- 1Children’s Hospital, Pediatric Research Center, University of Helsinki and Helsinki University Hospital, Helsinki, Finland
- 2Folkhälsan Research Center, Folkhälsan Institute of Genetics, Helsinki, Finland
- 3Research Program for Clinical and Molecular Metabolism, Faculty of Medicine, University of Helsinki, Helsinki, Finland
- 4Public Health Research, National Institute for Health and Welfare (THL), Helsinki, Finland
- 5PEDEGO Research Unit, MRC Oulu, Oulu University Hospital and University of Oulu, Oulu, Finland
- 6Department of Molecular Medicine and Surgery and Center for Molecular Medicine, Karolinska Institutet, and Clinical Genetics, Karolinska University Hospital, Stockholm, Sweden
Introduction: The effects of genetic variation in fibroblast growth factor 23 (FGF23) are unclear. This study explores the associations of single-nucleotide polymorphisms (SNPs) of FGF23 with phosphate and vitamin D metabolism and bone strength in early childhood.
Methods: The study is part of the vitamin D intervention in infant (VIDI) trial (2013–2016), in which healthy term infants born to mothers of Northern European origin received vitamin D3 supplementation of 10 or 30 μg/day from 2 weeks to 24 months of age (ClinicalTrials.gov NCT01723852). Intact and C-terminal FGF23 (cFGF23), 25-hydroxyvitamin D (25-OHD), parathyroid hormone, phosphate, and peripheral quantitative computed tomography (pQCT)-derived bone strength parameters were analyzed at 12 and 24 months. The study included 622 VIDI participants with genotyping data on FGF23 SNPs rs7955866, rs11063112, and rs13312770.
Results: Rs7955866 minor allele homozygotes had lowest cFGF23 at both time-points (mixed model for repeated measurements, pvariant = 0.009). Minor alleles of rs11063112 were associated with a greater age-related decrease in phosphate concentration (pinteraction = 0.038) from 12 to 24 months. Heterozygotes of rs13312770 had the greatest total bone mineral content (total BMC), cross-sectional area (total CSA), and polar moment of inertia (PMI) at 24 months (ANOVA p = 0.005, 0.037, and 0.036, respectively). Rs13312770 minor alleles were associated with a greater increase of total BMC, but a smaller increase of total CSA and PMI, during follow-up (pinteraction <0.001, 0.043, and 0.012, respectively). Genotype of FGF23 did not modify 25-OHD.
Conclusion: The study finds that genetic variation in FGF23 modifies cFGF23, phosphate, and pQCT-derived bone strength parameters from 12 to 24 months of age. These findings potentially promote an understanding of the regulation of FGF23 and its role in bone metabolism and temporal changes thereof during early childhood.
1 Introduction
The fibroblast growth factor 23 (FGF23) hormone, produced mainly by bone osteocytes, participates in phosphate (Pi) and vitamin D metabolism (ADHR Consortium, 2000). FGF23 was initially identified in studies on autosomal dominant hypophosphatemic rickets (ADHR), in which mutations in the FGF23 gene lead to excessive serum FGF23 concentration by preventing cleavage of the active intact FGF23 (iFGF23) into inactive N-terminal and C-terminal FGF23 (cFGF23). This results in higher iFGF23 concentrations and consequently in increased urinary phosphate loss (ADHR Consortium, 2000; White et al., 2001; Shimada et al., 2004; Takashi et al., 2018). Increased iFGF23 concentrations have, thereafter, been shown to be the underlying cause in several other genetic forms of hypophosphatemia (Shimada et al., 2001; Araya et al., 2005; Benet-Pagès et al., 2005; Martin et al., 2012; Gohil and Imel, 2019).
