
Anderson, W. F., Killos, L., Sanders-Haigh, L., Kretschmer, P. J., and Diacumakos, E. G. (1980). Replication and expression of thymidine kinase and human globin genes microinjected into mouse fibroblasts. Proc. Natl. Acad. Sci. U. S. A. 77 (9), 5399–5403. doi:10.1073/pnas.77.9.5399
Antoniou, P., Miccio, A., and Brusson, M. (2021). Base and prime editing technologies for blood disorders. Front. Genome Ed. 3, 618406. doi:10.3389/fgeed.2021.618406
Antony, J. S., Haque, A. A., Lamsfus-Calle, A., Daniel-Moreno, A., Mezger, M., and Kormann, M. S. (2018a). CRISPR/Cas9 system: A promising technology for the treatment of inherited and neoplastic hematological diseases. Adv. Cell. Gene Ther. 1 (1), e10. doi:10.1002/acg2.10
Antony, J. S., Latifi, N., Haque, A. K. M. A., Lamsfus-Calle, A., Daniel-Moreno, A., Graeter, S., et al. (2018b). Gene correction of HBB mutations in CD34+ hematopoietic stem cells using Cas9 mRNA and ssODN donors. Mol. Cell. Pediatr. 5 (1), 9. doi:10.1186/s40348-018-0086-1
Asmamaw, M., and Zawdie, B. (2021). Mechanism and applications of CRISPR/Cas-9-Mediated genome editing. Biologics 15, 353–361. doi:10.2147/btt.S326422
Bao, N., Le, T. T., Cheng, J. X., and Lu, C. (2010). Microfluidic electroporation of tumor and blood cells: Observation of nucleus expansion and implications on selective analysis and purging of circulating tumor cells. Integr. Biol. (Camb) 2 (2-3), 113–120. doi:10.1039/b919820b
Behr, M., Zhou, J., Xu, B., and Zhang, H. (2021). In vivo delivery of CRISPR-Cas9 therapeutics: Progress and challenges. Acta Pharm. Sin. B 11 (8), 2150–2171. doi:10.1016/j.apsb.2021.05.020
Braun, C. J., Boztug, K., Paruzynski, A., Witzel, M., Schwarzer, A., Rothe, M., et al. (2014). Gene therapy for Wiskott-Aldrich syndrome-long-term efficacy and genotoxicity. Sci. Transl. Med. 6 (227), 227ra33. doi:10.1126/scitranslmed.3007280
Bulcha, J. T., Wang, Y., Ma, H., Tai, P. W. L., and Gao, G. (2021). Viral vector platforms within the gene therapy landscape. Signal Transduct. Target Ther. 6 (1), 53. doi:10.1038/s41392-021-00487-6
Cannon, P., Asokan, A., Czechowicz, A., Hammond, P., Kohn, D. B., Lieber, A., et al. (2021). Safe and effective in vivo targeting and gene editing in hematopoietic stem cells: Strategies for accelerating development. Hum. Gene Ther. 32 (1-2), 31–42. doi:10.1089/hum.2020.263
Chen, H., Shi, M., Gilam, A., Zheng, Q., Zhang, Y., Afrikanova, I., et al. (2019). Hemophilia A ameliorated in mice by CRISPR-based in vivo genome editing of human Factor VIII. Sci. Rep. 9 (1), 16838. doi:10.1038/s41598-019-53198-y
Chen, S. H., Hsieh, Y. Y., Tzeng, H. E., Lin, C. Y., Hsu, K. W., Chiang, Y. S., et al. (2020). ABL genomic editing sufficiently abolishes oncogenesis of human chronic myeloid leukemia cells in vitro and in vivo. Cancers (Basel) 12 (6), 1399. doi:10.3390/cancers12061399
Daniel, H. I., and Molta, N. B. (1989). Efficacy of chloroquine in the treatment of malaria in children under five years in Baissa (Gongola State, Nigeria). Ann. Trop. Med. Parasitol. 83 (4), 331–338. doi:10.1080/00034983.1989.11812353
Daniel-Moreno, A., Lamsfus-Calle, A., Raju, J., Antony, J. S., Handgretinger, R., and Mezger, M. (2019). CRISPR/Cas9-modified hematopoietic stem cells-present and future perspectives for stem cell transplantation. Bone Marrow Transpl. 54 (12), 1940–1950. doi:10.1038/s41409-019-0510-8
