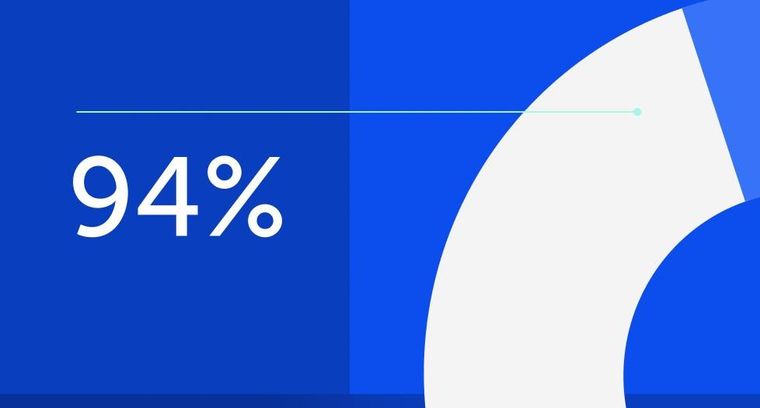
94% of researchers rate our articles as excellent or good
Learn more about the work of our research integrity team to safeguard the quality of each article we publish.
Find out more
REVIEW article
Front. Genet., 26 July 2024
Sec. Human and Medical Genomics
Volume 15 - 2024 | https://doi.org/10.3389/fgene.2024.1440430
This article is part of the Research TopicGene delivery strategies for disease treatmentView all 4 articles
Triple-negative breast cancer (TNBC) is the most severe form of breast cancer, characterized by the loss of estrogen, progesterone, and human epidermal growth factor receptors. It is caused by various genetic and epigenetic factors, resulting in poor prognosis. Epigenetic changes, such as DNA methylation and histone modification, are the leading mechanisms responsible for TNBC progression and metastasis. This review comprehensively covers the various subtypes of TNBC and their epigenetic causes. In addition, the genetic association of TNBC with all significant genes and signaling pathways linked to the progression of this form of cancer has been enlisted. Furthermore, the possible uses of natural compounds through different mechanistic pathways have also been discussed in detail for the successful management of TNBC.
Cancer is characterized by an uncontrolled growth of the body’s cells (Kaleem et al., 2022; Chunarkar-patil et al., 2024). Several genetic and epigenetic factors have been suggested to be responsible for the development of various types of cancer, resulting in the switch-off/on the tumor suppressor genes. Epigenetics involves studying the mechanisms that can change gene expression without affecting DNA sequence (Füllgrabe et al., 2011; Reddy Dachani et al., 2024). Several epigenetic modifications, such as histone acetylation and DNA methylation, are responsible for cancer development (Kaleem et al., 2021a; Kaleem et al., 2021b).
Breast cancer (BC) is the most common type of cancer among women globally (Michniak-Kohn et al., 2022; Kaleem et al., 2024). It is a highly complex form of cancer with distinct biological subtypes and several well-known prognostic markers of therapeutic importance (Hobbs et al., 2022). As per the report of 2020, nearly 2.3 million (11.7%) new cancer cases were diagnosed, and 6,84,996 (6.9%) deaths occurred due to breast cancer (Kaleem et al., 2022), and the incidence was anticipated to increase about 3 million new cases and one million mortalities by 2040 (Arnold et al., 2022). Several factors, such as sex, family history, genetic mutations, hormonal imbalance, diethylstilbestrol intake, obesity, alcohol consumption, smoking, vitamin D deficiency, radiation exposure, processed meat intake, etc., can cause breast cancer (Almansour, 2022). However, the prognosis and clinical manifestations vary considerably amongst patients. Notably, BC consists of human epidermal growth factor receptor 2 (HER2) overexpression, luminal A, luminal B, and subtypes of triple-negative breast cancer (TNBC) (Zhu et al., 2022).
TNBC is characterized by the loss of the cell surface’s progesterone, estrogen, and HER2 receptors. Among breast cancer, the incidence of TNBC is approximately 15%–20% and is more commonly reported in patients below 40 years (Bilani et al., 2020; Łukasiewicz et al., 2021). It has been noted to have higher death rates than other subcategories of BC. There is a significant decline in the lifespan of TNBC patients, approximately 45% develop metastatic tumors in the brain or other parts of the body (Schmid et al., 2018). Moreover, around 25% recovery percentage in TNBC patients has been reported by numerous studies (Zhu et al., 2022).
Although the scientific community has put significant efforts into discovering/developing potential moieties that can effectively mitigate breast cancer cell proliferation, their therapeutic efficacy is still very low (Hsu et al., 2022). The main reason is poor diagnosis since genomic changes in different cancers change their type, requiring specific treatments (Li Y. Z. et al., 2022). TNBC is a highly aggressive form of breast cancer, often results in the development of drug resistance via the expression of different efflux proteins on the cell surface, which decreases the treatment efficiency (Nedeljković and Damjanović, 2019). Along with the genetic changes, DNA methylation and histone modifications are epigenetic alterations that result in rapid development and metastasis of TNBC (Manoochehri et al., 2021). This article provide a detailed description of the genes linked to TNBC progression, their epigenetic modification, and the mechanism of action of natural compounds through the epigenetic modulation for TNBC treatment.
Six subtypes of TNBC were identified from the analysis of about 587 TNBC genes, including the high-expression growth factor pathway and myoepithelial markers basal-like 1 (BL1), basal-like 2 (BL2), mesenchymal-like (MES), mesenchymal/stem-like (MSL), immunomodulatory (IM), and luminal androgen receptor (LAR) (Yin et al., 2020). Recently, one study reported the molecular typing of TNBC which is the most popular and commonly referred as “Fudan typing” (Liu et al., 2016; Jiang et al., 2019). Using multi-omics sequencing, a research group categorized 465 TNBC specimens into four groups. Specifically, the MES type has enriched growth factor signaling pathways, the LAR type signals through androgen receptors, the IM type overexpresses immune cell and cytokine signaling genes, and the BL type uses reduced immune response genes to activate DNA repair and the cell cycle (Jiang et al., 2019).
BL tumors have high expression of proliferative genes and express ATR/BRCA pathways, which are involved in DNA damage response and accelerate cell proliferation (Ensenyat-Mendez et al., 2021). Since there is a lack of a repair system, polyADP-ribose polymerase 1 (PARP) inhibitors and platinum coordination complexes are widely accepted as treatment regimens for basal-like subtypes (Barchiesi et al., 2021). One study reported significant improvement in pathologic complete response (pCR) (58.7%) when carboplatin was used as an adjuvant compared to the non-carboplatin group (Von et al., 2014).
Mesenchymal tumors have higher levels of genomic instability, mutation burden, and copy number alterations than other subtypes. They are also associated with a lower number of immune cells and PDL1 expression, which indicate that these tumors have developed immune-evading mechanisms and contribute to their resistance to immunotherapy (Akhand et al., 2021).
Mesenchymal tumors are characterized by the alteration in growth factor signaling pathways and epithelial-to-mesenchymal transition (EMT). Due to phosphoinositide-3-kinase, catalytic, alpha polypeptide (PIK3CA) mutations or deficiency of phosphatase and tensin-homolog (PTEN), the mesenchymal subtype expresses the phosphoinositide 3-kinase/protein kinase B (PI3K/Akt) pathway and is sensitive to mammalian target of rapamycin (mTOR) inhibitors like NVP-BEZ235 (Zhao et al., 2020). EMT pathways can also be targeted by inhibiting the fibroblast growth factor receptor (Asleh et al., 2022).
LAR subtypes carry higher rates of ERBB2 mutations than other TNBC subtypes, despite being clinically classified as negative for HER2 genes (Lehmann et al., 2021). Estrogen/androgen metabolism pathways are differentially expressed in the luminal AR subtype. Therefore, inhibition of the luminal androgen receptor is a widely accepted treatment approach for this tumor subtype. Since PIK3CA mutations have also been reported in this tumor subtype, PI3K inhibitors can also be an effective therapeutic alternative (Ahn et al., 2016; Zagami and Carey, 2022).
The IM subtype is characterized by the abundant expression of genes associated with immune cell processes. Genes and pathways of signal transduction linked to immune cells, include the NK cell, B cell receptor signaling, dendritic cell, T cell receptor signaling, interleukin (IL)-12/IL-7, and Th1/Th2 pathway, are substantially activated in the IM subtype. Patients with breast cancer of the IM subtype are advised to be treated with PD1, PDL1, CTLA-4, along with other immune checkpoint inhibiting agents (Yin et al., 2020). The medullary cancer of the breast and the IM subtype are nearly identical (Yin et al., 2020). The IM subtype’s immune signaling genes are significantly similar to the gene profile of medullary breast cancer, a unusual but histologically different kind of TNBC with high-grade histology linked to positive prognosis (Hubalek et al., 2017).
The addition of a methyl group at the 5th position of the pyrimidine ring to form 5-methylcytosine (5-mC) is known as DNA methylation, which generally occurs at genomic CpG sites (Kaleem et al., 2021b). DNA methylation is a heritable epigenetic phenomenon in normal mammalian growth that regulates gene activity (Maenohara et al., 2017; Zeng and Chen, 2019). Nevertheless, the abnormal methylation of CpG islands is a characteristic of numerous malignancies in the promoter domains of genes, which are involved in the upregulation of oncogenes and downregulation of tumor suppressor genes (Kagara et al., 2012). One of the major causes of disruptions in the cell cycle is epigenetics-associated DNA methylation. Under normal conditions, CpG islands that are short DNA sequences enriched with cytosine and guanosine remain in a non-methylated state. A family of enzymes known as DNA methyltransferases (DNMTs) facilitates the transfer of the methyl moiety from donor S-adenosylmethionine (SAM) to DNA. Mammalian DNMTs are mainly involved in the maintenance and de novo methylation. During embryogenesis, DNMT3A, and DNMT3B play a key role in the maintenance of de novo methylation, while, during DNA replication, maintaining the methylation patterns from parent to offspring (Mensah et al., 2021). DNA methyltransferases (DNMT1, 3A, and 3B) mediates DNA methylation modifications, leading to gene expression alterations. DNA methylation pattern overlaps between TNBC and other breast cancers due to similarity in the involved genes (Kaleem et al., 2021b; Uddin and Fandy, 2021; Zolota et al., 2021). The CpG islands undergo hypermethylation in TNBC (Stirzaker et al., 2015; Quintas-Granados et al., 2021). It has been reported that RB, CD44, p73, MGMT, and CDKN2B genes remain in methylated state, while MLH1, MSH6, MSH2, MSH3, DLC1, CACNA1A, GSTP1, CACNA1G, ID4, Twist1, and PMS2 genes remain in non-methylated state in TNBC. The two-pore domain potassium channels are differentially expressed in TNBC and the over-expression of KCNK5 and KCNK9 genes takes place due to hypomethylation of CpG island loci (Dookeran et al., 2017). In the miR-200b promoter region, MYC incorporates DNMT3A, which results in hypermethylation of CpG islands, followed by suppression of miR-200b, promoting mammosphere formation and EMT in TNBC cells (Pang et al., 2018). Some studies suggested that DNA methylation suppresses CREB3L1 and BRMS1 genes, which suppress metastasis (Ward et al., 2016; Xia et al., 2017).
Despite widespread hypomethylation, hypermethylation of specific genes has also been linked to TNBC (Sandhu et al., 2012). In TNBC, the methylation of several genes associated with the DNA damage response, including BRCA1 and 14-3-3σ (also referred to as HME1) has been reported (Yu et al., 2019). Reduced expression of BRCA1 mRNA is linked to methylation of the BRCA1 promoter. Investigations using cancer cell lines and tissues as models have shown that the decrease in gene expression is mediated by CpG island methylation of the 14-3-3σ gene. Moreover, methylation has also been observed in TNBC for cell-to-cell adhesion molecules, including E-cadherin, the inactivation of which could facilitate metastasis (Kashiwagi et al., 2010; Yu et al., 2019).
After translation, the histone proteins undergo modifications, such as acetylation, ubiquitination, methylation, phosphorylation, and sumoylation at the N-terminal tails, which alters the structure of chromatin and gene transcription (Ramazi et al., 2020). Various levels of histone acetylation and methylation mark subclasses of breast tumors. The basal-like and HER2-positive tumors are the consequences of lysine acetylation (H3K18ac, H3K9ac, and H4K12ac) and methylation of arginine (H4R3me2) and lysine (H4K20me3, and H3K4me2) (Ramazi et al., 2020). In addition, the increased expression of H3K4ac and H3K4me3 has been suggested to modulate the EMT signaling pathway (Lin and Wu, 2020). The Brahma (BRM) expression levels are noted to be decreased in higher grade human breast cancer tissues than those of lower grade, as well as in triple-negative breast cancer cells (MDA-231), results in the loss of claudin group (Yang et al., 2019). A significant decline in the mRNA levels of eight HMTs, including PRDM6, SMYD3, EZH1, SETD7, SETD3, EHMT1, SETD1B, and PRDM4 and expression of PRDM15, SMYD2, SUV39H1, EZH2, SETD6, EHMT2/G9a, PRDM13, SMYD5, WHSC1, SUV39H2, SETDB, and SETD8 HMTs in basal-like tumor has been reported (Liu et al., 2015).
One study demonstrated a rise in H3K4ac and H3K4me3 markers in the metastatic MDA-MB-231 cell line compared to the mature MCF7 luminal cell line, emphasizing the variations amongst breast cancer subcategories (Messier et al., 2016). H3K4ac was believed to be involved in ER signaling in MCF-7 cells, while in the TNBC cell line (MDA-MB-231), H3K4ac and H3K4me3 are the EMT-linked genes that facilitate EMT-mediated signaling pathways (Zolota et al., 2021).