Phosphate is essential for bone mineralization and is mainly found in bone and intracellular compartments, with approximately 1% of total phosphate circulating in the blood stream. Circulating phosphate concentrations are tightly regulated by FGF23, 1,25 dihydroxyvitamin D (1,25-(OH)2D), and parathyroid hormone (PTH) (ADHR Consortium, 2000; Shimada et al., 2001; Erben and Andrukhova, 2017; Arnold et al., 2021). FGF23, in its intact form, directly reduces reabsorption of phosphate in the renal proximal tubules by suppressing the expression of sodium phosphate co-transporters, of which type IIa is encoded by the SLC34A1 gene. FGF23 also affects phosphate concentrations indirectly by participating in the regulation of 1,25-(OH)2D concentration by, for example, increasing the expression of 25-hydroxyvitamin D-24-hydroxylase, a catabolic enzyme of vitamin D, encoded by the CYP24A1 gene. Increased FGF23 leads to decreasing 1,25-(OH)2D concentrations and reduced intestinal phosphate uptake (Shimada, 2007; Martin et al., 2012; Quarles, 2012; Lederer, 2014; Takashi et al., 2018).
Circulating concentrations of phosphate and FGF23 show individual variation. Genome-wide association studies (GWAS) have identified several single-nucleotide polymorphisms (SNPs) associated with serum phosphate and FGF23 concentrations. SNPs near the genes FGF23 and SLC34A1 and CASR, the gene encoding the calcium sensing receptor, are associated with serum phosphate (Kestenbaum et al., 2010), while SNPs in genes involved in vitamin D metabolism, namely, CYP24A1, are associated with differences in circulating FGF23 concentrations (Robinson-Cohen et al., 2018).
The FGF23 polymorphism rs7955866 (SNP1) is a missense variant located in the third exon of FGF23 (c.716C>T, p.239T > M). Based on its location and the differing properties of the amino acid variants, rs7955866 is thought to significantly influence interactions of FGF23 with FGF receptors and Klotho, and thereby to have a role in phosphate homeostasis (Yamazaki et al., 2008; Rendina et al., 2012; Agoro et al., 2020). In adults with calcium nephrolithiasis, rs7955866 is associated with lower phosphate concentrations and increased urinary phosphate excretion (Rendina et al., 2012). FGF23 variation has also been described to relate to cardiovascular outcomes, particularly in patients with chronic kidney disease. The 3′UTR variant rs11063112 (c.2185A>T) (SNP2) and intronic variant rs13312770 (c.211 + 3287A>G) (SNP3) have been associated with elevated risk of cardiovascular mortality in hemodialysis patients with chronic kidney disease and end-stage chronic kidney disease, respectively (Rothe et al., 2017; Schwantes-An et al., 2019).
We have previously reported that in infants aged 3–24 months, the concentrations and modifying factors of FGF23 depend on sex and age, iFGF23 being higher in girls and both iFGF23 and cFGF23 decreasing with age (Holmlund-Suila et al., 2016; Holmlund-Suila et al., 2017; Enlund-Cerullo et al., 2020). Furthermore, in a cohort of 183 school-aged children and adolescents, we have previously observed that common variants of FGF23 (SNP1, SNP2, and rs3832879) show associations with PTH concentrations and urinary phosphate excretion, as well as with total hip bone mineral density (BMD) Z-score (Pekkinen et al., 2015), thereby linking FGF23 variants to bone mass.
It remains unknown whether genetic variation in FGF23 influences phosphate and vitamin D metabolism and bone strength during infancy. In this longitudinal cohort study, we examined associations of three FGF23 polymorphisms (SNP1–3), with FGF23, phosphate, and vitamin D concentrations and with bone strength, determined by peripheral quantitative computed tomography (pQCT), from age 12–24 months in 622 healthy infants participating in a vitamin D intervention trial.
2 Methods
2.1 Participants
This study is part of the randomized and controlled vitamin D intervention in infant (VIDI) trial, which was carried out at the large, tertiary Kätilöopisto Maternity Hospital in Helsinki, Finland, from January 2013 to June 2016. We have previously reported the detailed protocol and exclusion criteria of VIDI (Helve et al., 2017; Rosendahl et al., 2018). In short, 975 healthy term infants, born to mothers of Northern European origin and with birthweight appropriate for gestational age, were randomized to receive vitamin D3 supplementation of either 10 μg (400 IU) (Group10) or 30 μg (1200 IU) (Group30) per day, from 2 weeks to 2 years of age.