Daniel-Moreno, A., Lamsfus-Calle, A., Wilber, A., Chambers, C. B., Johnston, I., Antony, J. S., et al. (2020). Comparative analysis of lentiviral gene transfer approaches designed to promote fetal hemoglobin production for the treatment of β-hemoglobinopathies. Blood Cells Mol. Dis. 84, 102456. doi:10.1016/j.bcmd.2020.102456
Dash, P. K., Kaminski, R., Bella, R., Su, H., Mathews, S., Ahooyi, T. M., et al. (2019). Sequential LASER ART and CRISPR treatments eliminate HIV-1 in a subset of infected humanized mice. Nat. Commun. 10 (1), 2753. doi:10.1038/s41467-019-10366-y
Davis, B. R., Yannariello-Brown, J., Prokopishyn, N. L., Luo, Z., Smith, M. R., Wang, J., et al. (2000). Glass needle-mediated microinjection of macromolecules and transgenes into primary human blood stem/progenitor cells. Blood 95 (2), 437–444. doi:10.1182/blood.v95.2.437
DeWitt, M. A., Magis, W., Bray, N. L., Wang, T., Berman, J. R., Urbinati, F., et al. (2016). Selection-free genome editing of the sickle mutation in human adult hematopoietic stem/progenitor cells. Sci. Transl. Med. 8 (360), 360ra134. doi:10.1126/scitranslmed.aaf9336
Ding, Y., Jiang, Z., Saha, K., Kim, C. S., Kim, S. T., Landis, R. F., et al. (2014). Gold nanoparticles for nucleic acid delivery. Mol. Ther. 22 (6), 1075–1083. doi:10.1038/mt.2014.30
DiTommaso, T., Cole, J. M., Cassereau, L., Buggé, J. A., Hanson, J. L. S., Bridgen, D. T., et al. (2018). Cell engineering with microfluidic squeezing preserves functionality of primary immune cells in vivo. Proc. Natl. Acad. Sci. U. S. A. 115 (46), E10907-E10914–e10914. doi:10.1073/pnas.1809671115
Dong, W., and Kantor, B. (2021). Lentiviral vectors for delivery of gene-editing systems based on CRISPR/Cas: Current state and perspectives. Viruses 13 (7), 1288. doi:10.3390/v13071288
El-Kharrag, R., Berckmueller, K. E., Madhu, R., Cui, M., Campoy, G., Mack, H. M., et al. (2022). Efficient polymer nanoparticle-mediated delivery of gene editing reagents into human hematopoietic stem and progenitor cells. Mol. Ther. 30 (6), 2186–2198. doi:10.1016/j.ymthe.2022.02.026
Elaswad, A., Khalil, K., Cline, D., Page-McCaw, P., Chen, W., Michel, M., et al. (2018). Microinjection of CRISPR/Cas9 protein into channel catfish, <em>Ictalurus punctatus</em>, embryos for gene editing. J. Vis. Exp. 131, 56275. doi:10.3791/56275
Engelman, A., Englund, G., Orenstein, J. M., Martin, M. A., and Craigie, R. (1995). Multiple effects of mutations in human immunodeficiency virus type 1 integrase on viral replication. J. Virol. 69 (5), 2729–2736. doi:10.1128/jvi.69.5.2729-2736.1995
Ferrari, S., Jacob, A., Cesana, D., Laugel, M., Beretta, S., Varesi, A., et al. (2022). Choice of template delivery mitigates the genotoxic risk and adverse impact of editing in human hematopoietic stem cells. Cell. Stem Cell. 29 (10), 1428–1444.e9. doi:10.1016/j.stem.2022.09.001
Frangoul, H., Altshuler, D., Cappellini, M. D., Chen, Y. S., Domm, J., Eustace, B. K., et al. (2021). CRISPR-Cas9 gene editing for sickle cell disease and β-thalassemia. N. Engl. J. Med. 384 (3), 252–260. doi:10.1056/NEJMoa2031054