Histone deacetylases (HDACs) are generally used for various epigenetic treatments and regulate the tumor suppressor genes by removing acetyl groups from histones. Several solid and hematological malignancies have shown positive results, utilizing HDAC inhibitors (HDACi), inhibiting tumor growth and triggering apoptosis through various mechanisms (Mottamal et al., 2015). One study demonstrated that suberoyl anilide hydroxamic acid (SAHA, vorinostat) and sodium butyrate (NaB), both HDACIs, exhibit significant reductions in TNBC cell growth, induce cell cycle arrest at the G0/G1 phase, and trigger apoptosis. Furthermore, the phosphorylation, protein and mRNA levels of mutant p53 (mtp53) are reduced in TNBC cells by SAHA and NaB treatment (Wang et al., 2016). Panabinostat, another HDACi, has shown to induce hyperacetylation of histones H3 and H4 in TNBC cell lines, including MDA-MB-157, MDA-MB-231, MDA-MB-468, and BT-549, while reducing survival, proliferation, and apoptosis induction. Panabinostat has also been observed to reduce the growth of tumors in xenograft (MDA-MB-231 and BT549 cells) mouse models (Tate et al., 2012). Although knockdown of PTEN cells is resistant to these combinations, vorinostat has been noted to improve the growth inhibitory capacity of PARP inhibitor olaparib in TNBC cells (Min et al., 2015; Temian et al., 2018).
MicroRNA (miRNAs), 20–22 nucleotides long, are small single-stranded non-coding RNAs that control gene expression through post-translational silencing of the target genes. miRNAs have been suggested as crucial regulators of carcinogenesis and target different aspects of the TNBC microenvironment with increased efficacy (Qattan, 2020). MicroRNA expression can be monitored through various mechanisms, such as single nucleotide polymorphisms (SNPs), chromosomal abnormalities, and mutations in the primary transcripts (Lu et al., 2005). The significant decrease in the miR-200b/a/c expression through blocking PKC and targeting BMI1 proteins, (SIP1 and ZEB1/2), leads to down-regulation of EMT and suppresses TNBC cell proliferation (Piasecka et al., 2018). One study reported that the up-regulation of miR-200b suppresses the migration of MDA-MB-231 TNBC cells (Piperigkou et al., 2020). In addition, miR-200 and miR-205 suppress EMT and cancer metastasis through the hypermethylation of the ZEB2 and ZEB1 promoter regions (E-cadherin transcriptional repressors). By targeting the RabGTPase family 27a and MMP 11, MiR-145 suppresses cell proliferation, decreases cell-cell adhesion, and facilitates apoptosis (Tang et al., 2016; Piperigkou and Karamanos, 2019). Moreover, the overexpression of miR-155 in TNBC provides a protective effect and enhances patient survival through DNA-damaging pathways. Phosphatase and Tensin homolog (PTEN) and pro-apoptotic phosphatase could be miR-21 targets (Gasparini et al., 2014). Among miRs that are markedly elevated in TNBC is miR-27a/b and scientific studies have linked its high expression to a poor overall patient survival rate. On the other hand, the low expression of miR-30a has been linked to higher lymph node metastases and histological grade in TNBC patients. In addition, miR-30a has also been noted to inhibit TNBC cells’ epithelial-mesenchymal transition (Wang X. et al., 2018). A poor prognosis of TNBC has been linked to miR-210 elevated levels and low expression of miR-210 increases disease-free survival and overall survival (Wu, 2020; Santana et al., 2022). TNBC cell invasion and migration are facilitated by miR-454, which subsequently promotes TNBC cell proliferation. By controlling the levels of caspase 3/7 and the anti-apoptotic protein BCL-2, miR-454 suppresses radiation-induced apoptosis in TNBC cells. The elevated levels of miR-454 have also been shown to alter the expression of pAKT and PTEN in TNBC cells (Li et al., 2017). There is a poorer chance of survival for patients with elevated miR-146a levels compared to those having low expression levels. Moreover, a poorer prognosis is associated with elevated levels of miR-146a in TNBC, indicating that the miR-146 expression is an independent variable affecting patient prognosis (Santana et al., 2022).
Long non-coding RNAs (lncRNAs) are non-protein-coding transcripts and <200 nucleotides. Several studies reported dysregulated lncRNA expression through a variety of mechanisms in numerous kinds of tumors, such as ovarian, breast, hepatocellular carcinoma, and others (Tripathi et al., 2018; Wong et al., 2018). Furthermore, several lncRNAs have also been reported to play a vital role in different biological processes, such as invasion, differentiation, apoptosis, proliferation, and cell development. LncRNAs have been suggested to interfere with miRNA-mediated gene regulation by acting as miRNA “sponges” and competing with miRNA-targeted mRNAs. Molecular studies have demonstrated that the primary molecular mechanism of lncRNA biological function is competing endogenous RNA processes and network formation, which sequesters miRNAs while preventing post-translational regulation of protein-coding counterparts. Some lncRNAs associate with mRNAs to enhance and protect against the damaging effects of miRNA (Zhang W. et al., 2021). Growth arrest and DNA-damage-inducible alpha is regulated by lncRNA TARID (TCF21 antisense RNA-induced demethylation), which in turn stimulates tumor suppressor transcription factor 21 (TCF21) through promoter demethylation (Arab et al., 2014; Aram et al., 2017). HOX transcript antisense RNA, a lncRNA binds to polycomb repressive complex 2, causes the trimethylation of H3 lysine 27 (H3K27me3) of the homeobox D cluster locus, thereby promoting epigenetic silencing (Fang and Fullwood, 2016). To protect from antitumor cell metastasis, the natural antisense transcript (NAT) of zinc finger E-box binding homeobox 2 ZEB2 binds to an intronic 5′ splice site that prevents ZEB2 mRNA from interweaving (Huarte, 2015). Some lncRNAs function as tumor suppressors, whereas others function as oncogenes (Qi and Du, 2013). In hepatocellular carcinoma, the metastasis and epithelial-to-mesenchymal transition (EMT) are mediated by TGF-β activated lncRNA (lncRNA-ATB). NF-κB-with lncRNA interconnection functions as a scaffold to inhibit phosphorylation of IκB, which activates the tumorigenic NF-κB pathway (Peng et al., 2017). The suppressor of KAI1 in breast cancer inhibits the KAI1/CD82 metastasis suppressor gene and is known as metastasis-inducing lncRNA, which is upregulated in TNBC (Arab et al., 2014; Aram et al., 2017). In vitro and in vivo studies revealed suppression of tumor proliferation in the LINC01638 knockdown model since LINC01638 facilitates EMT via the Twist1 expression (Luo et al., 2018). Moreover, LncRNA, such as LUCAT1, PAPAS, and HCP, have shown to facilitate TNBC by altering miR-34a, miR-219a-5p, and miR-5702 miRNAs (Wang L. et al., 2019; Kong et al., 2019; Zhan et al., 2020). Apoptosis and cell proliferation suppression have been enhanced with lncRNA rhabdomyosarcoma 2-associated transcript (RMST) (Wang L. et al., 2018). The overexpression of LncRNA NEF inhibit migration and invasion of TNBC cells (Song et al., 2019). Similarly, LncRNA XIST block EMT via modulation of miR-454 in TNBC cells in vitro and in vivo models (Li et al., 2020; Zolota et al., 2021), as depicted in Figure 1. In the following section, we have covered different natural compounds that have shown encouraging results for the treatment of TNBC and highlight their potential through various mechanistic pathway targeting.
Epigallocatechin-3-gallate (EGCG), a green tea-derived polyphenol, exhibits anti-inflammatory, antioxidant, and antitumor activities (Alserihi et al., 2021; Mokra et al., 2022; Alserihi et al., 2023; Suhail et al., 2023). It inhibits the catechol-o-methyltransferase and DNA methyltransferase, thereby reducing the proliferation of the TNBC cells. It also causes the re-expression of the RAR-B, MGMT, and CDKN2A genes (Schröder et al., 2020). EGCG has been stated to upregulate the CASP1, CASP3, and CASP4 levels and decrease TP53 and FAS expression, repressing TNBC cell growth and proliferation (Bimonte et al., 2020). EGCG enhances the chemotherapeutic effect through β-catenin, EGFR, PI3K/Akt, MAPK, and NF-κβ pathways (Bimonte et al., 2020). Anti-apoptosis genes, such as induced myeloid leukemia cell differentiation protein (MCL1) and insulin-like growth factor-1 receptor (IGF1R) are reported to be downregulated and the upregulation of Receptor-interacting protein kinase 2 (RIPK2), Bcl2-associated athanogene 3 (BAG3), and X-linked inhibitor of apoptosis protein (XIAP) in response of EGCG treatment has also been noted (Ensenyat-Mendez et al., 2021). Expression of Golgi membrane protein 1 (GOLM1), metastasis promoter protein, has also been suppressed by EGCG in MDA-MB-231 cells through the HGF/HGFR/AKT/GSK-3/β-catenin/c-Myc signaling pathway (Xie et al., 2021).
Thymoquinone (2-methyl-5-isopropyl-1,4-benzoquinone) is isolated from black cumin (Nigella sativa) seeds. It has various pharmacological properties such as antioxidant, anti-inflammatory, anti-diabetic, and immunomodulatory effects (Kabil et al., 2018). Several investigations have shown that TQ exhibits therapeutic potential against different categories of cancer via a variety of mechanisms, such as the production of reactive oxygen species, induction of apoptosis, cell cycle arrest, suppression of angiogenesis, and cell proliferation (Banerjee et al., 2010; Abdullah et al., 2021; Kaleem et al., 2024). It can modulate numerous signaling targets/pathways involved in cancer, such as STAT3, PPAR-γ, NF-κB, and p53. TQ reduces the level of the eEF-2K protein and important downstream targets such as Src/FAK and Akt which are therapeutically significant. Additionally, TQ activates the tumor suppressor miR-603 in association with suppression of NF-kB, resulting in a notable reduction in TNBC cell migration, invasion, proliferation, and colony formation (Gomathinayagam et al., 2020). Administration of TQ (20 and 100 mg/kg) has shown a marked reduction in the growth of MDA-MB-231 tumors and prevented eEF-2K activity (Kabil et al., 2018). The anticancer potential of TQ is manifested through DNA methylation (DNMT1 dependent) in leukemia cells (Pang et al., 2017). The treatment of TQ results in the down-regulation of really interesting new gene (RING) finger domains 1 (UHRF1), HDAC1/4/9, KDM1B, G9A, DNMT1/3A/3B, and KMT2A/B/C/D/E, and ubiquitin-like containing plant homeodomain (PHD) (Ashraf et al., 2017; Qadi et al., 2019; Abdullah et al., 2021). One study observed that administration of TQ decreases the mRNA expression of TWIST1 (EMT-promoting transcription factor) in MDA-MB-435 cell lines (Khan et al., 2015).
Curcumin (1,7-bis (4-hydroxy-3-methoxyphenyl)-1,6-heptadiene-3,5-dione), also known as diferuloylmethane is a polyphenolic compound present in the rhizomes of Curcuma longa. In Asian nations, curcumin has been conventionally used as a medical herb because of their anti-inflammatory, antimutagenic, antimicrobial, antioxidant, and anticancer properties (Hewlings and Kalman, 2017; Ahmad et al., 2024). Scientific studies have reported the anticancer effects of curcumin against TNBC cells via EGFR tyrosine kinase, PI3K-Akt, Foxo, JAK-STAT, and HIF-1 signaling pathways. It also modulates PTGS2, EP300, STAT3, TNF-α, GSK3B, PPARG, AKT1, EGFR, NFE2L2, and MMP-9 genes expression and shows anti-proliferative activity. It has been reported to prevent the invasion and proliferation of the MDA-MB-231 cells and induce apoptosis (Deng et al., 2022). A study revealed that curcumin blocks the invasion, proliferation, migration, and mammosphere formation in TNBC cells (Li M. et al., 2022).
Curcumin has also been reported to successfully inhibit TNBC growth by preventing the protein salt-induced kinase-3 (SIK3) expression in tumor-derived xenograft mice from patients (TNBC-PDX). Tumor tissues exhibit a notably elevated SIK3 mRNA expression (73.25 times, n = 183) compared to the neighboring normal tissues. Curcumin (>25 μM) could prevent SIK3-mediated cyclin D overexpression, which arrests the G1/S cell cycle and suppresses the growth of TNBC (MDA-MB-231) cancer cells. During EMT, there is a correlation between the overexpression of SIK3 and higher levels of mesenchymal markers, such as, Twist, MMP3, α-SMA, and Vimentin. Curcumin substantially attenuates tumor migration by inhibiting SIK3-mediated EMT (Cheng et al., 2021).
Calycosin (CA) is a flavonoid (7,3ʹ-dihydroxy-4ʹ-methoxyisoflavone, C16H12O5) phytoestrogen obtained from Astragalus membranaceus and has shown anticancer activity especially against TNBC cells. It is also found in plants, such as Hedysarum Polybotrys Hand.-Mazz., Puerarialobata (Wild.) Ohwi, Pterocarpuserinaceus Poir., Caraganaacanthophylla Kom., Sophoraflavescens Aiton, Glycyrrhizauralensis Fisch, and Millettialaurentii De Wild (Deng et al., 2021). In the ER- and PR-negative subtype, higher expression of ER-α30 has been reported in BT-549 and MDA-MB-231 TNBC cell lines. ER-α30 expression has been noted to be blocked by CA along with the inhibition of TNBC metastasis (Li et al., 2023). Calycosin reduces the migration, invasion, and proliferation of breast tumor cell lines (T47D and MCF-7) in a time- and dose-dependent manner. In addition, basic leucine zipper ATF-like transcription factor (BATF) elevate TGFβ1 mRNA and protein levels, stimulating breast cancer cell migration and invasiveness. Treatment with CA inhibits EMT of breast cancer cells via decreasing levels of TGFβ1 and BATF while up-regulating the expression of E-cadherin and decreasing the expression of vimentin, CD147, MMP-2/9, and N-cadherin. Furthermore, CA increases invasiveness and migration when incubated with TGFβ1, while knockdown of TGFβ1 decreases these properties in BATF-overexpressing breast cancer cells. These findings indicate that CA prevent EMT and the growth of breast cancer cells via reducing BATF/TGFβ1 signaling (Zhang Z. et al., 2021). In conclusion, CA prevents the growth and metastasis of TNBC by targeting the ER-α splice variant ER-α30 and reducing its expression in cancer tissues (Li et al., 2023).
Mangois obtained from Mangifera indica L., family Anacardiaceae, a key South and Southeast Asia (Kumar et al., 2021). The mango leaf extracts have various biological properties, including lipid-lowering, liver protection, anti-diarrheal, antioxidant, anti-microbial, anti-cancer, and anti-diabetic effects (Kumar et al., 2021). Extracts from the leaves, seeds, and bark of M. indica have been used as pyruvate kinase M2 (PKM2) inhibitors. The extract of M. indica bark and seeds have shown growth inhibition through PKM2 blockage in the MDA-MB231 cells, indicating its potential for the management of TNBC (Rasul et al., 2021).