2.2 Clinical and pQCT data collection
Data regarding the birth of the participating infants were obtained from medical records at recruitment. Cord blood samples were collected at birth and stored at −20°C for later extraction of genomic DNA. Study visits at 12 and 24 months included measurements of growth, venous blood samples, and bone strength, using peripheral quantitative computed tomography (pQCT) (Stratec XCT 2000 L Research+, Stratec Medizintechnik GmbH, Pforzheim, Germany) (Rosendahl et al., 2018; Valkama et al., 2020). Growth parameters were transformed into corresponding standard deviation scores (SDS) using Finnish pediatric growth references (Saari et al., 2011). FGF23, 25-hydroxyvitamin D (25-OHD), PTH, phosphate (Pi), ionized calcium (Ca ion), and iron concentrations were determined from the samples obtained at 12 and 24 months, stored at −80°C until analysis. The detailed study protocol and follow-up have previously been described (Helve et al., 2017; Rosendahl et al., 2018).
PQCT measurements were taken at 20% distal length of the left tibia. total BMC. Total vBMD, total CSA, and polar moment of inertia were determined. The image quality of the pQCT scans was graded based on the presence of motion artifacts (I = excellent, II = good, III = moderate, IV = sufficient, or V = poor), as previously reported (Rosendahl et al., 2018; Valkama et al., 2020). Scans with major motion artifacts or incorrect positioning of the examined limb were excluded.
Of 975 participants in VIDI, a total of 622 subjects with available genotyping data for one or more of the three studied SNPs of FGF23 (rs7955866, rs11063112, and rs13312770) were included in the study. We have previously reported FGF23 concentrations and bone strength parameters in VIDI participants at 12 and 24 months, which were here included in analyses of association with FGF23 genetic variation.
2.3 Genotype analysis
Genomic DNA from cord blood samples was extracted following the manufacturers’ instructions, using either automated Chemagen MSM1 extraction (PerkinElmer Inc., Chemagen Technologie GmbH, Baesweiler, Germany) or the Gentra Puregene kit (Qiagen GmgH, Hilden, Germany), at the laboratory of the Finnish Institute for Health and Welfare.
Based on previously demonstrated associations with phosphate and bone metabolism, FGF23 SNPs rs7955866 (c.716C>T, p.239T > M) (SNP1) and rs11063112 (c.2185A>T) (SNP2) were chosen for the study (Rendina et al., 2012; Pekkinen et al., 2015). A third SNP, rs13312770 (c.211 + 3287A>G) (SNP3), was selected from the International HapMap project database (International HapMap Consortium, 2005) (http://www.ncbi.nlm.nih.gov/variation/tools/1000genomes/) using HaploView 4.2 software (Broad Institute, Cambridge, MA, United States; (http://www.broad.mit.edu/mpg/haploview) by tagging the SNP approach, using CEU population as a reference. The studied three tag SNPs (SNP1–3) also capture the variation in three other FGF23 SNPs; rs13312789, rs13312786, and rs13312756. The localization of the selected SNPs in FGF23 is shown in Figure 1.
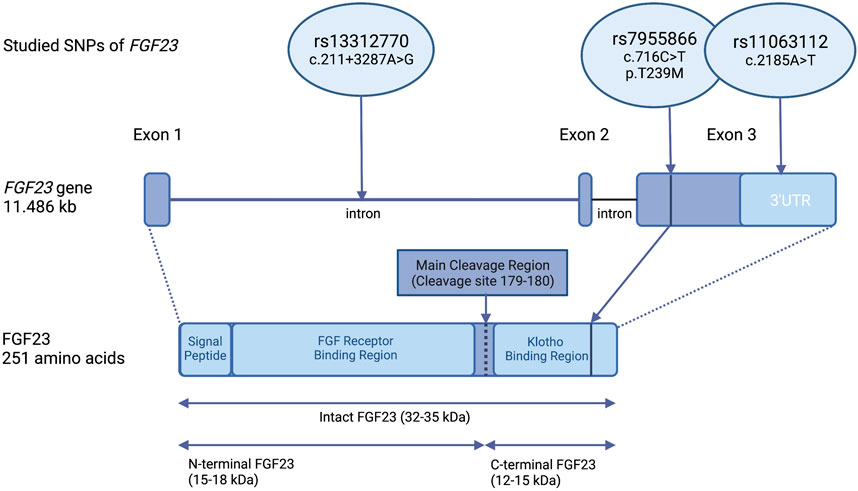
FIGURE 1. Location of the studied SNPs within the FGF23 gene and protein. Organization of the FGF23 gene and location of the studied variants; rs7955866 (SNP1), rs11063112 (SNP2), and rs13312770 (SNP3). Rs7955866 (c.716C>T), is a missense variant, located in the third exon of FGF23, which causes an amino acid change from threonine to methionine. The other two variants are located in non-translated regions of FGF23. Rs13312770 (c.211 + 3287A>G) is intronic, while rs110063112 (c.2185A>T) is located in the 3′- untranslated region (3′UTR) of FGF23. Based on the amino acid position, the variant rs7955866 is located in the Klotho binding region of FGF23 (position marked with a vertical line). Created with QmlvcmVuZGVyLmNvbQ==.
