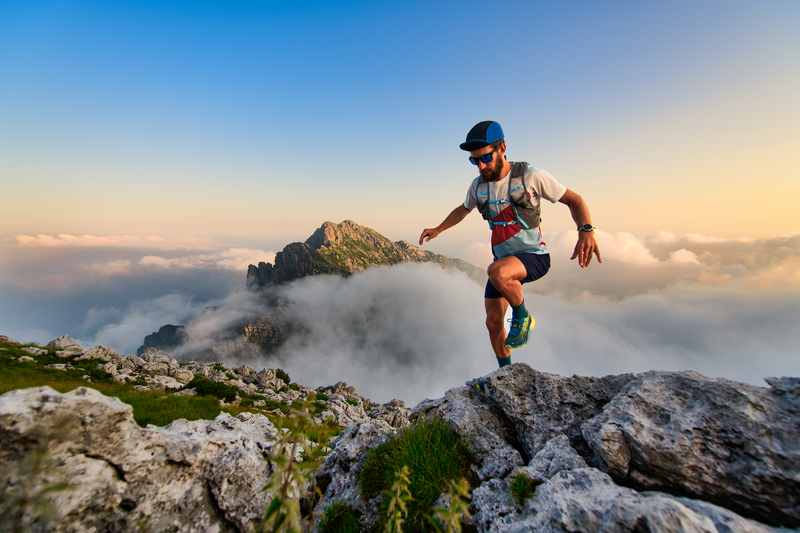
95% of researchers rate our articles as excellent or good
Learn more about the work of our research integrity team to safeguard the quality of each article we publish.
Find out more
ORIGINAL RESEARCH article
Front. Genet. , 10 November 2023
Sec. Genomics of Plants and the Phytoecosystem
Volume 14 - 2023 | https://doi.org/10.3389/fgene.2023.1290492
This article is part of the Research Topic Postharvest Ripening, Senescence, and Technology, Volume II View all 4 articles
Introduction: Aroma is a key inherent quality attributes of pepper fruit, yet the underlying mechanisms of aroma compound biosynthesis remain unclear.
Methods: In this study, the volatile profile of the QH (cultivated Capsicum chinense) and WH (cultivated Capsicum annuum) pepper varieties were putatively identified during fruit development using gas chromatography-mass spectrometry (GC-MS).
Results and discussion: The results identified 203 volatiles in pepper, and most of the esters, terpenes, aldehydes and alcohols were significantly down-regulated with fruit ripening. The comparison of volatile components between varieties revealed that aldehydes and alcohols were highly expressed in the WH fruit, while esters and terpenes with fruity or floral aroma were generally highly accumulated in the QH fruit, providing QH with a fruity odor. Transcriptome analysis demonstrated the close relationship between the synthesis of volatiles and the fatty acid and terpene metabolic pathways, and the high expression of the ADH, AAT and TPS genes was key in determining the accumulation of volatiles in pepper fruit. Furthermore, integrative metabolome and transcriptome analysis revealed that 208 differentially expressed genes were highly correlated with 114 volatiles, and the transcription factors of bHLH, MYB, ARF and IAA were identified as fundamental for the regulation of volatile synthesis in pepper fruit. Our results extend the understanding of the synthesis and accumulation of volatiles in pepper fruit.
Pepper belongs to the Solanaceae family and is one of the most widely cultivated vegetables and spice crops in the world. According to FAO statistics, the cultivated area of pepper reached 1.99 million ha in 2017, with an annual output of 36.1 million tons, and the cultivated area continues to expand (Ge et al., 2020). Pepper fruit is rich in nutrients, including capsaicin, flavonoids, carotenoids and vitamin C, and is favored by consumers due to its bright color, spiciness and aroma (Taiti et al., 2019). Aroma is an important component of pepper fruit flavor, and the loss of aroma will directly affect the sensory quality of the fruit, thereby affecting its economic value. Therefore, it is of great significance to strengthen the identification of volatile aroma components and analyze the molecular mechanism of their biosynthesis for the breeding and improvement of aromatic pepper varieties.
Aroma, a key index for the evaluation of fruit and vegetable quality, originates largely from the volatile organic components (VOCs) released by fruits. To date, over 2000 VOCs have been identified from fruits or flowers, including esters, aldehydes, alcohols, terpenes, phenols, ketones, ethers and other categories (Yang et al., 2019). Moreover, based on the different sensory effects, VOCs can be grouped into fruity aroma, sweet aroma, floral aroma, green aroma, spicy aroma, woody aroma and other types (Dudareva et al., 2006). More than 100 types of VOCs have been identified in pepper, mainly compromising esters, terpenes, alcohols and aldehydes (Kollmannsberger et al., 2011). In a quantitative analysis of volatiles in 10 pepper varieties with different colors, Pino et al. (2007) revealed the content of total volatiles to range from 1.37 to 11.84 mg·kg−1 (dry weight), with orange and brown varieties exhibiting higher levels of esters and a stronger aroma than red varieties. Furthermore, Capsicum chinense, one of the main cultivated species of pepper, is richer in volatiles than other cultivated species. The high accumulation of esters such as hexyl 2-methylbutanoate, heptyl 2-methylpropanoate, hexyl 3-methylbutanoate, hexyl pentanoate, heptyl pentanoate in the fruit of C. chinense result in a strong fruity and floral aroma (Garruti et al., 2013; Taiti et al., 2019). The accumulation of volatiles in pepper fruit is not only specific to the variety, but also varies with the development stage of the same variety. In the early stage of fruit development, esters and alcohols are the main volatile organic components in pepper fruit, but their contents gradually decrease with the development of fruits, accompanied by the increase of alkenes, aldehydes and ketones (Forero et al., 2009).
Volatile organic components are secondary metabolites that are primarily produced by fatty acid and terpene metabolic pathways (Liu et al., 2021). In particular, aldehydes, alcohols and esters are synthesized from fatty acid metabolic pathways. First, glycerides are catalyzed by lipases to form the starting substrate, namely, free fatty acids. The oxidation, cracking and dehydrogenation reactions catalyzed by fatty acid desaturase (FAD), lipoxygenase (LOX), hydroperoxide lyase (HPL) and alcohol dehydrogenase (ADH) are then followed by the production of aldehydes and alcohols from unsaturated fatty acids. Alcohols can be further catalyzed by alcohol acyltransferase (AAT) to form branched-chain esters (Beekwilder et al., 2004). However, the synthesis of lactones is more complicated than that of branched-chain esters. First, fatty acids need to form four or five hydroxy fatty acid intermediates through β-oxidation, and subsequently form lactones under self-cyclization or AAT catalysis (Sánchez et al., 2013). Sarde et al. (2018) identified eight potential LOX genes, of which CaLOX6 and CaLOX7 were predicted to be involved in the biosynthesis of green leaf volatiles. Previous gene expression analysis experiments indicate that the high expression of ADH, HPL, and LOX induced by methyl jasmonate and hexanal promoted the production of α-farnesene and hexanoic acid in fruit (Padmanabhan et al., 2020; Magalhães et al., 2021). In addition to regulating the production of VOCs, LOX has also been reported to participate in abiotic stress, the defense of microbial pathogens and cell death reactions (Lim et al., 2015).
Terpenes are mainly hydrocarbons or oxygenated hydrocarbon derivatives with isoprene structure, and are synthesized through the mevalonic acid pathway (MVA) and methylerythritol phosphate pathway (MEP) (Ramya et al., 2020). Based on the number of isoprenes in the molecule, terpenes are divided into monoterpene (C10), sesquiterpene (C15), diterpene (C20), triterpene (C30), tetraterpene (C40) and polyterpene (n C5, n > 10). Isopentenyl diphosphate (IPP) and diphosphates dimethylallyl diphosphate (DMAPP) are the precursors of all terpenoids. They are produced through the MVA pathway in the cytoplasm and the MEP pathway in the plastid to form various terpenoids catalyzed by terpene synthase (TPS), such as D-limonene, nerolidol, linalool and β-ionone (Zhang et al., 2020). Moreover, some carotenoids such as α-carotene and β-carotene can also be cleaved into sesquiterpene ketones (α-ionone and β-ionone) by carotenoid cleavage dioxygenase (CCD) (Shi et al., 2021). Research on the synthesis of terpenoids in pepper is limited, and only three QTLs (LG3, LG10.1 and LG1) have been associated with the synthesis of terpenoids in pepper fruit, of which LG10.1 and LG1 were identified to be involved in the accumulation of 15 monoterpenes including linalooloxide and p-menth-1-en-9-al (Eggink et al., 2013). It is worth noting that two sesquiterpene synthase (sTPS1 and sTPS2) have been isolated from pepper fruit, yet their involvement in the synthesis of terpenoids requires clarification (Zhang et al., 2019).
