- Department of Biology, Queens College, and PhD Program in Biology, The Graduate Center, City University of New York, New York City, NY, United States
The Transforming Growth Factor-β (TGF-β) superfamily of signaling molecules plays critical roles in development, differentiation, homeostasis, and disease. Due to the conservation of these ligands and their signaling pathways, genetic studies in invertebrate systems including the nematode Caenorhabditis elegans have been instrumental in identifying signaling mechanisms. C. elegans is also a premier organism for research in longevity and healthy aging. Here we summarize current knowledge on the roles of TGF-β signaling in aging and immunity.
Introduction
As organisms age, they undergo gradual cellular and molecular changes, accompanied by a decline in many physiological functions. Consequently, their susceptibility to age-related diseases and conditions increases (López-Otín et al., 2013; Son et al., 2019; Melzer et al., 2020). Many fundamental findings in the field of aging have come from studies in the small free-living nematode C. elegans (Murphy and Hu, 2013). C. elegans has been used as a model organism for decades due to its short lifespan of approximately 3 weeks, small size, transparent body, easy laboratory maintenance, genetic tractability, and conserved biological pathways (Brenner, 1974; C. elegans Sequencing Consortium, 1998). Approximately 83% of the C. elegans proteome has human homologs (Lai et al., 2000), and over 50% of human protein-coding genes have homologs in C. elegans (Sonnhammer and Durbin, 1997; Kuwabara and O’Neil, 2001; Harris et al., 2004). The insulin/IGF-1-like signaling pathway (IIS) was the first pathway identified to regulate lifespan in C. elegans. Mutations in daf-2, subsequently found to encode the sole Insulin/IGF-1-like receptor (Kimura et al., 1997), result in a doubled lifespan compared to wildtype (WT) (Kenyon et al., 1993). Further studies in C. elegans revealed the roles of other pathways that regulate aging including AMP-activated protein kinase (AMPK), and mechanistic target of rapamycin (mTOR) (Zhang et al., 2020). In addition, transforming growth factor beta (TGF-β) pathways are emerging as regulators of longevity and healthy aging that warrant further study.
One of the systems that faces the most consequential impacts of aging is the immune system, where age-associated decline is called immunosenescence. This decline manifests in increased infection susceptibility, decreased vaccination response, and increased risk for cancer and autoimmune diseases. The underlying changes that result in these physiologies in mammals are: a decrease in immune cell repertoire, cell intrinsic defects to lymphocytes, and increased inflammation (Akha, 2018). Aging and immunity can be regulated by shared molecular mechanisms, such as IIS, TGF-β, mTOR and nuclear factor kappa B (NF-κB) pathways (Powell et al., 2012; Papadopoli et al., 2019; Songkiatisak et al., 2022). Promoting healthy aging will need to address immunosenescence for an improved quality of life.
In addition to its pioneering contributions to the study of aging, C. elegans is also a valuable model system to study innate immunity (Nicholas and Hodgkin, 2002; Millet and Ewbank, 2004; Schulenburg et al., 2004). The animals’ diet consists of available bacteria in their environment, which in the laboratory is a non-pathogenic strain of Escherichia coli, OP50. This system is easily modified to study immunity, since the food source can be replaced with pathogenic bacteria, which allows bacterial pathogens to be easily introduced to the species. Additionally, C. elegans do not have antibody-based acquired immunity that could confound studies on innate immunity. As such, studies with C. elegans have identified conserved signaling pathways that regulate innate immunity, including IIS, p38 mitogen-activated protein kinase (MAPK) and TGF-β signaling (Ewbank, 2006; Kim and Ewbank, 2018). Immunity pathways overlap significantly with those that regulate aging (Kurz and Tan, 2004; Fabian et al., 2021). For example, mutations in DAF-2 and other IIS components not only extend lifespan, but also have improved resistance against bacterial pathogens than WT animals, although these effects can be uncoupled (Garsin et al., 2003; Kerry et al., 2006; Evans et al., 2008; Lee et al., 2021). Future studies are needed to expound these inter-connected physiologies, where improved understanding will positively contribute to healthy aging.
TGF-β signaling
The TGF-β superfamily of extracellular signaling molecules is an ancient and conserved mechanism of cell-cell communication in animals (Robertis, 2008). Disruptions in TGF-β signaling result in birth defects, as well as autoimmune disorders, cancer, and other diseases (Padua and Massagué, 2009; Wu and Hill, 2009). There are two major groups within the TGF-β superfamily, TGF-β/Activin and bone morphogenetic proteins (BMPs) (Herpin et al., 2004). TGF-β has known roles in cell proliferation, differentiation, apoptosis and reproductive function. For example, anti-müllerian hormone is required for follicular development in females, and when dysregulated, can cause polycystic ovarian syndrome, development of female reproductive structures in males, among others (Wu and Hill, 2009). Activin also plays a role in cell proliferation, differentiation, apoptosis, as well as reproductive function, commonly known for regulating many parts of the menstrual ovulatory cycle in humans (Adu-Gyamfi et al., 2020). Nodal is another member of the TGF-β/Activin group that is involved in embryonic development, such as axis formation and patterning (Wu and Hill, 2009). BMPs were first identified for their role in regulating bone and cartilage development (Urist and Strates, 1971; Wang et al., 1988; Wozney et al., 1988; Herpin et al., 2004). They are best known for roles in development and differentiation, such as embryonic body plan patterning and cell identity specification, but are emerging as modulators of homeostasis.
There is strong evidence that TGF-β signaling regulates aging, particularly in several age-associated diseases. TGF-β2 and the TGF-β receptors (I and II) have decreased expression in the cartilage of knee joints of old mice, compared to young mice. Blocking TGF-β activity demonstrated that TGF-β is necessary for a normal repair response, thus causing cartilage tissue damage (Davidson et al., 2005). This role has substantial implications in osteoarthritis, a prevalent age-associated disease. Another instance of age-related expression changes is BMP4, which was shown to increase with age in the dentate gyrus of both mice and humans (Meyers et al., 2016). The dentate gyrus is a part of the hippocampus that functions in learning and memory, and typically declines during aging. To establish a better relationship between cognitive function and BMP4 levels, a BMP4-expressing lentivirus was injected, which resulted in decreased cognition, compared to mice who had a control virus injected (Meyers et al., 2016). This function likely has implications in age-associated diseases that impair cognition, such as Alzheimer’s disease, and warrants continued study.
An enticing 2013 study found that TGF-β family member GDF11 could act as a circulating factor that reverses age-related decline (Loffredo et al., 2013). A conflicting report found that GDF11 levels increase rather than decrease during aging, and that the previous conclusions could have been confounded by reagents that cross react between GDF11 and GDF8 (myostatin) (Ahlberg et al., 2015). Controversy continues to the present day regarding the action of GDF11 in aging, a testament to the complexity of TGF-β signaling in the mammalian context (Ma et al., 2021). A less controversial GDF ligand is GDF-15, which has established roles in both immunity and aging (Pence, 2022). GDF-15 is a stress-induced cytokine, which acts as an immunoregulatory protein, dampening inflammatory responses in many immune cell types under pro-inflammatory conditions. Interestingly, GDF-15 has been shown to increase with age. One study examined the plasma proteome over lifespan and found that GDF-15 was the single protein most associated with age, increasing linearly.
TGF-β members also regulate immunity. Of the three TGF-β members in humans, TGF-β1 is most involved in immunity. TGF-β1 is a major regulator of T-cell development, homeostasis, and survival (Li and Flavell, 2008). In fact, mice lacking functional TGF-β1 are severely immunodeficient (Gough et al., 2021), and eventually develop fatal inflammatory diseases, even under germ-free conditions (Li and Flavell, 2008). Another TGF-β family ligand is Activin A, from the Activin subgroup, which plays a role in both innate and adaptive immunity. Based on cell type and context, Activin A can function as anti-inflammatory or pro-inflammatory, and is very widespread in its immune regulation (Chen and Dijke, 2016). The aging and immunity functions of TGF-β signaling intersect with TGF-β′s well-known role in cancer regulation. During homeostasis, TGF-β signaling maintains a healthy cellular environment. However, during tumor progression, this signaling can become disrupted, instead suppressing immune systems, promoting cancer (Batlle and Massagué, 2019). This relationship between cancer and immunity is largely through adaptive immunity, which C. elegans does not have, however there are some aspects of innate immunity involved, such as the inhibition of natural killer cells, and regulating macrophages.
The canonical signal transduction pathway begins with a TGF-β ligand dimer binding to a heterotetrameric complex composed of type I receptor and type II receptors (Dijke and Hill, 2004). The type II receptors’ serine/threonine kinase domain phosphorylates the type I receptors’ glycine-serine (GS) domain, which activates the type I receptor serine/threonine kinase. This kinase activity then phosphorylates receptor regulated Smads (R-Smads) at the C-terminus, which form a heterotrimeric complex with common mediator Smads (Co-Smads). The Smad complex enters the nucleus to regulate gene transcription (Massagué et al., 2005). TGF-β/Activin and BMP ligands signal predominantly through different receptors and Smads: Smad2/Smad3 act with Smad4 for TGF-β/Activin and Smad1/Smad5/Smad8 act with Smad4 for BMPs (Dijke and Hill, 2004).
TGF-β signaling in Caenorhabditis elegans-Overview
In the C. elegans genome, there are five genes encoding TGF-β family ligands: DBL-1, DAF-7, TIG-2, TIG-3 and UNC-129 (Gumienny and Savage-Dunn, 2013). In comparison, humans have over 30 TGF-β family members. The reduced complexity of TGF-β signaling in C. elegans is an experimental advantage for elucidating the functions and signaling mechanisms of the pathways (Table 1). DBL-1 and TIG-2 are members of the BMP family; DAF-7 and TIG-3 are related to TGF-β/Activin; and UNC-129 is more divergent (Savage-Dunn and Padgett, 2017). Two of the ligands, DBL-1 and DAF-7, have well-defined signaling pathways as described further below (Figure 1). While DAF-7 signaling has been associated with longevity since 2007 (Shaw et al., 2007), recent advances have demonstrated roles for all of these ligands in immunity (Ciccarelli et al., 2023b).
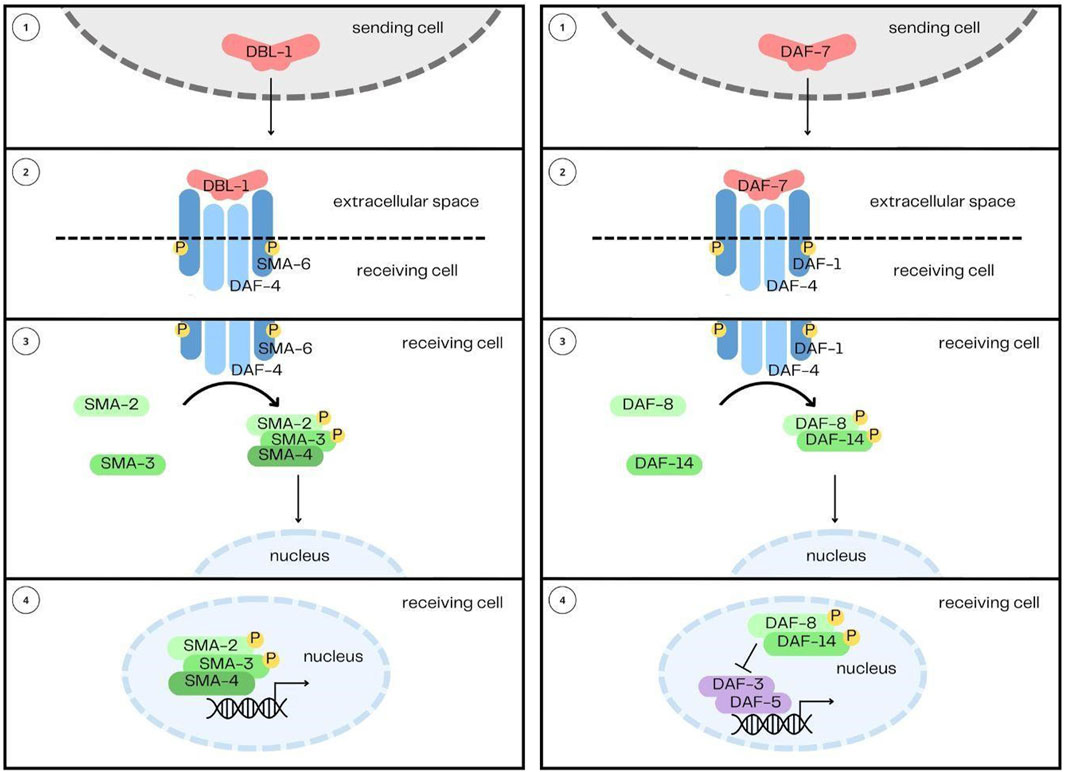
FIGURE 1. The DBL-1/BMP and DAF-7/TGF-β Signaling Pathways. The two pathways share their overall structure in common: 1) the ligands, DBL-1 and DAF-7, are secreted from their sending cells, 2) and are received by heterotetrameric complexes comprised of two type I receptors and two type II receptors. 3) Signal is then transduced by Smads, 4) which enter the nucleus and interact with transcription factors to regulate gene expression.