The samples were genotyped by SNP-specific TaqMan Assays (Thermo-Fisher, Waltham, MA, United States) (TaqMan Assay IDs: C__25605491_20, C___2768724_10, and C__34067840_10 for SNP1, SNP2, and SNP3, respectively) and qPCR Bio-Rad CFX384 C1000 Touch™ Real-Time PCR Detection System (Bio-Rad, Hercules, CA, United States), in accordance with the manufacturer’s instructions. The amplification protocol used was 95°C for 3 min, followed by 39 cycles of 15 s at 92°C and 1 min at 60°C. End-point protocol analysis performed using CFX Manager 3.1 (Bio-Rad, Hercules, CA, United States) software was used to determine genotyping results. The obtained results were validated by simultaneous analysis of previously genotyped controls as well as randomly chosen internal duplicates and negative controls.
The obtained genotypes of the three studied SNPs were also combined into haplotypes using HaploView 4.2 software. In comparative analyses, identified haplotype homozygotes were used.
2.4 Biochemical analyses
Concentrations of biochemical parameters were determined from the samples obtained at 12 and 24 months of age.
FGF23, 25-OHD, and PTH concentrations were analyzed in the laboratories of the Pediatric Research Center at the University of Helsinki. Intact and C-terminal FGF23 concentrations were analyzed from venous blood sample plasma aliquots using commercially available enzyme-linked immunosorbent assay (ELISA) kits by KAINOS Laboratories (Tokyo, Japan) and Biomedica Medizinprodukte GmbH and Co. KG (Vienna, Austria) for determining iFGF23 and cFGF23 concentrations, respectively. Randomly chosen samples showed a mean CV% of 2% vs. 5% for iFGF23 and 7% vs. 3% for cFGF23 in duplicate analysis, at 12 vs. 24 months, respectively.
Concentrations of 25-OHD and PTH were analyzed by the fully automated IDS-iSYS immunoassay with chemiluminescence detection (Immunodiagnostic Systems Ltd., Bolton, United Kingdom), as previously reported (Rosendahl et al., 2018). The accuracy and quality of the methodology used were assessed by the vitamin D External Quality Assessment Scheme (DEQAS, Charing Cross Hospital, London, United Kingdom).
Analyses of Ca ion, Pi, and iron concentrations were performed by standard methods, at the accredited Central Laboratory of Helsinki University Hospital (HUSLAB).
2.5 Statistics
The results are presented as medians and interquartile ranges (IQRs) or means and 95% confidence intervals (95% CI). Normal distribution of variables was visually confirmed. Non-normally distributed variables were logarithmically converted (Ln) to enable parametric analyses. Differences between the sexes and intervention groups were examined by independent samples t-test or Pearson chi-squared test for categorical variables.
Differences between means of biochemical or bone parameters and genotypes of the studied SNPs were examined by analysis of variance (ANOVA) or Welch test of equality of means when variances were not equal. Multiple comparisons tests were performed using Bonferroni or Tamhane corrections. Mean allelic effects of the genotype were evaluated using multivariate linear regression. The temporal change in the studied biochemical and bone parameters and their associations with the genotype of the studied SNPs were examined using linear mixed models for repeated measurements. Covariates for linear regression and mixed-model analyses were selected based on previously reported associations with the studied parameters (Pekkinen et al., 2015; Holmlund-Suila et al., 2017; Rosendahl et al., 2018; Enlund-Cerullo et al., 2020; Valkama et al., 2020), and significant covariates were included in the models. For linear mixed-model analyses, covariates of continuous parameters included both 12- and 24-month measurements. All analyses were primarily performed including all participants, followed by the sex and intervention group. An overview of the performed analyses is presented in Supplementary Figure S1. IBM SPSS Statistics 24 (IBM, Armonk, NY, United States) software was used for statistical analyses. A p-value of <0.05 was considered statistically significant.
