- 1School of Wine and Horticulture, Ningxia University, Yinchuan, China
- 2Key Laboratory of Modern Molecular Breeding for Dominant and Special Crops in Ningxia, Yinchuan, China
- 3Ningxia Modern Facility Horticulture Engineering Technology Research Center, Yinchuan, Ningxia, China
- 4Ningxia Facility Horticulture Technology Innovation Center, Ningxia University, Yinchuan, China
Watermelon (Citrullus lanatus) as a crop with important economic value, is widely cultivated around the world. The heat shock protein 70 (HSP70) family in plant is indispensable under stress conditions. However, no comprehensive analysis of watermelon HSP70 family is reported to date. In this study, 12 ClHSP70 genes were identified from watermelon, which were unevenly located in 7 out of 11 chromosomes and divided into three subfamilies. ClHSP70 proteins were predicted to be localized primarily in cytoplasm, chloroplast, and endoplasmic reticulum. Two pairs of segmental repeats and 1 pair of tandem repeats existed in ClHSP70 genes, and ClHSP70s underwent strong purification selection. There were many abscisic acid (ABA) and abiotic stress response elements in ClHSP70 promoters. Additionally, the transcriptional levels of ClHSP70s in roots, stems, true leaves, and cotyledons were also analyzed. Some of ClHSP70 genes were also strongly induced by ABA. Furthermore, ClHSP70s also had different degrees of response to drought and cold stress. The above data indicate that ClHSP70s may be participated in growth and development, signal transduction and abiotic stress response, laying a foundation for further analysis of the function of ClHSP70s in biological processes.
1 Introduction
With the acceleration of industrialization and global warming, plants are suffering from various abiotic stresses, including drought, salinity, heat, and low temperature (Zhang et al., 2022). Plants have developed a variety of regulatory mechanisms to cope with stresses during the long course of evolution, one of which involves heat shock proteins (HSPs). HSPs protect plant cells from stresses by folding and translocating new proteins and refolding denatured proteins (Dong et al., 2001; Timperio et al., 2008; Mohamed, 2011). Based on the molecular masses, HSPs can be divided into five subfamilies, including small HSP (sHSP), chaperonin (HSP60), 70-kDa-heat shock protein (HSP70), HSP90 and HSP100 subfamily (Wang et al., 2004). Among them, plant HSP70s are highly conserved and widespread, which are crucial housekeeping proteins, suggesting that HSP70s play essential roles under normal and stress conditions (Tanya and Elizabeth, 2007; Saeed et al., 2019).
Since the first discovery and identification in the early 1960s (Ritossa, 1996), great progress has been made in the study of HSP70s. HSP70 proteins consist of three conserved domains, an N-terminal ATPase domain (NBD, ∼44 kDa), a substrate binding domain (SBD, ∼18 kDa), and a C-terminal substrate binding domain (∼10 kDa) (Dragovic et al., 2006). According to different cell localization, plant HSP70s can be divided into four functional subfamilies: cytoplasm, endoplasmic reticulum (ER), plastid, and mitochondria (Guy and Li, 1998; Cho and Choi, 2009). Intracellular HSP70s were induced by heat stress to transfer to plasma membrane and liquid membrane, formed peripheral proteins outside the membrane, inhibited membrane protein degeneration, and ultimately improved biofilm stability and heat resistance (Jiang et al., 2021). Ectopic expression of cytoplasmic CaHSP70-2 from pepper improved heat tolerance by altering the expression of stress-related genes in transgenic Arabidopsis (Guo et al., 2016). Arabidopsis thaliana also showed diurnal variation in heat tolerance, with the highest tolerance in the daytime, which was positively correlated with the expression levels of AtHSP70s (Dickinson et al., 2018). Besides, the expression of HSP70s was associated with drought, virus, heavy metal, and cold stress (Queitsch et al., 2000; Fei et al., 2015; Zhang et al., 2015; Osman et al., 2019; Lubkowska et al., 2021). For instance, overexpression of Nicotiana tabacum NtHSP70-1 not only improved the plants’ thermoprotective activity but also enhanced drought tolerance (Cho and Hong, 2006). Cytoplasmic HSP70s could enhance the resistance of Nicotiana benthamiana to diverse viral infections (Chen et al., 2008). Aquatic Moss HSP70s might be related to detoxification of heavy metals, such as lead, cadmium, copper, and zinc (Esposito et al., 2012). However, as this family is multigenic, there may be functional redundancy among plant HSP70 members.
Watermelon (Citrullus lanatus) as an important horticultural crop with high economic and nutritional value, is widely cultivated in the world. It not only provides rich carbohydrates and dietary fiber, but also provides a variety of vitamins and compounds that are beneficial to human health (Makaepea et al., 2019; Mashilo et al., 2022). However, watermelon is sensitive to adverse conditions, and HSP70 proteins may play an important role in this response process. Members of the HSP70 family have been identified in different species, such as Arabidopsis (Lin et al., 2001), tobacco (Song et al., 2019), herbaceous peony (Zhao et al., 2019), sorghum (Nagaraju et al., 2020) and rice (Jung et al., 2013), but not in watermelon. The completion of genome sequencing of watermelon is helpful to identify its HSP70 family (Guo et al., 2013).
In this study, a total of 12 ClHSP70 genes were identified in watermelon. The molecular characteristics, gene structure, cis-acting elements, SSRs, GO enrichment analysis, and protein interactions of ClHSP70 members were further analyzed, as well as chromosome mapping, phylogenetic tree construction, interspecific collinearity analysis and binding sites targeted by miRNAs. In addition, the tissue specificity of ClHSP70s was analyzed by qRT-PCR, and their response to ABA, drought and cold stress was also discussed. These results help us to better understand the function of ClHSP70s and provide key clues to reveal the response mechanism of watermelon to ABA and abiotic stresses.
2 Materials and methods
2.1 Plant materials and treatments
The watermelon variety ‘Xi Nong 8’ was used in this study. Seedlings were cultured in a light incubator at day/night 28/22°C (16/8 h) for 30 days, and Hoagland nutrient solution was used for hydroponics. For tissue expression analysis, the roots, stems, true leaves and cotyledons of seedlings were sampled. The seedlings placed in the Hoagland nutrient solution containing 10% polyethylene glycol 6000 (PEG6000) were used for drought treatment, the seedlings under 4°C conditions were used for cold treatment, and the seedlings spraying 100 μM ABA (dissolved in 0.004% ethanol) for hormone treatment. The control seedlings for ABA treatment were sprayed with 0.004% ethanol, and the control for abiotic stresses were the hydroponic seedlings under normal conditions. The leaves were collected after treatment for 0, 0.5 (cold and drought stress), 1, 3, 6, 12, and 24 h. Three biological replicates were performed for each treatment, and samples for each replicate were collected from five seedlings.
2.2 Identification of ClHSP70 genes from watermelon
The identification process of ClHSP70 family in watermelon was shown in Supplementary Figure S1. Briefly, the watermelon genome sequence was downloaded from EnsemblPlants database (EB, http://plants.ensembl.org/info/data/index.html). The Hidden Markov Model (HMM) profile of HSP70 domain (PF00012) was downloaded from Protein family (Pfam) database (Finn et al., 2014) and used as a BLAST query against the watermelon genome database (E-value<1 × 10−5). Meanwhile, HMMER-3.0 program was employed to identify watermelon ClHSP70s (Finn et al., 2011). Subsequently, SMART (http://smart.embl-heidelberg.de/) and Pfam (http://pfam.xfam.org/search) were used to identify the HSP70 domains in all putative ClHSP70s to verify whether they belonged to HSP70 family.
The chromosome distributions, amino acid (AA), genome sequences and gene structures of watermelon ClHSP70 members were obtained from EB database. In addition, EXPASY Proteomics Server (https://web.expasy.org/protparam/) was used to analyze the AAs, molecular mass, and theoretical isoelectric points (Lopes-Caiter et al., 2013). The cell localization prediction of ClHSP70s was performed using Wolf PSORT program (http://wolfpsort.org/).
2.3 Phylogenetic analysis
The protein sequences of HSP70 family from various species, including A. thaliana, Solanum lycopersicum, Solanum tuberosum, Csativus sativus, Vitis vinifera, Zea mays, Oryza sativa and C. lanatus, were multiply aligned using the ClustalW program of MEGA11 (Tamura et al., 2021). The gene IDs of HSP70 family from different plants were shown in Supplementary Table S1. The phylogenetic tree was constructed based on the maximum likelihood (ML) method with 1000 bootstrap replications using TBtools (Chen et al., 2020).
2.4 Prediction of conserved motifs and three-dimensional structures and gene structure analysis
Conserved motifs in ClHSP70 sequences were searched by MEME suite (https://meme-suite.org/meme/) (Bailey et al., 2009), with the following parameters: any number of repetitions; maximum number: 10; optimum widths: 6-200 AAs. SWISS-Model (https://swissmodel.expasy.org/) was used to model three-dimensional (3D) structures of ClHSP70s based on homology modeling (Waterhouse et al., 2018). Global model quality estimation (GMQE) was used to evaluate the model quality (Wang et al., 2023). To illustrate the exon/intron structures of all ClHSP70s, the cDNA sequences were aligned with their genomic sequences via TBtools.
2.5 Chromosomal localization, synteny analysis and gene duplication
The chromosomal localizations of ClHSP70s and the genome files of watermelon, rice and Arabidopsis were obtained from EB database. The chromosome distribution and gene duplication of ClHSP70 genes were analyzed and mapped using TBtools software, which was also used to visualize the collinearity map between different species. The duplication of ClHSP70s was defined according to the following criteria: a) the sequence alignment length covered more than 70% of the longer gene sequence; b) more than 70% similarity between aligned gene regions (Gu et al., 2002; Yang et al., 2008). Tandem duplicated genes were defined as two genes separated by no more than five genes in a 100 kb chromosomal region (Wang et al., 2010). Paralogous genes located on duplicated chromosomal blocks were considered as segmental duplication (Wei et al., 2007).
The ratios of nonsynonymous (Ka) and synonymous (Ks) (Ka/Ks) were calculated for the results of ClHSP70 duplications, and Ks was converted into the differentiation time (T) with the formula: T = Ks/(2 × 6.1×10−9) ×10−6 million years ago (Mya) (Lynch and Conery, 2000).
2.6 Analysis of cis-regulatory elements and protein interaction
The promoter sequences of ClHSP70s (upstream sequence 2,000 bp) were identified by TBtools. The cis-elements were analyzed by PlantCARE online (https://bioinformatics.psb.ugent.be/webtools/plantcare/html/). The interaction analysis of ClHSP70s was performed using the STRING online (http://cn.string-db.org/), and Arabidopsis was used as a model plant for the query. The results were exported and plotted using Cystoscope software.
2.7 GO enrichment analysis and SSR mining of ClHSP70s
Gene Ontology (GO) enrichment analysis of watermelon ClHSP70s was conducted using AmiGO 2 (http://amigo.geneontology.org/amigo/landing) with the corrected p-value < 0.05. The GO analysis contains cellular component (CC), biological process (BP), and molecular function (MF).