The DBL-1/BMP pathway
The DBL-1 pathway was first identified from two mutant phenotypes: small body size (Sma) and male abnormal tail morphology (Mab) (Baird and Ellazar, 1999; Morita et al., 1999; Suzuki et al., 1999; Savage-Dunn et al., 2003), hence also giving the alternative pathway name Sma/Mab (Savage et al., 1996; Padgett et al., 1998). The DBL-1 pathway is not essential for organism viability, which has made many genetic manipulations and further studies possible. The DBL-1 signaling pathway, since its original identification, has been demonstrated to play a role in innate immunity, reproductive aging, mesodermal patterning, chemosensation, L1 development, and lipid metabolism (Mallo et al., 2002; Foehr et al., 2006; Vashlishan et al., 2008; Almedom et al., 2009; Luo et al., 2009; Kaplan et al., 2015; Yu et al., 2017; Clark et al., 2018). This pathway in C. elegans follows the canonical signal transduction pathway described earlier and begins with the ligand DBL-1, named for being Dpp and BMP-like (Morita et al., 1999; Suzuki et al., 1999). The signal from DBL-1 is received by a heterotetramer formed by the type I receptor SMA-6 (Krishna et al., 1999), and the type II receptor DAF-4 (Estevez et al., 1993). These two receptors have different recycling mechanisms (Gleason et al., 2014), regulated by tetraspanins (Liu Z. et al., 2020). Signal is then transduced by the receptor-regulated Smads, SMA-2, SMA-3, and the common mediator Smad, SMA-4 (Savage et al., 1996). Null mutants of sma-3 display phenotypes equally as severe as dbl-1 null alleles (Savage-Dunn et al., 2000), indicating a lack of redundancy between the Smads in the pathway. Downstream transcription factors include SMA-9, the homolog of Drosophila Schnurri (Liang et al., 2003), LIN-31/forkhead (Baird and Ellazar, 1999) and MAB-31 (Wong et al., 2010). DBL-1 is expressed and released by cholinergic neurons in the head and nerve cords (Morita et al., 1999; Suzuki et al., 1999; Duerr et al., 2008). This includes the AFD amphid neuron, ventral nerve cord, DA, DB, VA, and VB motorneurons, several neurons in the pharynx, and in the male tail, the spicule socket cells and the DVA neuron (Morita et al., 1999; Suzuki et al., 1999). An upstream transcription factor regulating dbl-1 expression is UNC-3 (Kratsios et al., 2012). DBL-1 receptors and Smads are expressed in the epidermis (hypodermis), pharynx and intestine (Patterson et al., 1997; Krishna et al., 1999; Yoshida et al., 2001; Mallo et al., 2002; Wang et al., 2002; Reece-Hoyes et al., 2007).
DBL-1 and aging
The DBL-1 pathway plays a major role in reproductive aging (Luo et al., 2009; Luo et al., 2010). In WT hermaphrodites, the mean reproductive span is 3.5 days, while in dbl-1 mutants, it is greater than 7 days (Luo et al., 2009). Other BMP pathway mutants, such as daf-4, sma-2, sma-3 and sma-9, also have increased reproductive spans (Luo et al., 2009), suggesting that the DBL-1 pathway is responsible for correctly timing cessation of reproduction. The regulation of reproductive span is uncoupled from longevity, and independent of DAF-2/Insulin signaling and dietary restriction because the phenotypes in DBL-1 pathway mutants do not require DAF-16 or PHA-4 (Luo et al., 2009). Inactivation of DBL-1 signaling delays cessation of reproduction by maintaining oocyte and germline quality non-cell autonomously. In sma-2 mutants, proliferating germ cells are maintained for longer. In aged adults, these mutants have improved oocyte morphology compared to WT, which results in improved embryo quality, and less embryonic lethality than WT. sma-2 mutant adults also have improved distal germline integrity, with fewer indicators of decline, such as cavities, graininess and cellularization (Luo et al., 2010). A genome-wide RNAi screen identified 32 genes that, when inactivated, extend reproductive span (Wang et al., 2014). 25 of these 32 genes were shown to interact with SMA-2 to enact this phenotype (Wang et al., 2014). Later work elucidated that inactivation of DBL-1 signaling reduces cAMP response element-binding protein (CREB) activity downstream. This reduction elevates the Hedgehog-related WRT-10, which interacts with Patched-related receptors PTC-1 and PTR-2 to promote oocyte quality maintenance during aging and prevent “normal” cessation of reproduction (Templeman et al., 2020). All core components of DBL-1/BMP signaling have been shown to regulate the vit-2 vitellogenin enhancer (Goszczynski et al., 2016). As a result, loss-of-function BMP mutations could have decreased vitellogenin transcription causing slower oocyte yolk accumulation and oocyte maturation time, however further experimental studies are needed. This could be a partial explanation for the extended reproductive span of these mutations.
While DBL-1 signaling plays a major role in reproductive aging, in somatic aging, the pathway plays a minor to moderate role, depending on genetic background. In different experiments, dbl-1 mutant animals have slightly reduced or slightly increased lifespan compared to WT (Mallo et al., 2002; Luo et al., 2009; So et al., 2011). Reduced lifespan may be due to an immune defect, where dbl-1 mutants are more sensitive to the mild pathogenic effects of OP50 (McCulloch and Gems, 2003; Luo et al., 2009; Haque and Nazir, 2016). Consistent with that hypothesis, dbl-1 mutants have an increase in survival on heat-killed OP50 versus live OP50, however not enough to return lifespan to WT levels on heat-killed OP50 (Mallo et al., 2002). Furthermore, with the addition of Floxuridine (FUdR), a DNA synthesis inhibitor used to prevent progeny development, the dbl-1 mutant lifespan was similar to or greater than WT lifespan (Mallo et al., 2002; Luo et al., 2009). The FUdR not only prevents progeny development, but also prevents bacterial replication, likely decreasing the pathogenic effects of OP50. Furthermore, on HB101, a less-pathogenic bacterial strain of E. coli, dbl-1 mutant animals have a comparable lifespan to N2 (So et al., 2011).
In sensitized backgrounds, DBL-1/BMP signaling plays a moderate role in the regulation of longevity. DBL-1 and other BMP signaling components are required for other mutants’ lifespan phenotypes. For example, daf-2 sma-3 double mutants have a reduced median lifespan when compared to daf-2 single mutants, indicating that BMP signaling is required to execute this long-lived phenotype (Clark et al., 2021). Another instance of DBL-1 signaling regulating longevity in sensitized backgrounds is with neuronal overexpression of heat shock factor HSF-1, which increases lifespan and reduces BMP pathway activity (Chauve et al., 2021). The lifespan extension requires DBL-1 and SMA-6 because HSF-1 transcriptionally represses DBL-1 by directly binding to the DBL-1 promoter (Arneaud et al., 2022). A third example is that sma-2 or sma-3 mutations are required for the longevity of ifg-1 (initiation factor 4G) mutants (Chomyshen et al., 2022). ifg-1 mutants have inhibited stress-induced alternative splicing, and upregulated RNA splicing regulators, which extend lifespan. The upregulation of RNA splicing regulators is mediated by SMA-2 (Chomyshen et al., 2022). These three examples demonstrate that BMP signaling components are required to enact the lifespan phenotypes of other mutants by regulating aging genes. Interestingly, SMA-4 was shown to be differentially regulated during aging, which may be an explanation for this regulation (He et al., 2014).
DBL-1 and the microbiome
The microbiome of C. elegans is a topic that intersects aging and immunity, as some microbe species can be beneficial to the host, while other species are detrimental and pathogenic. The DBL-1/BMP pathway has been shown to regulate both beneficial and pathogenic interactions with the microbiome.
Several bacterial strains are known to extend lifespan of C. elegans. The human gut microbes Propionibacterium freudenreichii, Butyricicoccus pullicaecorum and Megasphaera elsdenii extend lifespan in WT C. elegans (Kwon et al., 2016; 2018). However, dbl-1 mutant animals had no change in lifespan, indicating that this extension is acting through the DBl-1/BMP pathway (Kwon et al., 2016; 2018). A diet consisting of the bacterial species Chryseobacterium sp. CHNTR56 MYb120, from the C. elegans native microbiome, also results in lifespan extension, and causes an upregulation in several key DBL-1 pathway components compared to OP50 (Haçariz et al., 2021). Another instance of microbial benefit is from Lactobacillus spp. Lb21, which not only extends lifespan when fed to C. elegans, but also results in increased resistance against bacterial pathogen methicillin-resistant Staphylococcus aureus (MRSA) (Mørch et al., 2021). This increased resistance was dependent on DBL-1 (Mørch et al., 2021). Furthermore, ingestion of certain Lactobacillus isolates upregulates the expression of DBL-1 and also p38 MAPK signaling, resulting in increased resistance to Salmonella typhimurium dT104 (Zhou et al., 2021).
DBL-1 signaling not only carries out the benefits of some bacterial species, but also influences microbiome selection. In an experiment, animals at the first larval stage were placed on plates with synthetic microbiota, comprising 30 previously isolated C. elegans gut commensals in equal parts. After 3 days, dbl-1 mutants had a threefold increase in gut bacterial load compared to WT, and had an increased abundance of Enterobacter species (Berg et al., 2019). When a second synthetic community was tested, dbl-1 mutants continued to show an expanded gut microbiome, and increased Enterobacteriaceae, suggesting these are general qualities of dbl-1 mutants. Enterobacter is typically a commensal bacterial species, however in the context of these compromised mutants, Enterobacter becomes pathogenic (Berg et al., 2019). Fascinatingly, WT worms exposed to synthetic microbiota that represent a wild environment experience a bloom in gut Enterobacteriaceae during aging (Choi et al., 2023). This bloom results in increased infection susceptibility to E. faecalis in the aging population. A causative factor for this Enterobacteriaceae bloom turned out to be an age-dependent decline in DBL-1/BMP signaling (Choi et al., 2023). This study highlights how DBL-1/BMP regulation of the microbiome has consequences in aging and immunity. These findings are also supported by mammalian studies. One found that the intestinal microbiomes of patients with inflammatory bowel disease had increased Enterobacteriaceae (Morgan et al., 2012). In mice, increased colonic Enterobacteriaceae was associated with a loss of TGF-β signaling, suggesting that this role may be conserved (Ihara et al., 2016). In addition to this work, DBL-1 was found to positively regulate the host N-glycosylation protein BCF-1, shaping microbiome selection. BCF-1 directly binds E. coli cells using its fimbrial protein, encouraging colonization of the gut by E. coli cells (He et al., 2023).
DBL-1 and immunity
The DBL-1/BMP signaling pathway in C. elegans is a major regulator of innate immunity. Early evidence of this role emerged from observations showing an increased susceptibility of dbl-1 mutants to infection by Serratia marcescens (Mallo et al., 2002). In addition, the dbl-1 pathway component SMA-3 was identified in a genetic screen as having an increased susceptibility to Pseudomonas aeruginosa strain PA14 (Tan, 2001). Loss of DBL-1 signaling has consequences in the immune response to a range of pathogens, including bacteria E. coli, Enterococcus faecalis, P. aeruginosa PA14, Salmonella enterica, S. typhimurium strain SL1344, S. marcescens, Photorhabdus luminescens, and the nematophagous fungus D. coniospora (Mallo et al., 2002; Tenor and Aballay, 2008; Zugasti and Ewbank, 2009; So et al., 2011; Portal-Celhay et al., 2012; Ciccarelli et al., 2023a). It was recently shown that expression of SMA-3 in the pharynx increased survival on pathogenic bacteria compared to sma-3 mutant animals (Ciccarelli et al., 2023a). In the response to pathogenic fungus Drechmeria coniospora, SMA-3 but not SMA-2 or SMA-4 is required, indicating the existence of non-canonical signaling of R-Smad without a Co-Smad (Zugasti and Ewbank, 2009). In trying to identify patterns in how DBL-1 signaling responds to Gram-negative versus Gram-positive bacteria, one study tested a panel of three Gram-negative bacteria, and three Gram-positive bacteria, and found trends in DBL-1 pathway activity (Madhu and Gumienny, 2021). Using an integrated fluorescent DBL-1 reporter, they found an induction of DBL-1 signaling on Gram-negative bacteria, while no fluorescence was seen on Gram-positive bacteria. They also identified patterns in avoidance response discussed below. While these trends are interesting, other studies have reported data that contradict this pattern, thus a larger panel of bacteria needs to be studied to understand what DBL-1 signaling patterns exist in response to Gram-negative bacteria or Gram-positive bacteria. SMA-10, an extracellular regulator of the DBL-1/BMP pathway has been shown to regulate immunity, and sma-10 mutants have increased susceptibility to PA14 infection. Interestingly, SMA-10 seems to be acting independently of DBL-1, instead acting through DAF-2, further demonstrating the extensive crosstalk that exists with IIS (Lucas et al., 2021). All these data suggest that BMP signaling is a broad regulator of immune response to a range of pathogens, however activates different downstream immune effectors in a pathogen-specific manner.
DBL-1/BMP signaling not only regulates immune response, but also is responsible for enacting some consequences of infection. DBL-1/BMP and IIS pathways are responsible for a hormetic effect after developmental exposure to a pathogenic E. coli strain (Leroy et al., 2012). Hormesis is a phenomenon where low dose exposure to a typically harmful agent has a beneficial effect on an organism. In a study assessing the impact of bacterial infection on male morphology and spermatogenesis, a 24-h infection with S. aureus or Vibrio alginolyticus caused abnormal tail morphology and decreased sperm activation (Sharika et al., 2018). The compound pentagalloyl glucose (PGG) is a polyphenol derived from plants. The addition of PGG to C. elegans resulted in increased expression of DBL-1 and DAF-4, as well as increased survival on two P. aeruginosa strains (Zhang X. et al., 2021). The survival effect was dependent on DAF-4 (Zhang X. et al., 2021).