2.6 Study approval
The VIDI trial was approved by the Research Ethics Committee of the Hospital District of Helsinki and Uusimaa (107/13/03/03/2012) and carried out following the principles of the Helsinki Declaration. VIDI is registered in ClinicalTrials.gov (NCT01723852). Written informed consent was provided by the parents of participating infants at recruitment.
3 Results
3.1 Participant characteristics, biochemical findings, and bone parameters
This study included a total of 622 participants, with available genotyping data on three studied FGF23 SNPs (64% of the original VIDI cohort; 53.7% girls). Key anthropometric, biochemical, and bone parameters of the participants at 12 and 24 months are reported by the sex and intervention group as given in Tables 1, 2, respectively. Boys were taller and heavier (p < 0.005 for both) and had lower iFGF23 (p < 0.001) and Ca ion (p < 0.002) than girls at both time points. PTH and iron concentrations were higher in girls at 24 months (p < 0.015). Due to the original recruitment schedule, most study visits were conducted during spring (Table 1). The levels of 25-OHD and PTH differed between intervention groups, with higher 25-OHD and correspondingly lower PTH concentrations in participants receiving high-dose vitamin D supplementation (Group30). Iron level was higher in Group30 at 12 months (p = 0.013) (Table 2).
Bone characteristics, determined by pQCT of the tibia, indicated that the total bone mineral content (total BMC) was lower in girls than in boys at both studied time points (p < 0.001 for both), and total cross-sectional area of the bone (total CSA) and polar moment of inertia were lower in girls at 24 months (p < 0.005) (Table 1). Bone parameters did not differ between intervention groups (Table 2).
3.2 Genotyping results and genotype distributions
Genomic DNA was extracted from cord blood samples, and genotypes were determined for three previously characterized variants of FGF23, using SNP-specific TaqMan Assays. Genotype call rates varied between 86% and 93%. The genotype for all three studied SNPs was determined for 526 (84.6%) participants. Obtained genotype distributions were in Hardy–Weinberg equilibrium, and in line with available previously reported genotype data (Zerbino et al., 2018; Lek et al., 2016; S isuproject, 2018). The studied three SNPs, rs7955866, rs11063112, and rs13312770 (SNP1, SNP2, and SNP3, respectively) were in linkage disequilibrium (D’ = 1, r2 = 0.02). Four different combinations of haplotype homozygotes for SNP1–3 were observed; GTT (major allele homozygotes); GAT (major–minor–major homozygotes); GTC (major–major–minor homozygotes); and AAT (minor–minor–major allele homozygotes). Our study population did not include any subjects homozygous for all minor alleles. Genotype and haplotype distributions, presented in Table 3, did not differ between the sexes or intervention groups.
3.3 Associations of genotype and biochemical and bone parameters
Mean unadjusted concentrations by genotype and haplotype and results for analyses of differences between variants are presented in Figures 2, 3 and Tables 4, 5. The adjusted mean allelic effects of the studied genotypes on biochemical and bone parameters at 12 and 24 months are presented in Table 6.