As one of the key traits affecting pepper fruit quality, volatiles have been the subject of much research. However, the majority of studies focus on the identification of volatiles during postharvest processing, while research on the synthesis and regulation mechanism of volatiles during fruit development is limited. In recent years, with the continuous development of biological technology, multi-omics analysis has become one of the most effective methods for the analysis of plant physiological processes (Liu et al., 2020; Shi et al., 2021). In this study, two pepper varieties QH and WH, which are popular in Hunan, China, were identified as the experimental materials. Fruit of QH is wide and lantern-shaped, with strong aroma and high capsaicin, and is widely used for sauce, while fruit of WH is short and narrow, with poor aroma but moderately spicy, and is mainly used for fresh eating. We employed headspace solid-phase microextraction-gas chromatography-mass spectrometry (HS-SPME-GC-MS) to identify volatiles in two pepper varieties during fruit development, and subsequently adopted transcriptome sequencing technology to screen differentially expressed genes (DEGs) in these varieties. Finally, correlation analysis between transcripts and VOCs was performed to identify key genes involved in VOC biosynthesis.
Two pepper varieties QH (C. chinense with a strong fruity aroma) and WH (Capsicum annuum without a fruity aroma) were selected as the research materials for this experiment (Figure 1A). After standardized seedling raising, the two pepper varieties were transplanted to the biological garden of Hengyang Normal University (Hengyang City, Hunan Province, China) for routine cultivation and management in March 2021. The peppers were sampled at 30 days after anthesis (30 DAA) and 50 days after anthesis (50 DAA). Each sample consisted of six fleshes from three plants (two fleshes per plant). The collected flesh was immediately frozen in liquid nitrogen for subsequent volatile detection (six biological replicates), transcriptome sequencing (three biological replicates) and qRT-PCR analysis.
FIGURE 1. Fruit phenotype, types of volatiles and number of differentially expressed volatiles (DEVs) and differentially expressed genes (DEGs) between two pepper varieties. (A) Fruit phenotype of QH and WH varieties at 30 days after anthesis (DAA) and 50 DAA stages. (B) Volatile organic compounds detected in two pepper varieties and their classification. The number of DEVs (C) and DEGs (D) between two pepper varieties and two fruit developmental stages. QH-G, fruit samples of QH varieties at 30 DAA, QH-M, fruit samples of QH varieties at 50 DAA, WH-G, fruit samples of QH varieties at 30 DAA, WH-M, fruit samples of QH varieties at 50 DAA.
Pepper fruit fleshes were weighed and ground to a powder in liquid nitrogen. Solid phase micro extraction (SPME) was used to extract the volatile compounds of fruit with six biological replicates for each sample. The fruit fleshes (500 ± 1 mg) was placed in a 20 mL headspace bottle, and 10 μL 2-octanol (100 mg/L stock in dH2O) was then added as an internal standard. The SPME cycle of the CTC rail system adopted the following analytical conditions: incubate temperature of 50°C, preheat time of 15 min, incubate time of 30 min, and desorption time of 4 min. Subsequently, samples were directly injected into the injection port of a GC-MS.
The volatile organic compounds of the pepper fruit were detected as described previously (Li et al., 2021a) with some modifications. The Agilent 7890 gas chromatograph system combined with 5975C mass spectrometer was employed to identify and quantify VOCs. Volatile analysis was performed using a HP-INNOWax capillary column (30 m × 250 μm × 0.25 μm, J&W Scientific, Folsom, CA, United States), with splitless mode used for the injection at 260°C. Helium (Purity ≥ 99.999%) was used as the carrier gas for the GC-MS operation, with a front inlet purge flow of 3 mL·min−1 and gas flow rate through the column of 1 mL·min−1. The initial oven temperature was maintained at 40°C for 1 min, raised to 250°C at a rate of 5°C·min−1, and maintained for 5 min at 250°C. The transfer line temperature, ion source temperature and quad temperatures were 260°C, 230°C, and 150°C, respectively. The energy of electron impact (EI) was −70 eV. The mass spectrometry data were acquired in scan mode with the m/z range of 20–400 and solvent delay of 0 min. Chroma TOF 4.3X (LECO Corporation) and the NIST and NCBI databases were used for the raw peak extraction, data baselines filtering, baseline calibration, peak alignment, deconvolution analysis, spectrum matching, peak identification and peak area integration. VOCs with MS matching scores greater than 700 were retained, and the final substance type was determined after manual identification and comparison. Furthermore, the identified VOCs were subjected to orthogonal partial least squares discriminant analysis (OPLS-DA), and VOCs with | Log2 (fold change) | ≥1 and p-value <0.05 were considered as differentially expressed volatiles (DEVs).
The total RNA was extracted from frozen fleshes. The integrity and total amount of RNA were accurately detected with an Agilent 2100 bioanalyzer (Agilent Technologies, CA, United States). The qualified RNA was used to construct a sequencing library. A total amount of 3 µg RNA per sample was used as input material for the RNA sample preparations. Sequencing libraries were generated using NEBNex NEBNext® UltraTM RNA Library Prep Kit for Illumin Illumina® (NEB, United States) following manufacturer’s recommendations and index codes were added to attribute sequences to each sample. Subsequently, the quality of the library was evaluated on the Agilent Bioanalyzer 2100 system (Agilent Technologies, CA, United States), and the qualified library was sequenced on the Illumina HiSeq 4000 platform. After sequencing, the adaptor and low-quality sequences were removed using Fastp software with default parameters, and then the obtained clean reads were aligned to the Zunla reference genome using Hisat2 (Chen et al., 2018). Following this, based on the gene length, the expected number of fragments per kilobase of transcript sequence per million base pairs sequenced (FPKM) value of each gene was calculated to determine the expression level of the corresponding gene. Finally, differential expression analysis between sample groups was performed with DESeq2 software (Love et al., 2014). Genes satisfying | log2Fold Change | ≥ 1 and False Discovery Rate (FDR) < 0.05 were defined as differentially expressed genes (DEGs), which were further analyzed using the Kyoto Encyclopedia of Genes and Genomes (KEGG) database.
The Pearson correlation coefficient was calculated for metabolome and transcriptome data integration. To reduce the false positive rate and accurately obtain the key genes regulating the synthesis of volatiles, differentially expressed genes with a FPKM value below 10 in all samples and | log2Fold Change | < 2 were filtered out. Previous studies have shown that the terpene metabolism pathway, fatty acid metabolism, plant hormone, cell signaling and transcription factors are key to determining the synthesis of plant volatiles (Ramya et al., 2020; Shi et al., 2021). Therefore, the differentially expressed genes (FPKM >10 and | log2Fold Change | > 2) in the aforementioned factors were used to calculate the Pearson correlation coefficient with volatiles with the “Wu Kong” platform (https://www.omicsolution.com/wkomics/main/). Correlations greater than 0.85 were selected and the relationships between the DEGs and VOCs were visualized using Cytoscape (version 3.7.1, Cytoscape Team).
Total RNA was extracted from the fleshe of QH and WH using a MAGEN RNA Extraction Kit (Majorbio, China). The purified RNA was reverse transcribed into the first strand cDNA using the HiScript II 1st Strand cDNA Synthesis Kit (Vazyme, China). Synthesized cDNA was used as a template for qRT-PCR with a Real Master Mix (SYBR Green) kit (Vazyme, China). Nine genes related to volatile synthesis were selected for qRT-PCR analysis, with the β-actin gene used as the reference gene to correct gene expression. All primers used in this study are listed in Supplementary Table S1. qRT-PCR was conducted in a total volume of 20 µL containing 10 µL of mix, 0.4 µL of forward primer, 0.4 µL of reverse primer, 2 µL of cDNA, and 7.2 µL distilled water. Reactions were carried out on the LightCycler® 96 Real-Time PCR detection system (Roche, Switzerland) with default parameters. Three replicates were performed for each sample. The relative expressions of target genes were calculated using the 2−ΔΔCT method.
The volatile organic compounds and relative changes in content of the QH and WH varieties were putatively identified during fruit development. A total of 203 VOCs were detected in the two pepper varieties, including 62 esters (31%), 28 terpenoids (14%), 24 aldehydes (12%), 16 alkanes (8%), 14 alkenes (7%), 13 alcohols (6%), 12 ketones (6%), 12 acids (6%), 12 aromatic compounds (6%), 5 furans (2%) and 5 miscellaneous (2%) (Figure 1B, Supplementary Figure S1; Supplementary Table S2). The hierarchal clustering of the VOC profile showed that the 203 volatiles could generally be classified into three categories (Supplementary Figure S2). The first cluster contained 76 VOCs, such as esters, terpenes and alkenes, which are mainly accumulated in the QH variety. The second cluster was composed of 14 VOCs including terpenoids, alkenes and aromatic compounds, which were highly expressed in the 50 DAA stage of the WH variety, showing significant variety and time specificity. The 113 VOCs (including aldehydes, alcohols and terpenes) in the third cluster exhibited a higher accumulation in the 30 DAA stage of the WH variety (Supplementary Figure S2). Thus, the differences in the contents of esters, terpenes, aldehydes and alcohols between the two pepper cultivars were responsible for the differences in fruit odor.