We can define several mechanisms by which organisms can defend against pathogen exposure. They include: behavior, immune resistance (including innate immunity, which comprises transcriptional regulation of antimicrobial peptides (AMPs) and barrier functions), and tolerance. These mechanisms decrease infection susceptibility and increase survival chance, and operate together as part of a holistic immune response. All of these mechanisms are regulated by the DBL-1/BMP pathway.
The first immune strategy is behavior, which often begins before contact with potential pathogens is made. The primary behavior that supports immunity is avoidance, where a host organism identifies a risk of pathogen exposure, and modulates behavior to decrease this risk. This behavior is well characterized in C. elegans, where individuals detect a pathogen, and then distance themselves. A short exposure to certain pathogenic bacteria, such as PA14 and S. marcescens, will induce olfactory learning in C. elegans, where animals will avoid that bacteria in subsequent exposures (Zhang et al., 2005). This avoidance behavior was shown to require DBL-1 secreted from the AVA command interneurons, and SMA-6 in the epidermis (Zhang and Zhang, 2012). It was later found that production of DBL-1 in ASI and ASJ sensory neurons is prevented by AMPylase FIC-1 overexpression, consistent with their decreased pathogen avoidance (Hernandez-Lima et al., 2022). Loss of dbl-1 has also been seen to have an increased avoidance of E. coli, supporting how OP50 may have increased pathogenicity in these animals compared to WT (Madhu and Gumienny, 2021). In a panel of three Gram-positive bacteria and three Gram-negative bacteria, dbl-1 mutants displayed strong avoidance to all three Gram-negative bacteria, and a mild response to one of three Gram-positive bacteria. Unexpectedly, loss of SMA-4 had the opposite effect: a severe avoidance response to all three Gram-positive bacteria, correlated with increased SMA-4 expression. This led the authors to hypothesize that while the canonical pathway was involved in responding to all three Gram-negative bacteria, SMA-4 may act independently of DBL-1 in response to some Gram-positive bacteria, perhaps functioning with another pathway (Madhu and Gumienny, 2021). Further studies using a larger bacterial panel would be interesting, and instrumental to make stronger conclusions. C. elegans also detect pathogenic bacteria through bacterial secreted peptides, such as serrawettin W2 from S. marcescens (Pradel et al., 2007). Given the role of DBL-1 signaling in chemosensation, a fruitful area of future study may be identifying what pathogenic secreted peptides are detected through DBL-1 signaling.
The second immune strategy is the transcriptional regulation of genes involved in the immune response and the induction of AMPs. DBL-1/BMP signaling regulates gene expression of lectins, digestive enzymes like lysozymes and lipases, the PGP (P-glycoprotein) subclass of ATP-binding cassette (ABC) transporter family, caenacin (cnc) AMPs, saposin-like proteins or caenopore AMPs, and the glycocalyx (Mochii et al., 1999; Mallo et al., 2002; Alper et al., 2007; Liang et al., 2007; Zugasti and Ewbank, 2009; Roberts et al., 2010; Julien-Gau et al., 2014; Madhu et al., 2020). One group of AMPs, caenacins, are produced upon infection with a pathogen, and are critical to immune response. DBL-1 was shown to promote cnc-2 expression in the epidermis, in a dose-dependent manner (Zugasti and Ewbank, 2009). Recent work found that CNC-2, as well as another AMP, ABF-2, are regulated by SMA-3 activity in the pharynx (Ciccarelli et al., 2023a). Another AMP, the saposin-like protein SPP-9, was shown to be negatively regulated by DBL-1 signaling, and is used as a reporter for DBL-1 activity (Roberts et al., 2010; Madhu et al., 2020). The glycocalyx, a glycoprotein and glycolipid exterior layer of many cell membranes, is hypothesized to be involved in immune response. In C. elegans, BCF-1 is a believed component of the glycocalyx and is activated upon infection by P. aeruginosa and P. luminescens (Julien-Gau et al., 2014). BCF-1 requires DBL-1, SMA-6 and PMK-1, demonstrating its regulation by the DBL-1/BMP and MAPK pathways (Julien-Gau et al., 2014). AMPs are induced not only in response to infection, but also by wounding. Wounding was found to activate NAS-38, which enacted the AMP immune response in parallel through the DBL-1/BMP and p38 MAPK pathways (Sinner et al., 2021). Activation of these AMPs increases RIS neuron activity, which promotes sleep and ultimately contributes to survival after pathogen exposure (Sinner et al., 2021). Thus, DBL-1/BMP regulation of AMPs not only directly reduces pathogen load, but also promotes host well-being resulting in improved survival.
The third immune strategy is barrier functions that limit the amount of bacteria that can enter the organism. In some BMP mutants, particularly dbl-1, there is more live E. coli OP50 present in the gut (So et al., 2011). While this is partially due to the blunted induction of AMPs in dbl-1 mutants, which enables bacterial colonies to form in the intestine, it may also result from a pharyngeal defect, which allows more live bacteria to enter the intestine. While dbl-1 mutants appear to have normal pharyngeal pumping and peristalsis, they exhibit abnormal pharyngeal g1 gland cell morphology (Ramakrishnan et al., 2014). Gland cell abnormalities are likely a consequence of DBL-1’s activation of M4, a neuron in the pharynx responsible for initiating peristaltic contractions. The M4 neuron secretes DBL-1, regulated by the upstream homeodomain transcription factor CEH-28. Surprisingly, sma-2 and sma-3 mutants did not exhibit any gland cell abnormalities, suggesting that the regulation of gland cell morphology by DBL-1 may be independent of the R-Smads, perhaps instead functioning through a non-canonical pathway (Ramakrishnan et al., 2014). This study was conducted on OP50, so it is not known whether these effects change on more pathogenic bacteria. Later work demonstrated that loss of DBL-1 results in decreased pharyngeal pumping on select pathogens: Klebsiella oxytoca, S. marcescens, E. faecalis, or S. epidermidis (Madhu and Gumienny, 2021), suggesting that DBL-1 may be required for the pharynx to function correctly on some pathogens. sma-3 mutants have been shown to have decreased pharyngeal pumping on P. luminescens, which likely contributes to their increased infection susceptibility (Ciccarelli et al., 2023a). This decreased pumping was rescued when sma-3 was expressed in pharyngeal muscle, demonstrating another instance of DBL-1/BMP regulation of pharynx morphology.
Another physical barrier that is a part of innate immunity is the cuticle, a specialized extracellular matrix that comprises the outermost layer of the animal that separates it from its environment. Specific cuticle collagens that form furrows in the cuticle are responsible for its barrier function (Sandhu et al., 2021). The presence of these collagens enhances the longevity of daf-2/InsR mutants (Sandhu et al., 2021); however, their roles in immune barrier function have not yet been tested. DBL-1 is necessary to form the cuticle correctly, and alterations in DBL-1 signaling result in dose-dependent disruptions to cuticle organization and surface lipid content (Schultz et al., 2014). DBL-1 signaling regulates expression of cuticle collagen and extracellular matrix-associated genes (Liang et al., 2007; Luo et al., 2010; Roberts et al., 2010; Yin et al., 2015; Lakdawala et al., 2019), and cuticle collagens regulate DBL-1 signaling (Madaan et al., 2018; 2020), forming a feedback loop that requires further investigation (Goodman and Savage-Dunn, 2022). In dbl-1 mutants, the permeability of the cuticle is increased (Schultz et al., 2014), although what consequences this increased permeability has on immune response has yet to be assessed.
A fourth defense mechanism is immune tolerance, which aims to reduce the negative consequences that arise from infection. It mitigates detrimental effects caused either directly by the pathogen, or indirectly from host immunopathology resulting from the infection. This mechanism has been better studied in other organisms and needs to be addressed in C. elegans. The word “tolerance” itself was chosen for its definition of “the capacity to endure”, demonstrating how this understudied immune strategy may improve survival. This concept was first introduced in plant biology, where organisms from different wheat and oat species had varied tolerance to rust fungi (Caldwell et al., 1958; Schafer, 1971). This concept later was expanded to animal immunity (Ayres and Schneider, 2008; Read et al., 2008) after a study using mice and rodent malaria demonstrated that genetic variation exists for both disease resistance and disease tolerance between individuals, and are independent of one another (Raberg et al., 2009). More recent work from plants and Drosophila suggest that lipid metabolism and other homeostatic processes may affect immune tolerance (Lu et al., 2020; Laureano et al., 2021; Deshpande et al., 2022). In C. elegans, the fatty acid oleate is required for immune gene transcription, but is not sufficient for pathogen resistance on P. aeruginosa PA14, E. faecalis and S. marcescens (Anderson et al., 2019). The role of oleate, as well as other aspects of lipid metabolism, in immune tolerance is an interesting area that should be further explored. Together, the four mechanisms described have different aims and function at different times: behavior and barrier functions which aim to prevent pathogen load, the induction of AMPs aim to decrease pathogen load, and immune tolerance aims to decrease the burden that infection has on the host once established (Raberg et al., 2007; Medzhitov et al., 2012).
DBL-1 and response to xenobiotics
Studies on innate immunity have focused on the response to biological pathogens, such as bacteria and fungi. However, the response to non-biological toxins, xenobiotics, overlaps with the immune response. One such xenobiotic is nanoplastics, small pieces of plastic ranging from 1 to 100 nm, which are foreign and potentially harmful substances. It was found that DBL-1/BMP regulates response after exposure (Liu H. et al., 2020). Later work demonstrated that DBL-1 acts downstream of the glutamate receptor GLR-8 (Wang et al., 2021) and the Gα proteins GOA-1, GSA-1 and GPA-10 (Yang et al., 2021).
The DAF-7/dauer pathway
The DAF-7 pathway was first identified from its regulation of the dauer/continuous development switch, hence giving the alternative pathway name, the Dauer pathway (Riddle et al., 1981; Golden and Riddle, 1984; Thomas et al., 1993). Dauer is a developmental stage that occurs during adverse environmental conditions, such as high population density, or low food availability. Entering this stage results in morphological changes to the organism, including an elongated body, narrowed pharynx, as well as metabolic changes, which allow the animals to survive for several months without food and water. The duration they can survive in the dauer state far exceeds the typical lifespan of C. elegans, which is approximately 3 weeks. DAF-7 interacts with DAF-2/insulin signaling to regulate dauer entry and exit. In addition to its role in dauer formation, the DAF-7 pathway also functions in lipid metabolism, feeding behavior, aging, the germline proliferative zone, and autophagy (Fujiwara et al., 2002; Hirose et al., 2003; Greer et al., 2008; Park et al., 2010; Dalfó et al., 2012; Lee et al., 2017; Zhang et al., 2019). This pathway in C. elegans begins with the ligand DAF-7 (Ren et al., 1996). The ligand is received by a heterotetramer formed by the type I receptor DAF-1 (Georgi et al., 1990) and the type II receptor DAF-4 (Estevez et al., 1993). Signal is then transduced by the receptor-regulated Smads DAF-8 (Park et al., 2010) and DAF-14 (Inoue and Thomas, 2000), and common mediator Smad, DAF-3 (Patterson et al., 1997). DAF-5, the Sno/Ski homolog, acts downstream of the DAF-7 pathway and binds DAF-3 in a conserved manner (Graca et al., 2003; Tewari et al., 2004; Inoue and Imamura, 2008). Components of the DAF-7 pathway have diverged from TGF-β components in other organisms, but retain sequence features in common and may be more related to TGF-β/Activin components than to BMP components.
DAF-7 expression is detected primarily in ASI sensory neurons (Ren et al., 1996; Schackwitz et al., 1996), however other pathway components are more widely expressed. DAF-7 expression in ASI sensory neurons is repressed in unfavorable conditions, such as high population density and food scarcity. This repression contributes to the formation of dauer in these conditions (Ren et al., 1996). Consequently, null mutant alleles of DAF-7 display a dauer constitutive phenotype.
DAF-7 and aging
DAF-7 plays a significant role in somatic aging. Early reports concluded that DAF-7 and IIS pathways diverge in adulthood to regulate separate functions: DAF-2/InsR, but not DAF-7/TGF-β, was thought to regulate longevity (Kenyon et al., 1993; Larsen et al., 1995). However, later, it was shown that matricide of DAF-7 pathway mutants obscured their extended lifespan phenotype (Shaw et al., 2007). The matricide resulted from an egg-laying defect where embryos would hatch internally. When lifespans were conducted with the addition of FUdR, a significant increase in lifespan was seen for daf-7, daf-1, daf-4, daf-8 and daf-14 mutants, compared to WT (Shaw et al., 2007). The increase in lifespan requires DAF-16/FOXO, a downstream transcription factor of the DAF-2/insulin signaling pathway. This indicates that DAF-7/TGF-β regulates longevity through DAF-2/insulin signaling (Shaw et al., 2007). An additional instance of DAF-2/insulin and DAF-7/TGF-β signaling crosstalk regulating lifespan is through HSF-1. The lifespan extension of daf-7(e1372) mutant animals was strongly, but partially, suppressed by the hsf-1(sy441) mutation, indicating that HSF-1 is regulated by DAF-7 (Barna et al., 2012). It is already known that HSF-1 is required for lifespan extension in IIS mutants (Hsu et al., 2003), indicating that HSF-1 may be connecting IIS and TGF-β pathways with respect to aging. Another regulator of aging that is downstream of DAF-7 is DAF-9 (Gerisch et al., 2001), which decreases lifespan by inhibiting DAF-12, a nuclear hormone receptor (Jia et al., 2002).