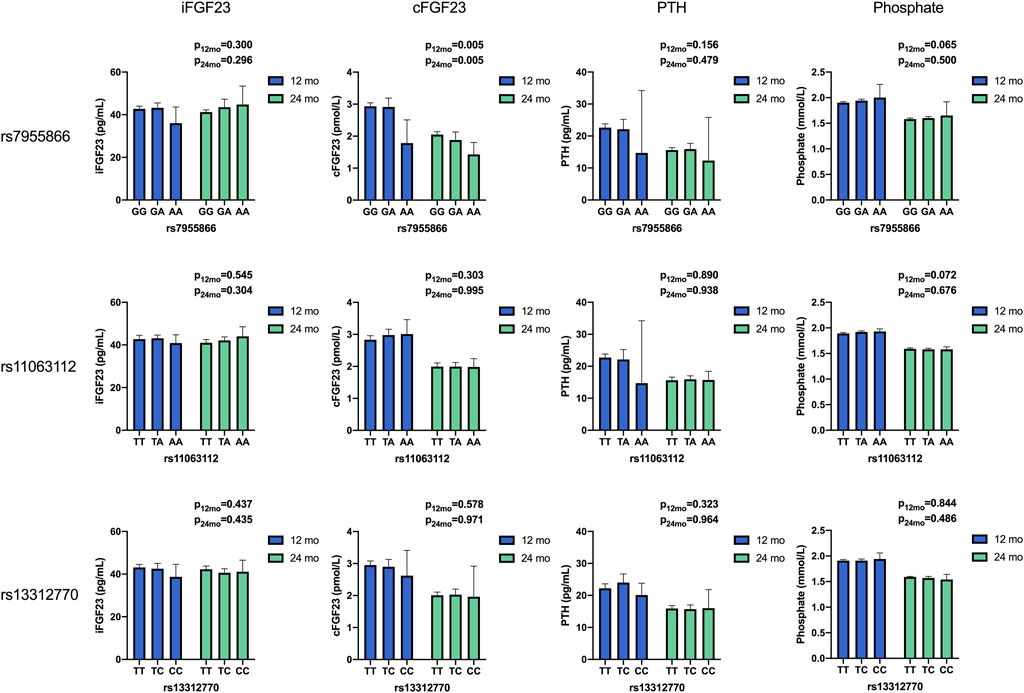
FIGURE 2. Unadjusted mean concentrations of biochemical parameters by the genotype of studied SNPs at 12 and 24 months. Results for differences between variants in ANOVA. (iFGF23, intact fibroblast growth factor 23; cFGF23, C-terminal fibroblast growth Factor 23; PTH, parathyroid hormone).
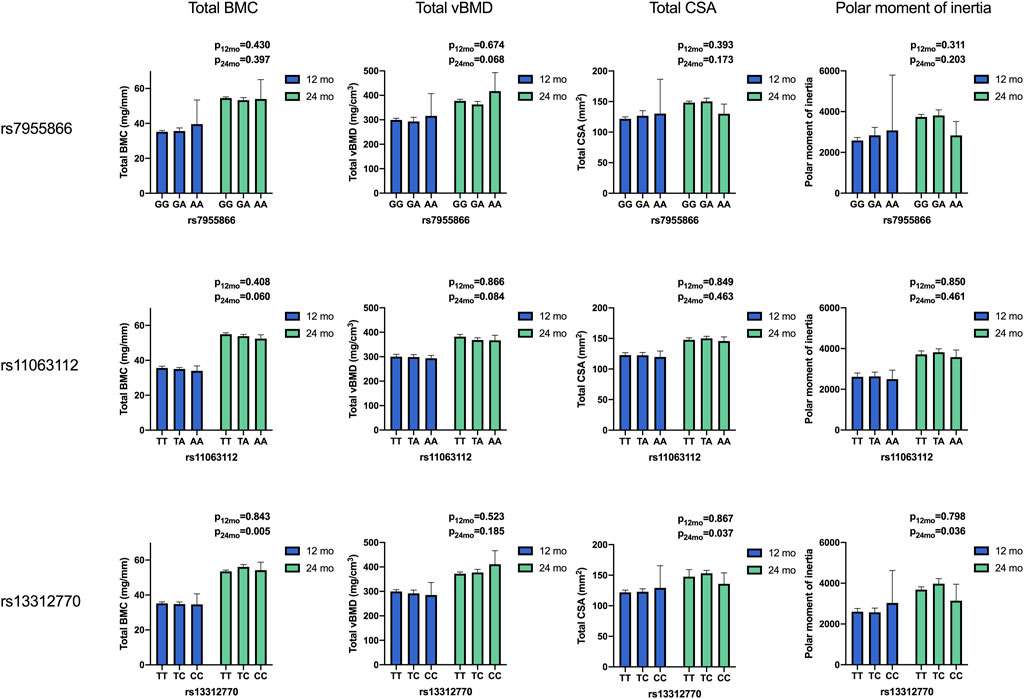
FIGURE 3. Means of bone parameters by the genotype of studied SNPs at 12 and 24 months. Results for differences between variants in ANOVA. (BMC, bone mineral content; vBMD, volumetric bone mineral density; CSA, cross-sectional area of the bone).