Cordycepin (CD) is a 3′-deoxyadenosine analog isolated from the caterpillar fungus Cordyceps militaris (Radhi et al., 2021). It is one of the major constituents found in Cordyceps mushrooms. The CD has various pharmacological properties, including antiviral, antitumor, immunity regulation, lipid-lowering, antiplatelet agglutination, antibacterial, and memory improvement (Liu X. C. et al., 2019). CD can inhibit the expression of SLUG and TWIST, thereby inactivating the EMT signaling pathway. In addition, cordycepin significantly downregulates the expressions of ZEB2, TWIST1, ZEB1, SLUG, and SNAIL, which are responsible for the inhibition of EMT in 4T1 and BT549 cells (Wei et al., 2022). The Hedgehog pathway has been suggested as the most prominent pathway in breast cancer. One study reported that cordycepin inhibits the Hedgehog pathway and suppresses growth and metastasis in the MDA-MB-231-induced mice xenograft model. The CD has been reported to markedly reduce the expression levels of the various components linked to apoptosis, metastasis, and growth in TNBC. Therefore, cordycepin could be a novel lead compound for TNBC therapy via Hedgehog pathway regulation (Wu et al., 2022).
Ailanthone (AIL) is a quassinoid extract isolated from Ailanthus altissima and a pentacyclic diterpene lactone compound belongs to Simaroubaceae family (Ding et al., 2020). It has shown antimalarial, anti-HIV, anti-microbial, anti-ulcer, anti-inflammatory, anti-allergic, anti-tuberculosis and antitumor properties (Wang R. et al., 2018; Ding et al., 2020). The bone marrow-derived macrophages (BMMs) are usually activated by the receptor activator of nuclear factor-κB nuclear ligand-receptor activator (RANKL), and MDA-MB-231 cell conditioned medium. These findings revealed a substantial reduction in the expression of osteoclast-forming genes and inhibition in infiltration and migration of MDA-MD-231 cells at low concentrations. AIL upregulates Forkhead box protein 3 (FOXP3) expressions while suppressing IL-1β and RANKL expression. AIL has also been reported to inhibit NF-κB, PI3K/protein kinase B (Akt), and MAPK signaling pathways (Wang et al., 2023).
Polyphyllin III is the main saponin obtained from Paris polyphylla rhizomes. The combination of polyphyllin III and sulphasalazine sensitizes TNBC cells by enhancing ferroptosis and lipid peroxidation. Ferroptosis through ACSL4-mediated lipid peroxidation is the primary mechanism of polyphyllin III anticancer activity (Zhou et al., 2021). One study reported a considerable decrease in invasion, colony formation ability, and cellular migration in MDA-MB-231 and MCF-7 cells in response to DS treatment. DS dramatically reduce the development of mammospheres in a dose-dependent manner during the process of differentiation triggering the manifestation of breast cancer stem-like cells. It also elevates p21 and p53 levels in breast cancer stem-like cells while decreasing cyclin B1 and cdc2 levels in MDA-MB-231 cells and cyclin D/E and CDK2/4 levels in MCF-7 cells. The administration of DS has also been noted to arrest cell cycle at G0/G1 and G2/M in MDA-MB-231 and MCF-7 cells, respectively. Additionally, DS has been noted to downregulate p-mTOR and p-Akt expression and increases p38 phosphorylation. These findings indicate that DS regulates the Akt/mTOR and p38 mitogen-activated protein kinase signaling pathways to inhibit the growth of breast cancer stem-like cells by inducing cell cycle arrest (Ock and Kim, 2021).
The lipophilic (Tanshinone I, IIA, IIB; cryptoTanshinone; other associated compounds) and hydrophilic (polyphenolic acids, danshensu, protocatechuic aldehyde, and protocatechuic acid) are the two most common active compounds obtained from the rhizomes and roots of Salvia miltiorrhiza (Labiatae family). Tanshinone IIA (Tan IIA), a diterpene quinone has been reported to have various therapeutic benefits against different diseases (Shang et al., 2012; Fang et al., 2021). It can target VEGF and mTOR/p70S6K/4E-BP1 signaling pathway and can inhibit VEGF and HIF-1α expression, which results in angiogenesis suppression (Li et al., 2015). Tan IIA prevents the total protein kinase C (PKC) activity, particularly PKC isoforms ζ and ε. In addition, Tan IIA prevents the Ras/MAPK pathway, which is tightly regulated by PKC signaling. Furthermore, Tan IIA trigger autophagy and cell cycle arrest and dramatically affect PI3K/Akt/mTOR signaling (Lv et al., 2018).
Soybean contain a high amount of isoflavonoids and belongs to the Papilionoideae subfamily. It is one of the major sources of oil and protein globally (Yue et al., 2023). Glyceollins are a group of phytoalexins, the main constituents in soybean and a secondary isoflavones metabolite (Lecomte et al., 2017). It is produced when exposed to fungi and in the presence of some abiotic elicitors, such as aluminum chloride, methyl jasmonate, and UV light (Park et al., 2017). Glyceollin I, II, and III are synthesized by the de novo pathway from the soy isoflavone daidzein. It is also reported to suppress the proinflammatory cytokines by inhibiting NF-κβ phosphorylation (Pham et al., 2019). Inhibition of the P13K/Akt/mTOR pathway and blocking of HIF-1 synthesis has been reported as one of the mechanism of its action for suppressing tumor growth in TNBC (Rhodes et al., 2012; Lee et al., 2015). Based on these studies, glyceollins seems to be promising therapeutic against triple-negative breast cancer.
Amentoflavone is a phenolic biflavonoid extracted from the leaves of Selaginella tamariscina (Imran et al., 2019). Anticancer activity of amentoflavone is brought through mediating nuclear factor kappa-B (NF-κβ), extracellular signal-regulated kinase (ERK), and PI3K/Akt pathway (Xiong et al., 2021). Amentoflavone has shown to block the Hedgehog signaling pathway by decreasing the expression of Gli1, OCT4, NANOG, and Smo. It also inhibits tumor sphere formation and controls the expression of different stem cell markers (CD24, ALDH1, and CD44) in a dose-dependent manner. The knockdown of Gli1 via siRNA leads to decreased formation of tumor sphere effectiveness in SUM159 breast cancer stem cells and dramatically affects ALDH1, OCT4, NANOG, and CD24/44 expression. These results suggest that amentoflavone suppresses the stemness of SUM159 breast cancer via targeting Hedgehog/Gli1 signaling, highlighting it as a promising molecule for the management of breast cancer stem cells (Bao et al., 2019).
Sulforaphane [1-isothiocyanato-4-(methylsulfinyl) butane] belongs to the isothiocyanate class of phytochemicals. Glucoraphanin, a glucosinolate precursor of SFN, is a glucosinolate found in cruciferous vegetables, such as broccoli, cabbage, cauliflower, and kale (Kim and Park, 2016). The MDA-MB-468 cells treated with SFN showed a reduction in mTOR and inhibition of Akt activity. SFN significantly suppresses the PI3K/Akt/mTOR pathway within cells where the downstream pathway is overactivated due to the loss of PTEN and EGFR upregulation. EGFR upregulation and PTEN reduction are common in TNBC and are linked to aggressive behavior and a poor prognosis of TNBC patients (Beg et al., 2015). SFN exerts growth-inhibitory activity against the MDA-MB-468 TNBC cell line, which shows the activation of the PI3K/Akt/mTOR signaling pathway and substantial rise in EGFR expression along with simultaneously suppression of phosphatase and tensin homolog (PTEN). These investigations indicated that SFN may serve as novel targeted therapeutic for treating TNBC, which is characterized by an overactive signaling pathway downstream of EGFR (Yasunaga et al., 2022).
Fisetin (3,3′,4′,7-tetrahydroxyflavone) is a bioactive flavonol molecule found in fruits and vegetables, such as strawberries, apples, persimmon, grapes, onion, cucumbers and has shown anticancer activity (Imran et al., 2020; Kubina et al., 2021). It is a potent blocker of matrix metalloproteinase (MMP)-1 activity, an enzyme essential for the remodeling and deterioration of the extracellular matrix that plays a significant role in cancer development. By inhibiting urokinase plasminogen activator, fisetin decrease angiogenesis, and inhibit tumor growth (Khan et al., 2013). It activates the caspase-3/8-dependent pathway through ERK1/2, Ca2+-dependent endonuclease, and caspase-3. Fisetin has also been reported to block mitogen-activated protein kinases (MAPK) and PI3K/Akt/mTOR signaling pathway (Chamcheu et al., 2019). These studies indicate the possible agonistic activity of fisetin on TNBC metastasis, which reversed EMT by blocking the PTEN-Akt-GSK-3β signaling pathway. These findings demonstrated that fisetin could be a novel, potentially effective therapy for metastatic breast cancer in TNBC patients (Li et al., 2018).
Ginsenoside is the active ingredient of American/Asin ginseng, isolated from rhizomes and roots of Panax ginseng/notoginseng of Araliaceae family. It has been reported to inhibit invasion and metastasis. The main constituents present in Panax herbs that exert an anticancer effect include PTS (20(S)-protopanaxatriol (PPT) saponins) and PDS (20(S)-protopanaxadiol (PPD) saponins) (Chen et al., 2016). PPD, one of the major active metabolites from P. ginseng, has been widely reported to possess pleiotropic anticancer activities against various cancers (Peng et al., 2019). A low concentration of PPD (<20 μM) has been reported to reduce the metastatic potential of SUM159 and MDA-MB-231 cells by directly inhibiting cell motility, invasiveness, and adhesion. In contrast, PPD at a dose of >30 μM has been observed to show decreased cell proliferation and induced apoptosis through cell cycle arrest at the G0/G1 phase. In addition, PPD therapy has also been observed to significantly reduce tumor growth in TNBC xenograft models. PPD induced the expression of tissue inhibitors of metalloproteinases (TIMPs) and reverts the EMT while reducing the expression and functioning of matrix metalloproteinases (MMPs). Through agonists or inhibitors of the EGFR and MAP kinases family, PPD therapy further confirmed the reduction in EGFR phosphorylation and down-regulation of ERK1/2, p38, and JNK signaling activation. These studies highlight PPD as a novel lead compound for the therapy of TNBC cells via the downregulation of the EGFR-mediated MAPK signaling pathway (Peng et al., 2019).
Quercetin is a flavonol mainly extracted from onion, which is present in the form of Que glycoside and has antioxidant properties (Salehi et al., 2020). Cherries, grapes, mangoes, citrus fruits, apples, plums, and buckwheat contain some quercetin (Kim et al., 2019; Wang et al., 2022). Quercetin can target insulin-like growth factor-1 receptor (IGF1R), Erk1/2 and inhibits the expression of EMT transcription factors Snail and Slug, thereby blocking the TNBC cell metastasis. It can activate IGF1R and its downstream kinases, such as Akt and Erk1/2, to prevent growth in human MDA-MB-231 breast cancer cells in a dose-dependent manner. It also inhibits the autocrine or paracrine loop of IGF1 signaling by increasing the production of IGF-binding protein-3 and decreasing the secretion of IGF1 in the culture medium of MDA-MB-231 cells. Quercetin has also been noted to inhibit the growth of MDA-MB-231 tumor xenografts mouse models through deactivation of IGF1R, upregulation of epithelial markers (keratins 18 and 19), and decreased levels of mesenchymal markers (Snail, Slug, fibronectin, and vimentin) (Chen et al., 2021).
Luteolin, (3′,4′,5,7-tetrahydroxyflavone), belongs to a group of naturally occurring compounds called flavonoids. It is present in high concentration in chrysanthemum flowers, onions, parsley, carrots, celery, broccoli, and bell peppers and have shown several biological activities, such as neuroprotective, antidiabetic, anticancer, antiallergic, anti-inflammatory, and anti-hypertensive properties (Çetinkaya and Baran, 2023). By inducing the expression of E-cadherin, causing shrinkage of the cytoskeleton, and downregulation of N-cadherin, vimentin, and snail, luteolin can reverse EMT. Its anticancer activity is through the modulation in the expression of ER stress-associated proteins, activation of reactive oxygen species (ROS), and mitochondrial dysfunction (Imran et al., 2019). Luteolin can block VEGF, which suppresses the β-catenin pathway, thus inhibiting proliferation and mammosphere formation in TNBC. Low luteolin dose significantly inhibits VEGF secretion in MDA-MB-231 (4175) LM2 cells (Cook, 2018). In vitro and in vivo experiments reported that luteolin induces suppression of SGK1, increases the translocation of FOXO3a into the nucleus, and subsequently transcribes BNIP3, which in turn enables BNIP3 to interact with autophagy and apoptosis proteins. These studies highlight that luteolin promotes apoptosis and autophagy in TNBC using the SGK1-FOXO3a-BNIP3 pathway, suggesting its potential against TNBC therapy (Wu et al., 2023).
Astragaliradix is an important tonifying herb, derived from the dried root of A. membranaceus (Fisch.) Bge. (family Leguminosae) and used for anemia, weakness, allergic reactions, etc. (Guo et al., 2019). Research has shown many biological properties of APS, including anti-inflammatory, antioxidant, immunoregulatory, and antitumor (Zhao et al., 2018). APS significantly inhibits the expression of PIK3CG, Akt, and Bcl-2 and substantially suppresses the activity, invasion, and apoptosis in MDA-MB-231 cells. Therefore, APS could be a promising therapeutic compound for the management of TNBC (Liu C. et al., 2019).