Gaj, T., Gersbach, C. A., and Barbas, C. F. (2013). ZFN, TALEN, and CRISPR/Cas-based methods for genome engineering. Trends Biotechnol. 31 (7), 397–405. doi:10.1016/j.tibtech.2013.04.004
Gao, J., Bergmann, T., Zhang, W., Schiwon, M., Ehrke-Schulz, E., and Ehrhardt, A. (2019). Viral vector-based delivery of CRISPR/Cas9 and donor DNA for homology-directed repair in an in vitro model for canine hemophilia B. Mol. Ther. Nucleic Acids 14, 364–376. doi:10.1016/j.omtn.2018.12.008
Genovese, P., Schiroli, G., Escobar, G., Tomaso, T. D., Firrito, C., Calabria, A., et al. (2014). Targeted genome editing in human repopulating haematopoietic stem cells. Nature 510 (7504), 235–240. doi:10.1038/nature13420
Ghanta, K. S., Chen, Z., Mir, A., Dokshin, G. A., Krishnamurthy, P. M., Yoon, Y., et al. (2021). 5'-Modifications improve potency and efficacy of DNA donors for precision genome editing. Elife 10, e72216. doi:10.7554/eLife.72216
Ghosh, S., Brown, A. M., Jenkins, C., and Campbell, K. (2020). Viral vector systems for gene therapy: A comprehensive literature review of progress and biosafety challenges. Appl. Biosaf. 25 (1), 7–18. doi:10.1177/1535676019899502
González-Romero, E., Martínez-Valiente, C., García-Ruiz, C., Vázquez-Manrique, R. P., Cervera, J., and Sanjuan-Pla, A. (2019). CRISPR to fix bad blood: A new tool in basic and clinical hematology. Haematologica 104 (5), 881–893. doi:10.3324/haematol.2018.211359
Greiner, V., Bou Puerto, R., Liu, S., Herbel, C., Carmona, E. M., and Goldberg, M. S. (2019). CRISPR-mediated editing of the B cell receptor in primary human B cells. iScience 12, 369–378. doi:10.1016/j.isci.2019.01.032
Guan, Y., Ma, Y., Li, Q., Sun, Z., Ma, L., Wu, L., et al. (2016). CRISPR/Cas9-mediated somatic correction of a novel coagulator factor IX gene mutation ameliorates hemophilia in mouse. EMBO Mol. Med. 8 (5), 477–488. doi:10.15252/emmm.201506039
Gurumoorthy, N., Nordin, F., Tye, G. J., Wan Kamarul Zaman, W. S., and Ng, M. H. (2022). Non-integrating lentiviral vectors in clinical applications: A glance through. Biomedicines 10 (1), 107. doi:10.3390/biomedicines10010107
Gutierrez-Guerrero, A., Cosset, F. L., and Verhoeyen, E. (2020). Lentiviral vector pseudotypes: Precious tools to improve gene modification of hematopoietic cells for research and gene therapy. Viruses 12 (9), 1016. doi:10.3390/v12091016
Hacein-Bey-Abina, S., Pai, S. Y., Gaspar, H. B., Armant, M., Berry, C. C., Blanche, S., et al. (2014). A modified γ-retrovirus vector for X-linked severe combined immunodeficiency. N. Engl. J. Med. 371 (15), 1407–1417. doi:10.1056/NEJMoa1404588
Hacein-Bey-Abina, S., von Kalle, C., Schmidt, M., Le Deist, F., Wulffraat, N., McIntyre, E., et al. (2003a). A serious adverse event after successful gene therapy for X-linked severe combined immunodeficiency. N. Engl. J. Med. 348 (3), 255–256. doi:10.1056/nejm200301163480314
Hacein-Bey-Abina, S., Von Kalle, C., Schmidt, M., McCormack, M. P., Wulffraat, N., Leboulch, P., et al. (2003b). LMO2-associated clonal T cell proliferation in two patients after gene therapy for SCID-X1. Science 302 (5644), 415–419. doi:10.1126/science.1088547
Han, J. P., Kim, M., Choi, B. S., Lee, J. H., Lee, G. S., Jeong, M., et al. (2022). In vivo delivery of CRISPR-Cas9 using lipid nanoparticles enables antithrombin gene editing for sustainable hemophilia A and B therapy. Sci. Adv. 8 (3), eabj6901. doi:10.1126/sciadv.abj6901