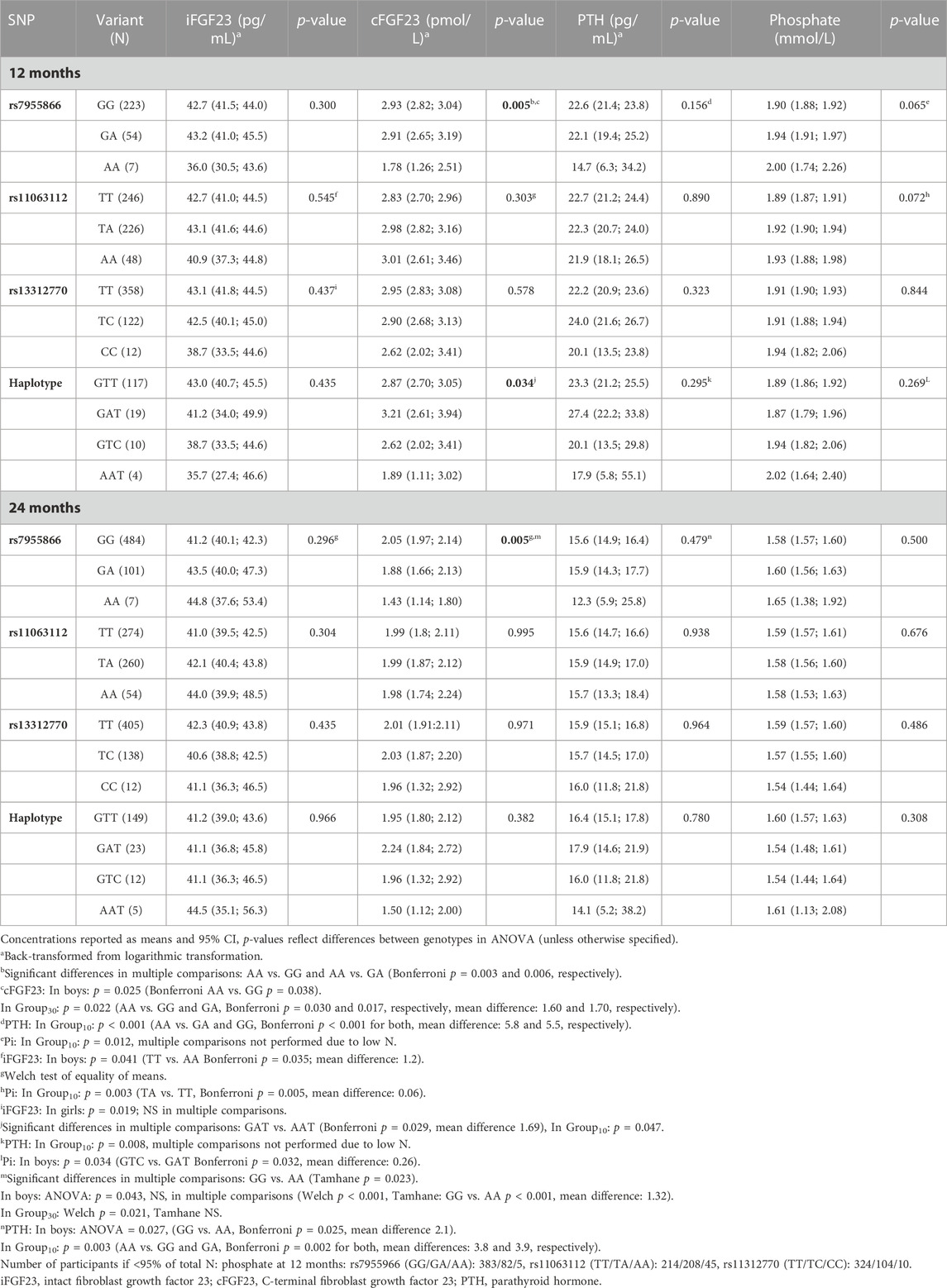
TABLE 4. Biochemical variables by the genotype at 12 and 24 months and difference between variants in ANOVA.