The sequences of 12 ClHSP70s were downloaded from the watermelon genome, and the bases were set as 2,000 bp before the start codon and 2,000 bp after the stop codon. These sequences were subjected to simple sequence repeat (SSR) mining by MISA (https://webblast.ipk-gatersleben.de/misa/index.php?action=1) (Beier et al., 2017). The minimum standard lengths for dinucleotide, trinucleotide and tetranucleotide repeats were 6, 5 and 5 repeats, respectively (Fu et al., 2023).
2.8 Response of ClHSP70s to cold and drought stress based on RNA-seq data
Fragments Per Kilobase Per Million (FPKM) values for each ClHSP70s in leaves under cold stress (4°C for 36 h, PRJNA328189) and drought stress conditions (4 days and 8 days, PRJNA454040) were retrieved from CuGenDB database (http://cucurbitgenomics.org/r naseq/home). Watermelon cultivar Y134 was used for cold treatment, while other two varieties Y34 and M20 were used for drought stress treatment. The data for each ClHSP70 were normalized by log2 (FPKM) (Supplementary Table S2) and shown as Heatmap using TBtools.
2.9 RNA extraction and RT-qPCR
Total RNA was extracted from samples by Total RNA kit (TIANGEN, Beijing, China), and reverse-transcribed by One-Step gDNA Removal and cDNA Synthesis Super Mix kit (TransGen, Beijing, China) according to the manufacturer’s instructions. The final cDNA was used as the template for subsequent quantitative real-time PCR (qRT-PCR).
Specific primers for detection of ClHSP70 gene expression (Supplementary Table S3) were designed using Primer 5 software. The yellow-leaf-specific proein8 (ClYLS8) and β-actin (ClACT) genes from watermelon were used as the reference genes (Kong et al., 2014). qRT-PCR was conducted using ChamQ universal SYBR qPCR Master Mix (Vazyme, Nanjing, China) with a 20 μL system on the qTOWER³ Series-Real-Time Thermal Cyclers (Analytik Jena, Thüringen, Germany). The experimental procedure of qRT-PCR carried out in accordance with the instruction manual of SYBR qPCR Master Mix, including an initial activation of 95°C for 30 s, then 40 cycles of 95°C for 10 s, 60°C for 30 s. The 2−△△CT method was used to calculate the relative transcript levels of ClHSP70s (Schmittgen and Livek, 2008).
2.10 Prediction of binding sites of ClHSP70s targeted by miRNA
The microRNA (miRNA) sequences were downloaded from PmiREN2.0 (https://www.pmiren.com/) (Guo et al., 2022). The prediction of binding sites of ClHSP70s targeted by miRNA was performed using psRNATarget (https://www.zhaolab.org/psRNATarget/) (Dai et al., 2018), and the parameters were set to default values. The track predicted ClHSP70 gene binding sites were listed in Supplementary Table S4.
3 Results
3.1 Identification and characterization of watermelon HSP70 family members
Using HMMER search and BLASTP alignment, 12 HSP70 family members were finally identified (Supplementary Table S5). They were named ClHSP70-1 (62.01 kDa) to ClHSP70-12 (102.80 kDa) based on their molecular weight. The physicochemical properties of ClHSP70s were analyzed by EXPASY online software. The ClHSP70 genes were unevenly distributed on 7 of 11 chromosomes. The minimum length of the encoding sequence was 1,716 bp and the maximum length was 2,766 bp, the number of AAs corresponding to the encoding proteins was 571 and 921, respectively (Table 1). The theoretical isoelectric points were between 4.9 (ClHSP70-6) and 5.78 (ClHSP70-7), indicating that ClHSP70 proteins were acidic. Only the total average hydrophilicity (GRAVY) of ClHSP70-1 was greater than 0, indicating that most ClHSP70s were hydrophilic proteins. Six members (ClHSP70-1, -2, -3, -4, -5, and -11) were mainly located in cytoplasm, one (ClHSP70-10) in nucleus, two (ClHSP70-6 and -9) in chloroplasts, one (ClHSP70-7) in mitochondria, and two (ClHSP70-8 and -12) in endoplasmic reticulum.
In addition, the intron number of all members was analyzed to gain insight into the structural evolution of ClHSP70s (Figure 1). The intron number of ClHSP70s ranged from 0 to 14 (Table 1). The intron number in Group Ⅱ and Ⅲ was higher than other groups, but ClHSP70-1 from Group Ⅲ had no introns. In general, the exon-intron distribution characteristics of ClHSP70s in the same group were similar, while the difference in intron number between different groups was large. Motif analysis showed that the types and amounts of the motifs in Group Ⅰ and Ⅱ were similar, while ClHSP70-10, -11, and -12 in Group Ⅲ did not contain Motif 2, and ClHSP70-11 and -12 did not contain Motif 4. Unlike other ClHSP70s, ClHSP70-12 did not yet contain Motif 6 (Figure 1). The 3D protein structures of ClHSP70s were predicted by SWISS-Model (Supplementary Figure S2) and their GMQE values were higher than 0.8. The number of β folds in the protein structure of each ClHSP70 member was higher than that of α helices, indicating that β folds were the main components of ClHSP70s. The protein structure of ClHSP70-1 was quite different from that of other members, mainly attributing to the fact that the number of α helices contained in ClHSP70-1 (13) was much lower than that of other members (≥18). This structural difference was consistent with the results of the cluster analysis of ClHSP70-1 (Figure 1A). The remaining protein structures were highly similar, such as ClHSP70-2, -3 and -4. They had the same number of α helices, while ClHSP70-4 had only one more β fold than the other two proteins. These results suggest that ClHSP70s are evolutionarily conserved and may have functional redundancy, while structural differences indicate functional specificity among different members.
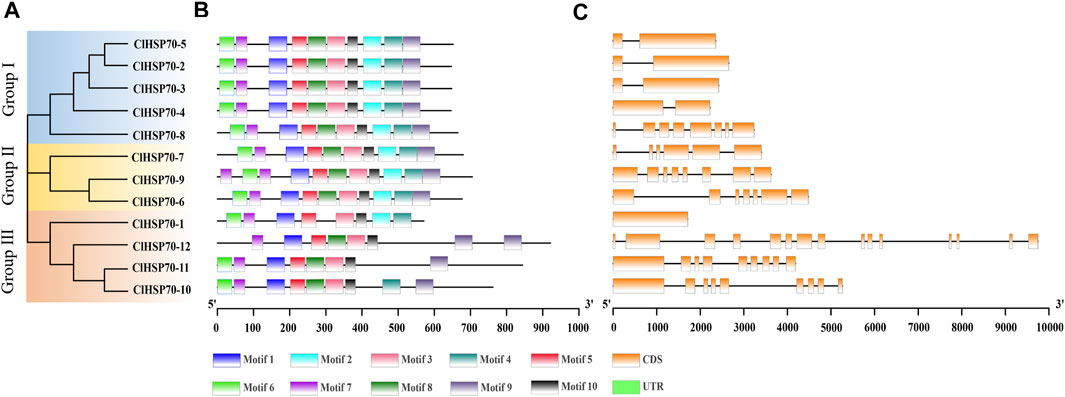
FIGURE 1. Phylogenetic relationships, gene structures and conserved motifs of all ClHSP70s in watermelon. (A) The unrooted phylogenetic tree was generated based on the amino acid sequences by the neighbor-joining (NJ) method using MEGA11. Bootstrap supports from 1000 replicates were indicated at each branch. (B) Motif analysis was performed using MEME 4.0 software. Different colored boxes represented different motifs in the corresponding position of each ClHSP70 protein. The sequences of different motifs were shown in Supplementary Table S6. (C) Gene structures were analyzed using TBtools. Yellow boxes indicated exons, and lines indicated introns.
3.2 Chromosomal locations and gene duplication of ClHSP70s
Based on the annotation file of the watermelon genome, TBtools was used to visualize the distribution of ClHSP70s on chromosomes (Chrs). Twelve watermelon ClHSP70 genes were randomly distributed on 7 Chrs, located on Chr1 (1), Chr4 (1), Chr5 (2), Chr6 (1), Chr9 (4), Chr10 (1) and Chr11 (2), respectively (Figure 2). Tandem duplicated and segmental duplication have certain effects on the formation of gene families during evolution (Cannon et al., 2004). Three pairs of duplication genes in ClHSP70 family were identified, of which ClHSP70-3/-5 was tandem duplicated, ClHSP70-2/-3 and ClHSP70-2/-5 were segmental duplication (Figure 2). To understand whether ClHSP70 genes were affected by natural selection during evolution, the Ka/Ks values of ClHSP70-2/-3, ClHSP70-2/-5 and ClHSP70-3/-5 were calculated. Their Ka/Ks ratios were all less than 1, suggesting that ClHSP70s underwent strong purifying selection during evolution (Li et al., 2022), and duplicated events occurred at 123.4 (ClHSP70-2/-5), 163.6 (ClHSP70-3/-5) and 175.5 (ClHSP70-2/-3) Mya, respectively (Table 2).
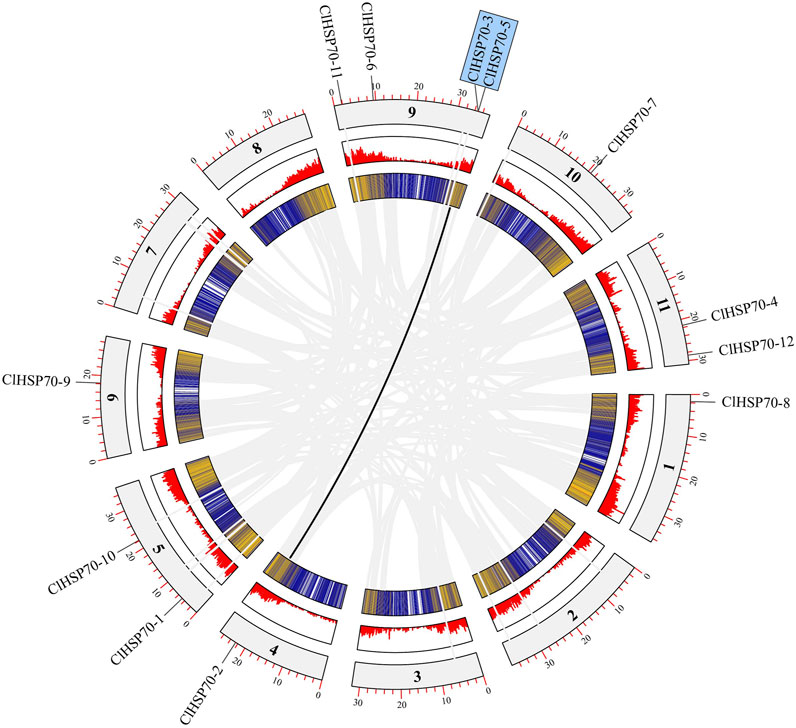
FIGURE 2. The collinearity analysis of ClHSP70s. The outermost circle represented the chromosome, the innermost circle was the gene density in the chromosome, and the middle circle was the gene density histogram display. Grey lines represented all collinearity genes in watermelon, and black lines represented ClHSP70 collinearity genes.