With respect to reproductive aging, there is little evidence that DAF-7 plays a role. However, it has been shown that inactivation of DAF-3 extends reproductive span (Wang et al., 2014).
DAF-7 and the microbiome
DAF-7 signaling influences microbiome selection, though to a lesser degree than DBL-1. A large synthetic microbiome was created with 63 bacterial strains that reflect most of the core families found in wild C. elegans microbiomes. Over 38 wild C. elegans isolates were placed on plates with this synthetic microbiome at the first larval stage. After 120 h, the microbiome compositions could be quantified and categorized; three types emerged. A commensal member of the wild C. elegans microbiome is Ochrobactrum, which frequently became the dominant microbiome species in 28 of the 38 wild C. elegans strains assayed. Host insulin signaling is responsible for driving Ochrobactrum to establish microbiotic dominance (Zhang F. et al., 2021). The animals with an Ochrobactrum-dominant gut microbiome showed increased expression of DAF-8 and DAF-14 Smads that might be associated with upregulated DAF-7 signaling. Further study is needed to identify how DAF-7 contributes to this microbiome selection.
DAF-7 and immunity
As described earlier, there are several mechanisms employed by organisms in host-pathogen interactions. DAF-7 has strongly been implicated in innate immunity with regards to pathogen avoidance. Odorant molecules or other metabolites secreted by pathogenic bacteria are one of the causes of aversive behavior in C. elegans. For example, P. aeruginosa produces the secondary metabolites phenazine-1-carboxamide and pyochelin. Chemosensory detection of these metabolites activates a G-protein-signaling pathway in ASJ neurons, which results in secretion of DAF-7. This activation of DAF-7/TGF-β signaling correlated with pathogen avoidance of P. aeruginosa (Meisel et al., 2014). A later paper showed that while chemosensation of these metabolites led to induction of DAF-7 in ASJ neurons, detection of these metabolites was not required for avoidance behavior (Singh and Aballay, 2019b). Instead, intestinal colonization by PA14, and subsequent intestinal bloating, was required for DAF-7-mediated pathogen avoidance (Singh and Aballay, 2019b; 2019a). Furthermore, daf-7(e1372) mutant animals show a partial loss of avoidance of PA14 (Singh and Aballay, 2019a) and loss of preference of E. coli over P. aeruginosa (Singh and Aballay, 2019b). Two other odorant molecules secreted by pathogenic bacteria are 2-nonanone and 1-undecene, which cause activation of the unfolded protein response of the endoplasmic reticulum (UPRER) when detected (De-Souza et al., 2022). DAF-7 has been shown to be required for avoidance of 2-nonanone (Harris et al., 2019), and required for UPRER activation in response to 1-undecene (De-Souza et al., 2022). Interestingly, a 24 h exposure to 1-undecene in WT animals had a hormetic effect, causing extended lifespan. This effect was mediated through the DAF-7-dependent UPRER response (De-Souza et al., 2022) and indicates that induction of an immune response can extend lifespan.
DAF-7 expression in ASI neurons has been shown to be necessary for pathogen avoidance. AMPylase FIC-1 overexpression suppresses DAF-7 production in ASI neurons, which results in decreased pathogen avoidance (Hernandez-Lima et al., 2022). Another study found that exposure to a pathogen’s isolated and purified small RNAs was sufficient for C. elegans to exhibit avoidance behavior, as well as in four subsequent progeny generations (Kaletsky et al., 2020). Animals that experience a brief exposure and subsequent escape from PA14 transmit learned PA14 avoidance epigenetically to progeny and grandprogeny. This “training” encourages their survival. Transgenerationally inherited pathogen avoidance is mediated by Piwi/PRG-1 Argonaute and TGF-β signaling, specifically DAF-7 expression in the ASI neurons (Moore et al., 2019), demonstrating again the critical requirement for DAF-7 expression in the ASI neurons. These last two studies highlight the benefits of C. elegans as a model system to study innate immunity, where the short lifespan allows for transgenerational studies to be done with greater ease.
With regards to transcriptional response to pathogens, a 5 h exposure to P. aeruginosa PA14 is sufficient to upregulate DAF-7 mRNA levels in young adults (Singh and Aballay, 2019a). DAF-7 induction using a GFP reporter is seen in ASI neurons after 24 h of PA14 infection, or 24 h of PA14 small RNA exposure (Kaletsky et al., 2020). DAF-7::GFP induction is also seen in ASJ neurons (Meisel et al., 2014). However, one study found a decrease in DAF-7::GFP after a 4 h PA14 infection at the second larval stage (Jensen et al., 2010). This stage-specific difference presumably would increase dauer pheromone production, which would encourage dauer formation. It is possible this is an immune strategy, however further studies are necessary to elucidate this. Another instance of transcriptional response is how DAF-7 mediates a defense response triggered by detection of hydrogen peroxide. Across many species, hydrogen peroxide is secreted from cells, to attack or to defend against other species. C. elegans encounter hydrogen peroxide in the wild from plant matter, as well as from bacterial pathogens, which secrete the compound and can cause damage to the worms. Thus, C. elegans require robust resistance mechanisms against hydrogen peroxide to promote their own survival. This peroxide resistance is regulated by DAF-7 secretion from ASI neurons, received by interneurons, and followed by transcription of insulin genes. DAF-2 is then activated by the insulins, which independently inhibit DAF-16 and SKN-1, resulting in improved peroxide resistance (Schiffer et al., 2020). Interestingly, this DAF-7 and IIS cascade depends on the presence of E. coli. When E. coli is abundant, a freeloading strategy will be taken, where animals will not induce their own hydrogen peroxide degrading catalases, and instead rely on catalases produced by E. coli. In the absence of E. coli, a self-defense strategy will be taken, and catalases will be induced without reliance on gut microbiota (Schiffer et al., 2020). This affirms how important host-environment dynamics are in regulating immune response, and ultimately, survival.
TIG-2, TIG-3, and UNC-129
Until very recently, there were no published functions for TIG-2 or TIG-3. However, TIG-2, TIG-3 and UNC-129 were recently shown to play a role in neuronal migration (Baltaci et al., 2022). In this function, they act non-redundantly through an atypical signaling pathway. The ligands act solely through SMA-6, the type I receptor, without a type II receptor, to regulate neural development (Baltaci et al., 2022). The pathways and functions of TIG-2, TIG-3 and UNC-129 are still being elucidated, and this recent work indicates that they may mediate signals differently from DAF-7 and DBL-1. Furthermore, a function for TIG-2 in regulating neuromuscular junctions (NMJs) was recently identified (Cheng et al., 2022). They found that tig-2 mutants had slower locomotion, increased cholinergic synapse density, decreased NMJ neurotransmitter release, as well as decreased muscle mitochondria and ATP production. In addition to these developmental functions for TIG-2 and TIG-3, another recent study demonstrated that all five TGF-β ligands are involved in regulating the C. elegans innate immune response (Ciccarelli et al., 2023b) (Figure 2). Mutations in any of these ligands result in decreased survival on P. luminescens. Genetic evidence and structural modeling suggest that in this role, TIG-2 and TIG-3 work together while DBL-1 and DAF-7 work with each other (Ciccarelli et al., 2023b). This result shows unanticipated cooperativity between TGF-β/Activin and BMP family ligands and has implications for how an acute signaling response can be distinguished from developmental signaling.
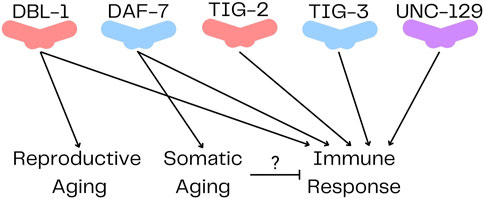
FIGURE 2. TGF-β Ligands in Caenorhabditis elegans. DBL-1 and TIG-2 are members of the BMP family; DAF-7 and TIG-3 are related to TGF-β/Activin; and UNC-129 is more divergent. All five ligands have been shown to regulate immune response, while only DBL-1 regulates reproductive aging, and DAF-7 regulates somatic aging.
TGF-β signaling crosstalk with IIS
The canonical C. elegans IIS pathway begins with insulin-like ligands, which bind and activate or inhibit DAF-2. Receptor activation recruits phosphoinositide-3 kinase, AGE-1/PI3K, which initiates a signaling cascade that includes PIP3, and the serine/threonine kinases PDK-1, AKT-1, and AKT-2 (Dorman et al., 1995; Morris et al., 1996; Paradis and Ruvkun, 1998; Paradis et al., 1999). Then, transcription factors are inhibited, including DAF-16/FOXO and SKN-1/Nrf2, preventing activation of downstream target genes that promote longevity. Secondary transcriptional outputs of IIS include the heat shock transcription factor HSF-1, which is activated by the IIS cascade (Morley and Morimoto, 2004; Chiang et al., 2012) and promotes longevity by cooperating with DAF-16 (Hsu et al., 2003). DAF-2 also activates TOR signaling, which inhibits PHA-4/FoxA to restrict normal growth and longevity (Sheaffer et al., 2008). Separately, the transcription factor PHA-4 was identified as mediating longevity via dietary restriction (Panowski et al., 2007).
Mutations in daf-2 extend lifespan by decreasing IIS activity and the PI3K cascade, thus activating the transcription factors and downstream targets. Decreased IIS results in changes to proteostasis, RNA homeostasis, oxidative stress, pathogen resistance, lipid and amino acid metabolism and endocrine signaling, all of which contribute to the longevity phenotype (Lee and Lee, 2022). The identification of this longevity pathway in C. elegans demonstrated the utility of C. elegans for discovery in the field of aging, and IIS-dependent aging has been shown to be conserved across many species, including rodent models and humans (Kenyon, 2010).
DBL-1 has numerous modes of interaction with the longevity-regulating IIS pathway. Both DBL-1 and DAF-2/InsR regulate reproductive aging, modulating the length of time before female reproductive capacity ceases (Luo et al., 2009; 2010). DBl-1 and DAF-2/InsR also regulate body size (Qi et al., 2017; Clark et al., 2018). In both roles, the pathways act independently. In the regulation of lipid metabolism, DBL-1 signaling negatively regulates DAF-2/InsR signaling, so that loss of daf-2 is genetically epistatic to loss of dbl-1, or its signaling components (Clark et al., 2018; 2021). The DBL-1 pathway transcriptionally regulates several insulin-like ligand genes (Liang et al., 2007; Luo et al., 2010). The DBL-1 pathway also regulates a subset of DAF-16/FOXO transcriptional targets, like fat-6 and fat-7 (Liang et al., 2007). SMA-3 acts upstream of insulin-like ligand INS-4 in the regulation of fat storage, which carries out homeostatic functions through DAF-16/FOXO (Clark et al., 2021). An antagonistic interaction between DBL-1 signaling and DAF-2/InsR was revealed by the partial suppression of daf-2 phenotypes of longevity, dauer formation, and autophagy by loss of dbl-1 or its signaling components (Clark et al., 2018). These two pathways have also been shown to interact in first larval stage (L1) arrest and Q cell divisions (Kaplan et al., 2015; Zheng et al., 2018).
The DAF-7 pathway also has many instances of crosstalk with IIS. DAF-7 signaling acts upstream of DAF-2/InsR signaling (Shaw et al., 2007), and interacts in a cooperative fashion with DAF-2/InsR in dauer formation, lipid metabolism, and longevity (Ogg et al., 1997; Narasimhan et al., 2011). It is hypothesized that these two pathways cooperate so that multiple signal inputs can be used to inform dauer and lifespan decisions (Shaw et al., 2007). Some of this signal integration may occur through DAF-12/nuclear hormone receptor (Antebi et al., 2000; Snow and Larsen, 2000). The DAF-7 pathway functions epistatically with IIS in regulating nictation behavior (Lee et al., 2017). Nictation is a waving behavior that enables dauer animals to “hitchhike” on more mobile animals as a way to move to an area with more favorable conditions, such as lower population density and an increased abundance of food. Furthermore, expression of several insulin-like ligands, such as INS-7 and INS-18, are regulated by the DAF-7 pathway (Liu et al., 2004; Shaw et al., 2007; Narasimhan et al., 2011). Lastly, the DAF-7 pathway promotes secretion of insulin-like ligand DAF-28 (Park et al., 2012).
Discussion
Studies using C. elegans have greatly contributed to understanding the functions and mechanisms of TGF-β signaling. This review focuses on the multifaceted connections between TGF-β/Activin and BMP signaling and their roles in regulating longevity and immunity in C. elegans. Interestingly, delving into these physiological aspects has unveiled many instances of non-canonical signaling, which may have implications for mammalian signaling and thus deserve more extensive study. Because TGF-β signaling is one of the key pathways co-regulating aging and immunity (Fabian et al., 2021), mechanisms identified in C. elegans are anticipated to shed light on these processes in all organisms. Notably, one study examined protein signatures of centenarians and identified TGF-β signaling, as well as IIS, as hallmark pathways of healthy aging (Sebastiani et al., 2021). Leveraging the advantages of the C. elegans system, research can now explore additional associations between aging and immunity, the relationship of the microbiome with these physiologies, crosstalk between TGF-β and IIS pathways, and mechanisms that differentiate TGF-β/Activin and BMP family activities. Additionally, the short-lived nature of C. elegans offers a unique opportunity to investigate transgenerational effects, paving the way for further groundbreaking studies in this field.