Hanlon, K. S., Kleinstiver, B. P., Garcia, S. P., Zaborowski, M. P., Volak, A., Spirig, S. E., et al. (2019). High levels of AAV vector integration into CRISPR-induced DNA breaks. Nat. Commun. 10 (1), 4439. doi:10.1038/s41467-019-12449-2
Hendel, A., Bak, R. O., Clark, J. T., Kennedy, A. B., Ryan, D. E., Roy, S., et al. (2015). Chemically modified guide RNAs enhance CRISPR-Cas genome editing in human primary cells. Nat. Biotechnol. 33 (9), 985–989. doi:10.1038/nbt.3290
Ho, T. C., Kim, H. S., Chen, Y., Li, Y., LaMere, M. W., Chen, C., et al. (2021). Scaffold-mediated CRISPR-Cas9 delivery system for acute myeloid leukemia therapy. Sci. Adv. 7 (21), eabg3217. doi:10.1126/sciadv.abg3217
Horii, T., Arai, Y., Yamazaki, M., Morita, S., Kimura, M., Itoh, M., et al. (2014). Validation of microinjection methods for generating knockout mice by CRISPR/Cas-mediated genome engineering. Sci. Rep. 4, 4513. doi:10.1038/srep04513
Juillerat, A., Pessereau, C., Dubois, G., Guyot, V., Maréchal, A., Valton, J., et al. (2015). Optimized tuning of TALEN specificity using non-conventional RVDs. Sci. Rep. 5, 8150. doi:10.1038/srep08150
Kaeppel, C., Beattie, S. G., Fronza, R., van Logtenstein, R., Salmon, F., Schmidt, S., et al. (2013). A largely random AAV integration profile after LPLD gene therapy. Nat. Med. 19 (7), 889–891. doi:10.1038/nm.3230
Kantor, A., McClements, M. E., and MacLaren, R. E. (2020). CRISPR-Cas9 DNA base-editing and prime-editing. Int. J. Mol. Sci. 21 (17), 6240. doi:10.3390/ijms21176240
Khalil, A. M. (2020). The genome editing revolution: Review. J. Genet. Eng. Biotechnol. 18 (1), 68. doi:10.1186/s43141-020-00078-y
Klaver-Flores, S., Zittersteijn, H. A., Canté-Barrett, K., Lankester, A., Hoeben, R. C., Gonçalves, M., et al. (2020). Genomic engineering in human hematopoietic stem cells: Hype or hope? Front. Genome Ed. 2, 615619. doi:10.3389/fgeed.2020.615619
Lattanzi, A., Meneghini, V., Pavani, G., Amor, F., Ramadier, S., Felix, T., et al. (2019). Optimization of CRISPR/Cas9 delivery to human hematopoietic stem and progenitor cells for therapeutic genomic rearrangements. Mol. Ther. 27 (1), 137–150. doi:10.1016/j.ymthe.2018.10.008
Lau, C. H., and Suh, Y. (2017). In vivo genome editing in animals using AAV-CRISPR system: Applications to translational research of human disease. F1000Res 6, 2153. doi:10.12688/f1000research.11243.1
Li, C., Mishra, A. S., Gil, S., Wang, M., Georgakopoulou, A., Papayannopoulou, T., et al. (2019). Targeted integration and high-level transgene expression in AAVS1 transgenic mice after in vivo HSC transduction with HDAd5/35++ vectors. Mol. Ther. 27 (12), 2195–2212. doi:10.1016/j.ymthe.2019.08.006
Li, C., Psatha, N., Sova, P., Gil, S., Wang, H., Kim, J., et al. (2018). Reactivation of γ-globin in adult β-YAC mice after ex vivo and in vivo hematopoietic stem cell genome editing. Blood 131 (26), 2915–2928. doi:10.1182/blood-2018-03-838540
Li, C., and Samulski, R. J. (2020). Engineering adeno-associated virus vectors for gene therapy. Nat. Rev. Genet. 21 (4), 255–272. doi:10.1038/s41576-019-0205-4