Pairwise comparisons were performed between QH and WH at each developmental stage. In the 30 DAA and 50 DAA stages, 71 (43 upregulated, 28 downregulated) and 60 (33 upregulated, 27 downregulated) DEVs were detected between the QH and WH varieties, respectively (Figure 1C and Supplementary Table S3). After removing the redundancy, a total of 91 DEVs were detected between the two varieties, including esters (34), aldehydes (16) and terpenes (11) (Supplementary Table S4). To visually compare the differences of VOCs between the two varieties, hierarchical clustering was performed on the aforementioned 91 DEVs. As shown in Figure 2, a total of 91 DEVs were clustered into six groups (A, B, C, D, E and F) in the heatmap dendrogram (Supplementary Table S4). Group A consisted of 37 DEVs, including esters (21), acids (5) and terpenes (4). The terpenes and esters in group A, such as hexyl 2-methylbutyrate, 3-methylbutyric acid hexyl ester (hexyl 3-methylbutanoate), isopentyl isopentanoate, isobutyl isovalerate, oxacyclotetradecan-2-one, hexyl caprylate, (E)-β-famesene, caryophyllene, β-elemen and α-cadinene, exhibited a higher accumulation in the QH variety (Figure 2A and Supplementary Table S4). Consistent with group A, the esters were the main components in group B, with the exception of 4-methylpentyl isovalerate and cis-3-hexenyl 2-methylbutanoate, which were highly accumulated in the WH variety. Aldehydes and alcohols were the dominant components of group C, with nine aldehydes (methacrolein, 2-propenal, isovaleraldehyde, isobutyraldehyde, 3-methyl-2-butenal, trans-2-pentenal, hexadecanal, propanal and butanal) and two alcohols (3-methyl-1-butanol and benzyl alcohol) exhibiting a higher content in the WH fruit (Figure 2A and Supplementary Table S4). Group D, consisting of seven compounds (E-2-hexadecacen-1-ol, benzaldehyde, 7-tetradecene, (E, E)-2,4-heptadienal, hexanal, (+)-a-longipinene and 2-methyl-4-hexanone), presented a high content in the QH and WH fruit at 30 DAA, while at 50 DAA they were not detected in the QH (Figure 2A and Supplementary Table S4). Group E consisted of 6-methylheptyl 3-methylbutanoate, octyl-3-methylbutanoate, 6-methylhept-4-en-1-yl 3-methylbutanoate, (+)-Δ-cadinene, α-calacorene and cis-9-Hexadecenal, which were abundant in the QH and WH fruit at 30 DAA, but not detected in the WH fruit at 50 DAA (Figure 2A and Supplementary Table S4). Note that 15 VOCs in group F, such as 5-ethylcyclopent-1-enecarboxaldehyde, (E, Z)-2,6-nonadienal, perillen, pentanoic acid, acetylacetone, geranylacetone and pentanoic acid, were also highly expressed in the WH variety, 12 of which were not detected in the QH variety (Figure 2A and Supplementary Table S4).
FIGURE 2. Heat map of differentially expressed volatiles. (A) Heat map of differentially expressed volatiles between varieties. (B) Heat map of differentially expressed volatiles between developmental stages. QH-G, fruit samples of QH varieties at 30 DAA, QH-M, fruit samples of QH varieties at 50 DAA, WH-G, fruit samples of QH varieties at 30 DAA, WH-M, fruit samples of QH varieties at 50 DAA.
During fruit development, 51 DEVs (48 downregulated and 3 upregulated) and 50 DEVs (49 downregulated and 1 upregulated) were detected in the WH and QH varieties between 30 DAA and 50 DAA, respectively (Figure 1C and Supplementary Table S3). After removing redundancy, a total of 88 DEVs were detected in the two pepper varieties between the two fruit development stages, including esters (32), aldehydes (15), alkenes (9), terpenes (8) (Supplementary Table S4). These DEVs were downregulated during fruit development, with the exception of 1-methylpyrrole, propanoic acid, perillen and acetylacetone (Supplementary Table S4). The hierarchical clustering of the DEVs showed that 88 volatiles could be divided into four groups (groups I, II, III, and VI) (Figure 2B and Supplementary Table S4). Group I contained 19 volatiles, of which aldehydes, alcohols and ketones were the main components. During fruit development, the volatiles of group I were significantly downregulated in the QH variety, while 16 of which were not detected in the QH fruit at 50 DAA (Figure 2B and Supplementary Table S4). In contrast to group I, the content of nine volatiles in group II decreased significantly in the WH fruit during fruit development, with (Z)-2-heptenal, (R)-β-himachalene, 6-methylheptyl 3-methylbutanoate, 2-methyl-1-propyl butyrate, resorcinol monobenzoate, (+)-a-longipinene and α-calacorene undetected in the WH fruit at 50 DAA (Figure 2B and Supplementary Table S4). The group C (26 members) and group D (34 members) volatiles exhibited a similar accumulation trend during the fruit development of the QH and WH varieties (Figure 2B and Supplementary Table S4). In the early stage of fruit development, the volatiles of groups C and D were highly abundant in the fruit of QH and WH, yet their content decreased slightly with fruit ripening.
The fruit samples of the two varieties were subjected to RNA-Seq analysis, with each sample run in triplicate. A total of 12 libraries were constructed, yielding 88.84 GB of clean reads (Supplementary Table S5). These clean reads were mapped to the reference genome with match ratios in the range of 88.94%–94.96%, and 26,366 genes predicted from the genome were found to be expressed in at least one sample (with FPKM >0) (Supplementary Tables S5, S6).
To further screen the genes related to the formation of fruit volatiles, the differential expression analysis of genes was performed. At 30 DAA and 50 DAA, 5330 (2304 upregulated, 3026 downregulated) and 6385 (3508 upregulated, 2877 downregulated) DEGs were detected between QH and WH, respectively (Figure 1D and Supplementary Table S7). After removing redundancy, a total of 8,814 DEGs (differentially expressed at least one developmental stage) were identified between the two varieties, 2901 of which were differentially expressed at 30 DAA and 50 DAA (Supplementary Figure S3). KEGG enrichment analysis identified “fatty acid metabolism”, “fatty acid degradation”, “fatty acid biosynthesis”, “peroxisome”, “carbon metabolism” and “a-linolenic acid metabolism” as the six most significant pathways enriched with the DEGs at 30 DAA (Figure 3A). In 50 DAA, the DEGs were concentrated in “ubiquinone and other terpenoid-quinone biosynthesis”, “biosynthesis of amino acids”, “carbon metabolism”, “fatty acid metabolism”, and “pyruvate metabolism” (Figure 3B). Thus, the significant enrichment of DEGs in the fatty acid and terpene metabolic pathways may be responsible for the large differences in the fatty acid and terpene contents between QH and WH.
FIGURE 3. Enrichment pathway of differentially expressed genes. (A) Top 20 enriched pathways of differentially expressed genes between QH and WH varieties at 30 days after anthesis. (B) Top 20 enriched pathways of differentially expressed genes between QH and WH varieties at 50 days after anthesis.
During fruit development, 9083 (3362 upregulated, 5721 downregulated) and 8,727 (3666 upregulated, 5061 upregulated) DEGs were detected in the WH and QH varieties, respectively (Figure 1D and Supplementary Table S7). After removing redundancy, 12,655 genes were differentially expressed in at least one variety, and 5155 genes were differentially expressed in both QH and WH during fruit development (Supplementary Figure S3). To identify the significantly enriched pathways, the DEGs of QH and WH during fruit development were mapped to the KEGG database. The results showed that the DEGs of QH were mainly enriched in “fatty acid biosynthesis”, “biosynthesis of amino acids”, “carbon metabolism” and “pyruvate metabolism”, while those of WH were mainly enriched in “ascorbate and aldarate metabolism”, “photosynthesis”, “sulfur metabolism” and “carbon metabolism” (Supplementary Figure S4).