Author contributions
KY: Writing first draft and editing. CS-D: Editing manuscript. All authors contributed to the article and approved the submitted version.
Acknowledgments
Work in the authors’ lab is funded by R21AG075315 and R15GM112147 to CS-D. We are grateful to Dr. Alicia Meléndez for critical reading of the manuscript.
Conflict of interest
The authors declare that the research was conducted in the absence of any commercial or financial relationships that could be construed as a potential conflict of interest.
Publisher’s note
All claims expressed in this article are solely those of the authors and do not necessarily represent those of their affiliated organizations, or those of the publisher, the editors and the reviewers. Any product that may be evaluated in this article, or claim that may be made by its manufacturer, is not guaranteed or endorsed by the publisher.
References
Adu-Gyamfi, E. A., Djankpa, F. T., Nelson, W., Czika, A., Sah, S. K., Lamptey, J., et al. (2020). Activin and inhibin signaling: from regulation of physiology to involvement in the pathology of the female reproductive system. Cytokine 133, 155105. doi:10.1016/j.cyto.2020.155105
Ahlberg, S. H., Joutsjoki, V., and Korhonen, H. J. (2015). Potential of lactic acid bacteria in aflatoxin risk mitigation. Int. J. Food Microbiol. 207, 87–102. doi:10.1016/j.ijfoodmicro.2015.04.042
Akha, A. A. S. (2018). Aging and the immune system: an overview. J. Immunol. Methods 463, 21–26. doi:10.1016/j.jim.2018.08.005
Almedom, R. B., Liewald, J. F., Hernando, G., Schultheis, C., Rayes, D., Pan, J., et al. (2009). An ER-resident membrane protein complex regulates nicotinic acetylcholine receptor subunit composition at the synapse. Embo J. 28, 2636–2649. doi:10.1038/emboj.2009.204
Alper, S., McBride, S. J., Lackford, B., Freedman, J. H., and Schwartz, D. A. (2007). Specificity and complexity of the Caenorhabditis elegans innate immune response. Mol. Cell Biol. 27, 5544–5553. doi:10.1128/mcb.02070-06
Anderson, S. M., Cheesman, H. K., Peterson, N. D., Salisbury, J. E., Soukas, A. A., and Pukkila-Worley, R. (2019). The fatty acid oleate is required for innate immune activation and pathogen defense in Caenorhabditis elegans. Plos Pathog. 15, e1007893. doi:10.1371/journal.ppat.1007893
Antebi, A., Yeh, W.-H., Tait, D., Hedgecock, E. M., and Riddle, D. L. (2000). daf-12 encodes a nuclear receptor that regulates the dauer diapause and developmental age in C. elegans. Gene Dev. 14, 1512–1527. doi:10.1101/gad.14.12.1512
Arneaud, S. L. B., McClendon, J., Tatge, L., Watterson, A., Zuurbier, K. R., Madhu, B., et al. (2022). Reduced bone morphogenic protein signaling along the gut–neuron axis by heat shock factor promotes longevity. Aging Cell 21, e13693. doi:10.1111/acel.13693
Ayres, J. S., and Schneider, D. S. (2008). A signaling protease required for melanization in Drosophila affects resistance and tolerance of infections. Plos Biol. 6, 2764–2773. doi:10.1371/journal.pbio.0060305
Baird, S. E., and Ellazar, S. A. (1999). TGFbeta-like signaling and spicule development in Caenorhabditis elegans. Dev. Biol. 212, 93–100. doi:10.1006/dbio.1999.9322
Baltaci, O., Pedersen, M. E., Sherry, T., Handley, A., Snieckute, G., Cao, W., et al. (2022). Atypical TGF-β signaling controls neuronal guidance in Caenorhabditis elegans. Iscience 25, 103791. doi:10.1016/j.isci.2022.103791
Barna, J., Princz, A., Kosztelnik, M., Hargitai, B., Takács-Vellai, K., and Vellai, T. (2012). Heat shock factor-1 intertwines insulin/IGF-1, TGF-β and cGMP signaling to control development and aging. Bmc Dev. Biol. 12, 32. doi:10.1186/1471-213x-12-32
Batlle, E., and Massagué, J. (2019). Transforming growth factor-β signaling in immunity and cancer. Immunity 50, 924–940. doi:10.1016/j.immuni.2019.03.024
Berg, M., Monnin, D., Cho, J., Nelson, L., Crits-Christoph, A., and Shapira, M. (2019). TGFβ/BMP immune signaling affects abundance and function of C. elegans gut commensals. Nat. Commun. 10, 604. doi:10.1038/s41467-019-08379-8
Brenner, S. (1974). The genetics of Caenorhabditis elegans. Genetics 77, 71–94. doi:10.1093/genetics/77.1.71
Caldwell, R. M., Schafer, J. F., Compton, L. E., and Patterson, F. L. (1958). Tolerance to cereal leaf rusts. Science 128, 714–715. doi:10.1126/science.128.3326.714
C. elegans Sequencing Consortium (1998). Genome sequence of the nematode C. elegans: A platform for investigating biology. Science 282, 2012–2018. doi:10.1126/science.282.5396.2012
Chauve, L., Hodge, F., Murdoch, S., Masoudzadeh, F., Mann, H.-J., Lopez-Clavijo, A. F., et al. (2021). Neuronal HSF-1 coordinates the propagation of fat desaturation across tissues to enable adaptation to high temperatures in C. elegans. Plos Biol. 19, e3001431. doi:10.1371/journal.pbio.3001431
Chen, W., and Dijke, P. (2016). Immunoregulation by members of the TGFβ superfamily. Nat. Rev. Immunol. 16, 723–740. doi:10.1038/nri.2016.112
Cheng, X., Yan, Z., Su, Z., and Liu, J. (2022). The transforming growth factor beta ligand TIG-2 modulates the function of neuromuscular junction and muscle energy metabolism in Caenorhabditis elegans. Front. Mol. Neurosci. 15, 962974. doi:10.3389/fnmol.2022.962974
Chiang, W.-C., Ching, T.-T., Lee, H. C., Mousigian, C., and Hsu, A.-L. (2012). HSF-1 regulators DDL-1/2 link insulin-like signaling to heat-shock responses and modulation of longevity. Cell 148, 322–334. doi:10.1016/j.cell.2011.12.019
Choi, R., Bodkhe, R., Pees, B., Kim, D., Berg, M., Monnin, D., et al. (2023). An Enterobacteriaceae bloom in aging animals is restrained by the gut microbiome. 2023.06.13.544815. bioRxiv. doi:10.1101/2023.06.13.544815
Chomyshen, S. C., Tabarraei, H., and Wu, C.-W. (2022). Translational suppression via IFG-1/eIF4G inhibits stress-induced RNA alternative splicing in Caenorhabditis elegans. Genetics 221, iyac075. doi:10.1093/genetics/iyac075
Ciccarelli, E. J., Bendelstein, M., and Savage-Dunn, C. (2023a). BMP signaling in the C. elegans immune response to bacterial pathogen regulates anti-microbial peptide expression through cell non-autonomous activity. bioRxiv. doi:10.1101/2023.03.06.531324
Ciccarelli, E. J., Wing, Z., Bendelstein, M., Johal, R. K., Singh, G., Monas, A., et al. (2023b). TGF-Β ligand interactions in the response of Caenorhabditis elegans to bacterial pathogens. bioRxiv. doi:10.1101/2023.05.05.539606
Clark, J. F., Ciccarelli, E. J., Kayastha, P., Ranepura, G., Yamamoto, K. K., Hasan, M. S., et al. (2021). BMP pathway regulation of insulin signaling components promotes lipid storage in Caenorhabditis elegans. Plos Genet. 17, e1009836. doi:10.1371/journal.pgen.1009836
Clark, J. F., Meade, M., Ranepura, G., Hall, D. H., and Savage-Dunn, C. (2018). Caenorhabditis elegans DBL-1/BMP regulates lipid accumulation via interaction with insulin signaling. Genes Genomes Genet. 8, 343–351. doi:10.1534/g3.117.300416
Dalfó, D., Michaelson, D., and Hubbard, E. J. A. (2012). Sensory regulation of the C. elegans germline through TGF-β-dependent signaling in the niche. Curr. Biol. 22, 712–719. doi:10.1016/j.cub.2012.02.064
Davidson, E. B., Scharstuhl, A., Vitters, E., Kraan, P., and Berg, W. (2005). Reduced transforming growth factor-beta signaling in cartilage of old mice: role in impaired repair capacity. Arthritis Res. Ther. 7, R1338–R1347. doi:10.1186/ar1833
De-Souza, E. A., Thompson, M. A., and Taylor, R. C. (2022). Olfactory chemosensation extends lifespan through TGF-β signaling and UPR activation. 2022.10.12.511902. Biorxiv. doi:10.1101/2022.10.12.511902
Deshpande, R., Lee, B., Qiao, Y., and Grewal, S. S. (2022). TOR signalling is required for host lipid metabolic remodelling and survival following enteric infection in Drosophila. Dis. Model Mech. 15, dmm049551. doi:10.1242/dmm.049551
Dijke, P., and Hill, C. S. (2004). New insights into TGF-β–Smad signalling. Trends Biochem. Sci. 29, 265–273. doi:10.1016/j.tibs.2004.03.008
Dorman, J. B., Albinder, B., Shroyer, T., and Kenyon, C. (1995). The age-1 and daf-2 genes function in a common pathway to control the lifespan of Caenorhabditis elegans. Genetics 141, 1399–1406. doi:10.1093/genetics/141.4.1399
Duerr, J. S., Han, H., Fields, S. D., and Rand, J. B. (2008). Identification of major classes of cholinergic neurons in the nematode Caenorhabditis elegans. J. Comp. Neurol. 506, 398–408. doi:10.1002/cne.21551
Estevez, M., Attisano, L., Wrana, J. L., Albert, P. S., Massagué, J., and Riddle, D. L. (1993). The daf-4 gene encodes a bone morphogenetic protein receptor controlling C. elegans dauer larva development. Nature 365, 644–649. doi:10.1038/365644a0
Evans, E. A., Kawli, T., and Tan, M.-W. (2008). Pseudomonas aeruginosa suppresses host immunity by activating the DAF-2 insulin-like signaling pathway in Caenorhabditis elegans. Plos Pathog. 4, e1000175. doi:10.1371/journal.ppat.1000175
Ewbank, J. (2006). Signaling in the immune response. Wormbook 1–12, 1–12. doi:10.1895/wormbook.1.83.1
Fabian, D. K., Fuentealba, M., Dönertaş, H. M., Partridge, L., and Thornton, J. M. (2021). Functional conservation in genes and pathways linking ageing and immunity. Immun. Ageing 18, 23. doi:10.1186/s12979-021-00232-1
Foehr, M. L., Lindy, A. S., Fairbank, R. C., Amin, N. M., Xu, M., Yanowitz, J., et al. (2006). An antagonistic role for the C. elegans Schnurri homolog SMA-9 in modulating TGFbeta signaling during mesodermal patterning. Development 133, 2887–2896. doi:10.1242/dev.02476
Fujiwara, M., Sengupta, P., and McIntire, S. L. (2002). Regulation of body size and behavioral state of C. elegans by sensory perception and the EGL-4 cGMP-dependent protein kinase. Neuron 36, 1091–1102. doi:10.1016/s0896-6273(02)01093-0
Garsin, D. A., Villanueva, J. M., Begun, J., Kim, D. H., Sifri, C. D., Calderwood, S. B., et al. (2003). Long-lived C. elegans daf-2 mutants are resistant to bacterial pathogens. Science 300, 1921. doi:10.1126/science.1080147
Georgi, L. L., Albert, P. S., and Riddle, D. L. (1990). daf-1, a C. elegans gene controlling dauer larva development, encodes a novel receptor protein kinase. Cell 61, 635–645. doi:10.1016/0092-8674(90)90475-t
Gerisch, B., Weitzel, C., Kober-Eisermann, C., Rottiers, V., and Antebi, A. (2001). A hormonal signaling pathway influencing C. elegans metabolism, reproductive development, and life span. Dev. Cell 1, 841–851. doi:10.1016/s1534-5807(01)00085-5
Gleason, R. J., Akintobi, A. M., Grant, B. D., and Padgett, R. W. (2014). BMP signaling requires retromer-dependent recycling of the type I receptor. Proc. Natl. Acad. Sci. 111, 2578–2583. doi:10.1073/pnas.1319947111
Golden, J. W., and Riddle, D. L. (1984). The Caenorhabditis elegans dauer larva: developmental effects of pheromone, food, and temperature. Dev. Biol. 102, 368–378. doi:10.1016/0012-1606(84)90201-x
Goodman, M. B., and Savage-Dunn, C. (2022). Reciprocal interactions between transforming growth factor beta signaling and collagens: insights from Caenorhabditis elegans. Dev. Dyn. 251, 47–60. doi:10.1002/dvdy.423