Physalisperuviana (golden berry) is an edible plant from the Solanaceae family. 4β-hydroxy withanolide E (4HW) is a natural compound isolated from an edible plant, Physalisperuviana. The 4bHWE withanolides are plant-derived C (28) steroidal lactones with potent anti-cancer properties (Chiu et al., 2013). Scientific studies have reported the anticancer potential of 4HW against prostate, oral, lung, and breast cancer (Tang et al., 2018; Hsieh et al., 2021). Similarly, withaferin A, [(4β,5β,6β,22R)-4,27-dihydroxy-5,6:22,26-diepoxyergosta-2,24-diene-1,26-dione] is a 28 carbon-containing withanolides with ergostane framework and a δ-lactone (Sultana et al., 2021). It is a steroidal lactone extracted from Withaniasomnifera L., also known as Ashwagandha (Dutta et al., 2019; Paul et al., 2021). WA has been reported to inhibit NF-κβ signaling, Hsp90 chaperone, angiogenesis, cell growth, and trigger apoptosis. On the other hand, 4-HW prevent the growth of cancer cells via inducing ROS-mediated DNA damage (You et al., 2014) and by binding directly to HSP90 molecules, which is comparable to the effects of WA on cancer cells (Wang et al., 2012). The withanolides 4-HW and WA induce apoptosis/necrosis and results in cell cycle arrest, significantly reducing TNBC cells’ viability. The PI3K/Akt pathway has been noted to be modulated in response to 4-HW and WA. These findings reveal that withanolides may have potential in the management of TNBC (Wang H. C. et al., 2019).
Fucoidan is isolated from marine sources, such as brown algae, sea cucumbers, Sargassum stenophyllum, and Fucus vesiculosus (Luthuli et al., 2019; Mansour et al., 2019). It exhibits potent biological activities, such as immunomodulatory, antivirus, antithrombotic, anticoagulant, and anti-tumor activity (Ale et al., 2011). It has been reported to downregulate the expression of vimentin and N-cadherin (mesenchymal markers) while upregulating E-cadherin and zonula occludens-1, which suppresses migration of MDA-MB-231 cells. Fucoidan treatment significantly reduce the HIF-1α protein expression through HIF1-α signaling pathway (Li et al., 2019). Therefore, fucoidan may be a promising therapeutic for treating TNBC through downregulating the HIF1-α signaling pathway.
Arctigenin (Atn) is a bioactive lignan obtained from the seeds of Arctium lappa L. (Batool et al., 2022). Cancerous inhibitor of protein phosphatase 2A (CIP2A) is a molecular marker in human cancer that inhibits the tumor-suppressor protein, protein phosphatase 2A (PP2A), and subsequently increases cancerous growth (Alzahrani et al., 2020). Arctigenin activates the PP2A and downregulates CIP2A, which phosphorylates and inhibits the Akt pathway, suppressing TNBC cell metastasis (Huang et al., 2017). It prevent MDA-MB-231 and BT549 cells from proliferation, migrating, and invasion and from undergoing EMT through inhibition of 4EBP1 (Luo et al., 2021). On the other hand, the upregulation of 4EBP1 might counteract the inhibitory effects of Atn on proliferation, migration, invasiveness, and EMT.
Propolis is a natural resinous mixture produced by honeybees from the substances collected from plants, buds, and exudates (Wagh, 2013). It contains caffeic, dihydrocinnamic, and p-coumaric acids. Treatment with p-coumaric acid and EGCG significantly reduce the cell viability of four triple-negative breast cancer cell lines (BT-20, BT-549, MDA-MB-231, and MDA-MB-436) through DNA methyltransferase targeting. Propolis and EGCG have been reported to demethylate the promoter region of RASSF1A in BT-549 cells highlighting their potential against TNBC (Assumpção et al., 2020).
Sanguinarine is a benzophenanthridine alkaloid, isoquinoline derivative, derived from rhizomes of the plant species Sanguinaria canadensis. This alkaloid is present as neutral alkanolamine and cationic iminium forms. Sanguinarine [13-methyl (1,3) benzodioxolo (5,6-c)-1,3-dioxolo (4,5) phenanthridinium] affects the Na+-K+-ATPase transmembrane protein, which leads to death of animal cells (Singh and Sharma, 2018). It is an excellent DNA and RNA intercalator where only the iminium ion binds (Basu and Kumar, 2016). SANG has been noted to inhibit Akt expression and upregulated the mRNA expression of Bcl-2, TNFRSF, and CASP in MDA-MB-468 cells, resulting in induced apoptosis caused by S-phase cell cycle arrest (Messeha et al., 2023). The mechanism underlying the antiangiogenic potential of SANG is TNF-α-mediated in, MDA-MB-231, and MDA-MB-468 cells. Furthermore, SANG block the NF-κB, ERK1/2 signaling pathway, and the CCL2 regulator gene IKBKE (Messeha et al., 2022).
Eupalinolide J is a sesquiterpene lactone, the main constituent in Eupatorium lindleyanum and popularly known as Yemazhui (Wu et al., 2020; Hu et al., 2023). It inhibits cancer cell growth by targeting the Akt and STAT3 signaling pathway (Lou et al., 2019). EJ suppresses the MMP-2/9 protein expression and inhibits metastasis. It inhibits TNBC cell proliferation by inducing cell apoptosis, hindering the mitochondrial membrane potential (MMP), and arresting the cell cycle.
Ursolic acid is a natural pentacyclic triterpene compound present in various fruits and vegetables. It is obtained from the leaves of several plants (rosemary, marjoram, lavender, thyme, and organum), fruits (apple fruit peel), flowers, and berries. UA has shown varied pharmacological activities, such as antioxidant anti-apoptotic, anti-carcinogenic, and anti-inflammatory properties. It inhibits cancer cells by inhibiting nuclear factor-kappa B signaling pathways (Seo et al., 2018). UA can dramatically suppress the growth, migration, invasion, and induction of cell cycle arrest and apoptosis in MDA-MB-231 and MDA-MB-468 cells in a concentration-dependent manner. The administration of UA has also been noted to result in a significant decrease in the tumor body and tumor weight of TNBC-xenograft mice, indicating its potential in vivo. One study observed that UA may inhibit the p53 signaling pathway, potentially reducing TNBC cell proliferation and decreasing the expression of PLK1 and CCNB1 proteins (Zhang Y. et al., 2021). These results provide compelling evidence that UA intervention with multi-target treatment effectively treats TNBC. Hence, UA could be a promising therapeutic for the treatment of TNBC.
The flavonoid morin, isolated from the Moraceace family, has shown numerous pharmacological properties, such as antioxidant, anti-inflammatory, and anti-cancer activity (Solairaja et al., 2021; Balaga et al., 2023). Morin has been reported to alter Kelch-like ECH-associated protein1/Nuclear erythroid-2-related factor (Keap1/Nrf2), MAPK pathways (Tian et al., 2017), and Wnt/β-catenin and induces apoptosis (Balaga et al., 2023). Inhibition of FOXM1 and attenuation of EGFR/STAT3 signaling pathways has been observed when a combination of morin and doxorubicin (Dox) is used. Phosphorylation of STAT3 and EGFR has also been reported to be suppressed in response to morin treatment (Maharjan et al., 2023). Based on these studies, the combination of morin with Dox may be a therapeutic strategy for treating TNBC (Maharjan et al., 2023).
7R-acetylmelodorinol (7R-AMDL) is a main compound isolated from the stem bark of Xylopia Pierrei (Chokchaisiri et al., 2017). It has been reported to significantly decrease the MDA-MB-231 cell viability, according to MTT and clonogenic experimentation. The treatment of 7R-AMDL (8 µM) leads to substantial activation of the caspase cascade, upregulation of death receptors and their ligands, activation of the intrinsic and extrinsic apoptosis pathways, and a reduction in the expression of Bcl-2-like 1 (BCL2L1/Bcl-xL). In addition, inhibition of cell migration and invasion by 7R-AMDL has been associated with reduced expression of metallopeptidase 9 (MMP-9) and activation of nuclear factor kappa B (NF-κB) in MDA-MB-231 cells (Thepmalee et al., 2023). These studies highlights that 7R-AMDL could be a promising therapeutic option for treating TNBC (Thepmalee et al., 2023). We have summarized this article in Table 1 and Figure 2.
Figure 2. Mechanism of action of selected natural compounds for the suppression of growth and migration of TNBC cells.
Given the above-mentioned experimental/laboratory results utilizing cell-based and xenograft models, the scientific community is highly optimistic about coming up with certain natural compounds or their combinations that could work effectively against TNBC in clinics. In addition, the effective utilization of some natural compounds as adjuvants could be a boost to our fight against this deadly and aggressive form of breast cancer. Table 2 enlists some natural compounds and/or their mixture in the different stages of clinical trials for the management of TNBC.
Table 2. Natural compounds/their combination currently in clinical trials for the management of triple-negative breast cancer.
TNBC continues to remain the most aggressive form of breast cancer with a poor prognosis due to several molecular subtypes and associated genetic modulations. Apart from genetics, epigenetics play a very crucial role in the etiology of TNBC. Epigenetic alterations like DNA methylation and histone modifications modify the gene expression, influence tumorigenesis and metastasis. Newer epigenetic information and knowledge gained through experimental work have resulted in the understanding of some unknown TNBC mechanisms. This article highlights several natural compounds. which have the potential to suppress the growth and metastasis of TNBC cells via interference and modulation of different signaling cascades, such as P13/Akt, PIK3CG/Bcl-2, EGFR/STAT3 and MAPK. However, further studies are required to utilize the potential of natural compounds either in combination or as an adjuvant in clinics.
MK: Investigation, Methodology, Visualization, Writing–original draft. MT: Investigation, Methodology, Visualization, Writing–original draft. ND: Investigation, Methodology, Visualization, Writing–original draft. AOA: Data curation, Investigation, Methodology, Visualization, Writing–original draft. WA: Data curation, Visualization, Writing–original draft. AmA: Investigation, Methodology, Visualization, Writing–original draft. MA: Supervision, Validation, Writing–review and editing. MAK: Conceptualization, Supervision, Validation, Writing–review and editing. ST: Conceptualization, Supervision, Writing–review and editing.
The author(s) declare that no financial support was received for the research, authorship, and/or publication of this article.
The authors greatly acknowledge the research facility provided by the King Fahd Medical Research Center, King Abdulaziz University, Jeddah, Saudi Arabia. The authors are also thankful to Dadasaheb Balpande College of Pharmacy, Nagpur, Maharashtra, India.
The authors declare that the research was conducted in the absence of any commercial or financial relationships that could be construed as a potential conflict of interest.
All claims expressed in this article are solely those of the authors and do not necessarily represent those of their affiliated organizations, or those of the publisher, the editors and the reviewers. Any product that may be evaluated in this article, or claim that may be made by its manufacturer, is not guaranteed or endorsed by the publisher.