Linoleic acid and linolenic acid are precursors for the synthesis of branched aliphatic alcohols, aldehydes, ketones and esters. In addition, linoleic acid can be converted into linolenic acid by fatty acid desaturase (FAD) (Liu et al., 2021). Four FADs were identified in QH and WH, and their expression patterns varied with the variety and developmental stage. FAD1 was highly expressed at 30 DAA, while FAD2 was highly expressed at 50 DAA. The remaining two FAD genes (FAD3 and FAD4) exhibited significant specificity to the variety, and were only highly expressed at the 30 DAA and 50 DAA stages of QH, respectively (Figure 4 and Supplementary Table S8). Lipoxygenases (LOX) and hydroperoxide lyase (HPL) are key enzymes in the biosynthesis of aldehydes, and three LOXs (LOX1, LOX2, and LOX4) and two HPLs (HPL1 and HPL2) were highly expressed in the WH fruit at the 30 DAA stage. This could be directly related to the high accumulation of aldehydes (e.g., hexanal, propanal, butanal, benzaldehyde, (E, E) −2,4-heptadienal and (E, Z) −2,6-nonadienal) in the WH fruit (Figure 4 and Supplementary Table S8). The aldehydes can be further converted into alcohols by alcohol dehydrogenase (ADH) (Wang et al., 2022a). In the WH fruit, the expressions of ADH1, ADH3, ADH4, and ADH5 exceeded those in QH, while the reverse was true for ADH2 and ADH7 (Figure 4 and Supplementary Table S8). This may be the reason for the differential accumulation of 3-methyl-1-butanol, (2E) -2-hexadecen-1-ol, 1-pentanol, 4-methyl-1-pentanol, benzyl alcohol and 1-hexanol in the two varieties. Alcohol acyltransferase (AAT) are the rate limiting enzymes for ester biosynthesis. Here we identified four AAT genes, which were differentially expressed between varieties and developmental stages. In the early stage of fruit development, AAT1, AAT2, and AAT3 were highly expressed in the QH and WH varieties, ensuring the accumulation of esters in the WH and QH fruit at 30 DAA, such as hexyl 2-methyl butyrate, isoamyl hexanoate, hexyl acetate and trans-2-hexenyl butyrate (Figure 4 and Supplementary Table S8). However, note that the AAT4 gene exhibited the highest transcriptional abundance in the WH fruit at 50 DAA, while the ester content was extremely low in this variety at 50 DAA.
FIGURE 4. Volatile aldehyde, alcohol and ester biosynthesis in two pepper varieties fruit. Only esters, aldehydes and aldehydes with the most significant differential expression were displayed due to limited space. FAD, fatty acid desaturase, LOX, lipoxygenases, HPL, hydroperoxide lyase, ADH, alcohol dehydrogenase, AAT, alcohol acyltransferase.
Terpenes are a large group of plant aromatic components and are mainly synthesized through the mevalonic acid (MVA) pathway in the cytoplasm and the methylerythritol phosphate (MEP) pathway in the plastids (Shi et al., 2021). During fruit development, the transcriptional abundance of 3-hydroxy-3-methylglutaryl-CoA synthase 1(HMGS1), 3-hydroxy-3-methylglutaryl-CoA reductase 5 (HMGR5), phosphomevalonate kinase 1 (PMK1) and mevalonate 5-diphosphate decarboxylase (MDD) decreased by varying degrees in the two pepper varieties, and the expression level of HMGS1 in WH and QH at 30 DAA was 5.9 and 12.3 times higher than that at 50 DAA, respectively (Figure 5 and Supplementary Table S8). The expression levels of genes in the MVA pathway are not only different during fruit development, but also vary greatly among varieties. The transcription levels of acetoacetyl-CoA thiolase 2 (AACT2), HMGR1 and PMK1 in QH were significantly higher than those in WH at the 30 DAA stage, while the transcription levels of HMGR1 and HMGR5 in QH were lower than those in WH at 50 DAA (Figure 5 and Supplementary Table S8). In addition, four upstream structural genes (1-deoxy-d-xylulose 5-phosphate synthase 1(DXS1), DXS4, 1-deoxy-d-xylulose 5-phosphate reductoisomerase (DXR) and CDP-ME synthase (CMS) in the MEP were downregulated during fruit development, thus reducing the flux into the terpene synthesis pathway with the decrease in terpenes content in the pepper fruit at 50 DAA. Geranyl pyrophosphate synthase (GPPS) is a key downstream gene in the terpene pathway that is able to convert isopentenyl pyrophosphate (IPP) or dimethyl allyl pyrophosphate (DMAPP) into geranylgeranyl pyrophosphate (GPP), the precursor of terpene synthesis (Zhang et al., 2020). We identified four GPPS genes (GPPS1-GPPS4), of which GPPS1, GPPS2 and GPPS3 exhibited a high transcriptional abundance in QH, while GPPS4 was highly expressed in WH (Figure 5 and Supplementary Table S8).
FIGURE 5. Volatile terpenoid biosynthesis in two pepper varieties fruit. Terpenoid volatile organic compounds are synthesized by the cytosolic mevalonic acid (MVA) and plastidial methylerythritol phosphate (MEP) pathways. Differentially expressed genes and volatiles were integrated into pathway-based maps. Dashed arrows indicate unverified steps in the pathway. HMG-CoA, 3-hydroxy-3-methylglutaryl coenzyme A, MVP, 5-phosphomevalonate, MVPP, 5-diphosphomevalonate, IPP, isopentenyl diphosphate, DMAPP, dimethylallyl diphosphate, DXP, 1-Deoxy-D-xylulose 5-phosphate, CDP-ME, 4-(cytidine 5′-diphospho)-2-C-methyl-D-erythritol, CDP-MEP, CDP ME 2-phosphate, ME-CPP, 2-C-methyl-D-erythritol 2,4-cyclodiphosphate, HBMPP, hydroxymethyl-butenyl 4-diphosphate, FPP, farnesyl diphosphate, GPP, geranyl diphosphate, AACT, acetoacetyl-CoA thiolase, HMGS, 3-hydroxy-3-methylglutaryl-CoA synthase, HMGR, HMG-CoA reductase, MVK, mevalonate kinase, PMK, phosphomevalonate kinase, MDD: Mevalonate 5-diphosphate decarboxylase, IDI, isopentenyl diphosphate isomerase, DXS, 1-deoxy-d-xylulose 5-phosphate synthase, DXR, 1-deoxy-d-xylulose 5-phosphate reductoisomerase, CMS, CDP-ME synthase, CMK, CDP-ME kinase, MCS, ME-CPP synthase, HDS, hydroxymethyl-butenyl 4-diphosphate synthase, FPPS, farnesyl pyrophosphate synthase, GPPS, geranyl pyrophosphate synthase, TPS, terpene synthase.
Terpene synthase (TPS) is a large family that determines the synthesis of different terpenoids in plants. In this study, 13 genes were predicted to encode TPS, and their transcription showed significant variety specificity. Seven TPS (TPS1-TPS6 and TPS12) had the highest transcription abundance in QH, while the remaining six TPS (TPS7-TPS11 and TPS13) were highly expressed in WH (Figure 5 and Supplementary Table S8). Note that the accumulation of terpenes also exhibited significant variety specificity. Monoterpenes, such as 3-carene, 3-thujene, eucalyptol, terpinolene and perillen, were mainly accumulated in WH, while sesquiterpenes, such as β-elemen, β-caryophyllene, (E)-β-famesene, (+)-Δ-cadinene, α-cadinene, α-calacoren and (+)-α-longipinene, were highly expressed in QH (Figure 5 and Supplementary Table S8). Therefore, the consistency of the TPS expression level and terpenoid content implies that TPS1-TPS6 and TPS12 may be mainly involved in the synthesis of sesquiterpenes in pepper fruit, while the remaining six TPS genes (TPS7-TPS11 and TPS13) may be primarily responsible for the synthesis of monoterpenes.
To understand the regulation mechanism of volatile biosynthesis, we performed correlation tests between the quantitative changes of metabolites and transcripts in the QH and WH pepper varieties. A total of 203 VOCs and 356 DEGs (43 for terpene metabolism, 43 for fatty acid metabolism, 50 for hormone metabolism, 44 for cell signaling, 176 for transcription factors, Supplementary Table S9) were subjected to Pearson correlation analysis. A total of 114 VOCs were strongly correlated with 208 DEGs (PCC > 0.85). These 114 volatiles were grouped into six groups (groups A-F) and the 208 genes were divided into six clusters (clusters I-VI) (Figure 6 and Supplementary Table S10).
FIGURE 6. The correlation networks of volatiles and differentially expressed genes (FPKM >10 and | log2Fold Change | > 2) in terpenes metabolism, fatty acids metabolism, hormones metabolism, cell signaling and transcription factors. Triangle, fatty acid metabolism genes (blue), Hexagon, Terpene metabolism genes (orange), Rectangle, cell signaling genes (purplish red), Rhombus, transcription factors (green), Parallelogram, hormones metabolism genes (pink).