Goszczynski, B., Captan, V. V., Danielson, A. M., Lancaster, B. R., and McGhee, J. D. (2016). A 44 bp intestine-specific hermaphrodite-specific enhancer from the C. elegans vit-2 vitellogenin gene is directly regulated by ELT-2, MAB-3, FKH-9 and DAF-16 and indirectly regulated by the germline, by daf-2/insulin signaling and by the TGF-β/Sma/Mab pathway. Dev. Biol. 413, 112–127. doi:10.1016/j.ydbio.2016.02.031
Gough, N. R., Xiang, X., and Mishra, L. (2021). TGF-Β signaling in liver, pancreas, and gastrointestinal diseases and cancer. Gastroenterology 161, 434–452.e15. doi:10.1053/j.gastro.2021.04.064
Graca, L. S. da, Zimmerman, K. K., Mitchell, M. C., Kozhan-Gorodetska, M., Sekiewicz, K., Morales, Y., et al. (2003). DAF-5 is a Ski oncoprotein homolog that functions in a neuronal TGFβpathway to regulate C. elegans dauer development. Development 131, 435–446. doi:10.1242/dev.00922
Greer, E. R., Pérez, C. L., Gilst, M. R. V., Lee, B. H., and Ashrafi, K. (2008). Neural and molecular dissection of a C. elegans sensory circuit that regulates fat and feeding. Cell Metab. 8, 118–131. doi:10.1016/j.cmet.2008.06.005
Gumienny, T. L., and Savage-Dunn, C. (2013). TGF-β signaling in C. elegans. Wormbook, 1–34. doi:10.1895/wormbook.1.22.2
Haçariz, O., Viau, C., Karimian, F., and Xia, J. (2021). The symbiotic relationship between Caenorhabditis elegans and members of its microbiome contributes to worm fitness and lifespan extension. Bmc Genomics 22, 364. doi:10.1186/s12864-021-07695-y
Haque, R., and Nazir, A. (2016). SMAD transcription factor, sma-9, attunes TGF-β signaling cascade towards modulating amyloid beta aggregation and associated outcome in transgenic C. elegans. Mol. Neurobiol. 53, 109–119. doi:10.1007/s12035-014-8988-y
Harris, G., Wu, T., Linfield, G., Choi, M.-K., Liu, H., and Zhang, Y. (2019). Molecular and cellular modulators for multisensory integration in C. elegans. Plos Genet. 15, e1007706. doi:10.1371/journal.pgen.1007706
Harris, T. W., Chen, N., Cunningham, F., Tello-Ruiz, M., Antoshechkin, I., Bastiani, C., et al. (2004). WormBase: a multi-species resource for nematode biology and genomics. Nucleic Acids Res. 32, D411–D417. doi:10.1093/nar/gkh066
He, K., Zhou, T., Shao, J., Ren, X., Zhao, Z., and Liu, D. (2014). Dynamic regulation of genetic pathways and targets during aging in Caenorhabditis elegans. Aging Albany N. Y. 6, 215–230. doi:10.18632/aging.100648
He, Y., Hao, F., Fu, H., Tian, G., Zhang, Y., Fu, K., et al. (2023). N-glycosylated intestinal protein BCF-1 shapes microbial colonization by binding bacteria via its fimbrial protein. Cell Rep. 42, 111993. doi:10.1016/j.celrep.2023.111993
Hernandez-Lima, M. A., Champion, M., Mattiola, Z., and Truttmann, M. C. (2022). The AMPylase FIC-1 modulates TGF-β signaling in Caenorhabditis elegans. Front. Mol. Neurosci. 15, 912734. doi:10.3389/fnmol.2022.912734
Herpin, A., Lelong, C., and Favrel, P. (2004). Transforming growth factor-β-related proteins: an ancestral and widespread superfamily of cytokines in metazoans. Dev. Comp. Immunol. 28, 461–485. doi:10.1016/j.dci.2003.09.007
Hirose, T., Nakano, Y., Nagamatsu, Y., Misumi, T., Ohta, H., and Ohshima, Y. (2003). Cyclic GMP-dependent protein kinase EGL-4 controls body size and lifespan in C. elegans. Development 130, 1089–1099. doi:10.1242/dev.00330
Hsu, A.-L., Murphy, C. T., and Kenyon, C. (2003). Regulation of aging and age-related disease by DAF-16 and heat-shock factor. Science 300, 1142–1145. doi:10.1126/science.1083701
Ihara, S., Hirata, Y., Serizawa, T., Suzuki, N., Sakitani, K., Kinoshita, H., et al. (2016). TGF-Β signaling in dendritic cells governs colonic homeostasis by controlling epithelial differentiation and the luminal microbiota. J. Immunol. 196, 4603–4613. doi:10.4049/jimmunol.1502548
Inoue, T., and Thomas, J. H. (2000). Targets of TGF-β signaling in Caenorhabditis elegans dauer formation. Dev. Biol. 217, 192–204. doi:10.1006/dbio.1999.9545
Inoue, Y., and Imamura, T. (2008). Regulation of TGF-β family signaling by E3 ubiquitin ligases. Cancer Sci. 99, 2107–2112. doi:10.1111/j.1349-7006.2008.00925.x
Jensen, V. L., Simonsen, K. T., Lee, Y.-H., Park, D., and Riddle, D. L. (2010). RNAi screen of DAF-16/FOXO target genes in C. elegans links pathogenesis and dauer formation. Plos One 5, e15902. doi:10.1371/journal.pone.0015902
Jia, K., Albert, P. S., and Riddle, D. L. (2002). DAF-9, a cytochrome P450 regulating C. elegans larval development and adult longevity. Development 129, 221–231. doi:10.1242/dev.129.1.221
Julien-Gau, I., Schmidt, M., and Kurz, C. L. (2014). f57f4.4p::gfp as a fluorescent reporter for analysis of the C. elegans response to bacterial infection. Dev. Comp. Immunol. 42, 132–137. doi:10.1016/j.dci.2013.08.024
Kaletsky, R., Moore, R. S., Vrla, G. D., Parsons, L. R., Gitai, Z., and Murphy, C. T. (2020). C. elegans interprets bacterial non-coding RNAs to learn pathogenic avoidance. Nature 586, 445–451. doi:10.1038/s41586-020-2699-5
Kaplan, R. E. W., Chen, Y., Moore, B. T., Jordan, J. M., Maxwell, C. S., Schindler, A. J., et al. (2015). dbl-1/TGF-β and daf-12/NHR signaling mediate cell-nonautonomous effects of daf-16/FOXO on starvation-induced developmental arrest. Plos Genet. 11, e1005731. doi:10.1371/journal.pgen.1005731
Kenyon, C., Chang, J., Gensch, E., Rudner, A., and Tabtiang, R. (1993). A C. elegans mutant that lives twice as long as wild type. Nature 366, 461–464. doi:10.1038/366461a0
Kerry, S., TeKippe, M., Gaddis, N. C., and Aballay, A. (2006). GATA transcription factor required for immunity to bacterial and fungal pathogens. Plos One 1, e77. doi:10.1371/journal.pone.0000077
Kim, D. H., and Ewbank, J. J. (2018). Signaling in the innate immune response. Wormbook 2018, 1–35. doi:10.1895/wormbook.1.83.2
Kimura, K. D., Tissenbaum, H. A., Liu, Y., and Ruvkun, G. (1997). daf-2, an insulin receptor-like gene that regulates longevity and diapause in Caenorhabditis elegans. Science 277, 942–946. doi:10.1126/science.277.5328.942
Kratsios, P., Stolfi, A., Levine, M., and Hobert, O. (2012). Coordinated regulation of cholinergic motor neuron traits through a conserved terminal selector gene. Nat. Neurosci. 15, 205–214. doi:10.1038/nn.2989
Krishna, S., Maduzia, L. L., and Padgett, R. W. (1999). Specificity of TGFbeta signaling is conferred by distinct type I receptors and their associated SMAD proteins in Caenorhabditis elegans. Development 126, 251–260. doi:10.1242/dev.126.2.251
Kurz, C. L., and Tan, M. (2004). Regulation of aging and innate immunity in C. elegans. Aging Cell 3, 185–193. doi:10.1111/j.1474-9728.2004.00108.x
Kuwabara, P. E., and O’Neil, N. (2001). The use of functional genomics in C. elegans for studying human development and disease. J. Inherit. Metab. Dis. 24, 127–138. doi:10.1023/a:1010306731764
Kwon, G., Lee, J., Koh, J.-H., and Lim, Y.-H. (2018). Lifespan extension of Caenorhabditis elegans by Butyricicoccus pullicaecorum and Megasphaera elsdenii with probiotic potential. Curr. Microbiol. 75, 557–564. doi:10.1007/s00284-017-1416-6
Kwon, G., Lee, J., and Lim, Y.-H. (2016). Dairy Propionibacterium extends the mean lifespan of Caenorhabditis elegans via activation of the innate immune system. Sci. Rep. 6, 31713. doi:10.1038/srep31713
Lai, C.-H., Chou, C.-Y., Ch’ang, L.-Y., Liu, C.-S., and Lin, W. (2000). Identification of novel human genes evolutionarily conserved in Caenorhabditis elegans by comparative proteomics. Genome Res. 10, 703–713. doi:10.1101/gr.10.5.703
Lakdawala, M. F., Madhu, B., Faure, L., Vora, M., Padgett, R. W., and Gumienny, T. L. (2019). Genetic interactions between the DBL-1/BMP-like pathway and dpy body size–associated genes in Caenorhabditis elegans. Mol. Biol. Cell 30, 3151–3160. doi:10.1091/mbc.e19-09-0500
Larsen, P. L., Albert, P. S., and Riddle, D. L. (1995). Genes that regulate both development and longevity in Caenorhabditis elegans. Genetics 139, 1567–1583. doi:10.1093/genetics/139.4.1567
Laureano, G., Cavaco, A. R., Matos, A. R., and Figueiredo, A. (2021). Fatty acid desaturases: uncovering their involvement in grapevine defence against downy mildew. Int. J. Mol. Sci. 22, 5473. doi:10.3390/ijms22115473
Lee, D., Lee, H., Kim, N., Lim, D. S., and Lee, J. (2017). Regulation of a hitchhiking behavior by neuronal insulin and TGF-β signaling in the nematode Caenorhabditis elegans. Biochem. Biophys. Res. Commun. 484, 323–330. doi:10.1016/j.bbrc.2017.01.113
Lee, H., and Lee, S.-J. V. (2022). Recent progress in regulation of aging by insulin/IGF-1 signaling in Caenorhabditis elegans. Mol. Cells 45, 763–770. doi:10.14348/molcells.2022.0097
Lee, Y., Jung, Y., Jeong, D.-E., Hwang, W., Ham, S., Park, H.-E. H., et al. (2021). Reduced insulin/IGF1 signaling prevents immune aging via ZIP-10/bZIP–mediated feedforward loop. J. Cell Biol. 220, e202006174. doi:10.1083/jcb.202006174
Leroy, M., Mosser, T., Manière, X., Alvarez, D. F., and Matic, I. (2012). Pathogen-induced Caenorhabditis elegans developmental plasticity has a hormetic effect on the resistance to biotic and abiotic stresses. Bmc Evol. Biol. 12, 187. doi:10.1186/1471-2148-12-187
Li, M. O., and Flavell, R. A. (2008). TGF-Beta: a master of all T cell trades. Cell 134, 392–404. doi:10.1016/j.cell.2008.07.025
Liang, J., Lints, R., Foehr, M. L., Tokarz, R., Yu, L., Emmons, S. W., et al. (2003). The Caenorhabditis elegans schnurri homolog sma-9mediates stage- and cell type-specific responses to DBL-1 BMP-related signaling. Development 130, 6453–6464. doi:10.1242/dev.00863
Liang, J., Yu, L., Yin, J., and Savage-Dunn, C. (2007). Transcriptional repressor and activator activities of SMA-9 contribute differentially to BMP-related signaling outputs. Dev. Biol. 305, 714–725. doi:10.1016/j.ydbio.2007.02.038
Liu, H., Zhang, R., and Wang, D. (2020a). Response of DBL-1/TGF-β signaling-mediated neuron-intestine communication to nanopolystyrene in nematode Caenorhabditis elegans. Sci. Total Environ. 745, 141047. doi:10.1016/j.scitotenv.2020.141047
Liu, T., Zimmerman, K. K., and Patterson, G. I. (2004). Regulation of signaling genes by TGFbeta during entry into dauer diapause in C. elegans. Bmc Dev. Biol. 4, 11. doi:10.1186/1471-213x-4-11
Liu, Z., Shi, H., Nzessi, A. K., Norris, A., Grant, B. D., and Liu, J. (2020b). Tetraspanins TSP-12 and TSP-14 function redundantly to regulate the trafficking of the type II BMP receptor in Caenorhabditis elegans. Proc. Natl. Acad. Sci. 117, 2968–2977. doi:10.1073/pnas.1918807117
Loffredo, F. S., Steinhauser, M. L., Jay, S. M., Gannon, J., Pancoast, J. R., Yalamanchi, P., et al. (2013). Growth differentiation factor 11 is a circulating factor that reverses age-related cardiac hypertrophy. Cell 153, 828–839. doi:10.1016/j.cell.2013.04.015
López-Otín, C., Blasco, M. A., Partridge, L., Serrano, M., and Kroemer, G. (2013). The hallmarks of aging. Cell 153, 1194–1217. doi:10.1016/j.cell.2013.05.039
Lu, Y., Liu, H., Yang, X., Liu, J., Dai, M., Wu, J., et al. (2020). Microarray analysis of lncRNA and mRNA reveals enhanced lipolysis along with metabolic remodeling in mice infected with larval echinococcus granulosus. Front. Physiol. 11, 1078. doi:10.3389/fphys.2020.01078