Lino, C. A., Harper, J. C., Carney, J. P., and Timlin, J. A. (2018). Delivering CRISPR: A review of the challenges and approaches. Drug Deliv. 25 (1), 1234–1257. doi:10.1080/10717544.2018.1474964
Luis, A. (2020). The old and the new: Prospects for non-integrating lentiviral vector technology. Viruses 12 (10), 1103. doi:10.3390/v12101103
Ma, Y., Han, X., Quintana Bustamante, O., Bessa de Castro, R., Zhang, K., Zhang, P., et al. (2017). Highly efficient genome editing of human hematopoietic stem cells via a nano-silicon-blade delivery approach. Integr. Biol. (Camb) 9 (6), 548–554. doi:10.1039/c7ib00060j
Ma, Y., Shen, B., Zhang, X., Lu, Y., Chen, W., Ma, J., et al. (2014). Heritable multiplex genetic engineering in rats using CRISPR/Cas9. PLoS One 9 (3), e89413. doi:10.1371/journal.pone.0089413
Makarova, K. S., Wolf, Y. I., Iranzo, J., Shmakov, S. A., Alkhnbashi, O. S., Brouns, S. J. J., et al. (2020). Evolutionary classification of CRISPR-cas systems: A burst of class 2 and derived variants. Nat. Rev. Microbiol. 18 (2), 67–83. doi:10.1038/s41579-019-0299-x
Naeimi Kararoudi, M., Dolatshad, H., Trikha, P., Hussain, S. A., Elmas, E., Foltz, J. A., et al. (2018). Generation of knock-out primary and expanded human NK cells using Cas9 ribonucleoproteins. J. Vis. Exp. 136, 58237. doi:10.3791/58237
Nahmad, A. D., Lazzarotto, C. R., Zelikson, N., Kustin, T., Tenuta, M., Huang, D., et al. (2022). In vivo engineered B cells secrete high titers of broadly neutralizing anti-HIV antibodies in mice. Nat. Biotechnol. 40 (8), 1241–1249. doi:10.1038/s41587-022-01328-9
Nguyen, D. N., Roth, T. L., Li, P. J., Chen, P. A., Apathy, R., Mamedov, M. R., et al. (2020). Polymer-stabilized Cas9 nanoparticles and modified repair templates increase genome editing efficiency. Nat. Biotechnol. 38 (1), 44–49. doi:10.1038/s41587-019-0325-6
Okamoto, S., Amaishi, Y., Maki, I., Enoki, T., and Mineno, J. (2019). Highly efficient genome editing for single-base substitutions using optimized ssODNs with Cas9-RNPs. Sci. Rep. 9 (1), 4811. doi:10.1038/s41598-019-41121-4
Ott, M. G., Schmidt, M., Schwarzwaelder, K., Stein, S., Siler, U., Koehl, U., et al. (2006). Correction of X-linked chronic granulomatous disease by gene therapy, augmented by insertional activation of MDS1-EVI1, PRDM16 or SETBP1. Nat. Med. 12 (4), 401–409. doi:10.1038/nm1393
Park, C. Y., Lee, D. R., Sung, J. J., and Kim, D. W. (2016). Genome-editing technologies for gene correction of hemophilia. Hum. Genet. 135 (9), 977–981. doi:10.1007/s00439-016-1699-x
Pensado, A., Seijo, B., and Sanchez, A. (2014). Current strategies for DNA therapy based on lipid nanocarriers. Expert Opin. Drug Deliv. 11 (11), 1721–1731. doi:10.1517/17425247.2014.935337
Piperno, A., Sciortino, M. T., Giusto, E., Montesi, M., Panseri, S., and Scala, A. (2021). Recent advances and challenges in gene delivery mediated by polyester-based nanoparticles. Int. J. Nanomedicine 16, 5981–6002. doi:10.2147/ijn.S321329
Rodríguez-Rodríguez, D. R., Ramírez-Solís, R., Garza-Elizondo, M. A., Garza-Rodríguez, M. L., and Barrera-Saldaña, H. A. (2019). Genome editing: A perspective on the application of CRISPR/cas9 to study human diseases (review). Int. J. Mol. Med. 43 (4), 1559–1574. doi:10.3892/ijmm.2019.4112