Abdullah, O., Omran, Z., Hosawi, S., Hamiche, A., Bronner, C., and Alhosin, M. (2021). Thymoquinone is a multitarget single epidrug that inhibits the UHRF1 protein complex. Genes 12 (5), 622. doi:10.3390/genes12050622
Abelman, R. O., Spring, L., Niemierko, A., Abraham, E., Mcneice, M., Goff, J., et al. (2022). Sequential combination of sacituzumab govitecan and talazoparib in metastatic triple negative breast cancer (mTNBC): results from a phase II study. J. Clin. Oncol. 42 (16), 4039230. doi:10.1200/JCO.2024.42.16_suppl.11
Ahmad, I., Ahmad, S., Ahmad, A., Zughaibi, T. A., Alhosin, M., and Tabrez, S. (2024). Curcumin, its derivatives, and their nanoformulations: revolutionizing cancer treatment. Cell Biochem. Funct. 42 (1), e3911. doi:10.1002/cbf.3911
Ahn, S. G., Kim, S. J., Kim, C., and Jeong, J. (2016). Molecular classification of triple-negative breast cancer. J. Breast Cancer 19 (3), 223–230. doi:10.4048/jbc.2016.19.3.223
Akhand, S. S., Chen, H., Purdy, S. C., Liu, Z., Anderson, J. C., Willey, C. D., et al. (2021). Fibroblast growth factor receptor facilitates recurrence of minimal residual disease following trastuzumab emtansine therapy. NPJ Breast Cancer 7 (1), 5. doi:10.1038/s41523-020-00213-5
Ale, M. T., Maruyama, H., Tamauchi, H., Mikkelsen, J. D., and Meyer, A. S. (2011). Fucoidan from Sargassum sp. and Fucus vesiculosus reduces cell viability of lung carcinoma and melanoma cells in vitro and activates natural killer cells in mice in vivo. Int. J. Biol. Macromol. 49 (3), 331–336. doi:10.1016/j.ijbiomac.2011.05.009
Almansour, N. M. (2022). Triple-negative breast cancer: a brief review about epidemiology, risk factors, signaling pathways, treatment and role of artificial intelligence. Front. Mol. Biosci. 9, 836417. doi:10.3389/fmolb.2022.836417
Alserihi, R. F., Mohammed, M. R. S., Kaleem, M., Imran Khan, M., Sechi, M., Zughaibi, T. A., et al. (2023). Comparative efficacy of epigallocatechin gallate and its nano-formulation in prostate cancer 3D spheroids model. J. King Saud. Univ. - Sci. 35 (4), 102627. doi:10.1016/j.jksus.2023.102627
Alserihi, R. F., Mohammed, M. R. S., Kaleem, M., Khan, M. I., Sechi, M., Sanna, V., et al. (2021). Development of (-)-epigallocatechin-3-gallate-loaded folate receptor-targeted nanoparticles for prostate cancer treatment. Nanotechnol. Rev. 11 (1), 298–311. doi:10.1515/ntrev-2022-0013
Alzahrani, R., Alrehaili, A. A., Gharib, A. F., Anjum, F., Ismail, K. A., and Elsawy, W. H. (2020). Cancerous inhibitor of protein phosphatase 2A as a molecular marker for aggressiveness and survival in oral squamous cell carcinoma. J. Cancer Prev. 25 (1), 21–26. doi:10.15430/JCP.2020.25.1.21
Arab, K., Park, Y. J., Lindroth, A. M., Schäfer, A., Oakes, C., Weichenhan, D., et al. (2014). Long noncoding RNA TARID directs demethylation and activation of the tumor suppressor TCF21 via GADD45A. Mol. Cell 55 (4), 604–614. doi:10.1016/j.molcel.2014.06.031
Aram, R., Dotan, I., Hotz-Wagenblatt, A., and Canaani, D. (2017). Identification of a novel metastasis inducing lncRNA which suppresses the KAI1/CD82 metastasis suppressor gene and is upregulated in triple-negative breast cancer. Oncotarget 8 (40), 67538–67552. doi:10.18632/oncotarget.18733
Arnold, M., Morgan, E., Rumgay, H., Mafra, A., Singh, D., Laversanne, M., et al. (2022). Current and future burden of breast cancer: global statistics for 2020 and 2040. Breast 66, 15–23. doi:10.1016/j.breast.2022.08.010
Ashraf, W., Ibrahim, A., Alhosin, M., Zaayter, L., Ouararhni, K., Papin, C., et al. (2017). The epigenetic integrator UHRF1: on the road to become a universal biomarker for cancer. Oncotarget 8 (31), 51946–51962. doi:10.18632/oncotarget.17393
Asleh, K., Riaz, N., and Nielsen, T. O. (2022). Heterogeneity of triple negative breast cancer: current advances in subtyping and treatment implications. J. Exp. Clin. Cancer Res. 41 (1), 265. doi:10.1186/s13046-022-02476-1
Assumpção, J. H. M., Takeda, A. A. S., Sforcin, J. M., and Rainho, C. A. (2020). Effects of propolis and phenolic acids on triple-negative breast cancer cell lines: potential involvement of epigenetic mechanisms. Molecules 25 (6), 1289. doi:10.3390/molecules25061289
Balaga, V. K. R., Pradhan, A., Thapa, R., Patel, N., Mishra, R., and Singla, N. (2023). Morin: a comprehensive review on its versatile biological activity and associated therapeutic potential in treating cancers. Pharmacol. Res. - Mod. Chin. Med. 7, 100264. doi:10.1016/j.prmcm.2023.100264
Banerjee, S., Padhye, S., Azmi, A., Wang, Z., Philip, P. A., Kucuk, O., et al. (2010). Review on molecular and therapeutic potential of thymoquinone in cancer. Nutr. Cancer 62 (7), 938–946. doi:10.1080/01635581.2010.509832
Bao, C., Chen, J., Kim, J. T., Qiu, S., Cho, J. S., and Lee, H. J. (2019). Amentoflavone inhibits tumorsphere formation by regulating the Hedgehog/Gli1 signaling pathway in SUM159 breast cancer stem cells. J. Funct. Foods 61, 103501. doi:10.1016/j.jff.2019.103501
Barchiesi, G., Roberto, M., Verrico, M., Vici, P., Tomao, S., and Tomao, F. (2021). Emerging role of PARP inhibitors in metastatic triple negative breast cancer. Current scenario and future perspectives. Front. Oncol. 11, 769280. doi:10.3389/fonc.2021.769280
Basu, P., and Kumar, G. S. (2016). Sanguinarine and its role in chronic diseases. Adv. Exp. Med. Biol. 928, 155–172. doi:10.1007/978-3-319-41334-1_7
Batool, A., Miana, G. A., Alam, M., Khan, M. T., Muddassir, M., Zaman, W., et al. (2022). Bioassay-guided fractionation and isolation of Arctigenin from Saussurea heteromalla for in vitro and in silico cytotoxic activity against HeLa cells. Physiol. Mol. Plant Pathol. 117, 101749. doi:10.1016/j.pmpp.2021.101749
Beg, S., Siraj, A. K., Prabhakaran, S., Jehan, Z., Ajarim, D., Al-Dayel, F., et al. (2015). Loss of PTEN expression is associated with aggressive behavior and poor prognosis in Middle Eastern triple-negative breast cancer. Breast Cancer Res. Treat. 151 (3), 541–553. doi:10.1007/s10549-015-3430-3
Bilani, N., Zabor, E. C., Elson, L., Elimimian, E. B., and Nahleh, Z. (2020). Breast cancer in the United States: a cross-sectional overview. J. Cancer Epidemiol. 2020, 6387378. doi:10.1155/2020/6387378
Bimonte, S., Cascella, M., Barbieri, A., Arra, C., and Cuomo, A. (2020). Current shreds of evidence on the anticancer role of EGCG in triple negative breast cancer: an update of the current state of knowledge. Infect. Agent Cancer 15, 2. doi:10.1186/s13027-020-0270-5
Çetinkaya, M., and Baran, Y. (2023). Therapeutic potential of luteolin on cancer. Cancer. Vaccines. 11 (3), 554. doi:10.3390/vaccines11030554
Chamcheu, J. C., Esnault, S., Adhami, V. M., Noll, A. L., Banang-Mbeumi, S., Roy, T., et al. (2019). Fisetin, a 3,7,3′,4′-tetrahydroxyflavone inhibits the PI3K/Akt/mTOR and MAPK pathways and ameliorates psoriasis pathology in 2D and 3D organotypic human inflammatory skin models. Cells 8 (9), 1089. doi:10.3390/cells8091089
Chen, W. J., Tsai, J. H., Hsu, L. S., Lin, C. L., Hong, H. M., and Pan, M. H. (2021). Quercetin blocks the aggressive phenotype of triple-negative breast cancer by inhibiting igf1/igf1r-mediated emt program. J. Food Drug Anal. 29 (1), 98–112. doi:10.38212/2224-6614.3090
Chen, X. J., Zhang, X. J., Shui, Y. M., Wan, J. B., and Gao, J. L. (2016). Anticancer activities of protopanaxadiol- and protopanaxatriol-type ginsenosides and their metabolites. Evid. Based Complement. Altern. Med. 2016, 5738694. doi:10.1155/2016/5738694
Cheng, T. C., Sayseng, J. O., Tu, S. H., Juan, T. C., Fang, C. L., Liao, Y. C., et al. (2021). Curcumin-induced antitumor effects on triple-negative breast cancer patient-derived xenograft tumor mice through inhibiting salt-induced kinase-3 protein. J. Food Drug Anal. 29 (4), 622–637. doi:10.38212/2224-6614.3387
Chiu, C. C., Haung, J. W., Chang, F. R., Huang, K. J., Huang, H. M., Huang, H. W., et al. (2013). Golden berry-derived 4β-hydroxywithanolide E for selectively killing oral cancer cells by generating ROS, DNA damage, and apoptotic pathways. PLoS One 8 (5), e64739. doi:10.1371/journal.pone.0064739
Chokchaisiri, R., Kunkaewom, S., Chokchaisiri, S., Ganranoo, L., Chalermglin, R., and Suksamrarn, A. (2017). Potent cytotoxicity against human small cell lung cancer cells of the heptenes from the stem bark of Xylopia pierrei Hance. Med. Chem. Res. 26 (6), 1291–1296. doi:10.1007/s00044-017-1843-8
Chunarkar-patil, P., Kaleem, M., Mishra, R., Ray, S., Ahmad, A., Verma, D., et al. (2024). Anticancer drug discovery based on natural products: from computational approaches to clinical studies. Biomedicines 12 (1), 201. doi:10.3390/biomedicines12010201
Cook, M. T. (2018). Mechanism of metastasis suppression by luteolin in breast cancer. Breast Cancer (Dove Med. Press) 10, 89–100. doi:10.2147/BCTT.S144202
Deng, M., Chen, H., Long, J., Song, J., Xie, L., and Li, X. (2021). Calycosin: a review of its pharmacological effects and application prospects. Expert Rev. Anti Infect. Ther. 19 (7), 911–925. doi:10.1080/14787210.2021.1863145
Deng, Z., Chen, G., Shi, Y., Lin, Y., Ou, J., Zhu, H., et al. (2022). Curcumin and its nano-formulations: defining triple-negative breast cancer targets through network pharmacology, molecular docking, and experimental verification. Front. Pharmacol. 13, 920514. doi:10.3389/fphar.2022.920514
Ding, H., Yu, X., Hang, C., Gao, K., Lao, X., Jia, Y., et al. (2020). Ailanthone: a novel potential drug for treating human cancer. Oncol. Lett. 20 (2), 1489–1503. doi:10.3892/ol.2020.11710
Dookeran, K. A., Zhang, W., Stayner, L., and Argos, M. (2017). Associations of two-pore domain potassium channels and triple negative breast cancer subtype in the Cancer Genome Atlas: systematic evaluation of gene expression and methylation. BMC Res. Notes 10 (1), 475. doi:10.1186/s13104-017-2777-4
Dutta, R., Khalil, R., Green, R., Mohapatra, S. S., and Mohapatra, S. (2019). Withania somnifera (Ashwagandha) and withaferin a: potential in integrative oncology. Int. J. Mol. Sci. 20 (21), 5310. doi:10.3390/ijms20215310
Ensenyat-Mendez, M., Llinàs-Arias, P., Orozco, J. I. J., Íñiguez-Muñoz, S., Salomon, M. P., Sesé, B., et al. (2021). Current triple-negative breast cancer subtypes: dissecting the most aggressive form of breast cancer. Front. Oncol. 11, 681476. doi:10.3389/fonc.2021.681476
Fang, Y., and Fullwood, M. J. (2016). Roles, functions, and mechanisms of long non-coding RNAs in cancer. Genomics Proteomics Bioinforma. 14 (1), 42–54. doi:10.1016/j.gpb.2015.09.006
Fang, Z., Zhang, M., Liu, J. N., Zhao, X., Zhang, Y. Q., and Fang, L. (2021). Tanshinone IIA: a review of its anticancer effects. Front. Pharmacol. 11, 611087. doi:10.3389/fphar.2020.611087
Füllgrabe, J., Kavanagh, E., and Joseph, B. (2011). Histone onco-modifications. Oncogene 30 (31), 3391–3403. doi:10.1038/onc.2011.121
Gasparini, P., Lovat, F., Fassan, M., Casadei, L., Cascione, L., Jacob, N. K., et al. (2014). Protective role of miR-155 in breast cancer through RAD51 targeting impairs homologous recombination after irradiation. Proc. Natl. Acad. Sci. U. S. A. 111 (12), 4536–4541. doi:10.1073/pnas.1402604111
Gomathinayagam, R., Ha, J. H., Jayaraman, M., Song, Y. S., Isidoro, C., and Dhanasekaran, D. N. (2020). Chemopreventive and anticancer effects of thymoquinone: cellular and molecular targets. J. Cancer Prev. 25 (3), 136–151. doi:10.15430/JCP.2020.25.3.136
Guo, Z., Lou, Y., Kong, M., Luo, Q., Liu, Z., and Wu, J. (2019). A systematic review of phytochemistry, pharmacology and pharmacokinetics on astragali radix: implications for astragali radix as a personalized medicine. Int. J. Mol. Sci. 20 (6), 1463. doi:10.3390/ijms20061463
Hewlings, S. J., and Kalman, D. S. (2017). Curcumin: a review of its effects on human health. Foods 6 (10), 92. doi:10.3390/foods6100092
Hobbs, E. A., Chen, N., Kuriakose, A., Bonefas, E., and Lim, B. (2022). Prognostic/predictive markers in systemic therapy resistance and metastasis in breast cancer. Ther. Adv. Med. Oncol. 14, 17588359221112698. doi:10.1177/17588359221112698
Hsieh, K. Y., Tsai, J. Y., Lin, Y. H., Chang, F. R., Wang, H. C., and Wu, C. C. (2021). Golden berry 4β-hydroxywithanolide E prevents tumor necrosis factor α-induced procoagulant activity with enhanced cytotoxicity against human lung cancer cells. Sci. Rep. 11 (1), 4610. doi:10.1038/s41598-021-84207-8
Hsu, J. Y., Chang, C. J., and Cheng, J. S. (2022). Survival, treatment regimens and medical costs of women newly diagnosed with metastatic triple-negative breast cancer. Sci. Rep. 12 (1), 729. doi:10.1038/s41598-021-04316-2
Hu, H., Bai, H., Huang, L., Yang, B., Zhao, H., and Eupalinolide, J. (2023). Eupalinolide J inhibits cancer metastasis by promoting STAT3 ubiquitin-dependent degradation. Molecules 28 (7), 3143. doi:10.3390/molecules28073143
Huang, Q., Qin, S., Yuan, X., Zhang, L., Ji, J., Liu, X., et al. (2017). Arctigenin inhibits triple-negative breast cancers by targeting CIP2A to reactivate protein phosphatase 2A. Oncol. Rep. 38 (1), 598–606. doi:10.3892/or.2017.5667
Huarte, M. (2015). The emerging role of lncRNAs in cancer. Nat. Med. 21 (11), 1253–1261. doi:10.1038/nm.3981
Hubalek, M., Czech, T., and Müller, H. (2017). Biological subtypes of triple-negative breast cancer. Breast Care 12 (1), 8–14. doi:10.1159/000455820
Imran, M., Rauf, A., Abu-Izneid, T., Nadeem, M., Shariati, M. A., Khan, I. A., et al. (2019). Luteolin, a flavonoid, as an anticancer agent: a review. Biomed. Pharmacother. 112, 108612. doi:10.1016/j.biopha.2019.108612
Imran, M., Saeed, F., Gilani, S. A., Shariati, M. A., Imran, A., Afzaal, M., et al. (2020). Fisetin: an anticancer perspective. Food Sci. Nutr. 9 (1), 3–16. doi:10.1002/fsn3.1872
Jiang, Y. Z., Ma, D., Suo, C., Shi, J., Xue, M., Hu, X., et al. (2019). Genomic and transcriptomic landscape of triple-negative breast cancers: subtypes and treatment strategies. Cancer Cell 35 (3), 428–440. doi:10.1016/j.ccell.2019.02.001
Kabil, N., Bayraktar, R., Kahraman, N., Mokhlis, H. A., Calin, G. A., Lopez-Berestein, G., et al. (2018). Thymoquinone inhibits cell proliferation, migration, and invasion by regulating the elongation factor 2 kinase (eEF-2K) signaling axis in triple-negative breast cancer. Breast Cancer Res. Treat. 171 (3), 593–605. doi:10.1007/s10549-018-4847-2
Kagara, N., Huynh, K. T., Kuo, C., Okano, H., Sim, M. S., Elashoff, D., et al. (2012). Epigenetic regulation of cancer stem cell genes in triple-negative breast cancer. Am. J. Pathol. 181 (1), 257–267. doi:10.1016/j.ajpath.2012.03.019
Kaleem, M., Alhosin, M., Khan, K., Ahmad, W., Hosawi, S., Nur, S. M., et al. (2021b). “Epigenetic basis of polyphenols in cancer prevention and therapy,” in Polyphenols-based nanotherapeutics for cancer management. Editors S. Tabrez, and M. I. Khan (Springer Nature), 189–238.