Lucas, M. P., Jiménez, M., Sánchez-Pavón, P., Sáez, A. G., and Lozano, E. (2021). SMA-10 is a non-canonical member of the TGF-β sma/mab pathway and immunity regulator via the DAF-2 insulin receptor in Caenorhabditis elegans. Int. J. Mol. Sci. 22, 638. doi:10.3390/ijms22020638
Luo, S., Kleemann, G. A., Ashraf, J. M., Shaw, W. M., and Murphy, C. T. (2010). TGF-Β and insulin signaling regulate reproductive aging via oocyte and germline quality maintenance. Cell 143, 299–312. doi:10.1016/j.cell.2010.09.013
Luo, S., Shaw, W. M., Ashraf, J., and Murphy, C. T. (2009). TGF-beta Sma/Mab signaling mutations uncouple reproductive aging from somatic aging. Plos Genet. 5, e1000789. doi:10.1371/journal.pgen.1000789
Ma, Y., Liu, Y., Han, F., Qiu, H., Shi, J., Huang, N., et al. (2021). Growth differentiation factor 11: a “rejuvenation factor” involved in regulation of age-related diseases? Aging (Albany NY) 13, 12258–12272. doi:10.18632/aging.202881
Madaan, U., Faure, L., Chowdhury, A., Ahmed, S., Ciccarelli, E. J., Gumienny, T. L., et al. (2020). Feedback regulation of BMP signaling by Caenorhabditis elegans cuticle collagens. Mol. Biol. Cell 31, 825–832. doi:10.1091/mbc.e19-07-0390
Madaan, U., Yzeiraj, E., Meade, M., Clark, J. F., Rushlow, C. A., and Savage-Dunn, C. (2018). BMP signaling determines body size via transcriptional regulation of collagen genes in Caenorhabditis elegans. Genetics 210, 1355–1367. doi:10.1534/genetics.118.301631
Madhu, B., and Gumienny, T. L. (2021). The DBL-1/TGF-β signaling pathway regulates pathogen-specific innate immune responses in C. elegans. 2021.03.30.437693. Biorxiv. doi:10.1101/2021.03.30.437693
Madhu, B., Lakdawala, M. F., Issac, N. G., and Gumienny, T. L. (2020). Caenorhabditis elegans saposin-like spp-9 is involved in specific innate immune responses. Genes Immun. 21, 301–310. doi:10.1038/s41435-020-0108-6
Mallo, G. V., Kurz, C. L., Couillault, C., Pujol, N., Granjeaud, S., Kohara, Y., et al. (2002). Inducible antibacterial defense system in C. elegans. Curr. Biol. 12, 1209–1214. doi:10.1016/s0960-9822(02)00928-4
Massagué, J., Seoane, J., and Wotton, D. (2005). Smad transcription factors. Gene Dev. 19, 2783–2810. doi:10.1101/gad.1350705
McCulloch, D., and Gems, D. (2003). Body size, insulin/IGF signaling and aging in the nematode Caenorhabditis elegans. Exp. Gerontol. 38, 129–136. doi:10.1016/s0531-5565(02)00147-x
Medzhitov, R., Schneider, D. S., and Soares, M. P. (2012). Disease tolerance as a defense strategy. Science 335, 936–941. doi:10.1126/science.1214935
Meisel, J. D., Panda, O., Mahanti, P., Schroeder, F. C., and Kim, D. H. (2014). Chemosensation of bacterial secondary metabolites modulates neuroendocrine signaling and behavior of C. elegans. Cell 159, 267–280. doi:10.1016/j.cell.2014.09.011
Melzer, D., Pilling, L. C., and Ferrucci, L. (2020). The genetics of human ageing. Nat. Rev. Genet. 21, 88–101. doi:10.1038/s41576-019-0183-6
Meyers, E. A., Gobeske, K. T., Bond, A. M., Jarrett, J. C., Peng, C.-Y., and Kessler, J. A. (2016). Increased bone morphogenetic protein signaling contributes to age-related declines in neurogenesis and cognition. Neurobiol. Aging 38, 164–175. doi:10.1016/j.neurobiolaging.2015.10.035
Millet, A. C., and Ewbank, J. J. (2004). Immunity in Caenorhabditis elegans. Curr. Opin. Immunol. 16, 4–9. doi:10.1016/j.coi.2003.11.005
Mochii, M., Yoshida, S., Morita, K., Kohara, Y., and Ueno, N. (1999). Identification of transforming growth factor-β- regulated genes in Caenorhabditis elegans by differential hybridization of arrayed cDNAs. Proc. Natl. Acad. Sci. 96, 15020–15025. doi:10.1073/pnas.96.26.15020
Moore, R. S., Kaletsky, R., and Murphy, C. T. (2019). Piwi/PRG-1 Argonaute and TGF-β mediate transgenerational learned pathogenic avoidance. Cell 177, 1827–1841. doi:10.1016/j.cell.2019.05.024
Mørch, M. G. M., Møller, K. V., Hesselager, M. O., Harders, R. H., Kidmose, C. L., Buhl, T., et al. (2021). The TGF-β ligand DBL-1 is a key player in a multifaceted probiotic protection against MRSA in C. elegans. Sci. Rep. 11, 10717. doi:10.1038/s41598-021-89831-y
Morgan, X. C., Tickle, T. L., Sokol, H., Gevers, D., Devaney, K. L., Ward, D. V., et al. (2012). Dysfunction of the intestinal microbiome in inflammatory bowel disease and treatment. Genome Biol. 13, R79. doi:10.1186/gb-2012-13-9-r79
Morita, K., Chow, K. L., and Ueno, N. (1999). Regulation of body length and male tail ray pattern formation of Caenorhabditis elegans by a member of TGF-beta family. Development 126, 1337–1347. doi:10.1242/dev.126.6.1337
Morley, J. F., and Morimoto, R. I. (2004). Regulation of longevity in Caenorhabditis elegans by heat shock factor and molecular chaperones. Mol. Biol. Cell 15, 657–664. doi:10.1091/mbc.e03-07-0532
Morris, J. Z., Tissenbaum, H. A., and Ruvkun, G. (1996). A phosphatidylinositol-3-OH kinase family member regulating longevity and diapause in Caenorhabditis elegans. Nature 382, 536–539. doi:10.1038/382536a0
Murphy, C. T., and Hu, P. J. (2013). Insulin/insulin-like growth factor signaling in C. elegans. Wormbook 1–43, 1–43. doi:10.1895/wormbook.1.164.1
Narasimhan, S. D., Yen, K., Bansal, A., Kwon, E.-S., Padmanabhan, S., and Tissenbaum, H. A. (2011). PDP-1 links the TGF-β and IIS pathways to regulate longevity, development, and metabolism. Plos Genet. 7, e1001377. doi:10.1371/journal.pgen.1001377
Nicholas, H. R., and Hodgkin, J. (2002). Innate immunity: the worm fights back. Curr. Biol. 12, R731–R732. doi:10.1016/s0960-9822(02)01249-6
Ogg, S., Paradis, S., Gottlieb, S., Patterson, G. I., Lee, L., Tissenbaum, H. A., et al. (1997). The Fork head transcription factor DAF-16 transduces insulin-like metabolic and longevity signals in C. elegans. Nature 389, 994–999. doi:10.1038/40194
Padgett, R. W., Das, P., and Krishna, S. (1998). TGF-β signaling, Smads, and tumor suppressors. Bioessays 20, 382–390. doi:10.1002/(sici)1521-1878(199805)20:5<382::aid-bies5>3.0.co;2-q
Padua, D., and Massagué, J. (2009). Roles of TGFbeta in metastasis. Cell Res. 19, 89–102. doi:10.1038/cr.2008.316
Panowski, S. H., Wolff, S., Aguilaniu, H., Durieux, J., and Dillin, A. (2007). PHA-4/Foxa mediates diet-restriction-induced longevity of C. elegans. Nature 447, 550–555. doi:10.1038/nature05837
Papadopoli, D., Boulay, K., Kazak, L., Pollak, M., Mallette, F. A., Topisirovic, I., et al. (2019). mTOR as a central regulator of lifespan and aging. F1000research 8, F1000 Faculty Rev-998. doi:10.12688/f1000research.17196.1
Paradis, S., Ailion, M., Toker, A., Thomas, J. H., and Ruvkun, G. (1999). A PDK1 homolog is necessary and sufficient to transduce AGE-1 PI3 kinase signals that regulate diapause in Caenorhabditis elegans. Gene Dev. 13, 1438–1452. doi:10.1101/gad.13.11.1438
Paradis, S., and Ruvkun, G. (1998). Caenorhabditis elegans Akt/PKB transduces insulin receptor-like signals from AGE-1 PI3 kinase to the DAF-16 transcription factor. Gene Dev. 12, 2488–2498. doi:10.1101/gad.12.16.2488
Park, D., Estevez, A., and Riddle, D. L. (2010). Antagonistic Smad transcription factors control the dauer/non-dauer switch in C. elegans. Development 137, 477–485. doi:10.1242/dev.043752
Park, D., Jones, K. L., Lee, H., Snutch, T. P., Taubert, S., and Riddle, D. L. (2012). Repression of a potassium channel by nuclear hormone receptor and TGF-β signaling modulates insulin signaling in Caenorhabditis elegans. Plos Genet. 8, e1002519. doi:10.1371/journal.pgen.1002519
Patterson, G. I., Koweek, A., Wong, A., Liu, Y., and Ruvkun, G. (1997). The DAF-3 Smad protein antagonizes TGF-β-related receptor signaling in the Caenorhabditis elegansdauer pathway. Gene Dev. 11, 2679–2690. doi:10.1101/gad.11.20.2679
Pence, B. D. (2022). Growth differentiation factor-15 in immunity and aging. Front. Aging 3, 837575. doi:10.3389/fragi.2022.837575
Portal-Celhay, C., Bradley, E. R., and Blaser, M. J. (2012). Control of intestinal bacterial proliferation in regulation of lifespan in Caenorhabditis elegans. Bmc Microbiol. 12, 49. doi:10.1186/1471-2180-12-49
Powell, J. D., Pollizzi, K. N., Heikamp, E. B., and Horton, M. R. (2012). Regulation of immune responses by mTOR. Annu. Rev. Immunol. 30, 39–68. doi:10.1146/annurev-immunol-020711-075024
Pradel, E., Zhang, Y., Pujol, N., Matsuyama, T., Bargmann, C. I., and Ewbank, J. J. (2007). Detection and avoidance of a natural product from the pathogenic bacterium Serratia marcescens by Caenorhabditis elegans. Proc. Natl. Acad. Sci. 104, 2295–2300. doi:10.1073/pnas.0610281104
Qi, W., Yan, Y., Pfeifer, D., Gromoff, E. D. v., Wang, Y., Maier, W., et al. (2017). C. elegans DAF-16/FOXO interacts with TGF-ß/BMP signaling to induce germline tumor formation via mTORC1 activation. Plos Genet. 13, e1006801. doi:10.1371/journal.pgen.1006801
Raberg, L., Graham, A. L., and Read, A. F. (2009). Decomposing health: tolerance and resistance to parasites in animals. Philos. Trans. R. Soc. B Biol. Sci. 364, 37–49. doi:10.1098/rstb.2008.0184
Raberg, L., Sim, D., and Read, A. F. (2007). Disentangling genetic variation for resistance and tolerance to infectious diseases in animals. Science 318, 812–814. doi:10.1126/science.1148526
Ramakrishnan, K., Ray, P., and Okkema, P. G. (2014). CEH-28 activates dbl-1 expression and TGF-β signaling in the C. elegans M4 neuron. Dev. Biol. 390, 149–159. doi:10.1016/j.ydbio.2014.03.015
Read, A. F., Graham, A. L., and Råberg, L. (2008). Animal defenses against infectious agents: is damage control more important than pathogen control? Plos Biol. 6, e4. doi:10.1371/journal.pbio.1000004
Reece-Hoyes, J. S., Shingles, J., Dupuy, D., Grove, C. A., Walhout, A. J., Vidal, M., et al. (2007). Insight into transcription factor gene duplication from Caenorhabditis elegans Promoterome-driven expression patterns. Bmc Genomics 8, 27. doi:10.1186/1471-2164-8-27
Ren, P., Lim, C.-S., Johnsen, R., Albert, P. S., Pilgrim, D., and Riddle, D. L. (1996). Control of C. elegans larval development by neuronal expression of a TGF-β homolog. Science 274, 1389–1391. doi:10.1126/science.274.5291.1389
Riddle, D. L., Swanson, M. M., and Albert, P. S. (1981). Interacting genes in nematode dauer larva formation. Nature 290, 668–671. doi:10.1038/290668a0
Robertis, E. M. D. (2008). Evo-devo: variations on ancestral themes. Cell 132, 185–195. doi:10.1016/j.cell.2008.01.003
Roberts, A. F., Gumienny, T. L., Gleason, R. J., Wang, H., and Padgett, R. W. (2010). Regulation of genes affecting body size and innate immunity by the DBL-1/BMP-like pathway in Caenorhabditis elegans. Bmc Dev. Biol. 10, 61. doi:10.1186/1471-213x-10-61
Sandhu, A., Badal, D., Sheokand, R., Tyagi, S., and Singh, V. (2021). Specific collagens maintain the cuticle permeability barrier in Caenorhabditis elegans. Genetics 217, iyaa047. doi:10.1093/genetics/iyaa047
Savage, C., Das, P., Finelli, A. L., Townsend, S. R., Sun, C. Y., Baird, S. E., et al. (1996). Caenorhabditis elegans genes sma-2, sma-3, and sma-4 define a conserved family of transforming growth factor beta pathway components. Proc. Natl. Acad. Sci. 93, 790–794. doi:10.1073/pnas.93.2.790