3.3 Phylogenetic analysis of ClHSP70 proteins
A ML phylogenetic tree was constructed using the protein sequences of 157 HSP70s from multiple plants, including A. thaliana (18), S. lycopersicum (19), S. tuberosum (21), C. sativus (13), V. vinifera (15), Z. mays (28), O. sativa (31), and C. lanatus (12) (Figure 3; Supplementary Table S1). Based on their evolutionary relationship, HSP70 proteins were classified into five subfamilies, including cytosol (C), ER, mitochondria (M), chloroplast (Chl) and nucleus (N) subfamily. Nearly half of HSP70s belonged to the cytoplasmic subfamily, which was consistent with the subcellular localization of watermelon ClHSP70s, suggesting that plant HSP70s function mainly in the cytoplasm. HSP70 members from different species of the same subfamilies were more closely related than members from different subfamilies. For example, watermelon ClHSP70-2 and cucumber Cucsa.066080.1 in C subfamily, rice Os06g46600.1 and maize GRMZM2G056766-T01 in N subfamily. Each subfamily had different HSP70 branches formed by monocots and dicots, which also indicated that HSP70 family was formed before the differentiation of monocots and dicots. Twelve pairs of orthologous genes were identified between watermelon and cucumber, suggesting that the ancestral genes of this family were formed before these two species. Additionally, there were several pairs of paralogous genes, indicating that ClHSP70s had undergone multiple replications after watermelon speciation.
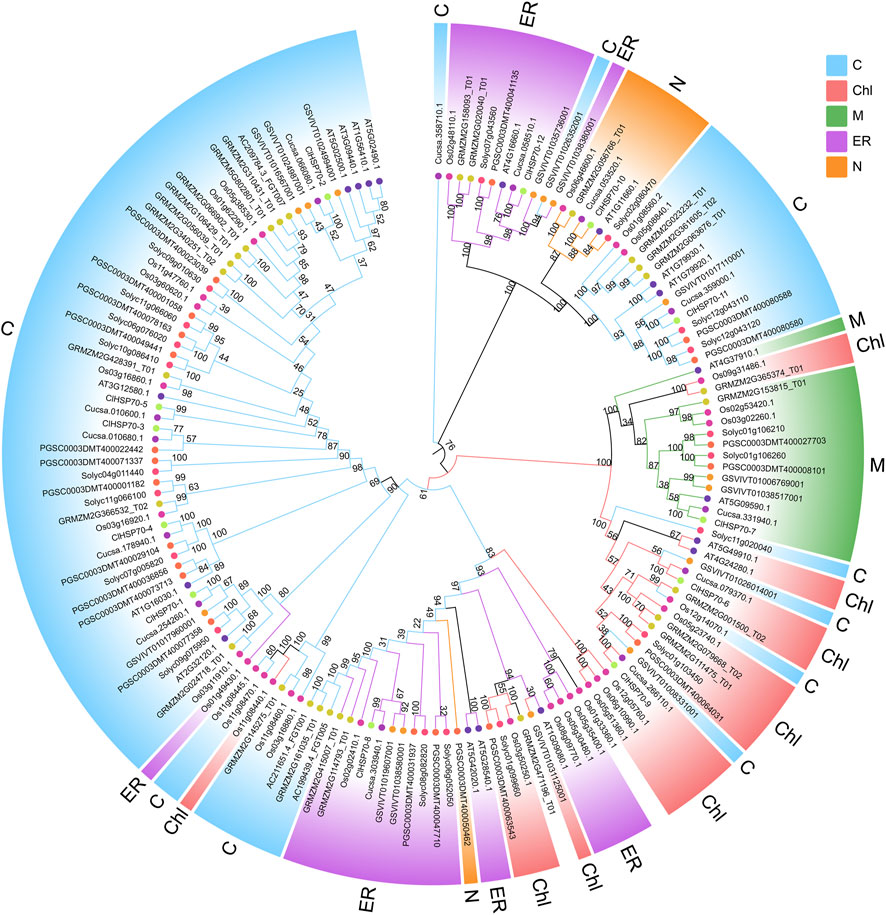
FIGURE 3. Phylogenetic relationship of ClHSP70 proteins. Phylogenetic trees were constructed using the ML method with bootstrap tests by MEGA11. The diverse HSP70 subfamilies were indicated with different color arcs. The different colored symbols at the branch tips represented different species. C, cytosol; ER, endoplasmic reticulum; Chl, chloroplast; N, nucleus; M, mitochondria. HSP70 proteins that did not belong to the above subfamilies were not colored.
3.4 Interspecific collinearity analysis and cis-acting element prediction of ClHSP70s
To explore the underlying evolution of ClHSP70 family, the collinearity of watermelon with model plants Arabidopsis and monocotyledon rice was analyzed (Figure 4A). Three pairs of homologous gene pairs between watermelon and Arabidopsis (ClHSP70-2/AtHSP70-2, ClHSP70-2/AtHSP70-3, and ClHSP70-8/AtHSP70-12) were identified (Lin et al., 2001), which was much less than 8 pairs between watermelon and rice (ClHSP70-2/OscHSP70-1, ClHSP70-2/OscHSP70-5, ClHSP70-2/OscHSP70-6, ClHSP70-3/OscHSP70-1, ClHSP70-3/OscHSP70-2, ClHSP70-3/OscHSP70-5, ClHSP70-11/OsHSP110-1, and ClHSP70-11/OsHSP110-4) (Jung et al., 2013).
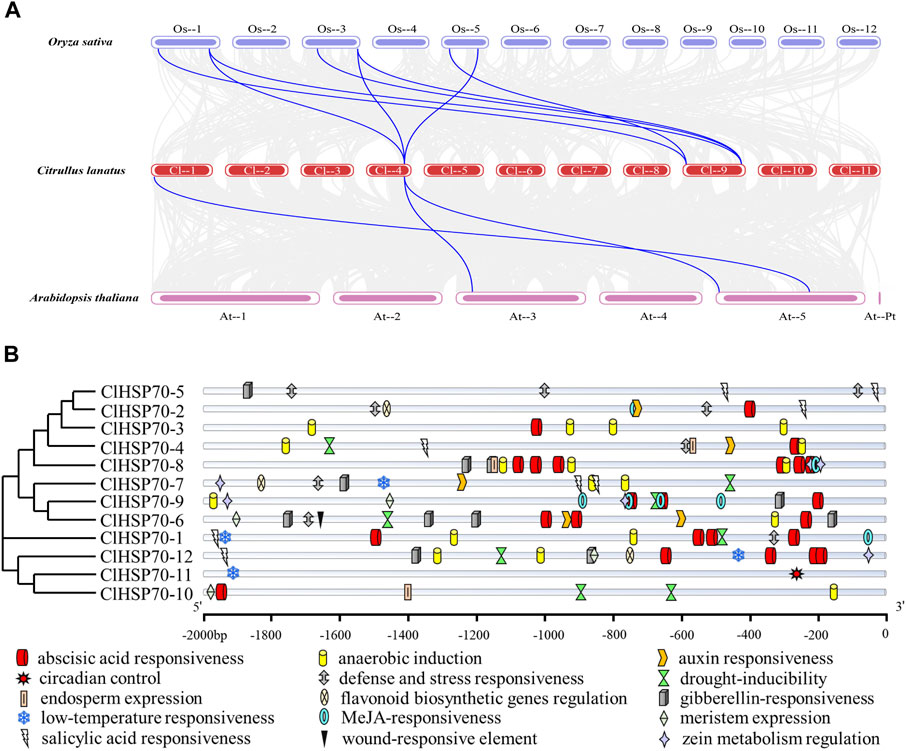
FIGURE 4. Collinearity and promoter sequence analysis of ClHSP70 genes. (A) Collinearity analysis of watermelon ClHSP70 genes with Oryza sativa and Arabidopsis thaliana. The gray lines denoted collinearity between all genes and the blue lines denoted collinearity between the two ClHSP70 family members. (B) Types and numbers of cis-acting elements in promoters of watermelon in the ClHSP70 gene family.
To investigate the expression characteristics and potential functions of ClHSP70 family, analysis of the gene promoter sequences (2,000 bp upstream of the start codon) was performed (Supplementary Table S7). The distributions of cis-acting elements of ClHSP70 promoters were visualized, and 15 functional elements were labeled (Figure 4B). The identified cis-elements were divided into hormone response group, stress response group, and growth and development group. Among them, the hormone response group contained the most elements, up to 66, mainly including ABA (24), methyl jasmonic (15), gibberellin (11), and salicylic (8). The stress response group also had many cis-elements, such as anaerobic induction (19), defense and stress (9), drought stress (8), and low-temperature stress (4). However, only 14 elements in the growth and development group. In summary, ClHSP70s may present different expression patterns during developmental and environmental stress response.
3.5 Protein interaction network of ClHSP70 family
To provide more clues about the function of ClHSP70s, STRING online software was used to predict the protein interaction network of this family members based on the interolog of the Arabidopsis interactome. ClHSP70-8 and -12 both interacted with other proteins, while ClHSP70-2 and -5 did not interact with each other, and neither of them interacted with other three proteins (ClHSP70-1, -3, and -4). Additionally, ClHSP70-7 did not interact with ClHSP70-6 or -9 (Figure 5). These data provide a reference for in-depth study of the biological function of ClHSP70s.
3.6 GO enrichment and SSR analysis of ClHSP70s
In order to deeply understand the functions of this family, GO annotation and enrichment analysis of ClHSP70s were performed (Supplementary Figure S3). In MF, all ClHSP70s were assigned in binding, such as unfold protein, small molecule, anion and ATP binding. However, only 3 ClHSP70s (25%) were assigned in unfold protein binding. In CC, cellular component was enriched in all 12 genes. Nevertheless, only three members (25%) were assigned in protein folding of BP. These data suggest that ClHSP70s may function as molecular chaperones in cells.
A total of 66 SSRs were discovered from 12 ClHSP70 sequences (Supplementary Table S8). Only the ClHSP70-9 sequence had one SSR, and the remaining members all had multiple SSRs. In addition, 3 SSRs present in compound formation were detected in ClHSP70-6, -11 and -12 sequences. The 2000 bp promoter regions contained 23 SSRs, the gene regions contained 19 SSRs, the post-2000 bp sequences contained 24 SSRs, and ClHSP70-6 contained the most SSR sites (14) (Supplementary Table S8). Among all the SSR types, dinucleotide repeats were the most abundant (53.33%), followed by trinucleotide repeats (40.00%), while there was only one SSR (6.67%) with a tetranucleotide repeat. SSRs with five sequence repetitions (26.67%) were the most abundant (Supplementary Table S9).
3.7 Tissue-specific expression analysis of watermelon ClHSP70s
To analyze the tissue expression specificity of ClHSP70s, the relative levels of all members in 4 tissues (roots, stems, true leaves and cotyledons) of 30-day-old seedlings were determined by qRT-PCR (Figure 6). The expression levels of ClHSP70-1 and -4 were the highest in stems and the lowest in true leaves. The levels of ClHSP70-2, -3, -11 and -12 in stems were similar to those in roots, but significantly increased in cotyledons and true leaves. The abundance of other six genes (ClHSP70-5, -6, -7, -8, -9, and -10) in stems was the lowest. The expression levels of ClHSP70-5, -8 and -9 in cotyledons and true leaves were significantly higher than those in other tissues, while ClHSP70-6 and -10 were preferentially expressed in cotyledons. In general, ClHSP70s may function in the biological processes of different tissues in watermelon.