Kaleem, M., Dalhat, M. H., Azmi, L., Asar, T. O., Ahmad, W., Alghanmi, M., et al. (2022). An insight into molecular targets of breast cancer brain metastasis. Int. J. Mol. Sci. 23 (19), 11687. doi:10.3390/ijms231911687
Kaleem, M., Kayali, A., Sheikh, R. A., Kuerban, A., Hassan, M. A., Almalki, N. A. R., et al. (2024). In vitro and in vivo preventive effects of thymoquinone against breast cancer: role of DNMT1. Molecules 29 (2), 434. doi:10.3390/molecules29020434
Kaleem, M., Perwaiz, M., Nur, S. M., Abdulrahman, A. O., Ahmad, W., Al-Abbasi, F. A., et al. (2021a). Epigenetics of triple-negative breast cancer via natural compounds. Curr. Med. Chem. 29 (8), 1436–1458. doi:10.2174/0929867328666210707165530
Kashiwagi, S., Yashiro, M., Takashima, T., Nomura, S., Noda, S., Kawajiri, H., et al. (2010). Significance of E-cadherin expression in triple-negative breast cancer. Br. J. Cancer 103 (2), 249–255. doi:10.1038/sj.bjc.6605735
Khan, M. A., Tania, M., Wei, C., Mei, Z., Fu, S., Cheng, J., et al. (2015). Thymoquinone inhibits cancer metastasis by downregulating TWIST1 expression to reduce epithelial to mesenchymal transition. Oncotarget 6 (23), 19580–19591. doi:10.18632/oncotarget.3973
Khan, N., Syed, D. N., Ahmad, N., and Mukhtar, H. (2013). Fisetin: a dietary antioxidant for health promotion. Antioxid. Redox Signal 19 (2), 151–162. doi:10.1089/ars.2012.4901
Kim, D. H., Khan, H., Ullah, H., Hassan, S. T. S., Šmejkal, K., Efferth, T., et al. (2019). MicroRNA targeting by quercetin in cancer treatment and chemoprotection. Pharmacol. Res. 147, 104346. doi:10.1016/j.phrs.2019.104346
Kim, J. K., and Park, S. U. (2016). Current potential health benefits of sulforaphane. EXCLI J. 15, 571–577. doi:10.17179/excli2016-485
Kong, Y., Geng, C., and Dong, Q. (2019). LncRNA PAPAS may promote triple-negative breast cancer by downregulating miR-34a. J. Int. Med. Res. 47 (8), 3709–3718. doi:10.1177/0300060519850724
Kubina, R., Iriti, M., and Kabała-Dzik, A. (2021). Anticancer potential of selected flavonols: fisetin, kaempferol, and quercetin on head and neck cancers. Nutrients 13 (3), 845. doi:10.3390/nu13030845
Kumar, M., Saurabh, V., Tomar, M., Hasan, M., Changan, S., Sasi, M., et al. (2021). Mango (Mangifera indica l.) leaves: nutritional composition, phytochemical profile, and health-promoting bioactivities. Antioxidants 10 (2), 299. doi:10.3390/antiox10020299
Lecomte, S., Chalmel, F., Ferriere, F., Percevault, F., Plu, N., Saligaut, C., et al. (2017). Glyceollins trigger anti-proliferative effects through estradiol-dependent and independent pathways in breast cancer cells. Cell Commun. Signal 15 (1), 26. doi:10.1186/s12964-017-0182-1
Lee, S. H., Jee, J. G., Bae, J. S., Liu, K. H., and Lee, Y. M. (2015). A group of novel HIF-1α inhibitors, glyceollins, blocks HIF-1α synthesis and decreases its stability via inhibition of the PI3K/AKT/mTOR pathway and Hsp90 binding. J. Cell Physiol. 230 (4), 853–862. doi:10.1002/jcp.24813
Lehmann, B. D., Colaprico, A., Silva, T. C., Chen, J., An, H., Ban, Y., et al. (2021). Multi-omics analysis identifies therapeutic vulnerabilities in triple-negative breast cancer subtypes. Nat. Commun. 12 (1), 6276. doi:10.1038/s41467-021-26502-6
Li, G., Shan, C., Liu, L., Zhou, T., Zhou, J., Hu, X., et al. (2015). Tanshinone IIA inhibits HIF-1α and VEGF expression in breast cancer cells via mTOR/p70S6K/RPS6/4E-BP1 signaling pathway. PLoS One 10 (2), e0117440. doi:10.1371/journal.pone.0117440
Li, J., Gong, X., Jiang, R., Lin, D., Zhou, T., Zhang, A., et al. (2018). Fisetin inhibited growth and metastasis of triple-negative breast cancer by reversing epithelial-to-mesenchymal transition via PTEN/Akt/GSK3β signal pathway. Front. Pharmacol. 9 (9), 772. doi:10.3389/fphar.2018.00772
Li, M., Guo, T., Lin, J., Huang, X., Ke, Q., Wu, Y., et al. (2022b). Curcumin inhibits the invasion and metastasis of triple negative breast cancer via Hedgehog/Gli1 signaling pathway. J. Ethnopharmacol. 283, 114689. doi:10.1016/j.jep.2021.114689
Li, Q., Liu, J., Meng, X., Pang, R., and Li, J. (2017). MicroRNA-454 may function as an oncogene via targeting AKT in triple negative breast cancer. J. Biol. Res. 24 (1), 10. doi:10.1186/s40709-017-0067-x
Li, W., Xue, D., Xue, M., Zhao, J., Liang, H., Liu, Y., et al. (2019). Fucoidan inhibits epithelial-to-mesenchymal transition via regulation of the HIF-1α pathway in mammary cancer cells under hypoxia. Oncol. Lett. 18 (1), 330–338. doi:10.3892/ol.2019.10283
Li, X., Hou, L., Yin, L., and Zhao, S. (2020). LncRNA XIST interacts with miR-454 to inhibit cells proliferation, epithelial mesenchymal transition and induces apoptosis in triple-negative breast cancer. J. Biosci. 45 (1), 45. doi:10.1007/s12038-020-9999-7
Li, Y., Hu, S., Chen, Y., Zhang, X., Gao, H., Tian, J., et al. (2023). Calycosin inhibits triple-negative breast cancer progression through down-regulation of the novel estrogen receptor-α splice variant ER-α30-mediated PI3K/AKT signaling pathway. Phytomedicine 118, 154924. doi:10.1016/j.phymed.2023.154924
Li, Y. Z., Chen, B., Lin, X. Y., Zhang, G. C., Lai, J. G., Li, C., et al. (2022a). Clinicopathologic and genomic features in triple-negative breast cancer between special and No-special morphologic pattern. Front. Oncol. 12, 830124. doi:10.3389/fonc.2022.830124
Lin, Y. T., and Wu, K. J. (2020). Epigenetic regulation of epithelial-mesenchymal transition: focusing on hypoxia and TGF-β signaling. J. Biomed. Sci. 27 (1), 39. doi:10.1186/s12929-020-00632-3
Litton, J. K., Beck, J. T., Jones, J. M., Andersen, J., Blum, J. L., Mina, L. A., et al. (2023). Neoadjuvant talazoparib in patients with germline BRCA1/2 mutation-positive, early-stage triple-negative breast cancer: results of a phase II study. Oncologist 28 (10), 845–855. doi:10.1093/oncolo/oyad139
Liu, C., Wang, K., Zhuang, J., Gao, C., Li, H., Liu, L., et al. (2019b). The modulatory properties of Astragalus membranaceus treatment on triple-negative breast cancer: an integrated pharmacological method. Front. Pharmacol. 10, 1171. doi:10.3389/fphar.2019.01171
Liu, J., Liu, Q., Li, Y., Li, Q., Su, F., Yao, H., et al. (2020). Efficacy and safety of camrelizumab combined with apatinib in advanced triple-negative breast cancer: an open-label phase II trial. J. Immunother. Cancer 8 (1), e000696. doi:10.1136/jitc-2020-000696
Liu, J., Wang, Y., Tian, Z., Lin, Y., Li, H., Zhu, Z., et al. (2022). Multicenter phase II trial of Camrelizumab combined with Apatinib and Eribulin in heavily pretreated patients with advanced triple-negative breast cancer. Nat. Commun. 13 (1), 3011. doi:10.1038/s41467-022-30569-0
Liu, L., Kimball, S., Liu, H., Holowatyj, A., and Yang, Z. Q. (2015). Genetic alterations of histone lysine methyltransferases and their significance in breast cancer. Oncotarget 6 (4), 2466–2482. doi:10.18632/oncotarget.2967
Liu, X. C., Zhu, Z. Y., Liu, Y. L., and Sun, H. Q. (2019a). Comparisons of the anti-tumor activity of polysaccharides from fermented mycelia and cultivated fruiting bodies of Cordyceps militaris in vitro. Int. J. Biol. Macromol. 130, 307–314. doi:10.1016/j.ijbiomac.2019.02.155
Liu, Y. R., Jiang, Y. Z., Xu, X. E., Yu, K. D., Jin, X., Hu, X., et al. (2016). Comprehensive transcriptome analysis identifies novel molecular subtypes and subtype-specific RNAs of triple-negative breast cancer. Breast Cancer Res. 18 (1), 33. doi:10.1186/s13058-016-0690-8
Loi, S., Francis, P. A., Zdenkowski, N., Gebski, V., Fox, S. B., White, M., et al. (2024). Phase II study of neoadjuvant ipilimumab and nivolumab in combination with paclitaxel following anthracycline-based chemotherapy in patients with treatment resistant stage III triple negative breast cancer (TNBC): BCT1702 — survival results. J. Clin. Oncol. 42 (16), 608.
Lou, C., Chen, Y., Zhang, J., Yang, B., and Zhao, H. (2019). Eupalinolide J Suppresses the growth of triple-negative breast cancer cells via targeting STAT3 signaling pathway. Front. Pharmacol. 10, 1071. doi:10.3389/fphar.2019.01071
Lu, J., Getz, G., Miska, E. A., Alvarez-Saavedra, E., Lamb, J., Peck, D., et al. (2005). MicroRNA expression profiles classify human cancers. Nature 435 (7043), 834–838. doi:10.1038/nature03702
Łukasiewicz, S., Czeczelewski, M., Forma, A., Baj, J., Sitarz, R., and Stanisławek, A. (2021). Breast cancer—epidemiology, risk factors, classification, prognostic markers, and current treatment strategies—an updated review. Cancers. 13 (17), 4287. doi:10.3390/cancers13174287
Luo, L., Tang, H., Ling, L., Li, N., Jia, X., Zhang, Z., et al. (2018). LINC01638 lncRNA activates MTDH-Twist1 signaling by preventing SPOP-mediated c-Myc degradation in triple-negative breast cancer. Oncogene 37 (47), 6166–6179. doi:10.1038/s41388-018-0396-8
Luo, W., Wang, F., Luo, H., and Liu, H. (2021). Arctigenin inhibits human breast cancer cell proliferation, migratory and invasive abilities and epithelial to mesenchymal transition by targeting 4EBP1. Exp. Ther. Med. 21 (6), 547. doi:10.3892/etm.2021.9979
Luthuli, S., Wu, S., Cheng, Y., Zheng, X., Wu, M., and Tong, H. (2019). Therapeutic effects of fucoidan: a review on recent studies. Mar. Drugs 17 (9), 487. doi:10.3390/md17090487
Lv, C., Zeng, H. W., Wang, J. X., Yuan, X., Zhang, C., Fang, T., et al. (2018). The antitumor natural product tanshinone IIA inhibits protein kinase C and acts synergistically with 17-AAG. Cell Death Dis. 9 (2), 165. doi:10.1038/s41419-017-0247-5
Maenohara, S., Unoki, M., Toh, H., Ohishi, H., Sharif, J., Koseki, H., et al. (2017). Role of UHRF1 in de novo DNA methylation in oocytes and maintenance methylation in preimplantation embryos. Plos Genet. 13 (10), e1007042. doi:10.1371/journal.pgen.1007042
Maharjan, S., Lee, M. G., Kim, S. Y., Lee, K. S., and Nam, K. S. (2023). Morin sensitizes MDA-MB-231 triple-negative breast cancer cells to doxorubicin cytotoxicity by suppressing FOXM1 and attenuating EGFR/STAT3 signaling pathways. Pharmaceuticals 16 (5), 672. doi:10.3390/ph16050672
Manoochehri, M., Hielscher, T., Borhani, N., Gerhäuser, C., Fletcher, O., Swerdlow, A. J., et al. (2021). Epigenetic quantification of circulating immune cells in peripheral blood of triple-negative breast cancer patients. Clin. Epigenetics 13 (1), 207. doi:10.1186/s13148-021-01196-1
Mansour, M. B., Balti, R., Yacoubi, L., Ollivier, V., Chaubet, F., and Maaroufi, R. M. (2019). Primary structure and anticoagulant activity of fucoidan from the sea cucumber Holothuria polii. Int. J. Biol. Macromol. 121, 1145–1153. doi:10.1016/j.ijbiomac.2018.10.129
Mensah, I. K., Norvil, A. B., Alabdi, L., McGovern, S., Petell, C. J., He, M., et al. (2021). Misregulation of the expression and activity of DNA methyltransferases in cancer. Nar. Cancer 3 (4), zcab045. doi:10.1093/narcan/zcab045
Messeha, S. S., Noel, S., Zarmouh, N. O., Womble, T., Latinwo, L. M., and Soliman, K. F. A. (2023). Involvement of AKT/PI3K pathway in sanguinarine’s induced apoptosis and cell cycle arrest in triple-negative breast cancer cells. Cancer Genomics Proteomics 20 (4), 323–342. doi:10.21873/cgp.20385
Messeha, S. S., Zarmouh, N. O., Antonie, L., and Soliman, K. F. A. (2022). Sanguinarine inhibition of TNF-α-Induced CCL2, IKBKE/NF-κB/ERK1/2 signaling pathway, and cell migration in human triple-negative breast cancer cells. Int. J. Mol. Sci. 23 (15), 8329. doi:10.3390/ijms23158329
Messier, T. L., Gordon, J. A. R., Boyd, J. R., Tye, C. E., Browne, G., Stein, J. L., et al. (2016). Histone H3 lysine 4 acetylation and methylation dynamics define breast cancer subtypes. Oncotarget 7 (5), 5094–5109. doi:10.18632/oncotarget.6922