Group A, consisting of 14 terpenoids, exhibited a strong correlation with the cluster II gene. In cluster II, 13 terpene metabolism-related genes (GGPS2, D27, CCD7, CCD4, DLAT5, PKP2, AAO3-like, TPS, TPS2, TPS3, TPS6, and TPS11) had a high correlation with the VOCs in group A, contributing 26.3% to the correlation in group C (Figure 6). CCD is a structural gene in the carotenoid metabolic pathway that can cleave carotenoids into low-carbon terpenes (Shi et al., 2021). In cluster II, CCD4 and CCD7 were strongly correlated with (+)-α-longipinene and α-ionol (PCC >0.9), indicating the possible key roles of these genes in the production of (+)-α-longipinene and α-ionol (Figure 6). Moreover, six TPS genes (TPS1, TPS2, TPS3, TPS6, TPS11, and TPS12) were identified in cluster II, but their associated volatile components were different. TPS1, TPS2, TPS3, TPS6, and TPS12 mainly interacted with α-ionol, β-ionone and (+)-α-longipinene, while TPS11 was associated with geranylacetone, (E, E) −2,4-heptadienal and (E, Z) −2,6-nonadienal (Figure 6), furthering proving that these six TPS genes have potential functions in regulating terpene synthesis. In addition to terpene metabolism genes, 27 transcription factors, such as bHLH142, bHLH147, bZIP9, MYB1, MYB2, MYB306, MYB35, and WRKY4, were also identified to have a strong correlation with geranylacetone, (E, Z)-2,6-nonadienal, perillen, (+)-α-longipinene and (E, E)-2,4-heptadienal (Figure 6).
Group B, consisting of six alkanes and five alkenes, was mainly related to cluster III and cluster VI genes (Figure 6). Fatty acid metabolism-related genes were significantly enriched in cluster III, and nine genes, including ADH2, acetyl-CoA acyltransferase 1 (ACAA1), β-ketoacyl-CoA synthase 1 (KCS1) and KCS11, were highly correlated with the volatiles in group B, contributing 26.6% correlation of group B. In cluster VI, transcription and hormone response factors including bHLH162, ARF8, ARF12, ARF8-like, TCP14, GATA4, C2H2-6, and REV were associated with group B volatiles, and were only correlated with 2-methyldodecane (Figure 6). Group C contained seven ketones and five acids, which were mainly related to the genes in clusters I and VI. Among them, 2,3-pentanedione and 2-methyl-4-hexanone were superior, exhibiting strong correlations with 41 and 31 genes, respectively, including nine auxin response-related transcription factors or proteins, three bHLH transcription factors, three WRKY transcription factors and three β-ketoacyl-CoA synthases (Figure 6).
Alcohols and aldehydes (group D, 21 members) were closely related to the genes of clusters IV, V and VI. Among the alcohol volatiles, 2-butanol and 1-hexanol were highly correlated with 56 and 22 differentially expressed genes, respectively, including sixteen fatty acid synthesis genes, three ARF (ARF3, ARF5, and ARF5-like), three WRKY (WRKY21, WRKY23, and WRKY6), four bHLH (bHLH143, bHLH48, bHLH68, and bHLH79) and five MYB (MYB3, MYB306, MYB86, MYB1R1, and EFM) transcription factors (Figure 6). Aldehydes were the main components of group D, seven of which (propanal, methacrolein, 2-butenal, (Z)-2-heptenal, 5-ethylcyclopent-1-enecarboxaldehyde, ben-zaldehyde and heptadecanal) were strongly correlated with more than 20 DEGs, respectively, contributing 55.6% to the correlation in group D. After removing redundancy, seven aldehydes interacted with 95 differentially expressed genes, including 14 fatty acid metabolism related genes, eight bHLH transcription factors (bHLH142, bHLH143, bHLH147, bHLH162, bHLH48, bHLH62, bHLH68, and bHLH79), and 17 abscisic acid or auxin response factors [ARF12, ARF3, ARF5-like, ARF6, ARF8, ARF8-like, ARF8-like2, IAA16, IAA27, IAA27-like, IAA4-like, SAUR52, GH3-1, PYL4 (abscisic acid receptor PYL4), PYL8, PYL9, and ABF1] (Figure 6). Consistent with group D, group E, consisted of four furans, four aromatic compounds and two miscellaneous volatiles, and also exhibited a strong correlation with the cluster VI genes. Fatty acid synthesis genes (KCS1, KCS10, KCS11, and KCS11-like), auxin response factors (ARF12, ARF3, ARF6, ARF8, ARF8-like, IAA16, IAA27, IAA27-like, IAA29, and IAA4-like) and bHLH transcription factors (bHLH142, bHLH143, bHLH147, bHLH162, bHLH62, and bHLH79) were the main genes interacting with the group E volatiles (Figure 6).
Esters (group F, 46 members) were mainly related to genes in cluster Ⅲ and cluster Ⅵ. In cluster III, fatty acid synthesis genes were dominant components, and were highly correlated with multiple esters. For example, KCS1 gene was associated with 14 esters, such as cis-3-hexenyl hexanoate, hexyl acetate, isopentyl hexanoate and hexyl 2-methylbutyrate (Figure 6). Furthermore, 20 transcription factors in cluster VI, including four homeobox-leucine zipper proteins (ATB13, ATHB16, ATHB16-like, and REV), three bHLH transcription factors (bHLH162, bHLH62, and bHLH79) and one MYB transcription factor (MYB50), were identified to interact with esters such as isoamyl propionate, isobutyl isobutyrate and benzyl isobutyrate (Figure 6).
To confirm the integrity of the RNA-Seq results, qRT-PCR experiments were performed on 9 selected genes (including six genes in the terpene or fatty acid metabolism pathways and three transcription factors) in the two varieties at 30 DAA and 50 DAA. The expression patterns of these genes were consistent with the RNA-seq results during fruit development (Supplementary Figure S5), indicating that the RNA data are valid and reliable.
Aroma is an important quality characteristic of fruits and is mainly determined by the type and content of volatile substances in fruits (Dudareva et al., 2006). The volatile substances in pepper fruit are rich in variety, with esters, terpenes, aldehydes and alcohols as the dominant types (Kollmannsberger et al., 2011). In this study, the accumulation of esters, terpenes, alcohols and aldehydes showed obvious variety specificity. Esters and terpenoids including hexyl 2-methylbutyrate, 3-methylbutyric acid hexyl ester, 4-methylpentyl isovalerate, isopentyl isopentanoate, hexyl caprylate, (E)-β-famesene, β-elemen and α-cadinene were mainly accumulated in C. chinense QH. Furthermore, aldehydes and alcohols such as methacrolein, 2-propenal, isovaleraldehyde, isobutyraldehyde, 3-methyl-2-butenal, trans-2-pentenal, propanal, butanal, hexanal, 4-methyl-1-pentanol and 3-methyl-1-butanol were the dominant components of volatiles in WH fruit. Previous studies have shown that esters and terpenes are the main aroma contributors in C. chinense cultivars. Esters such as hexyl 2-methylbutyrate, 3-methylbutyric acid hexyl ester, 4-methylpentyl isovalerate and methyl salicylate have been identified as marker aroma compounds, leading to fruity aromas in the fruit of C. chinense cultivars (Heng et al., 2023; Huang et al., 2023). At present, a few aldehydes and alcohols have been identified as marker aroma compounds. (E)-2-hexenal and (Z)-3-hexenal have been reported to provide cherry tomatoes with strong green grass and green leaf odors (Selli et al., 2014). Moreover, the accumulation of aldehydes and alcohols induce the grassy aroma of immature peach fruit (Zhang et al., 2010; Niu et al., 2021). In summary, the large accumulation of esters and terpenoids with fruit aroma in QH fruit can explain the release of fruit aroma by QH fruit. However, although WH fruit exhibited high aldehyde and alcohol contents, the fruit did not emit green aroma. This may be because the content of these aldehydes and alcohols had not yet reached the corresponding aroma threshold. As the fruit developed, the type and content of volatiles in the QH and WH pepper varieties decreased to varying degrees. This agrees with Heng et al. (2023), who determined that the total volatile content decreased as the fruit changed from green to red. Therefore, compared with the physiological maturity stage, pepper fruit in the green maturity stage has a higher content of volatile substances such as esters, terpenes and aldehydes, and its fruit aroma and green grass odor are stronger. These properties may be more suitable for consumption.