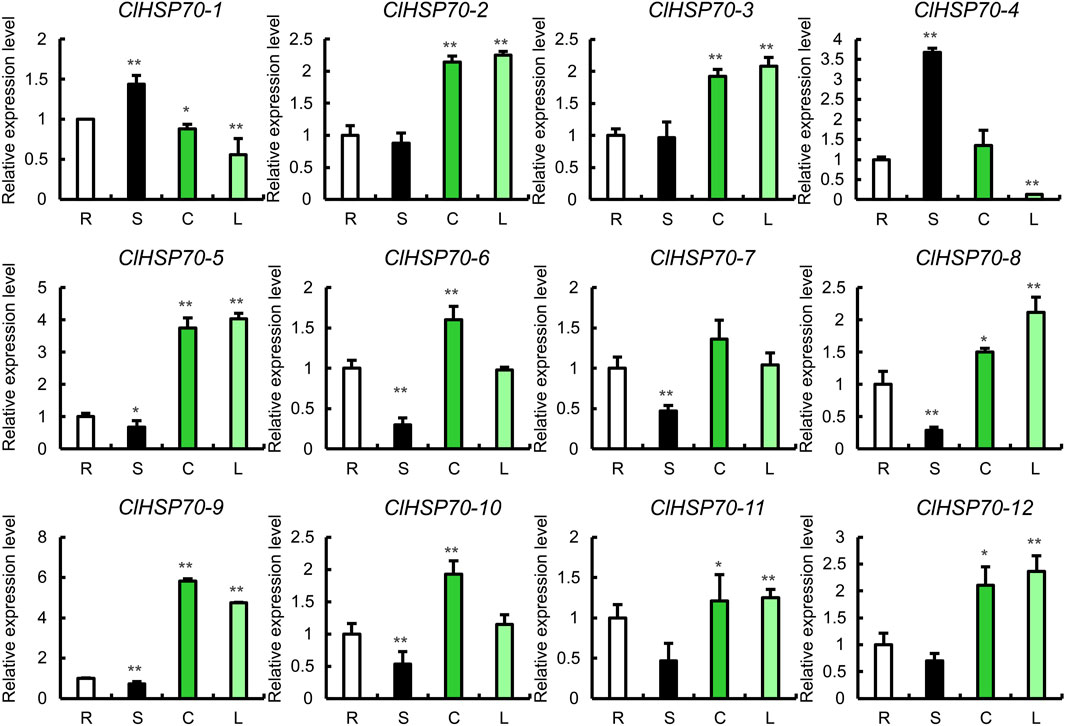
FIGURE 6. The expression of the ClHSP70 family genes in different tissues. R, S, C, and L represented roots, stems, true leaves, and cotyledons, respectively. qRT-PCR data were normalized using the watermelon ClACT gene. The relative expression levels of genes in roots were set to 1. All statistical tests were two-tailed Student’s t-tests at the 0.05 and 0.01 levels, and the p-values were given by the symbols * (p < 0.05) and ** (p < 0.01), respectively.
3.8 Response of ClHSP70s to drought and cold stress
HSP70s as molecular chaperones are widely involved in plant heat adaptation, and their roles in other abiotic stresses (such as drought and cold stress) have also been demonstrated in a variety of plants (Wang et al., 2004). However, the response of watermelon ClHSP70s to drought and cold stress remains unknown. To understand the response of ClHSP70 to cold and drought stress in detail, the effects of cold and drought stress on ClHSP70 expression were analyzed based on the RNA-seq data from the CuGenDB database (Figure 7). Under cold conditions, all ClHSP70s except ClHSP70-11 responded to low temperature (4°C for 36 h) to varying degrees. In particular, the levels of ClHSP70-1 and -4 were significantly downregulated (Figure 7A). Three genes (ClHSP70-2, -5, and -9) were not sensitive to drought stress, however, the levels of other nine members were observably regulated by drought treatment (4 or 8 days) (Figure 7B).
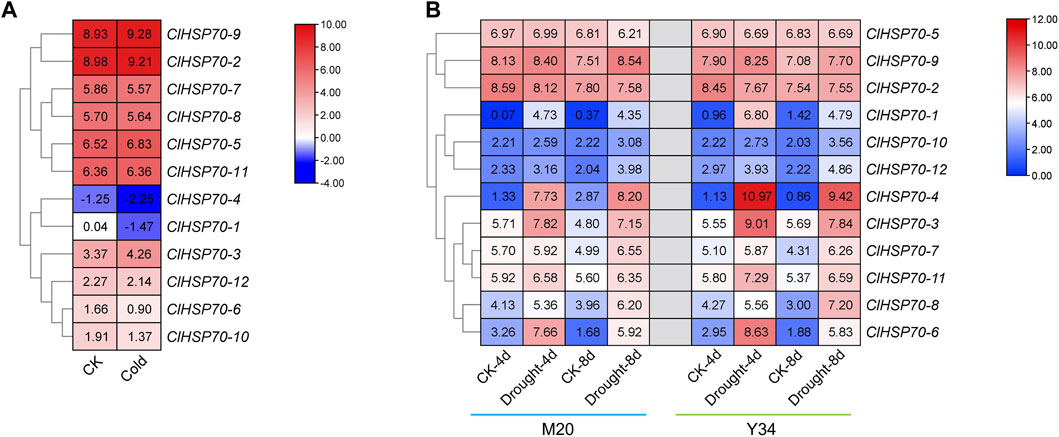
FIGURE 7. The expression patterns of the ClHSP70s under cold stress and drought stress in watermelon. (A) The expression of ClHSP70s in response to cold stress in Y134. (B) The expression of ClHSP70s in response to drought stress in M20 and Y34. CK, control; Cold, the seedlings were treated with 4°C for 36 h; Drought, seedlings imposed to drought stress for 4 days and 8 days. The expression of ClHSP70s were shown on a heatmap using log2FPKM value, and −4.00 to 10.00 (A) and 0.00 to 12.00 (B) were artificially set with the color scale limits according to the normalized value.
Moreover, the dynamic expression patterns of all members in 30-day-old seedlings were analyzed under simulated drought (10% PEG6000) and cold stress (4°C) conditions (treatment for 0, 0.5, 1, 3, 6, 12, and 24 h). As shown in Figure 8A, the expression levels of all ClHSP70s except ClHSP70-1 and -4 were higher after 12 h and/or 24 h of simulated drought treatment. The expression level of ClHSP70-1 was the highest after drought treatment for 6 h, and the level of ClHSP70-4 reached the peak after treatment for 0.5 h. Notably, ClHSP70-1 and -7 were significantly downregulated after 1 h, the levels of ClHSP70-2 and -8 reached the lowest after 6 h, while the lowest level of ClHSP70-6 was found after 3 h. The expression of ClHSP70-1, -3, -4, -5, -7, -8 and -10 were significantly upregulated by short-term (0.5 or 1 h) cold treatment (Figure 8B). Prolonged treatment inhibited the transcription of many genes to varying degrees. For example, both ClHSP70-6 and -8 were significantly downregulated after 3 and 6 h, while the lowest level of ClHSP70-12 appeared after 12 h. The levels of ClHSP70-2 and -3 at 12 and 24 h of treatment were higher than those at other time periods. Different from other ClHSP70s regulated by low temperature, ClHSP70-9 was not sensitive to cold stress. In summary, ClHSP70s may function in the formation of drought and cold stress response networks in watermelon.
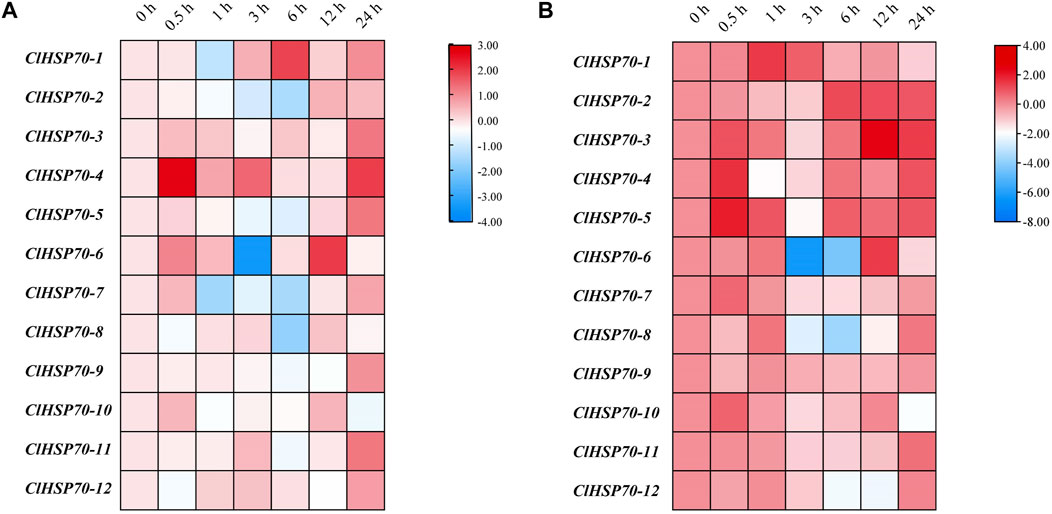
FIGURE 8. Expression profiles of ClHSP70 genes in response to drought and cold stress. qRT-PCR analysis of relative ClHSP70 transcript levels in watermelon plants exposed to drought stress (A) and cold stress (B). Drought stress, 30-day-old seedlings treated with 10% PEG6000 for 24 h; cold stress, 30-day-old seedlings treated with 4°C for 24 h. Blocks with blue colors indicated the decreased and red ones indicated the increased transcription levels. qRT-PCR data were normalized using the watermelon ClYLS8 and ClACT genes. The expression levels were relative to that of the corresponding control (seedlings without treatment at the same time). The relative expression data were log2 transformed. The heat maps were created with TBtools.
3.9 Response of ClHSP70s to exogenous ABA
ABA signaling pathway is involved in various stresses. The promoters of ClHSP70s contained many ABA-responsive elements, suggesting that the expression of these genes may be regulated by ABA. Therefore, 30-day-old watermelon seedlings were treated with exogenous ABA (100 μM), and the expression patterns of ClHSP70s in leaves at different times after treatment (0, 1, 3, 6, 12, and 24 h) were analyzed. As shown in Figure 9, the levels of five members (ClHSP70-7, -9, -10, -11, and -12) were downregulated by ABA, while the remaining seven genes (ClHSP70-1, -2, -3, -4, -5, -6, and -8) were upregulated at specific time points. The expression patterns of ClHSP70-1 and -6 were similar. Their levels increased gradually with the extension of post-treatment time and reached the highest at 24 h after treatment, which increased 2.7- and 24-fold, respectively. The response of ClHSP70-4 to ABA was relatively slow, and its expression level increased significantly (nearly 30-fold) only at 24 h after treatment. On the contrary, ClHSP70-2, -3 and -5 responded rapidly to ABA, and were strongly induced at 1 h after treatment, which increased by about 300-, 30- and 500-fold, respectively (Figure 9). These data suggest that watermelon ClHSP70s may be widely involved in ABA-mediated regulation of abiotic stresses.