Michniak-Kohn, B. B., Deol, P. K., Kotta, S., Aldawsari, H. M., Badr-Eldin, S. M., Nair, A. B., et al. (2022). Thermosensitive hydrogels loaded with resveratrol nanoemulsion: formulation optimization by central composite design and evaluation in MCF-7 human breast cancer cell lines. Gels 8 (7), 450. doi:10.3390/gels8070450
Min, A., Im, S. A., Kim, D. K., Song, S. H., Kim, H. J., Lee, K. H., et al. (2015). Histone deacetylase inhibitor, suberoylanilide hydroxamic acid (SAHA), enhances anti-tumor effects of the poly (ADP-ribose) polymerase (PARP) inhibitor olaparib in triple-negative breast cancer cells. Breast Cancer Res. 17 (1), 33. doi:10.1186/s13058-015-0534-y
Mokra, D., Adamcakova, J., and Mokry, J. (2022). Green tea polyphenol (-)-Epigallocatechin-3-Gallate (EGCG): a time for a new player in the treatment of respiratory diseases. Antioxidants 11 (8), 1566. doi:10.3390/antiox11081566
Mottamal, M., Zheng, S., Huang, T. L., and Wang, G. (2015). Histone deacetylase inhibitors in clinical studies as templates for new anticancer agents. Molecules 20 (3), 3898–3941. doi:10.3390/molecules20033898
Nedeljković, M., and Damjanović, A. (2019). Mechanisms of chemotherapy resistance in triple-negative breast cancer-how we can rise to the challenge. Cells 8 (9), 957. doi:10.3390/cells8090957
Ock, C. W., and Kim, G. D. (2021). Dioscin decreases breast cancer stem-like cell proliferation via cell cycle arrest by modulating p38 mitogen-activated protein kinase and AKT/mTOR signaling pathways. J. Cancer Prev. 26 (3), 183–194. doi:10.15430/JCP.2021.26.3.183
O’Shaughnessy, J., Brufsky, A., Rugo, H. S., Tolaney, S. M., Punie, K., Sardesai, S., et al. (2022). Analysis of patients without and with an initial triple-negative breast cancer diagnosis in the phase 3 randomized ASCENT study of sacituzumab govitecan in metastatic triple-negative breast cancer. Breast Cancer Res. Treat. 195 (2), 127–139. doi:10.1007/s10549-022-06602-7
O’Shaughnessy, J., Moroose, R. L., Babu, S., Baramidze, K., Chan, D., Leitner, S. P., et al. (2020). Results of ENCORE 602 (TRIO025), a phase II, randomized, placebo-controlled, double-blinded, multicenter study of atezolizumab with or without entinostat in patients with advanced triple-negative breast cancer (aTNBC). J. Clin. Oncol. 38 (15_Suppl. l), 1014. doi:10.1200/jco.2020.38.15_suppl.1014
Pang, J., Shen, N., Yan, F., Zhao, N., Dou, L., Wu, L. C., et al. (2017). Thymoquinone exerts potent growth-suppressive activity on leukemia through DNA hypermethylation reversal in leukemia cells. Oncotarget 8 (21), 34453–34467. doi:10.18632/oncotarget.16431
Pang, Y., Liu, J., Li, X., Xiao, G., Wang, H., Yang, G., et al. (2018). MYC and DNMT3A-mediated DNA methylation represses microRNA-200b in triple negative breast cancer. J. Cell Mol. Med. 22 (12), 6262–6274. doi:10.1111/jcmm.13916
Park, I. S., Kim, H. J., Jeong, Y. S., Kim, W. K., and Kim, J. S. (2017). Differential abilities of Korean soybean varieties to biosynthesize glyceollins by biotic and abiotic elicitors. Food Sci. Biotechnol. 26 (1), 255–261. doi:10.1007/s10068-017-0034-1
Paul, S., Chakraborty, S., Anand, U., Dey, S., Nandy, S., Ghorai, M., et al. (2021). Withania somnifera (L.) Dunal (Ashwagandha): a comprehensive review on ethnopharmacology, pharmacotherapeutics, biomedicinal and toxicological aspects. Biomed. Pharmacother. 143, 112175. doi:10.1016/j.biopha.2021.112175
Peng, B., He, R., Xu, Q., Yang, Y., Hu, Q., Hou, H., et al. (2019). Ginsenoside 20(S)-protopanaxadiol inhibits triple-negative breast cancer metastasis in vivo by targeting EGFR-mediated MAPK pathway. Pharmacol. Res. 142, 1–13. doi:10.1016/j.phrs.2019.02.003
Peng, W. X., Koirala, P., and Mo, Y. Y. (2017). LncRNA-mediated regulation of cell signaling in cancer. Oncogene. Oncogene 36 (41), 5661–5667. doi:10.1038/onc.2017.184
Pham, T. H., Lecomte, S., Efstathiou, T., Ferriere, F., and Pakdel, F. (2019). An update on the effects of glyceollins on human health: possible anticancer effects and underlying mechanisms. Nutrients 11 (1), 79. doi:10.3390/nu11010079
Piasecka, D., Braun, M., Kordek, R., Sadej, R., and Romanska, H. (2018). MicroRNAs in regulation of triple-negative breast cancer progression. J. Cancer Res. Clin. Oncol. 144 (8), 1401–1411. doi:10.1007/s00432-018-2689-2
Piperigkou, Z., Franchi, M., Riethmüller, C., Götte, M., and Karamanos, N. K. (2020). miR-200b restrains EMT and aggressiveness and regulates matrix composition depending on ER status and signaling in mammary cancer. Matrix Biol. Plus, 6–7. doi:10.1016/j.mbplus.2020.100024
Piperigkou, Z., and Karamanos, N. K. (2019). Dynamic interplay between miRNAs and the extracellular matrix influences the tumor microenvironment. Trends Biochem. Sci. 44 (12), 1076–1088. doi:10.1016/j.tibs.2019.06.007
Qadi, S. A., Hassan, M. A., Sheikh, R. A., Baothman, OAAS, Zamzami, M. A., Choudhry, H., et al. (2019). Thymoquinone-induced reactivation of tumor suppressor genes in cancer cells involves epigenetic mechanisms. Epigenet Insights 12, 2516865719839011. doi:10.1177/2516865719839011
Qattan, A. (2020). Novel mirna targets and therapies in the triple-negative breast cancer microenvironment: an emerging hope for a challenging disease. Int. J. Mol. Sci. 21 (23), 8905. doi:10.3390/ijms21238905
Qi, P., and Du, X. (2013). The long non-coding RNAs, a new cancer diagnostic and therapeutic gold mine. Mod. Pathol. 26 (2), 155–165. doi:10.1038/modpathol.2012.160
Quintas-Granados, L. I., Cortés, H., Carmen, M. G. D., Leyva-Gómez, G., Bustamante-Montes, L. P., Rodríguez-Morales, M., et al. (2021). The high methylation level of a novel 151-bp CpG island in the ESR1 gene promoter is associated with a poor breast cancer prognosis. Cancer Cell Int. 21 (1), 649. doi:10.1186/s12935-021-02343-7
Radhi, M., Ashraf, S., Lawrence, S., Tranholm, A. A., Wellham, P. A. D., Hafeez, A., et al. (2021). A systematic review of the biological effects of cordycepin. Molecules 26 (19), 5886. doi:10.3390/molecules26195886
Ramazi, S., Allahverdi, A., and Zahiri, J. (2020). Evaluation of post-translational modifications in histone proteins: a review on histone modification defects in developmental and neurological disorders. J. Biosci. 45, 135. doi:10.1007/s12038-020-00099-2
Rasul, A., Riaz, A., Wei, W., Sarfraz, I., Hassan, M., Li, J., et al. (2021). Mangifera indica extracts as novel PKM2 inhibitors for treatment of triple negative breast cancer. Biomed. Res. Int. 2021, 5514669. doi:10.1155/2021/5514669
Reddy Dachani, S., Kaleem, M., Ali Mujtaba, M., Mahajan, N., Ali S, E., F Almutairy, A., et al. (2024). A comprehensive review of various therapeutic strategies for the management of skin cancer. ACS Omega 9, 10030–10048. doi:10.1021/acsomega.3c09780
Rhodes, L. V., Tilghman, S. L., Boue, S. M., Wang, S., Khalili, H., Muir, S. E., et al. (2012). Glyceollins as novel targeted therapeutic for the treatment of triple-negative breast cancer. Oncol. Lett. 3 (1), 163–171. doi:10.3892/ol.2011.460
Roussos Torres, E. T., Rafie, C., Wang, C., Lim, D., Brufsky, A., LoRusso, P., et al. (2021). Phase I study of entinostat and nivolumab with or without ipilimumab in advanced solid tumors (ETCTN-9844). Clin. Cancer Res. 27 (21), 5828–5837. doi:10.1158/1078-0432.CCR-20-5017
Salehi, B., Machin, L., Monzote, L., Sharifi-Rad, J., Ezzat, S. M., Salem, M. A., et al. (2020). Therapeutic potential of quercetin: new insights and perspectives for human health. ACS Omega 5 (20), 11849–11872. doi:10.1021/acsomega.0c01818
Sandhu, R., Rivenbark, A. G., and Coleman, W. B. (2012). Enhancement of chemotherapeutic efficacy in hypermethylator breast cancer cells through targeted and pharmacologic inhibition of DNMT3b. Breast Cancer Res. Treat. 131 (2), 385–399. doi:10.1007/s10549-011-1409-2
Santana, T. A. B. d. S., de Oliveira Passamai, L., de Miranda, F. S., Borin, T. F., Borges, G. F., Luiz, W. B., et al. (2022). The role of miRNAs in the prognosis of triple-negative breast cancer: a systematic review and meta-analysis. Diagnostics 13 (1), 127. doi:10.3390/diagnostics13010127
Schmid, P., Adams, S., Rugo, H. S., Schneeweiss, A., Barrios, C. H., Iwata, H., et al. (2018). Atezolizumab and nab-paclitaxel in advanced triple-negative breast cancer. N. Engl. J. Med. 379 (22), 2108–2121. doi:10.1056/NEJMoa1809615
Schröder, L., Marahrens, P., Koch, J. G., Heidegger, H., Vilsmaier, T., Phan-Brehm, T., et al. (2020). Erratum: effects of green tea, matcha tea and their components epigallocatechin gallate and quercetin on MCF-7 and MDA-MB-231 breast carcinoma cells. Oncol. Rep. 43 (2), 747. doi:10.3892/or.2019.7430
Seo, D. Y., Lee, S. R., Heo, J. W., No, M. H., Rhee, B. D., Ko, K. S., et al. (2018). Ursolic acid in health and disease. Korean J. Physiol. Pharmacol. 22 (3), 235–248. doi:10.4196/kjpp.2018.22.3.235
Shahi Thakuri, P., Gupta, M., Singh, S., Joshi, R., Glasgow, E., Lekan, A., et al. (2020). Phytochemicals inhibit migration of triple negative breast cancer cells by targeting kinase signaling. BMC Cancer 20 (1), 4. doi:10.1186/s12885-019-6479-2
Shang, Q., Xu, H., and Huang, L. (2012). Tanshinone IIA: a promising natural cardioprotective agent. Evid. Based Complement. Altern. Med. 2012, 716459. doi:10.1155/2012/716459
Singh, N., and Sharma, B. (2018). Toxicological effects of berberine and sanguinarine. Front. Mol. Biosci. 19 (5), 21. doi:10.3389/fmolb.2018.00021
Solairaja, S., Andrabi, M. Q., Dunna, N. R., and Venkatabalasubramanian, S. (2021). Overview of morin and its complementary role as an adjuvant for anticancer agents. Nutr. Cancer 73 (6), 927–942. doi:10.1080/01635581.2020.1778747
Song, X., Liu, Z., and Yu, Z. (2019). LncRNA NEF is downregulated in triple negative breast cancer and correlated with poor prognosis. Acta Biochim. Biophys. Sin. (Shanghai). 51 (4), 386–392. doi:10.1093/abbs/gmz021
Stirzaker, C., Zotenko, E., Song, J. Z., Qu, W., Nair, S. S., Locke, W. J., et al. (2015). Methylome sequencing in triple-negative breast cancer reveals distinct methylation clusters with prognostic value. Nat. Commun. 6, 5899. doi:10.1038/ncomms6899
Suhail, M., Rehan, M., Tarique, M., Tabrez, S., Husain, A., and Zughaibi, T. A. (2023). Targeting a transcription factor NF-κB by green tea catechins using in silico and in vitro studies in pancreatic cancer. Front. Nutr. 9, 1078642. doi:10.3389/fnut.2022.1078642
Sultana, T., Okla, M. K., Ahmed, M., Akhtar, N., Al-Hashimi, A., Abdelgawad, H., et al. (2021). Withaferin A: from ancient remedy to potential drug candidate. Molecules 26 (24), 7696. doi:10.3390/molecules26247696
Tang, J. Y., Huang, H. W., Wang, H. R., Chan, Y. C., Haung, J. W., Shu, C. W., et al. (2018). 4β-Hydroxywithanolide E selectively induces oxidative DNA damage for selective killing of oral cancer cells. Environ. Toxicol. 33 (3), 295–304. doi:10.1002/tox.22516
Tang, L., Wei, D., and Yan, F. (2016). MicroRNA-145 functions as a tumor suppressor by targeting matrix metalloproteinase 11 and Rab GTPase family 27a in triple-negative breast cancer. Cancer Gene Ther. 23 (8), 258–265. doi:10.1038/cgt.2016.27
Tate, C. R., Rhodes, L. V., Segar, H. C., Driver, J. L., Pounder, F. N., Burow, M. E., et al. (2012). Targeting triple-negative breast cancer cells with the histone deacetylase inhibitor panobinostat. Breast Cancer Res. 14 (3), R79. doi:10.1186/bcr3192
Temian, D. C., Pop, L. A., Irimie, A. I., and Berindan-Neagoe, I. (2018). The epigenetics of triple-negative and basal-like breast cancer: current knowledge. J. Breast Cancer 21 (3), 233–243. doi:10.4048/jbc.2018.21.e41
Thepmalee, C., Sawasdee, N., Jenkham, P., Thephinlap, C., Khoothiam, K., Suwannasom, N., et al. (2023). Anti-cancer effect of a phytochemical compound – 7R-acetylmelodorinol – against triple-negative breast cancer cells. Biomed. Pharmacother. 166 (October), 115286. doi:10.1016/j.biopha.2023.115286