The fatty acid metabolism pathway mainly includes four genes, namely, LOX, HPL, ADH, and AAT. The expressions of these genes play key roles in the synthesis of aldehydes, alcohols and esters (Beekwilder et al., 2004). LOX and HPL are two enzymes in the upstream of the fatty acid metabolism pathway, which can convert the precursor substances linoleic acid and linolenic acid into aldehydes through a two-step oxidation reaction (Huang et al., 2023). Recent studies have shown that four LOX genes (MdLOX1-MdLOX4) are positively correlated with the synthesis of aldehyde volatiles in apple (Liu et al., 2021). HPL is the last enzyme in the biosynthesis of aldehydes, and the loss of its function will directly affect the synthesis of aldehydes and alcohols. In Nicotiana attenuate, silencing the NaHPL gene reduced the release of (Z)-3-hexenal (Halitschke et al., 2004). The loss of the HPL13 function led to a strong decrease in the total content of C6 volatiles in olive, accompanied by a decrease in the accumulation of hexanol, hexanal and (E)-2-hexanol (Cerezo et al., 2021). In this study, three LOX (LOX1, LOX2 and LOX4) and two HPL (HPL1 and HPL2) genes were highly expressed in the WH fruit at 30 DAA. This is consistent with the higher aldehyde content in WH fruit compared to QH fruit during fruit development, indicating that the aforementioned LOX and HPL genes play a key positive regulatory role in the biosynthesis of aldehydes. The aldehydes produced in the fatty acid and isoleucine metabolic pathways can be further converted into alcohols by alcohol dehydrogenase (ADH) (Wang et al., 2022b). Huang et al. (2023) detected 11 differentially expressed ADH genes in pepper, nine of which were transcribed at a higher level in C. chinense than in the C. annuum cultivated species. In this study, seven differentially expressed ADH genes were identified, but only ADH2 and ADH7 were highly expressed in the QH variety, while the transcription levels of the ADH1, ADH3, ADH4, and ADH5 genes in QH were lower than those in WH. Moreover, we also observed that benzyl alcohol and 1-hexanol were highly accumulated in QH, while 3-methyl-1-butanol, (2E) -2-hexadecen-1-ol, 1-pentanol and 4-methyl-1-pentanol were highly accumulated in WH. This suggests that the ADH family members may have functional differentiation during evolution, and each family member may be responsible for the synthesis of different alcohols. Similar to the expression pattern of the ADH gene, four AAT genes were differentially expressed between the varieties, accompanied by the differential accumulation of esters. Terpene synthase determines the synthesis of different terpenoids in plants. In citrus, 95 genes were identified as potential TPS genes, of which the STPS genes were involved in the synthesis of monoterpenes, such as sabinene, D-limonene, linalool, trans-β-ocimene, β-myrcene and α-pinene (Zhang et al., 2020). The MdAFS1 gene (terpene synthases) was reported to be responsible for the synthesis of sesquiterpene α-farnesene in apple (Liu et al., 2021). In this study, 13 differentially expressed TPS genes were identified. Among them, the expression pattern of seven TPSs (TPS1-TPS6 and TPS12) was consistent with the accumulation pattern of sesquiterpenes, all of which had a higher transcriptional abundance or content in the QH variety. However, the remaining six TPSs (TPS7-TPS11 and TPS13) were highly expressed in WH, accompanied by high monoterpene contents in this variety. The consistency of the TPS expression level and terpenoid content implies the critical involvement of TPS1-TPS6 and TPS12 in the synthesis of sesquiterpenes in pepper fruit, while the remaining seven TPSs genes (TPS7-TPS11 and TPS13) may be primarily responsible for the synthesis of monoterpenes.
Previous studies have shown that transcription factors play a primary role in regulating volatile plant secondary metabolites (Lu et al., 2020). In petunia, three MYB transcription factors (ODO1, EOBII, and PhMYB4) regulate the accumulation of isoeugenol and eugenol by controlling the flux into alcohol/phenol metabolism pathways (Verdonk et al., 2005; Spitzer-Rimon et al., 2010; Colquhoun et al., 2011). The bHLH transcription factor MYC2-1 in apple was identified to regulate the synthesis of aldehydes in the early stage of fruit development and the synthesis of esters in the late stage of fruit development (Liu et al., 2021). MYB4 was predicted to be involved in the synthesis of cinnamyl acetate, benzyl acetate and eugenol in Prunus mume (Wang et al., 2022a). In patchouli (Pogostemon cablin), the transcription factors PcWRKY44 and PatSWC4 (MYB transcription factor) interacted with the jasmonic acid responsive protein JAZ4 to activate the expression of the patchoulol synthase gene (PatPTS) or farnesyl pyrophosphate synthase (FPPS), thus promoting the synthesis of sesquiterpene patchoulol (Chen et al., 2020; Wang et al., 2022b). However, the bHLH transcription factor PatGT-1 negatively regulates the synthesis of patchoulol by inhibiting the expression of HMGR genes in patchouli (Li et al., 2021b). Furthermore, MYC2 (bHLH) in Arabidopsis thaliana (Hong et al., 2012), MYB14 in conifer trees (Bedon et al., 2010), PbbHLH4 and PbbZIP4 in Phalaenopsis bellina (Ramya et al., 2020), and CgWRKY1 and CgWRKY2 in Cymbidium goeringii (Rchb.f.) Rchb.F.(Caputi and Aprea, 2011). have also been reported to be involved in the synthesis of terpenes. In this study, 96 transcription factors (including 12 bZIP, 11 bHLH, 11 MYB, 6 C3H, and 5 WRKY transcription factors) were significantly correlated with volatiles. Among them, transcription factors such as bHLH62, bHLH143, bHLH147, MYB306, WRKY23, and bZIP9 were significantly correlated with multiple volatiles and were highly expressed in the QH or WH varieties. This indicates that these transcription factors may be involved in the synthesis of aroma components by regulating the expression of key genes in the volatile synthesis pathway of pepper fruit.
Previous research has demonstrated the involvement of plant hormones in regulating the synthesis of plant volatiles (Wu et al., 2018). Exogenous auxin and abscisic acid can change the volatile profile of tomato fruit by affecting the expression of volatile metabolic pathway genes (Wu et al., 2018; Tobaruela et al., 2021). In patchouli (Pogostemon cablin), the ethylene response factor PatDREB interacted with the MYB transcription factor PatSWC4 to activate the expression of the patchoulol synthase gene PatPTS, thus promoting the synthesis of sesquiterpene patchoulol (Chen et al., 2022). The ethylene response factor CitERF71 regulates the synthesis of E-geraniol (E) by activating the expression of the CitTPS16 gene in citrus fruit (Li et al., 2017). The jasmonic acid response factor ORCA3 has been linked to the production of terpenoid indole alkaloids in Catharanthus roseus (van der Fits and Memelink, 2000). The auxin-responsive protein HcIAA4 activates the expression of HcTPS5 by interacting with HcMYB1, thereby regulating the synthesis of linalool and methyl benzoate in Hedychium coronarium (Ke et al., 2021). In this study, 37 hormone response factors or hormone synthetic structure genes were identified to be significantly correlated with volatiles, including 21 auxin response factors. Among them, auxin response factors such as IAA27, IAA27-like, ARF8, ARF8-like, ARF12, and ARF3 were closely linked to more than 10 volatiles, respectively, and were located at key nodes in the interaction network of differentially expressed genes and metabolites. This suggests that these auxin response factors play a crucial role in regulating the synthesis of aroma substances. At present, the mechanism of auxin regulating volatile synthesis remains unclear. However, in this study, a large number of auxin response factors were enriched in the interaction network, providing new tools to analyze the mechanism underlying the auxin regulation of volatile synthesis. The specific functions of these auxin factors in volatile synthesis will be the focus of future studies.
Esters, aldehydes, alcohols and terpenes were the dominant volatile components in pepper fruits, and their contents decreased with the ripening of pepper fruits. The higher accumulation of esters and terpenes may be responsible for the release of fruit aroma by QH fruit. The biosynthesis of volatile compounds was closely related to fatty acid and terpenoid metabolic pathways, and the high expression of genes in fatty acid and terpenoid metabolic pathways was key in determining the accumulation of volatiles in pepper fruit. Furthermore, we constructed a regulatory network of volatile biosynthesis, identified potential candidate genes that may be involved in the biosynthesis of volatile compounds, and found that auxin may regulate the accumulation of volatiles in pepper fruits by inducing the high expression of auxin-responsive proteins or factors. These results have established a practical basis for further exploration of the regulatory mechanisms underlying the formation of aroma compounds in pepper.
The original contributions presented in the study are publicly available. This data can be found here: https://www.ncbi.nlm.nih.gov/search/all/?term=PRJNA1014701.
YuL: Formal Analysis, Funding acquisition, Methodology, Writing–original draft. JZ: Formal Analysis, Writing–original draft. CY: Methodology, Writing–original draft. FC: Investigation, Writing–original draft. YaL: Investigation, Writing–original draft. YiL: Investigation, Writing–original draft. ZZ: Project administration, Resources, Writing–review & editing. WL: Formal Analysis, Methodology, Writing–review and editing. JL: Writing–review and editing, Funding acquisition, Project administration.
The authors declare financial support was received for the research, authorship, and/or publication of this article. This research was partially supported by the National Natural Science Foundation of China (Grant No. 32302528), Hunan Provincial Natural Science Foundation of China (Grant No. 2022JJ40011), The Special Project of Biological Seed Industry and Fine and Deep Processing of Agricultural Products (Grant No. 202202AE090031), Scientific Research Fund of Hunan Provincial Education Department (Grant No. 21C0545), The Foundation of Hunan Key Laboratory for Conservation and Utilization of Biological Resources in the Nanyue Mountainous Region (Grant No. 2022HSKFJJ017).