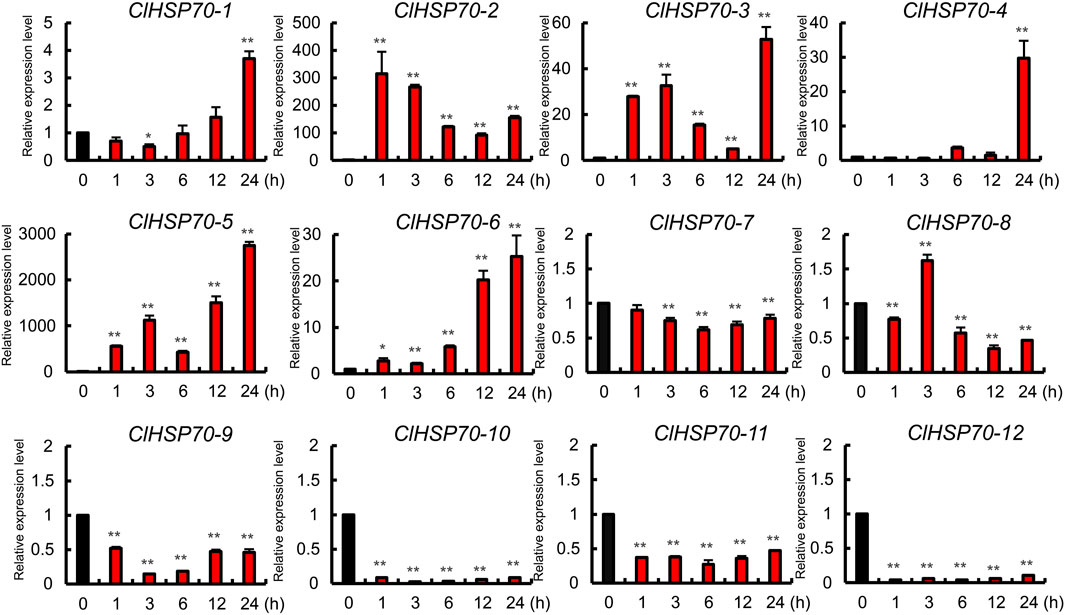
FIGURE 9. Expression analysis of ClHSP70 genes in response to ABA. qRT-PCR data were normalized using the watermelon ClACT gene. ABA treatment, 30-day-old seedlings were sprayed with 100 μM ABA, and the expression levels of ClHSP70s in leaves at different times after treatment were tested. The expression levels were relative to that of the corresponding control (seedlings without treatment at the same time). All statistical tests were two-tailed Student’s t-tests at the 0.05 and 0.01 levels, and the p-values are given by the symbols * (p < 0.05) and ** (p < 0.01), respectively.
3.10 Analysis of the binding site of ClHSP70s targeted by miRNA in watermelon
To determine whether ClHSP70s were regulated by microRNAs (miRNAs) through targeted binding sites, the binding sites of miRNAs targeting ClHSP70s were predicted. As shown in Figure 10, 10 ClHSP70s (ClHSP70-1, -2, -3, -4, -6, -8, -9, -10, -11, and -12) might be regulated by 28 miRNAs. Among them, four genes (ClHSP70-2, -3, -10, and -11) contained only one miRNA binding site, while the binding sites of ClHSP70-12 targeted by miRNA was the most. Notably, among the five binding sites regulating ClHSP70-4, two sites each were targeted by miR396a and miR396c, and one was targeted by miRN828. Furthermore, both miR396a and miR396c could regulate ClHSP70-6 and -9, and miR397 regulated ClHSP70-10 and -11 (Figure 10; Supplementary Table S4). These results indicate that one miRNA can regulate multiple ClHSP70s and vice versa.
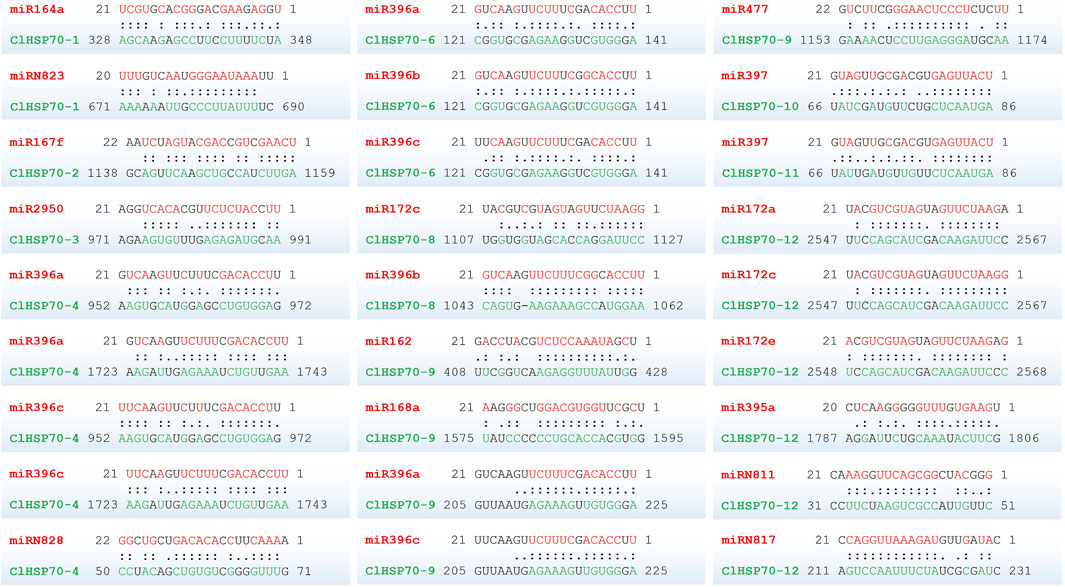
FIGURE 10. The predicted binding site of the ClHSP70s targeted by miRNAs in watermelon. Two dots indicated paired successfully between bases, and one dot indicated that there was also a pairing between U and G in the secondary structure. Blank space indicated that two bases failed to be paired. miRNAs and their paired bases were marked in red, and ClHSP70s and their paired bases were marked in green.
4 Discussion
Abiotic stresses adversely affect the growth and development of plants and lead to a significant decrease in crop yield. The molecular chaperone HSP70 proteins can enhance stress tolerance in plants (Saeed et al., 2019). Therefore, a comprehensive analysis of HSP70 family in plants is of great biological significance. Characterizing the HSP70 family at the genome-wide level has been performed in a variety of plants, such as Arabidopsis, tomato, pumpkin, grape, maize, and rice (Sung et al., 2001; Sarkar et al., 2013; Jiang et al., 2021; Abbas et al., 2022; Davoudi et al., 2022; Liu et al., 2022). However, this family in watermelon has not been systematically studied.
In this study, 12 ClHSP70s were identified in watermelon, which was similar to the number in cucumber (13) and grapevine (15), but less than that in tomato (19), maize (28) and rice (31) (Figure 3; Supplementary Table S1). This difference may be related to their genome sizes or evolutionary diversities (Sung et al., 2001; Zhang et al., 2015; Guo et al., 2016; Kang et al., 2022). Gene structure and phylogenetic tree were helpful to analyze the evolutionary relationship among genes(Wang et al., 2019). Genes closely related to phylogeny usually have similar properties or functions, and are generally consistent in subcellular locations (Cho and Choi, 2009; Kose et al., 2012). The Group I members of watermelon ClHSP70 family, such as ClHSP70-2, -3, -4 and -5, were closely related in evolution and had almost the same gene structure. All of them contained one intron and two exons, and the proteins encoding by these genes were localized in cytoplasm, indicating that they may have the similar function. The number of introns was generally affected by transcriptional regulation (Yu et al., 2021). Genes with many introns generally respond slowly to stresses, while genes with a small number of introns were sensitive to stresses (Jeffares et al., 2008; Jin et al., 2014). The distributions of exons among ClHSP70s in Group Ⅰ, Ⅱ, and Ⅲ were different, while the difference within the group was not significant (Figure 1), which was consistent with that in tea plants (Chen et al., 2018). Phylogenetic analysis revealed that watermelon ClHSP70 family was more closely related to cucumber than to Arabidopsis (Figure 3), as well as the results of pumpkin HSP70 family (Davoudi et al., 2022), indicating that HSP70 family is conserved during evolution, but also exhibits species specificity.
Gene duplication events not only support the functional evolution of genes but also innovate their structures, which is crucial to the evolution of gene families (Moore and Purugganam, 2003; Kong et al., 2007). Factors such as polyploidy and segmental and tandem duplication have extended the number of plant gene families and mutations in the regulatory regions of new members probably cloud modify the expression patterns and function of duplicated genes (Hashemipetroudi et al., 2023; Yaghobi and Heidari, 2023). Two or more adjacent gene duplications on the same chromosome were considered tandem duplications, while gene duplications on two different chromosomes were considered segmental duplications (Cannon et al., 2004). Collinear analysis identified two pairs of segmental repeats (ClHSP70-2/-3 and ClHSP70-2/-5) and a pair of tandem repeats (ClHSP70-3/-5) (Figure 2), which was consistent with the finding that segmental duplication was more than tandem duplication in soybean families (Schlueter et al., 2007). The Ka/Ks ratios of all gene pairs were less than 1 (Table 2), suggesting that this species had a strong Darwinian positive evolutionary selection as early as 1.7 Mya (Omidbakhshfard et al., 2015).
Plant HSP genes, such as HSP20s, HSP70s and HSP90s, have obvious tissue specificity in various species (Wehmeyer and Vierling, 2000; Wang et al., 2004; Liu et al., 2018). For example, the levels of some Arabidopsis AtHSP90s were lower in stems and flowers, but higher in roots, while AtHSP90-1 only expressed in roots (Yabe et al., 1994). In Populus, except for PtHSP90-5a and -5b, PtHSP90 genes were mainly expressed in stems (Zhang et al., 2013). Moreover, the expression patterns of tomato SlHSP20s and tea CsHSP70s showed obvious tissue specificity (Yu et al., 2016; Chen et al., 2018). Similarly, the expression of watermelon ClHSP70s was also significantly different in multiple tissues (Figure 6). For instance, ClHSP70-4 highly expressed in stems, while its level in cotyledons was low or even absent, revealing that different ClHSP70s may function in specific tissues. Interestingly, ClHSP70-2/-3, ClHSP70-2/-5 and ClHSP70-3/-5 homologous genes pairs had similar expression patterns in different tissues. Their levels in true leaves and cotyledons were significantly higher than other two tissues, which was similar to the results in soybean (Cho and Hong, 2006). However, the expression patterns of homologous gene pairs were not completely consistent. For example, the levels of pepper CaHSP70-11/-16 homologous genes were significantly different in different tissues (Guo et al., 2016).