Romero, Z., Lomova, A., Said, S., Miggelbrink, A., Kuo, C. Y., Campo-Fernandez, B., et al. (2019). Editing the sickle cell disease mutation in human hematopoietic stem cells: Comparison of endonucleases and homologous donor templates. Mol. Ther. 27 (8), 1389–1406. doi:10.1016/j.ymthe.2019.05.014
Rosewell Shaw, A., Porter, C., Biegert, G., Jatta, L., and Suzuki, M. (2022). HydrAd: A helper-dependent adenovirus targeting multiple immune pathways for cancer immunotherapy. Cancers (Basel) 14 (11), 2769. doi:10.3390/cancers14112769
Sather, B. D., Romano Ibarra, G. S., Sommer, K., Curinga, G., Hale, M., Khan, I. F., et al. (2015). Efficient modification of CCR5 in primary human hematopoietic cells using a megaTAL nuclease and AAV donor template. Sci. Transl. Med. 7 (307), 307ra156. doi:10.1126/scitranslmed.aac5530
Seema Rani Padhiary, S. S. (2021). Role of CRISPR-cas/9 in hematological disorders: A review. Arch Biomed Eng Biotechnol 5 (3). (ABEB.MS.ID.000613). doi:10.33552/abeb.2021.05.000613
Sehgal, A., Barros, S., Ivanciu, L., Cooley, B., Qin, J., Racie, T., et al. (2015). An RNAi therapeutic targeting antithrombin to rebalance the coagulation system and promote hemostasis in hemophilia. Nat. Med. 21 (5), 492–497. doi:10.1038/nm.3847
Shahbazi, R., Sghia-Hughes, G., Reid, J. L., Kubek, S., Haworth, K. G., Humbert, O., et al. (2019). Targeted homology-directed repair in blood stem and progenitor cells with CRISPR nanoformulations. Nat. Mater 18 (10), 1124–1132. doi:10.1038/s41563-019-0385-5
Shalaby, K., Aouida, M., and El-Agnaf, O. (2020). Tissue-specific delivery of CRISPR therapeutics: Strategies and mechanisms of non-viral vectors. Int. J. Mol. Sci. 21 (19), 7353. doi:10.3390/ijms21197353
Shaw, A., and Cornetta, K. (2014). Design and potential of non-integrating lentiviral vectors. Biomedicines 2 (1), 14–35. doi:10.3390/biomedicines2010014
Shy, B. R., Vykunta, V. S., Ha, A., Talbot, A., Roth, T. L., Nguyen, D. N., et al. (2022). High-yield genome engineering in primary cells using a hybrid ssDNA repair template and small-molecule cocktails. Nat. Biotechnol. doi:10.1038/s41587-022-01418-8
Siva, N., Gupta, S., Gupta, A., Shukla, J. N., Malik, B., and Shukla, N. (2021). Genome-editing approaches and applications: A brief review on CRISPR technology and its role in cancer. 3 Biotech. 11 (3), 146. doi:10.1007/s13205-021-02680-4
Smith, C., Abalde-Atristain, L., He, C., Brodsky, B. R., Braunstein, E. M., Chaudhari, P., et al. (2015). Efficient and allele-specific genome editing of disease loci in human iPSCs. Mol. Ther. 23 (3), 570–577. doi:10.1038/mt.2014.226
Song, F., and Stieger, K. (2017). Optimizing the DNA donor template for homology-directed repair of double-strand breaks. Mol. Ther. Nucleic Acids 7, 53–60. doi:10.1016/j.omtn.2017.02.006
Stein, S., Ott, M. G., Schultze-Strasser, S., Jauch, A., Burwinkel, B., Kinner, A., et al. (2010). Genomic instability and myelodysplasia with monosomy 7 consequent to EVI1 activation after gene therapy for chronic granulomatous disease. Nat. Med. 16 (2), 198–204. doi:10.1038/nm.2088
Stewart, M. P., Langer, R., and Jensen, K. F. (2018). Intracellular delivery by membrane disruption: Mechanisms, strategies, and concepts. Chem. Rev. 118 (16), 7409–7531. doi:10.1021/acs.chemrev.7b00678