The authors declare that the research was conducted in the absence of any commercial or financial relationships that could be construed as a potential conflict of interest.
All claims expressed in this article are solely those of the authors and do not necessarily represent those of their affiliated organizations, or those of the publisher, the editors and the reviewers. Any product that may be evaluated in this article, or claim that may be made by its manufacturer, is not guaranteed or endorsed by the publisher.
The Supplementary Material for this article can be found online at: https://www.frontiersin.org/articles/10.3389/fgene.2023.1290492/full#supplementary-material
Bedon, F., Bomal, C., Caron, S., Levasseur, C., Boyle, B., Mansfield, S. D., et al. (2010). Subgroup 4 R2R3-MYBs in conifer trees: gene family expansion and contribution to the isoprenoid-and flavonoid-oriented responses. J. Exp. Bot. 61 (14), 3847–3864. doi:10.1093/jxb/erq196
Beekwilder, J., Alvarez-Huerta, M., Neef, E., Verstappen, F. W., Bouwmeester, H. J., and Aharoni, A. (2004). Functional characterization of enzymes forming volatile esters from strawberry and banana. Plant Physiol. 135 (4), 1865–1878. doi:10.1104/pp.104.042580
Caputi, L., and Aprea, E. (2011). Use of terpenoids as natural flavouring compounds in food industry. Recent Pat. Food Nutr. Agric. 3 (1), 9–16. doi:10.2174/2212798411103010009
Cerezo, S., Hernández, M. L., Palomo-Ríos, E., Gouffi, N., García-Vico, L., Sicardo, M. D., et al. (2021). Modification of 13-hydroperoxide lyase expression in olive affects plant growth and results in altered volatile profile. Plant Sci. 313, 111083. doi:10.1016/j.plantsci.2021.111083
Chen, S., Zhou, Y., Chen, Y., and Gu, J. (2018). fastp: an ultra-fast all-in-one FASTQ preprocessor. Bioinformatics 34 (17), i884–i890. doi:10.1093/bioinformatics/bty560
Chen, X., Li, J., Liu, Y., Wu, D., Huang, H., Zhan, R., et al. (2020). PatSWC4, a methyl jasmonate-responsive MYB (v-myb avian myeloblastosis viral oncogene homolog)-related transcription factor, positively regulates patchoulol biosynthesis in Pogostemon cablin. Ind. Crops Prod. 154, 112672. doi:10.1016/j.indcrop.2020.112672
Chen, X., Wang, X., Wu, D., Li, J., Huang, H., Wang, X., et al. (2022). PatDREB transcription factor activates patchoulol synthase gene promoter and positively regulates jasmonate-induced patchoulol biosynthesis. J. Agric. Food Chem. 70, 7188–7201. doi:10.1021/acs.jafc.2c01660
Colquhoun, T. A., Kim, J. Y., Wedde, A. E., Levin, L. A., Schmitt, K. C., Schuurink, R. C., et al. (2011). PhMYB4 fine-tunes the floral volatile signature of Petunia x hybrida through PhC4H. J. Exp. Bot. 62 (3), 1133–1143. doi:10.1093/jxb/erq342
Dudareva, N., Negre, F., Nagegowda, D. A., and Orlova, I. (2006). Plant volatiles: recent advances and future perspectives. Crit. Rev. Plant Sci. 25 (5), 417–440. doi:10.1080/07352680600899973
Eggink, P. M., Tikunov, Y., Maliepaard, C., Haanstra, J., Visser, R., Vogelaar, A., et al. (2013). Capturing flavors from Capsicum baccatum by introgression in sweet pepper. Theor. Appl. Genet. 127 (2), 373–390. doi:10.1007/s00122-013-2225-3
Forero, M. D., Quijano, C. E., and Pino, J. A. (2009). Volatile compounds of Chile pepper (Capsicum annuum L. var. glabriusculum) at two ripening stages. Flavour Frag. J. 24 (1), 25–30. doi:10.1002/ffj.1913
Garruti, D. d.S., Pinto, N. d.O. F., Alves, V. C. C., Penha, M. F. A. d., Tobaruela, E. d.C., and Araújo, Í. M. d.S. (2013). Volatile profile and sensory quality of new varieties of Capsicum chinense pepper. Food Sci. Tech. 33, 102–108. doi:10.1590/s0101-20612013000500016
Ge, S., Chen, Y., Ding, S., Zhou, H., Jiang, L., Yi, Y., et al. (2020). Changes in volatile flavor compounds of peppers during hot air drying process based on headspace-gas chromatography-ion mobility spectrometry (HS-GC-IMS). J. Ssi Food Agr. 100 (7), 3087–3098. doi:10.1002/jsfa.10341
Halitschke, R., Ziegler, J., Keinänen, M., and Baldwin, I. T. (2004). Silencing of hydroperoxide lyase and allene oxide synthase reveals substrate and defense signaling crosstalk in Nicotiana attenuata. Plant J. 40 (1), 35–46. doi:10.1111/j.1365-313X.2004.02185.x
Heng, Z., Xu, X., Xu, X., Wang, H., Liu, L., Li, Z., et al. (2023). Characterization of odor-contributing volatile in Capsicum chinense ‘JT-1’fruits during development and transcriptome analysis of key fruit-aroma formation periods. Sci. Hortic. 309, 111691. doi:10.1016/j.scienta.2022.111691
Hong, G. J., Xue, X. Y., Mao, Y. B., Wang, L. J., and Chen, X. Y. (2012). Arabidopsis MYC2 interacts with DELLA proteins in regulating sesquiterpene synthase gene expression. Plant Cell 24 (6), 2635–2648. doi:10.1105/tpc.112.098749
Huang, C., Sun, P., Yu, S., Fu, G., Deng, Q., Wang, Z., et al. (2023). Analysis of volatile aroma components and regulatory genes in different kinds and development stages of pepper fruits based on non-targeted metabolome combined with transcriptome. Int. J. Mol. Sci. 24 (9), 7901. doi:10.3390/ijms24097901
Ke, Y., Abbas, F., Zhou, Y., Yu, R., and Fan, Y. (2021). Auxin-responsive R2R3-MYB transcription factors HcMYB1 and HcMYB2 activate volatile biosynthesis in Hedychium coronarium Flowers. Front. Plant Sci. 12, 710826. doi:10.3389/fpls.2021.710826
Kollmannsberger, H., Rodríguez-Burruezo, A., Nitz, S., and Nuez, F. (2011). Volatile and capsaicinoid composition of ají (Capsicum baccatum) and rocoto (Capsicum pubescens), two Andean species of Chile peppers. J. Sci. Food Agr. 91 (9), 1598–1611. doi:10.1002/jsfa.4354
Li, C., Xin, M., Li, L., He, X., Yi, P., Tang, Y., et al. (2021a). Characterization of the aromatic profile of purple passion fruit (Passiflora edulis Sims) during ripening by HS-SPME-GC/MS and RNA sequencing. Food Chem. 355, 129685. doi:10.1016/j.foodchem.2021.129685
Li, J., Chen, X., Zhou, X., Huang, H., Wu, D., Shao, J., et al. (2021b). Identification of trihelix transcription factors in Pogostemon cablin reveals PatGT-1 negatively regulates patchoulol biosynthesis. Ind. Crops Prod. 161, 113182. doi:10.1016/j.indcrop.2020.113182
Li, X., Xu, Y., Shen, S., Yin, X., Klee, H., Zhang, B., et al. (2017). Transcription factor CitERF71 activates the terpene synthase gene CitTPS16 involved in the synthesis of E-geraniol in sweet orange fruit. J. Exp. Bot. 68, 4929–4938. doi:10.1093/jxb/erx316
Lim, C. W., Han, S. W., Hwang, I. S., Kim, D. S., Hwang, B. K., and Lee, S. C. (2015). The Pepper lipoxygenase CaLOX1 plays a role in osmotic, drought and high salinity stress response. Plant Cell Physiol. 56 (5), 930–942. doi:10.1093/pcp/pcv020
Liu, X., Hao, N., Feng, R., Meng, Z., Li, Y., and Zhao, Z. (2021). Transcriptome and metabolite profiling analyses provide insight into volatile compounds of the apple cultivar ‘Ruixue’and its parents during fruit development. BMC Plant Biol. 21 (1), 231–316. doi:10.1186/s12870-021-03032-3
Liu, Y., Lv, J., Liu, Z., Wang, J., Yang, B., Chen, W., et al. (2020). Integrative analysis of metabolome and transcriptome reveals the mechanism of color formation in pepper fruit (Capsicum annuum L.). Food Chem. 306, 125629. doi:10.1016/j.foodchem.2019.125629
Love, M. I., Huber, W., and Anders, S. (2014). Moderated estimation of fold change and dispersion for RNA-seq data with DESeq2. Genome Biol. 15 (12), 550–621. doi:10.1186/s13059-014-0550-8