In addition, cis-acting elements in promoters also play central roles in gene expression. The ClHSP70 promoters mainly contained ABA-response elements (ABREs) and stress response elements (Figure 4B). The leucine zipper transcription factor AREBs usually bind to ABREs in the promoters to regulate downstream genes (Yoshida et al., 2010). ABA was widely involved in abiotic stress response in plants (Wasilewska et al., 2008; Lee and Luan, 2012). It can be speculated that ClHSP70s may be regulated by ABA and abiotic stresses. There were 70 ABRE elements in promoters of N. tabacum NtHSP70s, most of which were activated by ABA (Song et al., 2019). In this experiment, most ClHSP70s were also regulated by ABA (Figure 9). ClHSP70-2, -3, -4, -5 and -6 showed ultra-high expression, while the levels of ClHSP70-10 and -12 decreased under ABA treatment. This different response to ABA may be closely related to the number and location of ABREs in their promoters. However, the function of ABREs, ABA and AREBs in the expression regulation of watermelon ClHSP70s needs further study. Furthermore, SSRs may play an important role in gene regulation (Panzade et al., 2021). In this study, a total of 66 potential SSRs were mined from ClHSP70s (Supplementary Table S8). Most SSRs were located in the intergenic region (promoter regions and 3′untranslated regions), which was similar to that in Ziziphus jujuba (Panzade et al., 2021). The identification and analysis of SSRs of these genes will help to identify the functions of ClHSP70s and enhance the efficiency of stress tolerance breeding in crops (Fu et al., 2023).
Plant HSP70 genes are involved in various abiotic stress responses and have potential functions in improving resistance to stresses (Alvim et al., 2001). Most tea CsHSP70 genes had a positive response to drought stress (Chen et al., 2018). Twelve barley HvHSP70s were induced after drought stress, and PtHSP70s showed different expression patterns in drought-sensitive and -tolerant Populus species after drought stress (Zhang et al., 2013; Chaudhary et al., 2019). Based on the analysis of RNA-seq data, watermelon ClHSP70s were found to be involved in the response to cold and drought stress (Figure 7). qRT-PCR analysis further found that ClHSP70-1, -4, and -6 were strongly induced by PEG-simulated drought treatment (Figure 8A), suggesting that these genes may participate in drought response. In addition, HSP70s also responded to cold stress (Chaudhry and Sidhu, 2022). Most grape VaHSP70s showed obvious stress regulation under cold conditions, among which VaHSP70-19 was continuously highly expressed under cold treatment, whereas other VaHSP70s were downregulated (Liu et al., 2022). The levels of Arabidopsis AtHSP70s and the chaperone genes were upregulated after cold treatment, while AtHSP70-16 was negatively regulated in seed germination, which may be a protective mechanism against cold stress (Ashraf et al., 2021). The expression of watermelon ClHSP70-3, -4 and -5 was upregulated under cold stress, however, ClHSP70-6 and -8 were significantly downregulated at 3 and 6 h (Figure 8B). In conclusion, ClHSP70s were regulated by ABA, cold and drought stress, suggesting that ClHSP70 family may function in abiotic stress response mediated by ABA.
The heat shock elements (HSEs) in promoters are important for the regulation of corresponding genes under heat stress (Sarkar et al., 2013; Guo et al., 2016). However, no HSEs were identified in ClHSP70 promoters, consistent with the case of Pyropia yezoensis PyHSP70s (Yu et al., 2021). Despite this, ClHSP70 promoters contained many elements related to stress and hormone response (Figure 4B), implying the potential function of ClHSP70s in heat response. In fact, the molecular chaperone function of HSP70s was identified mainly under heat stress conditions (Sung et al., 2001). GO enrichment analysis also showed that some ClHSP70s were involved in protein folding and unfolded protein binding (Supplementary Figure S3). ClHSP70s may participate in the heat response of watermelon through indirect ways, such as hormonal signals or cross pathways between different stresses. Identification of ClHSP70s′ functions under high temperature conditions is helpful to clarify the molecular mechanism of heat response, providing theoretical guidance for the genetic improvement of watermelon heat tolerance. However, there may be redundancy in the functions of different members of the ClHSP70 family in heat or other abiotic stress response, making it particularly important to parse the function of each ClHSP70 member. Rapid advances in biotechnology are expected to accelerate this goal. For example, genetic transformation technology can be used to create materials for excessive gene expression, RNA interference (RNAi) or virus induced gene silencing technology (VIGS) can be used to knock down the expression of a single target gene. At the same time, transcriptome sequencing can be used to analyze the effect of specific target gene changes on the expression of other genes in plants under abiotic stresses. The co-expression network constructed using transcriptome data can functionally annotate the regulated genes (Panahi et al., 2019), thus clarifying the roles of watermelon ClHSP70s in different biological pathways under abiotic stresses. In addition, gene editing techniques can be used to simultaneously edit multiple members of ClHSP70 family, creating mutants with multiple genes knocked out, as in tomato (Li et al., 2018). This method not only helps to verify the interaction of different members under abiotic stresses, but also provides new germplasm for genetic improvement of watermelon stress tolerance.
miRNAs are essential modulators of transcriptional gene regulation in plants (Zakeel et al., 2019). Up to now, many plant miRNAs have been identified to respond to different stress conditions. For example, in Arabidopsis, miR396 played an important role in response to salt stress (Li et al., 2008) and miRNA397 improved plant tolerance to cold stress (Dong and Pei, 2014). In addition, several studies have revealed that miR396 and miR397 targeted the stress (heat, cold, drought, and salt)-responsive HSP70 genes in Brassica rapa (Tabusam et al., 2022), Populus (Yer et al., 2016) and common bean (Büyük et al., 2016). In this study, miR396 targeted ClHSP70-4, -6, -8 and -9, while miR397 targeted ClHSP70-10 and -11. Many other miRNAs, such as miR164, miR167, miR168, miR172 and miR395, also targeted watermelon ClHSP70s (Figure 10; Supplementary Table S4). Therefore, the identification of miRNAs may play a crucial role in understanding the function of ClHSP70 family genes.
In this study, ClHSP70 family in watermelon genome was characterized for the first time. A total of 12 genes were identified. We analyzed the physical and chemical properties of this family. The systematic analysis of gene structure, phylogenetic tree, cis-acting elements in promoters, protein interactions, SSRs, GO enrichment analysis, binding sites targeted by miRNAs, and collinearity were performed. In addition, the expression patterns of ClHSP70s were identified by RNA-seq and qRT-PCR. Watermelon ClHSP70s were tissue specific, and had different degrees of response to ABA, drought and cold stress. Our results provide a reference for a better understanding of the functions of ClHSP70 family in watermelon.
Data availability statement
The original contributions presented in the study are included in the article/Supplementary Material, further inquiries can be directed to the corresponding author.
Author contributions
MG and XW designed the research. XW, ZJ, and YD performed the research. XW and ZJ analyzed the data and discussed the results. XW wrote the paper. MG reviewed the manuscript. All authors contributed to the article and approved the submitted version.
Funding
This work was supported by the Science and Technology Major Project of Ningxia (Grant Nos. 2021BBF02024 and NXNYYZ20200101), the Natural Science Foundation of Ningxia (Grant No. 2021AAC03099), and the Key R&D Program of Ningxia (Grant No. 2021BEB04057).
Conflict of interest
The authors declare that the research was conducted in the absence of any commercial or financial relationships that could be construed as a potential conflict of interest.
Publisher’s note
All claims expressed in this article are solely those of the authors and do not necessarily represent those of their affiliated organizations, or those of the publisher, the editors and the reviewers. Any product that may be evaluated in this article, or claim that may be made by its manufacturer, is not guaranteed or endorsed by the publisher.
Supplementary material
The Supplementary Material for this article can be found online at: https://www.frontiersin.org/articles/10.3389/fgene.2023.1201535/full#supplementary-material
References
Abbas, M., Li, Y., Elbaiomy, R. G., Yan, K., Ragauskas, A. J., Yadav, V., et al. (2022). Genome-wide analysis and expression profiling of SlHsp70 gene family in Solanum lycopersicum revealed higher expression of SlHsp70-11 in roots under Cd2+ stress. Front. Biosci.-Landmrk 27, 186. doi:10.31083/j.fbl2706186
Alvim, F. C., Carolino, S. M., Cascardo, J. C., Nunes, C. C., Martinez, C. A., Otoni, W. C., et al. (2001). Enhanced accumulation of BiP in transgenic plants confers tolerance to water stress. Plant Physiol. 126, 1042–1054. doi:10.1104/pp.126.3.1042
Ashraf, M., Mao, Q., Hong, J., Shi, L., Ran, X., Liaquat, F., et al. (2021). HSP70-16 and VDAC3 jointly inhibit seed germination under cold stress in Arabidopsis. Plant Cell Environ. 44, 3616–3627. doi:10.1111/pce.14138
Bailey, T. L., Boden, M., Buske, F. A., Frith, M., Grant, C. E., Clementi, L., et al. (2009). Meme suite: Tools for motif discovery and searching. Nucleic Acids Res. 37, W202. doi:10.1093/nar/gkp335
Beier, S., Thiel, T., Münch, T., Scholz, U., and Mascher, M. (2017). MISA-Web: A web server for microsatellite prediction. Bioinformatics 33, 2583–2585. doi:10.1093/bioinformatics/btx198