Sun, J. Y., Hu, H. B., Cheng, Y. X., and Lu, X. J. (2020). CRISPR in medicine: Applications and challenges. Brief. Funct. Genomics 19 (3), 151–153. doi:10.1093/bfgp/elaa011
Sürün, D., Schwäble, J., Tomasovic, A., Ehling, R., Stein, S., Kurrle, N., et al. (2018). High efficiency gene correction in hematopoietic cells by donor-template-free CRISPR/Cas9 genome editing. Mol. Ther. Nucleic Acids 10, 1–8. doi:10.1016/j.omtn.2017.11.001
Sweeney, C. L., Zou, J., Choi, U., Merling, R. K., Liu, A., Bodansky, A., et al. (2017). Targeted repair of CYBB in X-CGD iPSCs requires retention of intronic sequences for expression and functional correction. Mol. Ther. 25 (2), 321–330. doi:10.1016/j.ymthe.2016.11.012
Traxler, E. A., Yao, Y., Wang, Y. D., Woodard, K. J., Kurita, R., Nakamura, Y., et al. (2016). A genome-editing strategy to treat β-hemoglobinopathies that recapitulates a mutation associated with a benign genetic condition. Nat. Med. 22 (9), 987–990. doi:10.1038/nm.4170
Ureña-Bailén, G., Lamsfus-Calle, A., Daniel-Moreno, A., Raju, J., Schlegel, P., Seitz, C., et al. (2019). CRISPR/Cas9 technology: Towards a new generation of improved CAR-T cells for anticancer therapies. Briefings Funct. Genomics 19 (3), 191–200. doi:10.1093/bfgp/elz039
Vaidyanathan, S., Azizian, K. T., Haque, A., Henderson, J. M., Hendel, A., Shore, S., et al. (2018). Uridine depletion and chemical modification increase Cas9 mRNA activity and reduce immunogenicity without HPLC purification. Mol. Ther. Nucleic Acids 12, 530–542. doi:10.1016/j.omtn.2018.06.010
Vetrini, F., and Ng, P. (2010). Gene therapy with helper-dependent adenoviral vectors: Current advances and future perspectives. Viruses 2 (9), 1886–1917. doi:10.3390/v2091886
von Levetzow, G., Spanholtz, J., Beckmann, J., Fischer, J., Kögler, G., Wernet, P., et al. (2006). Nucleofection, an efficient nonviral method to transfer genes into human hematopoietic stem and progenitor cells. Stem Cells Dev. 15 (2), 278–285. doi:10.1089/scd.2006.15.278
Vuelta, E., Ordoñez, J. L., Alonso-Pérez, V., Méndez, L., Hernández-Carabias, P., Saldaña, R., et al. (2020). CRISPR/Cas9 technology abolishes the BCR/ABL1 oncogene in chronic myeloid leukemia and restores normal hematopoiesis. bioRxiv, 237610. 2020.2008.2005. doi:10.1101/2020.08.05.237610
Wang, Q., Zhong, X., Li, Q., Su, J., Liu, Y., Mo, L., et al. (2020). CRISPR-Cas9-Mediated in vivo gene integration at the albumin locus recovers hemostasis in neonatal and adult hemophilia B mice. Mol. Ther. Methods Clin. Dev. 18, 520–531. doi:10.1016/j.omtm.2020.06.025
Wilbie, D., Walther, J., and Mastrobattista, E. (2019). Delivery aspects of CRISPR/Cas for in vivo genome editing. Acc. Chem. Res. 52 (6), 1555–1564. doi:10.1021/acs.accounts.9b00106
Wu, C. M., Roth, T. L., Baglaenko, Y., Ferri, D. M., Brauer, P., Zuniga-Pflucker, J. C., et al. (2018). Genetic engineering in primary human B cells with CRISPR-Cas9 ribonucleoproteins. J. Immunol. Methods 457, 33–40. doi:10.1016/j.jim.2018.03.009
Xie, F., Ye, L., Chang, J. C., Beyer, A. I., Wang, J., Muench, M. O., et al. (2014). Seamless gene correction of β-thalassemia mutations in patient-specific iPSCs using CRISPR/Cas9 and piggyBac. Genome Res. 24 (9), 1526–1533. doi:10.1101/gr.173427.114
Xu, C. L., Ruan, M. Z. C., Mahajan, V. B., and Tsang, S. H. (2019). Viral delivery systems for CRISPR. Viruses 11 (1), 28. doi:10.3390/v11010028