Lu, H., Luo, Z., Wang, L., Liu, W., Li, D., Belwal, T., et al. (2020). FaMYB9 is involved in the regulation of C6 volatile biosynthesis in strawberry. Plant Sci. 293, 110422. doi:10.1016/j.plantsci.2020.110422
Magalhães, H. C. R., Alves Filho, E. G., dos Santos Garruti, D., Massaretto, I. L., and Purgatto, E. (2021). Effect of postharvest methyl jasmonate and ethylene treatments on the biosynthesis of volatile compounds of hot pepper fruits. Sci. Hortic. 289 (13), 110477. doi:10.1016/j.scienta.2021.110477
Niu, Y., Deng, J., Xiao, Z., and Zhu, J. (2021). Characterization of the major aroma-active compounds in peach (Prunus persica L. Batsch) by gas chromatography-olfactometry, flame photometric detection and molecular sensory science approaches. Food Res. Int. 147, 110457. doi:10.1016/j.foodres.2021.110457
Padmanabhan, P., Cheema, A. S., Todd, J. F., Lim, L. T., and Paliyath, G. (2020). Ripening responses, fruit quality and phospholipase D gene expression in bell peppers exposed to hexanal vapor. Postharvest Biol. Tec. 170 (1), 111317. doi:10.1016/j.postharvbio.2020.111317
Pino, J., Gonzalez, M., Ceballos, L., Centurion-Yah, A. R., Trujillo-Aguirre, J., Latournerie-Moreno, L., et al. (2007). Characterization of total capsaicinoids, colour and volatile compounds of Habanero chilli pepper (Capsicum chinense Jack.) cultivars grown in Yucatan. Food Chem. 104 (4), 1682–1686. doi:10.1016/j.foodchem.2006.12.067
Ramya, M., Jang, S., An, H. R., Lee, S. Y., Park, P. M., and Park, P. H. (2020). Volatile organic compounds from orchids: From synthesis and function to gene regulation. Int. J. Mol. Sci. 21 (3), 1160. doi:10.3390/ijms21031160
Sánchez, G., Venegas-Calerón, M., Salas, J. J., Monforte, A., Badenes, M. L., and Granell, A. (2013). An integrative “omics” approach identifies new candidate genes to impact aroma volatiles in peach fruit. BMC Genomics 14 (1), 343–423. doi:10.1186/1471-2164-14-343
Sarde, S. J., Kumar, A., Remme, R. N., and Dicke, M. (2018). Genome-wide identification, classification and expression of lipoxygenase gene family in pepper. Plant Mol. Biol. 98 (4), 375–387. doi:10.1007/s11103-018-0785-y
Selli, S., Kelebek, H., Ayseli, M. T., and Tokbas, H. (2014). Characterization of the most aroma-active compounds in cherry tomato by application of the aroma extract dilution analysis. Food Chem. 165, 540–546. doi:10.1016/j.foodchem.2014.05.147
Shi, J., Wang, J., Lv, H., Peng, Q., Schreiner, M., Baldermann, S., et al. (2021). Integrated proteomic and metabolomic analyses reveal the importance of aroma precursor accumulation and storage in methyl jasmonate-primed tea leaves. Hortic. Res. 8 (1), 95. doi:10.1038/s41438-021-00528-9
Spitzer-Rimon, B., Marhevka, E., Barkai, O., Marton, I., Edelbaum, O., Masci, T., et al. (2010). EOBII, a gene encoding a flower-specific regulator of phenylpropanoid volatiles' biosynthesis in petunia. Plant Cell 22 (6), 1961–1976. doi:10.1105/tpc.109.067280
Taiti, C., Costa, C., Migliori, C. A., Comparini, D., Figorilli, S., and Mancuso, S. (2019). Correlation between volatile compounds and spiciness in domesticated and wild fresh chili peppers. Food Bioprocess Tech. 12 (5), 1366–1380. doi:10.1007/s11947-019-02297-9
Tobaruela, E. C., Gomes, B. L., Bonato, V. C. B., de Lima, E. S., Freschi, L., and Purgatto, E. (2021). Ethylene and auxin: hormonal regulation of volatile compound production during tomato (Solanum lycopersicum L.) fruit ripening. Front. Plant Sci. 12, 765897. doi:10.3389/fpls.2021.765897
van der Fits, L., and Memelink, J. (2000). ORCA3, a jasmonate-responsive transcriptional regulator of plant primary and secondary metabolism. Science 289 (5477), 295–297. doi:10.1126/science.289.5477.295
Verdonk, J. C., Haring, M. A., Van Tunen, A. J., and Schuurink, R. C. (2005). ODORANT1 regulates fragrance biosynthesis in petunia flowers. Plant Cell 17 (5), 1612–1624. doi:10.1105/tpc.104.028837
Wang, X., Tang, Y., Huang, H., Wu, D., Chen, X., Li, J., et al. (2022a). Functional analysis of Pogostemon cablin farnesyl pyrophosphate synthase gene and its binding transcription factor PcWRKY44 in regulating biosynthesis of patchouli alcohol. Front. Plant Sci. 13, 946629. doi:10.3389/fpls.2022.946629
Wang, X. J., Song, Z. Q., Ti, Y. J., Ma, K. F., and Li, Q. W. (2022b). Comparative transcriptome analysis linked to key volatiles reveals molecular mechanisms of aroma compound biosynthesis in Prunus mume. BMC plant Biol. 22 (1), 1–19. doi:10.1186/s12870-022-03779-3
Wu, Q., Tao, X., Ai, X., Luo, Z., Mao, L., Ying, T., et al. (2018). Contribution of abscisic acid to aromatic volatiles in cherry tomato (Solanum lycopersicum L.) fruit during postharvest ripening. Plant Physiol. Biochem. 130, 205–214. doi:10.1016/j.plaphy.2018.06.039
Yang, Y. N., Zheng, F. P., Yu, A. N., and Sun, B. G. (2019). Changes of the free and bound volatile compounds in Rubus corchorifolius L. f. fruit during ripening. Food Chem. 287, 232–240. doi:10.1016/j.foodchem.2019.02.080
Zhang, B., Shen, J. Y., Wei, W. W., Xi, W. P., Xu, C. J., Ferguson, I., et al. (2010). Expression of genes associated with aroma formation derived from the fatty acid pathway during peach fruit ripening. J. Agric. Food Chem. 58 (10), 6157–6165. doi:10.1021/jf100172e
Zhang, H., Chen, M., Wen, H., Wang, Z., Chen, J., Fang, L., et al. (2020). Transcriptomic and metabolomic analyses provide insight into the volatile compounds of citrus leaves and flowers. BMC Plant Biol. 20 (1), 7–14. doi:10.1186/s12870-019-2222-z
Keywords: pepper, volatile organic compounds, metabolome, transcriptome, transcription factors
Citation: Liu Y, Zhou J, Yi C, Chen F, Liu Y, Liao Y, Zhang Z, Liu W and Lv J (2023) Integrative analysis of non-targeted metabolome and transcriptome reveals the mechanism of volatile formation in pepper fruit. Front. Genet. 14:1290492. doi: 10.3389/fgene.2023.1290492
Received: 07 September 2023; Accepted: 27 October 2023;
Published: 10 November 2023.
Edited by:
Sunil Kumar Sahu, Beijing Genomics Institute (BGI), ChinaReviewed by:
Rajesh Kumar Singh, Institute of Himalayan Bioresource Technology (CSIR), IndiaCopyright © 2023 Liu, Zhou, Yi, Chen, Liu, Liao, Zhang, Liu and Lv. This is an open-access article distributed under the terms of the Creative Commons Attribution License (CC BY). The use, distribution or reproduction in other forums is permitted, provided the original author(s) and the copyright owner(s) are credited and that the original publication in this journal is cited, in accordance with accepted academic practice. No use, distribution or reproduction is permitted which does not comply with these terms.
*Correspondence: Zhuqing Zhang, Y3N6enFAMTI2LmNvbQ==; Wei Liu, MTc3MTE2NDIxNDlAMTYzLmNvbQ==; Junheng Lv, anVuaGVuZ2x2QGhudS5lZHUuY24=
Disclaimer: All claims expressed in this article are solely those of the authors and do not necessarily represent those of their affiliated organizations, or those of the publisher, the editors and the reviewers. Any product that may be evaluated in this article or claim that may be made by its manufacturer is not guaranteed or endorsed by the publisher.
Research integrity at Frontiers
Learn more about the work of our research integrity team to safeguard the quality of each article we publish.