Büyük, İ., Inal, B., Ilhan, E., Tanriseven, M., Aras, S., and Erayman, M. (2016). Genome-wide identification of salinity responsive HSP70s in common bean. Mol. Biol. Rep. 43, 1251–1266. doi:10.1007/s11033-016-4057-0
Cannon, S. B., Mitra, A., Baumgarten, A., Young, N. D., and May, G. (2004). The roles of segmental and tandem gene duplication in the evolution of large gene families in Arabidopsis thaliana. BMC Plant Biol. 4, 10. doi:10.1186/1471-2229-4-10
Chaudhary, R., Baranwal, V. K., Kumar, R., Sircar, D., and Chauhan, H. (2019). Genome-wide identification and expression analysis of Hsp70, Hsp90, and Hsp100 heat shock protein genes in barley under stress conditions and reproductive development. Funct. Integr. Genomics 19, 1007–1022. doi:10.1007/s10142-019-00695-y
Chaudhry, S., and Sidhu, G. (2022). Climate change regulated abiotic stress mechanisms in plants: A comprehensive review. Plant Cell Rep. 41, 1–31. doi:10.1007/s00299-021-02759-5
Chen, C., Chen, H., Zhang, Y., Thomas, H. R., Frank, M. H., He, Y., et al. (2020). TBtools: An integrative toolkit developed for interactive analyses of big biological data. Mol. Plant 13, 1194–1202. doi:10.1016/j.molp.2020.06.009
Chen, J., Gao, T., Wan, S., Zhang, Y., Yang, J., Yu, Y., et al. (2018). Genome-wide identification, classification and expression analysis of the HSP gene superfamily in tea plant (Camellia sinensis). Int. J. Mol. Sci. 19, 2633. doi:10.3390/ijms19092633
Chen, Z., Zhou, T., Wu, X., Hong, Y., Fan, Z., and Li, H. (2008). Influence of cytoplasmic heat shock protein 70 on viral infection of Nicotiana benthamiana. Mol. Plant Pathol. 9, 809–817. doi:10.1111/j.1364-3703.2008.00505.x
Cho, E. K., and Choi, Y. J. (2009). A nuclear-localized HSP70 confers thermoprotective activity and drought-stress tolerance on plants. Biotechnol. Lett. 31, 597–606. doi:10.1007/s10529-008-9880-5
Cho, E. K., and Hong, C. B. (2006). Over-expression of tobacco NtHSP70-1 contributes to drought-stress tolerance in plants. Plant Cell Rep. 25, 349–358. doi:10.1007/s00299-005-0093-2
Dai, X., Zhuang, Z., and Zhao, P. X. (2018). psRNATarget: a plant small RNA target analysis server (2017 release). Nucleic Acids Res. 46, W49–W54. doi:10.1093/nar/gky316
Davoudi, M., Chen, J., and Lou, Q. (2022). Genome-wide identification and expression analysis of heat shock protein 70 (HSP70) gene family in pumpkin (Cucurbita moschata) rootstock under drought stress suggested the potential role of these chaperones in stress tolerance. Int. J. Mol. Sci. 23, 1918. doi:10.3390/ijms23031918
Dickinson, P. J., Kumar, M., Martinho, C., Yoo, S. J., Lan, H., Artavanis, G., et al. (2018). Chloroplast signaling gates thermotolerance in Arabidopsis. Cell Rep. 22, 1657–1665. doi:10.1016/j.celrep.2018.01.054
Dong, C. H., and Pei, H. (2014). Over-expression of miR397 improves plant tolerance to cold stress in Arabidopsis thaliana. J. Plant Biol. 57, 209–217. doi:10.1007/s12374-013-0490-y
Dong, Y. S., Fatma, K., and Charles, L. G. (2001). Plant Hsp70 molecular chaperones: Protein structure, gene family, expression and function. Physiol. Plant. 113, 443–451. doi:10.1034/j.1399-3054.2001.1130402.x
Dragovic, Z., Broadley, S. A., Shomura, Y., Bracher, A., and Hartl, F. U. (2006). Molecular chaperones of the Hsp110 family act as nucleotide exchange factors of Hsp70s. EMBO J. 25, 2519–2528. doi:10.1038/sj.emboj.7601138
Esposito, S., Sorbo, S., Conte, B., and Basile, A. (2012). Effects of heavy metals on ultrastructure and HSP70s induction in the aquatic moss Leptodictyum riparium Hedw. Int. J. Phytoremediat. 14, 443–455. doi:10.1080/15226514.2011.620904
Fei, J., Wang, Y., Zhou, Q., and Gu, J. (2015). Cloning and expression analysis of HSP70 gene from mangrove plant Kandelia obovata under cold stress. Ecotoxicology 24, 1677–1685. doi:10.1007/s10646-015-1484-y
Finn, R. D., Bateman, A., Clements, J., Coggill, P., Eberhardt, R. Y., Eddy, S. R., et al. (2014). Pfam: The protein families database. Nucleic Acids Res. 42, 222–230. doi:10.1093/nar/gkt1223
Finn, R. D., Clements, J., and Eddy, S. R. (2011). HMMER web server: Interactive sequence similarity searching. Nucleic Acids Res. 39, 29–37. doi:10.1093/nar/gkr367
Fu, X., Yang, N., Du, Y., Kamran, H. M., Wang, H., Chen, S., et al. (2023). Development of SSR molecular markers and genetic diversity analysis of TPS gene family in Chimonanthus praecox. Agriculture 13, 893. doi:10.3390/agriculture13040893
Gu, Z., Cavalcanti, A., Chen, F. C., Bouman, P., and Li, W. H. (2002). Extent of gene duplication in the genomes of Drosophila, nematode, and yeast. Mol. Biol. Evol. 19, 256–262. doi:10.1093/oxfordjournals.molbev.a004079
Guo, M., Liu, J. H., Ma, X., Zhai, Y. F., Gong, Z. H., and Lu, M. H. (2016). Genome-wide analysis of the Hsp70 family genes in pepper (Capsicum annuum L.) and functional identification of CaHsp70-2 involvement in heat stress. Plant Sci. 252, 246–256. doi:10.1016/j.plantsci.2016.07.001
Guo, S., Zhang, J., Sun, H., Salse, J., Lucas, W. J., Zhang, H., et al. (2013). The draft genome of watermelon (Citrullus lanatus) and resequencing of 20 diverse accessions. Nat. Genet. 45, 51–58. doi:10.1038/ng.2470
Guo, Z., Kuang, Z., Zhao, Y., Deng, Y., He, H., Wan, M., et al. (2022). PmiREN2. 0: From data annotation to functional exploration of plant microRNAs. Nucleic Acids Res. 50, D1475–D1482. doi:10.1093/nar/gkab811
Guy, C. L., and Li, Q. B. (1998). The organization and evolution of the spinach stress 70 molecular chaperone gene family. Plant Cell 10, 539–556. doi:10.1105/tpc.10.4.539
Hashemipetroudi, S. H., Arab, M., Heidari, P., and Kuhlmann, M. (2023). Genome-wide analysis of the laccase (lac) gene family in Aeluropus littoralis: A focus on identification, evolution and expression patterns in response to abiotic stresses and ABA treatment. Front. Plant Sci. 14, 297. doi:10.3389/fpls.2023.1112354
Jeffares, D. C., Penkett, C. J., and Bahler, J. (2008). Rapidly regulated genes are intron poor. Trends Genet. 24, 375–378. doi:10.1016/j.tig.2008.05.006
Jiang, L., Hu, W., Qian, Y., Ren, Q., and Zhang, J. (2021). Genome-wide identification, classification and expression analysis of the Hsf and Hsp70 gene families in maize. Gene 770, 145348. doi:10.1016/j.gene.2020.145348
Jin, Z., Chandrasekaran, U., and Liu, A. (2014). Genome-wide analysis of the Dof transcription factors in castor bean (Ricinus communis L.). Genes Genom 36, 527–537. doi:10.1007/s13258-014-0189-6
Jung, K. H., Gho, H. J., Nguyen, M. X., Kim, S. R., and An, G. (2013). Genome-wide expression analysis of HSP70 family genes in rice and identification of a cytosolic HSP70 gene highly induced under heat stress. Funct. Integr. Genomics 13, 391–402. doi:10.1007/s10142-013-0331-6
Kang, L., Teng, Y., Cen, Q., Fang, Y., Tian, Q., Zhang, X., et al. (2022). Genome-wide identification of R2R3-MYB transcription factor and expression analysis under abiotic stress in rice. Plants (Basel) 11, 1928. doi:10.3390/plants11151928
Kong, H., Landherr, L. L., Frohlich, M. W., Leebens-Mack, J., Ma, H., and DePamphilis, C. W. (2007). Patterns of gene duplication in the plant SKP1 gene family in angiosperms: Evidence for multiple mechanisms of rapid gene birth. Plant J. 50, 873–885. doi:10.1111/j.1365-313X.2007.03097.x
Kong, Q., Yuan, J., Gao, L., Zhao, S., Jiang, W., Huang, Y., et al. (2014). Identification of suitable reference genes for gene expression normalization in qRT-PCR analysis in watermelon. PLoS One 9, e90612. doi:10.1371/journal.pone.0090612
Kose, S., Furuta, M., Imamoto, N., and Hikeshi, (2012). A nuclear import carrier for Hsp70s, protects cells from heat shock-induced nuclear damage. Cell 149, 578–589. doi:10.1016/j.cell.2012.02.058
Lee, S. C., and Luan, S. (2012). ABA signal transduction at the crossroad of biotic and abiotic stress responses. Plant Cell Environ. 35, 53–60. doi:10.1111/j.1365-3040.2011.02426.x
Li, F., Duan, P., Zhang, H., Lu, X., Shi, Z., and Cui, J. (2022). Genome-wide identification of CmaGH3 family genes, and expression analysis in response to cold and hormonal stresses in Cucurbita maxima. Sci. Hortic. 304, 111256. doi:10.1016/j.scienta.2022.111256
Li, T., Yang, X., Yu, Y., Si, X., Zhai, X., Zhang, H., et al. (2018). Domestication of wild tomato is accelerated by genome editing. Nat. Biotechnol. 36, 1160–1163. doi:10.1038/nbt.4273
Li, W. X., Oono, Y., Zhu, J., He, X. J., Wu, J. M., Iida, K., et al. (2008). The Arabidopsis NFYA5 transcription factor is regulated transcriptionally and posttranscriptionally to promote drought resistance. Plant Cell 20, 2238–2251. doi:10.1105/tpc.108.059444
Lin, B. L., Wang, J. S., Liu, H. C., Chen, R. W., Meyer, Y., Barakat, A., et al. (2001). Genomic analysis of the Hsp70 superfamily in Arabidopsis thaliana. Cell Stress Chaperones 6, 201–208. doi:10.1379/1466-1268(2001)006<0201:GAOTHS>2.0.CO;2
Liu, J., Pang, X., Cheng, Y., Yin, Y., Zhang, Q., Su, W., et al. (2018). The Hsp70 gene family in Solanum tuberosum: Genome-wide identification, phylogeny, and expression patterns. Sci. Rep. 8, 16628. doi:10.1038/s41598-018-34878-7
Liu, X., Chen, H., Li, S., and Wang, L. (2022). Genome-wide identification of the Hsp70 gene family in grape and their expression profile during abiotic stress. Horticulturae 8, 743. doi:10.3390/horticulturae8080743
Lopes-Caitar, V. S., de Carvalho, M. C., Darben, L. M., Kuwahara, M. K., Nepomuceno, A. L., Dias, W. P., et al. (2013). Genome-wide analysis of the Hsp20 gene family in soybean: Comprehensive sequence, genomic organization and expression profile analysis under abiotic and biotic stresses. BMC Genomics 14, 1–17. doi:10.1186/1471-2164-14-577
Lubkowska, A., Pluta, W., Strońska, A., and Lalko, A. (2021). Role of heat shock proteins (HSP70 and HSP90) in viral infection. Int. J. Mol. Sci. 22, 9366. doi:10.3390/ijms22179366
Lynch, M., and Conery, J. S. (2000). The evolutionary fate and consequences of duplicate genes. Science 290, 1151–1155. doi:10.1126/science.290.5494.1151
Makaepea, M. M., Daniso, B., and Afam, I. O. J. (2019). Watermelon as a potential fruit snack. Int. J. Food Prop. 22, 355–370. doi:10.1080/10942912.2019.1584212
Mashilo, J., Shimelis, H., Ngwepe, R. M., and Thungo, Z. (2022). Genetic analysis of fruit quality traits in sweet watermelon (Citrullus lanatus var. lanatus): A review. Front. Plant Sci. 13, 834696. doi:10.3389/fpls.2022.834696