Tian, Y., Li, Z., Shen, B., Zhang, Q., and Feng, H. (2017). Protective effects of morin on lipopolysaccharide/D-galactosamine-induced acute liver injury by inhibiting TLR4/NF-κB and activating Nrf2/HO-1 signaling pathways. Int. Immunopharmacol. 45, 148–155. doi:10.1016/j.intimp.2017.02.010
Tripathi, M. K., Doxtater, K., Keramatnia, F., Zacheaus, C., Yallapu, M. M., Jaggi, M., et al. (2018). Role of lncRNAs in ovarian cancer: defining new biomarkers for therapeutic purposes. Drug Discov. Today 23 (9), 1635–1643. doi:10.1016/j.drudis.2018.04.010
Uddin, M. G., and Fandy, T. E. (2021). DNA methylation inhibitors: retrospective and perspective view. Adv. Cancer Res. 152, 205–223. doi:10.1016/bs.acr.2021.03.007
vel Szic, K. S., Declerck, K., Crans, R. A. J., Diddens, J., Scherf, D. B., Gerhäuser, C., et al. (2017). Epigenetic silencing of triple negative breast cancer hallmarks by Withaferin A. Oncotarget 8 (25), 40434–40453. doi:10.18632/oncotarget.17107
Von, M. G., Schneeweiss, A., Loibl, S., Salat, C., Denkert, C., Rezai, M., et al. (2014). Neoadjuvant carboplatin in patients with triple-negative and HER2-positive early breast cancer (GeparSixto; GBG 66): a randomised phase 2 trial. Lancet Oncol. 15 (7), 747–756. doi:10.1016/S1470-2045(14)70160-3
Wagh, V. D. (2013). Propolis: a wonder bees product and its pharmacological potentials. Adv. Pharmacol. Sci. 2013, 308249. doi:10.1155/2013/308249
Wang, G., Wang, Y., Yao, L., Gu, W., Zhao, S., Shen, Z., et al. (2022). Pharmacological activity of quercetin: an updated review. Evid. Based Complement. Altern. Med. 2022, 3997190. doi:10.1155/2022/3997190
Wang, H. C., Hu, H. H., Chang, F. R., Tsai, J. Y., Kuo, C. Y., Wu, Y. C., et al. (2019b). Different effects of 4β-hydroxywithanolide E and withaferin A, two withanolides from Solanaceae plants, on the Akt signaling pathway in human breast cancer cells. Phytomedicine 53, 213–222. doi:10.1016/j.phymed.2018.09.017
Wang, H. C., Tsai, Y. L., Wu, Y. C., Chang, F. R., Liu, M. H., Chen, W. Y., et al. (2012). Withanolides-induced breast cancer cell death is correlated with their ability to inhibit heat protein 90. PLoS One 7 (5), e37764. doi:10.1371/journal.pone.0037764
Wang, L., Liu, D., Wu, X., Zeng, Y., Li, L., Hou, Y., et al. (2018b). Long non-coding RNA (LncRNA) RMST in triple-negative breast cancer (TNBC): expression analysis and biological roles research. J. Cell Physiol. 233 (10), 6603–6612. doi:10.1002/jcp.26311
Wang, L., Luan, T., Zhou, S., Lin, J., Yang, Y., Liu, W., et al. (2019a). LncRNA HCP5 promotes triple negative breast cancer progression as a ceRNA to regulate BIRC3 by sponging miR-219a-5p. Cancer Med. 8 (9), 4389–4403. doi:10.1002/cam4.2335
Wang, R., Lu, Y., Li, H., Sun, L., Yang, N., Zhao, M., et al. (2018c). Antitumor activity of the Ailanthus altissima bark phytochemical ailanthone against breast cancer MCF-7 cells. Oncol. Lett. 15 (4), 6022–6028. doi:10.3892/ol.2018.8039
Wang, X., Qiu, H., Tang, R., Song, H., Pan, H., Feng, Z., et al. (2018a). miR-30a inhibits epithelial-mesenchymal transition and metastasis in triple-negative breast cancer by targeting ROR1. Oncol. Rep. 39 (6), 2635–2643. doi:10.3892/or.2018.6379
Wang, Y., Zhong, Z., Ma, M., Zhao, Y., Zhang, C., Qian, Z., et al. (2023). The role played by ailanthone in inhibiting bone metastasis of breast cancer by regulating tumor-bone microenvironment through the RANKL-dependent pathway. Front. Pharmacol. 13, 1081978. doi:10.3389/fphar.2022.1081978
Wang, Z. T., Chen, Z. J., Jiang, G. M., Wu, Y. M., Liu, T., Yi, Y. M., et al. (2016). Histone deacetylase inhibitors suppress mutant p53 transcription via HDAC8/YY1 signals in triple negative breast cancer cells. Cell Signal 28 (5), 506–515. doi:10.1016/j.cellsig.2016.02.006
Ward, A. K., Mellor, P., Smith, S. E., Kendall, S., Just, N. A., Vizeacoumar, FJFS, et al. (2016). Epigenetic silencing of CREB3L1 by DNA methylation is associated with high-grade metastatic breast cancers with poor prognosis and is prevalent in triple negative breast cancers. Breast Cancer Res. 18 (1), 12. doi:10.1186/s13058-016-0672-x
Wei, C., Khan, M. A., Du, J., Cheng, J., Tania, M., Leung, E. L. H., et al. (2022). Cordycepin inhibits triple-negative breast cancer cell migration and invasion by regulating EMT-TFs SLUG, TWIST1, SNAIL1, and ZEB1. Front. Oncol. 12, 898583. doi:10.3389/fonc.2022.898583
Wong, C. M., Tsang, F. H., and Ng, I. O. L. (2018). Non-coding RNAs in hepatocellular carcinoma: molecular functions and pathological implications. Nat. Rev. Gastroenterol. Hepatol. 15 (3), 137–151. doi:10.1038/nrgastro.2017.169
Wu, L., Lin, Y., Gao, S., Wang, Y., Pan, H., Wang, Z., et al. (2023). Luteolin inhibits triple-negative breast cancer by inducing apoptosis and autophagy through SGK1-FOXO3a-BNIP3 signaling. Front. Pharmacol. 14, 1200843. doi:10.3389/fphar.2023.1200843
Wu, W., Li, X., Qi, M., Hu, X., Cao, F., Wu, X., et al. (2022). Cordycepin inhibits growth and metastasis formation of MDA-MB-231 xenografts in nude mice by modulating the Hedgehog pathway. Int. J. Mol. Sci. 23 (18), 10362. doi:10.3390/ijms231810362
Wu, X. (2020). Expressions of MiR-21 and MiR-210 in breast cancer and their predictive values for prognosis. Iran. J. Public Health 49 (1), 21–29. doi:10.18502/ijph.v49i1.3048
Wu, Z., Xu, X., Dai, L., Wang, Y., Yang, B., Zhao, H., et al. (2020). Eupalinolide J induces apoptosis, cell cycle arrest, mitochondrial membrane potential disruption and DNA damage in human prostate cancer cells. J. Toxicol. Sci. 45 (1), 15–23. doi:10.2131/jts.45.15
Xia, J., Lü, Z., Zhou, P., Cui, S., Kong, B., Liu, D., et al. (2017). DNA methylation modification of BRMS1 in triple-negative breast cancer and its correlation with tumor metastasis. Natl. Med. J. China 97 (44), 3483–3487. doi:10.3760/cma.j.issn.0376-2491.2017.44.010
Xie, L., Yi, J., Song, Y., Zhao, M., Fan, L., and Zhao, L. (2021). Suppression of GOLM1 by EGCG through HGF/HGFR/AKT/GSK-3β/β-catenin/c-Myc signaling pathway inhibits cell migration of MDA-MB-231. Food Chem. Toxicol. 157, 112574. doi:10.1016/j.fct.2021.112574
Xiong, X., Tang, N., Lai, X., Zhang, J., Wen, W., Li, X., et al. (2021). Insights into amentoflavone: a natural multifunctional biflavonoid. Front. Pharmacol. 12, 768708. doi:10.3389/fphar.2021.768708
Yang, Y., Liu, L., Fang, M., Bai, H., and Xu, Y. (2019). The chromatin remodeling protein BRM regulates the transcription of tight junction proteins: implication in breast cancer metastasis. Biochim. Biophys. Acta - Gene Regul. Mech. 1862 (5), 547–556. doi:10.1016/j.bbagrm.2019.03.002
Yasunaga, A., Ono, M., Takeshima, M., and Nakano, S. (2022). Sulforaphane suppresses the growth of EGFR-overexpressing MDA-MB-468 triple-negative breast cancer cells in vivo and in vitro. Int. J. Funct. Nutr. 3 (2), 3–8. doi:10.3892/ijfn.2022.26
Yin, L., Duan, J. J., Bian, X. W., and Yu, S. C. (2020). Triple-negative breast cancer molecular subtyping and treatment progress. Breast Cancer Res. 22 (1), 61. doi:10.1186/s13058-020-01296-5
You, B. J., Wu, Y. C., Lee, C. L., and Lee, H. Z. (2014). Non-homologous end joining pathway is the major route of protection against 4β-hydroxywithanolide E-induced DNA damage in MCF-7 cells. Food Chem. Toxicol. 65, 205–212. doi:10.1016/j.fct.2013.12.026
Yu, J., Zayas, J., Qin, B., and Wang, L. (2019). Targeting DNA methylation for treating triple-negative breast cancer. Pharmacogenomics 20 (16), 1151–1157. doi:10.2217/pgs-2019-0078
Yue, Z., He, S., Wang, J., Jiang, Q., Wang, H., Wu, J., et al. (2023). Glyceollins from soybean: their pharmacological effects and biosynthetic pathways. Heliyon 9 (11), e21874. doi:10.1016/j.heliyon.2023.e21874
Zagami, P., and Carey, L. A. (2022). Triple negative breast cancer: pitfalls and progress. NPJ Breast Cancer 8 (1), 95. doi:10.1038/s41523-022-00468-0
Zeng, Y., and Chen, T. (2019). DNA methylation reprogramming during mammalian development. Genes 10 (4), 257. doi:10.3390/genes10040257
Zhan, H., Tu, S., Zhang, F., Shao, A., and Lin, J. (2020). MicroRNAs and long non-coding RNAs in c-met-regulated cancers. Front. Cell Dev. Biol. 8, 145. doi:10.3389/fcell.2020.00145
Zhang, W., Guan, X., and Tang, J. (2021a). The long non-coding RNA landscape in triple-negative breast cancer. Cell Prolif. 54 (2), e12966. doi:10.1111/cpr.12966
Zhang, Y., Lu, Q., Li, N., Xu, M., Miyamoto, T., and Liu, J. (2022). Sulforaphane suppresses metastasis of triple-negative breast cancer cells by targeting the RAF/MEK/ERK pathway. npj Breast Cancer 8 (1), 40. doi:10.1038/s41523-022-00402-4
Zhang, Y., Ma, X., Li, H., Zhuang, J., Feng, F., Liu, L., et al. (2021c). Identifying the effect of ursolic acid against triple-negative breast cancer: coupling network pharmacology with experiments verification. Front. Pharmacol. 12, 685773. doi:10.3389/fphar.2021.685773
Zhang, Z., Lin, M., Wang, J., Yang, F., Yang, P., Liu, Y., et al. (2021b). Calycosin inhibits breast cancer cell migration and invasion by suppressing EMT via BATF/TGF-β1. Aging (Albany NY) 13 (12), 16009–16023. doi:10.18632/aging.203093
Zhao, L., Tan, S., Zhang, H., Liu, P., Tan, Y. Z., Li, J. C., et al. (2018). Astragalus polysaccharides exerts anti-infective activity by inducing human cathelicidin antimicrobial peptide LL-37 in respiratory epithelial cells. Phyther Res. 32 (8), 1521–1529. doi:10.1002/ptr.6080
Zhao, S., Zuo, W. J., Shao, Z. M., and Jiang, Y. Z. (2020). Molecular subtypes and precision treatment of triple-negative breast cancer. Ann. Transl. Med. 8 (7), 499. doi:10.21037/atm.2020.03.194
Zhou, Y., Yang, J., Chen, C., Li, Z., Chen, Y., Zhang, X., et al. (2021). Polyphyllin Ⅲ-Induced ferroptosis in MDA-MB-231 triple-negative breast cancer cells can Be protected against by KLF4-mediated upregulation of xCT. Front. Pharmacol. 12, 670224. doi:10.3389/fphar.2021.670224
Zhu, S., Wu, Y., Song, B., Yi, M., Yan, Y., Mei, Q., et al. (2022). Recent advances in therapeutic strategies for triple-negative breast cancer. J. Hematol. Oncol. 15 (1), 121. doi:10.1186/s13045-022-01341-0
Keywords: DNA methylation, genes, histone modification, natural drugs, TNBC, epigenetic mechanims
Citation: Kaleem M, Thool M, Dumore NG, Abdulrahman AO, Ahmad W, Almostadi A, Alhashmi MH, Kamal MA and Tabrez S (2024) Management of triple-negative breast cancer by natural compounds through different mechanistic pathways. Front. Genet. 15:1440430. doi: 10.3389/fgene.2024.1440430
Received: 29 May 2024; Accepted: 09 July 2024;
Published: 26 July 2024.
Edited by:
Kai Wang, Harvard Medical School, United StatesReviewed by:
Kun Man, Emory University, United StatesCopyright © 2024 Kaleem, Thool, Dumore, Abdulrahman, Ahmad, Almostadi, Alhashmi, Kamal and Tabrez. This is an open-access article distributed under the terms of the Creative Commons Attribution License (CC BY). The use, distribution or reproduction in other forums is permitted, provided the original author(s) and the copyright owner(s) are credited and that the original publication in this journal is cited, in accordance with accepted academic practice. No use, distribution or reproduction is permitted which does not comply with these terms.
*Correspondence: Shams Tabrez, c2hhbXN0YWJyZXoxQGdtYWlsLmNvbQ==
Disclaimer: All claims expressed in this article are solely those of the authors and do not necessarily represent those of their affiliated organizations, or those of the publisher, the editors and the reviewers. Any product that may be evaluated in this article or claim that may be made by its manufacturer is not guaranteed or endorsed by the publisher.
Research integrity at Frontiers
Learn more about the work of our research integrity team to safeguard the quality of each article we publish.