Xu, X., Hulshoff, M. S., Tan, X., Zeisberg, M., and Zeisberg, E. M. (2020). CRISPR/Cas derivatives as novel gene modulating tools: Possibilities and in vivo applications. Int. J. Mol. Sci. 21 (9), 3038. doi:10.3390/ijms21093038
Yang, Y., Xu, J., Ge, S., and Lai, L. (2021). CRISPR/Cas: Advances, limitations, and applications for precision cancer research. Front. Med. (Lausanne) 8, 649896. doi:10.3389/fmed.2021.649896
Yen, J., Fiorino, M., Liu, Y., Paula, S., Clarkson, S., Quinn, L., et al. (2018). Triamf: A new method for delivery of Cas9 ribonucleoprotein complex to human hematopoietic stem cells. Sci. Rep. 8 (1), 16304. doi:10.1038/s41598-018-34601-6
Yin, C., Zhang, T., Qu, X., Zhang, Y., Putatunda, R., Xiao, X., et al. (2017). In vivo excision of HIV-1 provirus by saCas9 and multiplex single-guide RNAs in animal models. Mol. Ther. 25 (5), 1168–1186. doi:10.1016/j.ymthe.2017.03.012
Yip, B. H. (2020). Recent advances in CRISPR/Cas9 delivery strategies. Biomolecules 10 (6), 839. doi:10.3390/biom10060839
Zhang, H., and McCarty, N. (2016). CRISPR-Cas9 technology and its application in haematological disorders. Br. J. Haematol. 175 (2), 208–225. doi:10.1111/bjh.14297
Zhang, J. P., Cheng, X. X., Zhao, M., Li, G. H., Xu, J., Zhang, F., et al. (2019). Curing hemophilia A by NHEJ-mediated ectopic F8 insertion in the mouse. Genome Biol. 20 (1), 276. doi:10.1186/s13059-019-1907-9
Zhang, S., Shen, J., Li, D., and Cheng, Y. (2021). Strategies in the delivery of Cas9 ribonucleoprotein for CRISPR/Cas9 genome editing. Theranostics 11 (2), 614–648. doi:10.7150/thno.47007
Zhang, X., Li, T., Ou, J., Huang, J., and Liang, P. (2022). Homology-based repair induced by CRISPR-Cas nucleases in mammalian embryo genome editing. Protein Cell. 13 (5), 316–335. doi:10.1007/s13238-021-00838-7
Zhao, Z., Li, C., Tong, F., Deng, J., Huang, G., and Sang, Y. (2021). Review of applications of CRISPR-Cas9 gene-editing technology in cancer research. Biol. Proced. Online 23 (1), 14. doi:10.1186/s12575-021-00151-x
Keywords: CRISPR/Cas, blood disorder, physical delivery, viral vectors, non-viral vectors
Citation: Mohammadian Gol T, Ureña-Bailén G, Hou Y, Sinn R, Antony JS, Handgretinger R and Mezger M (2023) CRISPR medicine for blood disorders: Progress and challenges in delivery. Front. Genome Ed. 4:1037290. doi: 10.3389/fgeed.2022.1037290
Received: 05 September 2022; Accepted: 22 December 2022;
Published: 06 January 2023.
Edited by:
Eleni Papanikolaou, Miltenyi Biotec, GermanyReviewed by:
Tatjana Cornu, University of Freiburg Medical Center, GermanyCopyright © 2023 Mohammadian Gol, Ureña-Bailén, Hou, Sinn, Antony, Handgretinger and Mezger. This is an open-access article distributed under the terms of the Creative Commons Attribution License (CC BY). The use, distribution or reproduction in other forums is permitted, provided the original author(s) and the copyright owner(s) are credited and that the original publication in this journal is cited, in accordance with accepted academic practice. No use, distribution or reproduction is permitted which does not comply with these terms.
*Correspondence: Markus Mezger, bWFya3VzLm1lemdlckBtZWQudW5pLXR1ZWJpbmdlbi5kZQ==