Mohamed, H. A. (2011). Plant heat-shock proteins: A mini review. J. King Saud. Univ. Sci. 23, 139–150. doi:10.1016/j.jksus.2010.06.022
Moore, R. C., and Purugganan, M. D. (2003). The early stages of duplicate gene evolution. Proc. Natl. Acad. Sci. U. S. A. 100, 15682–15687. doi:10.1073/pnas.2535513100
Nagaraju, M., Kumar, A., Rajasheker, G., Manohar Rao, D., and Kavi Kishor, P. B. (2020). DnaJs, the critical drivers of Hsp70s: Genome-wide screening, characterization and expression of DnaJ family genes in Sorghum bicolor. Mol. Biol. Rep. 47, 7379–7390. doi:10.1007/s11033-020-05793-w
Omidbakhshfard, M. A., Proost, S., Fujikura, U., and Mueller-Roeber, B. (2015). Growth-regulating factors (GRFs): A small transcription factor family with important functions in plant biology. Mol. Plant 8, 998–1010. doi:10.1016/j.molp.2015.01.013
Osman, A. G. M., Wuertz, S., and Mohammed-Geba, K. (2019). Lead-induced heat shock protein (HSP70) and metallothionein (MT) gene expression in the embryos of African catfish Clarias gariepinus (Burchell, 1822). Sci. Afr. 3, e00056. doi:10.1016/j.sciaf.2019.e00056
Panahi, B., Mohammadi, S. A., Ruzicka, K., Abbasi Holaso, H., and Zare Mehrjerdi, M. (2019). Genome-wide identification and co-expression network analysis of nuclear factor-Y in barley revealed potential functions in salt stress. Physiol. Mol. Biol. Pla. 25, 485–495. doi:10.1007/s12298-018-00637-1
Panzade, K. P., Kale, S. S., Chavan, N. R., and Hatzade, B. (2021). Genome-wide analysis of Hsp70 and Hsp100 gene families in Ziziphus jujuba. Cell Stress Chaperon 26, 341–353. doi:10.1007/s12192-020-01179-w
Queitsch, C., Hong, S. W., Vierling, E., and Lindquist, S. (2000). Heat shock protein 101 plays a crucial role in thermotolerance in Arabidopsis. Plant Cell 12, 479–492. doi:10.1105/tpc.12.4.479
Ritossa, F. (1996). Discovery of the heat shock response. Cell Stress Chaperones 1, 97. doi:10.1379/1466-1268(1996)001<0097:dothsr>2.3.co;2
Saeed, U. H., Abid, K., Muhammad, A., Abdul, M. K., Wen-Xian, G., Huai-Xia, Z., et al. (2019). Heat shock proteins: Dynamic biomolecules to counter plant biotic and abiotic stresses. Int. J. Mol. Sci. 20, 5321. doi:10.3390/ijms20215321
Sarkar, N. K., Kundnani, P., and Grover, A. (2013). Functional analysis of Hsp70 superfamily proteins of rice (Oryza sativa). Cell Stress Chaperones 18, 427–437. doi:10.1007/s12192-012-0395-6
Schlueter, J. A., Lin, J. Y., Schlueter, S. D., Vasylenko-Sanders, I. F., Deshpande, S., Yi, J., et al. (2007). Gene duplication and paleopolyploidy in soybean and the implications for whole genome sequencing. BMC Genomics 8, 330. doi:10.1186/1471-2164-8-330
Schmittgen, T. D., and Livak, K. J. (2008). Analyzing real-time PCR data by the comparative CT method. Nat. Protoc. 3, 1101–1108. doi:10.1038/nprot.2008.73
Song, Z., Pan, F., Lou, X., Wang, D., Yang, C., Zhang, B., et al. (2019). Genome-wide identification and characterization of Hsp70 gene family in Nicotiana tabacum. Mol. Biol. Rep. 46, 1941–1954. doi:10.1007/s11033-019-04644-7
Sung, D. Y., Vierling, E., and Guy, C. L. (2001). Comprehensive expression profile analysis of the Arabidopsis Hsp70 gene family. Plant Physiol. 126, 789–800. doi:10.1104/pp.126.2.789
Tabusam, J., Shi, Q., Feng, D., Zulfiqar, S., Shen, S., Ma, W., et al. (2022). HSP70 gene family in Brassica rapa: Genome-wide identification, characterization, and expression patterns in response to heat and cold stress. Cells 11, 2316. doi:10.3390/cells11152316
Tamura, K., Stecher, G., and Kumar, S. (2021). MEGA11: Molecular evolutionary genetics analysis version 11. Mol. Biol. Evol. 38, 3022–3027. doi:10.1093/molbev/msab120
Tanya, R., and Elizabeth, R. W. (2007). Comparative genomic analysis of the Hsp70s from five diverse photosynthetic eukaryotes. Cell Stress Chaperones 12, 172. doi:10.1379/CSC-230R1.1
Timperio, A. M., Egidi, M. G., and Zolla, L. (2008). Proteomics applied on plant abiotic stresses: Role of heat shock proteins (HSP). J. Proteomics 71, 391–411. doi:10.1016/j.jprot.2008.07.005
Wang, C., Ye, D., Li, Y., Hu, P., Xu, R., and Wang, X. (2023). Genome-wide identification and bioinformatics analysis of the WRKY transcription factors and screening of candidate genes for anthocyanin biosynthesis in azalea (Rhododendron simsii). Front. Genet. 14, 1172321. doi:10.3389/fgene.2023.1172321
Wang, L., Guo, K., Li, Y., Tu, Y., Hu, H., Wang, B., et al. (2010). Expression profiling and integrative analysis of the CESA/CSL superfamily in rice. BMC Plant Biol. 10, 282. doi:10.1186/1471-2229-10-282
Wang, W. X., Vinocur, B., Shoseyov, O., and Altman, A. (2004). Role of plant heat-shock proteins and molecular chaperones in the abiotic stress response. Trends Plant Sci. 9, 244–252. doi:10.1016/j.tplants.2004.03.006
Wang, X. R., Wang, C., Ban, F. X., Zhu, D. T., Liu, S. S., and Wang, X. W. (2019). Genome-wide identification and characterization of HSP gene superfamily in whitefly (Bemisia tabaci) and expression profiling analysis under temperature stress. Insect Sci. 26, 44–57. doi:10.1111/1744-7917.12505
Wasilewska, A., Vlad, F., Sirichandra, C., Redko, Y., Jammes, F., Valon, C., et al. (2008). An update on abscisic acid signaling in plants and more. Mol. Plant 1, 198–217. doi:10.1093/mp/ssm022
Waterhouse, A., Bertoni, M., Bienert, S., Studer, G., Tauriello, G., Gumienny, R., et al. (2018). SWISS-MODEL: Homology modelling of protein structures and complexes. Nucleic Acids Res. 46, W296–W303. doi:10.1093/nar/gky427
Wehmeyer, N., and Vierling, E. (2000). The expression of small heat shock proteins in seeds responds to discrete developmental signals and suggests a general protective role in desiccation tolerance. Plant Physiol. 122, 1099–1108. doi:10.1104/pp.122.4.1099
Wei, F., Coe, E., Nelson, W., Bharti, A. K., Engler, F., Butler, E., et al. (2007). Physical and genetic structure of the maize genome reflects its complex evolutionary history. PLoS Genet. 3, e123. doi:10.1371/journal.pgen.0030123
Yabe, N., Takahashi, T., and Komeda, Y. (1994). Analysis of tissue-specific expression of Arabidopsis thaliana HSP90-family gene HSP81. Plant Cell Physiol. 35, 1207–1219. doi:10.1093/oxfordjournals.pcp.a078715
Yaghobi, M., and Heidari, P. (2023). Genome-wide analysis of aquaporin gene family in Triticum turgidum and its expression profile in response to salt stress. Genes 14, 202. doi:10.3390/genes14010202
Yang, S., Zhang, X., Yue, J. X., Tian, D., and Chen, J. Q. (2008). Recent duplications dominate NBS-encoding gene expansion in two woody species. Mol. Genet. Genomics 280, 187–198. doi:10.1007/s00438-008-0355-0
Yer, E. N., Baloglu, M. C., Ziplar, U. T., Ayan, S., and Unver, T. (2016). Drought-responsive Hsp70 gene analysis in Populus at genome-wide level. Plant Mol. Biol. Rep. 34, 483–500. doi:10.1007/s11105-015-0933-3
Yoshida, T., Fujita, Y., Sayama, H., Kidokoro, S., Maruyama, K., Mizoi, J., et al. (2010). AREB1, AREB2, and ABF3 are master transcription factors that cooperatively regulate ABRE-dependent ABA signaling involved in drought stress tolerance and require ABA for full activation. Plant J. 61, 672–685. doi:10.1111/j.1365-313X.2009.04092.x
Yu, C., Rong, M., Liu, Y., Sun, P., Xu, Y., and Wei, J. (2021). Genome-wide identification and characterization of HSP70 gene family in sinensis (Lour.) Gilg. Genes (Basel). 13, 8. doi:10.3390/genes13010008
Yu, J., Cheng, Y., Feng, K., Ruan, M., Ye, Q., Wang, R., et al. (2016). Genome-wide identification and expression profiling of tomato Hsp20 gene family in response to biotic and abiotic stresses. Front. Plant Sci. 7, 1215. doi:10.3389/fpls.2016.01215
Yu, X., Mo, Z., Tang, X., Gao, T., and Mao, Y. (2021). Genome-wide analysis of HSP70 gene superfamily in Pyropia yezoensis (bangiales, rhodophyta): Identification, characterization and expression profiles in response to dehydration stress. BMC Plant Biol. 21, 435. doi:10.1186/s12870-021-03213-0
Zakeel, M. C. M., Safeena, M. I. S., and Komathy, T. (2019). In silico identification of microRNAs and their target genes in watermelon (Citrullus lanatus). Sci. Hortic. 252, 55–60. doi:10.1016/j.scienta.2019.02.012
Zhang, H., Zhu, J., Gong, Z., and Zhu, J. (2022). Abiotic stress responses in plants. Nat. Rev. Genet. 23, 104–119. doi:10.1038/s41576-021-00413-0
Zhang, J., Li, J., Liu, B., Zhang, L., Chen, J., and Lu, M. (2013). Genome-wide analysis of the Populus Hsp90 gene family reveals differential expression patterns, localization, and heat stress responses. BMC Genomics 14, 532. doi:10.1186/1471-2164-14-532
Zhang, L., Zhao, H., Dong, Q., Zhang, Y., Wang, Y., Li, H., et al. (2015). Genome-wide analysis and expression profiling under heat and drought treatments of HSP70 gene family in soybean (Glycine max L.). Front. Plant Sci. 6, 773. doi:10.3389/fpls.2015.00773
Keywords: heat shock proteins, genome-wide analysis, expression pattern, ABA, abiotic stress
Citation: Wang X, Jin Z, Ding Y and Guo M (2023) Characterization of HSP70 family in watermelon (Citrullus lanatus): identification, structure, evolution, and potential function in response to ABA, cold and drought stress. Front. Genet. 14:1201535. doi: 10.3389/fgene.2023.1201535
Received: 06 April 2023; Accepted: 22 May 2023;
Published: 31 May 2023.
Edited by:
Diaa Abd El Moneim, Arish University, EgyptReviewed by:
Balpreet Kaur Dhatt, University of Nebraska-Lincoln, United StatesBahman Panahi, Agricultural Biotechnology Research Institute of Iran, Iran
Anuj Kumar, Dalhousie University, Canada
Parviz Heidari, Shahrood University of Technology, Iran
Copyright © 2023 Wang, Jin, Ding and Guo. This is an open-access article distributed under the terms of the Creative Commons Attribution License (CC BY). The use, distribution or reproduction in other forums is permitted, provided the original author(s) and the copyright owner(s) are credited and that the original publication in this journal is cited, in accordance with accepted academic practice. No use, distribution or reproduction is permitted which does not comply with these terms.
*Correspondence: Meng Guo, bWd1b0BueHUuZWR1LmNu