Savage-Dunn, C., Maduzia, L. L., Zimmerman, C. M., Roberts, A. F., Cohen, S., Tokarz, R., et al. (2003). Genetic screen for small body size mutants in C. elegans reveals many TGFbeta pathway components. Genesis 35, 239–247. doi:10.1002/gene.10184
Savage-Dunn, C., and Padgett, R. W. (2017). The TGF-β family in Caenorhabditis elegans. Csh Perspect. Biol. 9, a022178. doi:10.1101/cshperspect.a022178
Savage-Dunn, C., Tokarz, R., Wang, H., Cohen, S., Giannikas, C., and Padgett, R. W. (2000). SMA-3 smad has specific and critical functions in DBL-1/SMA-6 TGFbeta-related signaling. Dev. Biol. 223, 70–76. doi:10.1006/dbio.2000.9713
Schackwitz, W. S., Inoue, T., and Thomas, J. H. (1996). Chemosensory neurons function in parallel to mediate a pheromone response in C. elegans. Neuron 17, 719–728. doi:10.1016/s0896-6273(00)80203-2
Schafer, J. F. (1971). Tolerance to plant disease. Annu. Rev. Phytopathol. 9, 235–252. doi:10.1146/annurev.py.09.090171.001315
Schiffer, J. A., Servello, F. A., Heath, W. R., Amrit, F. R. G., Stumbur, S. V., Eder, M., et al. (2020). Caenorhabditis elegans processes sensory information to choose between freeloading and self-defense strategies. Elife 9, e56186. doi:10.7554/elife.56186
Schulenburg, H., Kurz, C. L., and Ewbank, J. J. (2004). Evolution of the innate immune system: the worm perspective. Immunol. Rev. 198, 36–58. doi:10.1111/j.0105-2896.2004.0125.x
Schultz, R. D., Bennett, E. E., Ellis, E. A., and Gumienny, T. L. (2014). Regulation of extracellular matrix organization by BMP signaling in Caenorhabditis elegans. Plos One 9, e101929. doi:10.1371/journal.pone.0101929
Sebastiani, P., Federico, A., Morris, M., Gurinovich, A., Tanaka, T., Chandler, K. B., et al. (2021). Protein signatures of centenarians and their offspring suggest centenarians age slower than other humans. Aging Cell 20, e13290. doi:10.1111/acel.13290
Sharika, R., Subbaiah, P., and Balamurugan, K. (2018). Studies on reproductive stress caused by candidate Gram positive and Gram negative bacteria using model organism, Caenorhabditis elegans. Gene 649, 113–126. doi:10.1016/j.gene.2018.01.088
Shaw, W. M., Luo, S., Landis, J., Ashraf, J., and Murphy, C. T. (2007). The C. elegans TGF-β dauer pathway regulates longevity via insulin signaling. Curr. Biol. 17, 1635–1645. doi:10.1016/j.cub.2007.08.058
Sheaffer, K. L., Updike, D. L., and Mango, S. E. (2008). The target of rapamycin pathway antagonizes pha-4/FoxA to control development and aging. Curr. Biol. 18, 1355–1364. doi:10.1016/j.cub.2008.07.097
Singh, J., and Aballay, A. (2019a). Intestinal infection regulates behavior and learning via neuroendocrine signaling. Elife 8, e50033. doi:10.7554/elife.50033
Singh, J., and Aballay, A. (2019b). Microbial colonization activates an immune fight-and-flight response via neuroendocrine signaling. Dev. Cell 49, 89–99. doi:10.1016/j.devcel.2019.02.001
Sinner, M. P., Masurat, F., Ewbank, J. J., Pujol, N., and Bringmann, H. (2021). Innate immunity promotes sleep through epidermal antimicrobial peptides. Curr. Biol. 31, 564–577.e12. doi:10.1016/j.cub.2020.10.076
Snow, M. I., and Larsen, P. L. (2000). Structure and expression of daf-12: a nuclear hormone receptor with three isoforms that are involved in development and aging in Caenorhabditis elegans. Biochimica Biophysica Acta (BBA) - Gene Struct Expr 1494, 104–116. doi:10.1016/s0167-4781(00)00224-4
So, S., Tokumaru, T., Miyahara, K., and Ohshima, Y. (2011). Control of lifespan by food bacteria, nutrient limitation and pathogenicity of food in C. elegans. Mech. Ageing Dev. 132, 210–212. doi:10.1016/j.mad.2011.02.005
Son, H. G., Altintas, O., Kim, E. J. E., Kwon, S., and Lee, S. V. (2019). Age-dependent changes and biomarkers of aging in Caenorhabditis elegans. Aging Cell 18, e12853. doi:10.1111/acel.12853
Songkiatisak, P., Rahman, S. M. T., Aqdas, M., and Sung, M.-H. (2022). NF-κB, a culprit of both inflamm-ageing and declining immunity? Immun. Ageing 19, 20. doi:10.1186/s12979-022-00277-w
Sonnhammer, E. L. L., and Durbin, R. (1997). Analysis of protein domain families inCaenorhabditis elegans. Genomics 46, 200–216. doi:10.1006/geno.1997.4989
Suzuki, Y., Yandell, M. D., Roy, P. J., Krishna, S., Savage-Dunn, C., Ross, R. M., et al. (1999). A BMP homolog acts as a dose-dependent regulator of body size and male tail patterning in Caenorhabditis elegans. Development 126, 241–250. doi:10.1242/dev.126.2.241
Tan, M.-W. (2001). Genetic and genomic dissection of host-pathogen interactions using a P. aeruginosa-C. elegans pathogenesis model. Pediatr. Pulmonol. 32, 96–97.
Templeman, N. M., Cota, V., Keyes, W., Kaletsky, R., and Murphy, C. T. (2020). CREB non-autonomously controls reproductive aging through hedgehog/patched signaling. Dev. Cell 54, 92–105. doi:10.1016/j.devcel.2020.05.023
Tenor, J. L., and Aballay, A. (2008). A conserved Toll-like receptor is required for Caenorhabditis elegans innate immunity. Embo Rep. 9, 103–109. doi:10.1038/sj.embor.7401104
Tewari, M., Hu, P. J., Ahn, J. S., Ayivi-Guedehoussou, N., Vidalain, P.-O., Li, S., et al. (2004). Systematic interactome mapping and genetic perturbation analysis of a C. elegans TGF-β signaling network. Mol. Cell 13, 469–482. doi:10.1016/s1097-2765(04)00033-4
Thomas, J. H., Birnby, D. A., and Vowels, J. J. (1993). Evidence for parallel processing of sensory information controlling dauer formation in Caenorhabditis elegans. Genetics 134, 1105–1117. doi:10.1093/genetics/134.4.1105
Urist, M. R., and Strates, B. S. (1971). Bone morphogenetic protein. J. Dent. Res. 50, 1392–1406. doi:10.1177/00220345710500060601
Vashlishan, A. B., Madison, J. M., Dybbs, M., Bai, J., Sieburth, D., Ch’ng, Q., et al. (2008). An RNAi screen identifies genes that regulate GABA synapses. Neuron 58, 346–361. doi:10.1016/j.neuron.2008.02.019
Wang, E. A., Rosen, V., Cordes, P., Hewick, R. M., Kriz, M. J., Luxenberg, D. P., et al. (1988). Purification and characterization of other distinct bone-inducing factors. Proc. Natl. Acad. Sci. 85, 9484–9488. doi:10.1073/pnas.85.24.9484
Wang, J., Tokarz, R., and Savage-Dunn, C. (2002). The expression of TGFbeta signal transducers in the hypodermis regulates body size in C. elegans. Development 129, 4989–4998. doi:10.1242/dev.129.21.4989
Wang, M. C., Oakley, H. D., Carr, C. E., Sowa, J. N., and Ruvkun, G. (2014). Gene pathways that delay Caenorhabditis elegans reproductive senescence. Plos Genet. 10, e1004752. doi:10.1371/journal.pgen.1004752
Wang, S., Liu, H., Qu, M., and Wang, D. (2021). Response of tyramine and glutamate related signals to nanoplastic exposure in Caenorhabditis elegans. Ecotoxicol. Environ. Saf. 217, 112239. doi:10.1016/j.ecoenv.2021.112239
Wong, Y.-F., Sheng, Q., Chung, J. W., Chan, J. K., and Chow, K. L. (2010). mab-31 and the TGF-β pathway act in the ray lineage to pattern C. elegansmale sensory rays. Bmc Dev. Biol. 10, 82. doi:10.1186/1471-213x-10-82
Wozney, J. M., Rosen, V., Celeste, A. J., Mitsock, L. M., Whitters, M. J., Kriz, R. W., et al. (1988). Novel regulators of bone formation: molecular clones and activities. Science 242, 1528–1534. doi:10.1126/science.3201241
Wu, M. Y., and Hill, C. S. (2009). TGF-Β superfamily signaling in embryonic development and homeostasis. Dev. Cell 16, 329–343. doi:10.1016/j.devcel.2009.02.012
Yang, Y., Wu, Q., and Wang, D. (2021). Neuronal Gα subunits required for the control of response to polystyrene nanoparticles in the range of μg/L in C. elegans. Ecotoxicol. Environ. Saf. 225, 112732. doi:10.1016/j.ecoenv.2021.112732
Yin, J., Madaan, U., Park, A., Aftab, N., and Savage-Dunn, C. (2015). Multiple cis elements and GATA factors regulate a cuticle collagen gene in Caenorhabditis elegans. Genesis 53, 278–284. doi:10.1002/dvg.22847
Yoshida, S., Morita, K., Mochii, M., and Ueno, N. (2001). Hypodermal expression of Caenorhabditis elegans TGF-β type I receptor SMA-6 is essential for the growth and maintenance of body length. Dev. Biol. 240, 32–45. doi:10.1006/dbio.2001.0443
Yu, Y., Mutlu, A. S., Liu, H., and Wang, M. C. (2017). High-throughput screens using photo-highlighting discover BMP signaling in mitochondrial lipid oxidation. Nat. Commun. 8, 865. doi:10.1038/s41467-017-00944-3
Zhang, F., Weckhorst, J. L., Assié, A., Hosea, C., Ayoub, C. A., Khodakova, A. S., et al. (2021a). Natural genetic variation drives microbiome selection in the Caenorhabditis elegans gut. Curr. Biol. 31, 2603–2618.e9. doi:10.1016/j.cub.2021.04.046
Zhang, S., Li, F., Zhou, T., Wang, G., and Li, Z. (2020). Caenorhabditis elegans as a useful model for studying aging mutations. Front. Endocrinol. 11, 554994. doi:10.3389/fendo.2020.554994
Zhang, X., Li, W., Feng, K., Xiao, J., Du, J., Cao, Y., et al. (2021b). Immunomodulatory effect of pentagalloyl glucose in LPS-stimulated RAW264.7 macrophages and PAO1-induced Caenorhabditis elegans. Exp. Gerontol. 150, 111388. doi:10.1016/j.exger.2021.111388
Zhang, X., and Zhang, Y. (2012). DBL-1, a TGF-β, is essential for Caenorhabditis elegans aversive olfactory learning. Proc. Natl. Acad. Sci. 109, 17081–17086. doi:10.1073/pnas.1205982109
Zhang, Y., Lu, H., and Bargmann, C. I. (2005). Pathogenic bacteria induce aversive olfactory learning in Caenorhabditis elegans. Nature 438, 179–184. doi:10.1038/nature04216
Zhang, Y., Qi, L., and Zhang, H. (2019). TGFβ-like DAF-7 acts as a systemic signal for autophagy regulation in C. elegans. J. Cell Biol. 218, 3998–4006. doi:10.1083/jcb.201907196
Zheng, S., Qu, Z., Zanetti, M., Lam, B., and Chin-Sang, I. (2018). C. elegans PTEN and AMPK block neuroblast divisions by inhibiting a BMP-insulin-PP2A-MAPK pathway. Development 145, dev166876. doi:10.1242/dev.166876
Zhou, M., Liu, X., Yu, H., and Gong, J. (2021). Lactobacillus regulates Caenorhabditis elegans cell signaling to combat Salmonella infection. Front. Immunol. 12, 653205. doi:10.3389/fimmu.2021.653205
Keywords: aging, innate immunity, TGF-β, BMP, C. elegans
Citation: Yamamoto KK and Savage-Dunn C (2023) TGF-β pathways in aging and immunity: lessons from Caenorhabditis elegans. Front. Genet. 14:1220068. doi: 10.3389/fgene.2023.1220068
Received: 10 May 2023; Accepted: 23 August 2023;
Published: 05 September 2023.
Edited by:
Zhiyong Shao, Fudan University, ChinaReviewed by:
Tina L. Gumienny, Texas Woman’s University, United StatesYunhan Yang, Southeast University, China
Copyright © 2023 Yamamoto and Savage-Dunn. This is an open-access article distributed under the terms of the Creative Commons Attribution License (CC BY). The use, distribution or reproduction in other forums is permitted, provided the original author(s) and the copyright owner(s) are credited and that the original publication in this journal is cited, in accordance with accepted academic practice. No use, distribution or reproduction is permitted which does not comply with these terms.
*Correspondence: Cathy Savage-Dunn, Y2F0aHkuc2F2YWdlZHVubkBxYy5jdW55LmVkdQ==