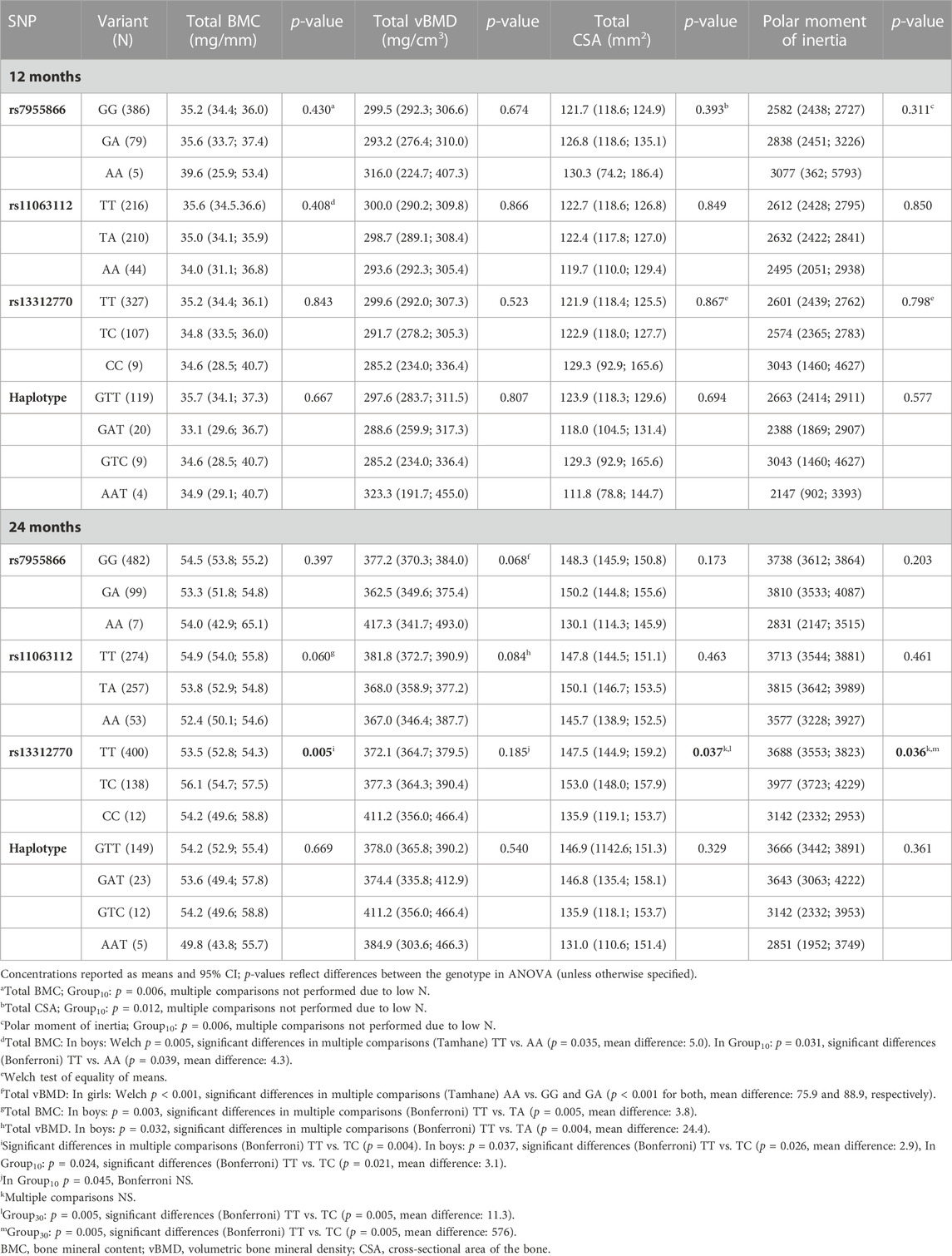
TABLE 5. Bone parameters by the genotype at 12 and 24 months and difference between variants in ANOVA.
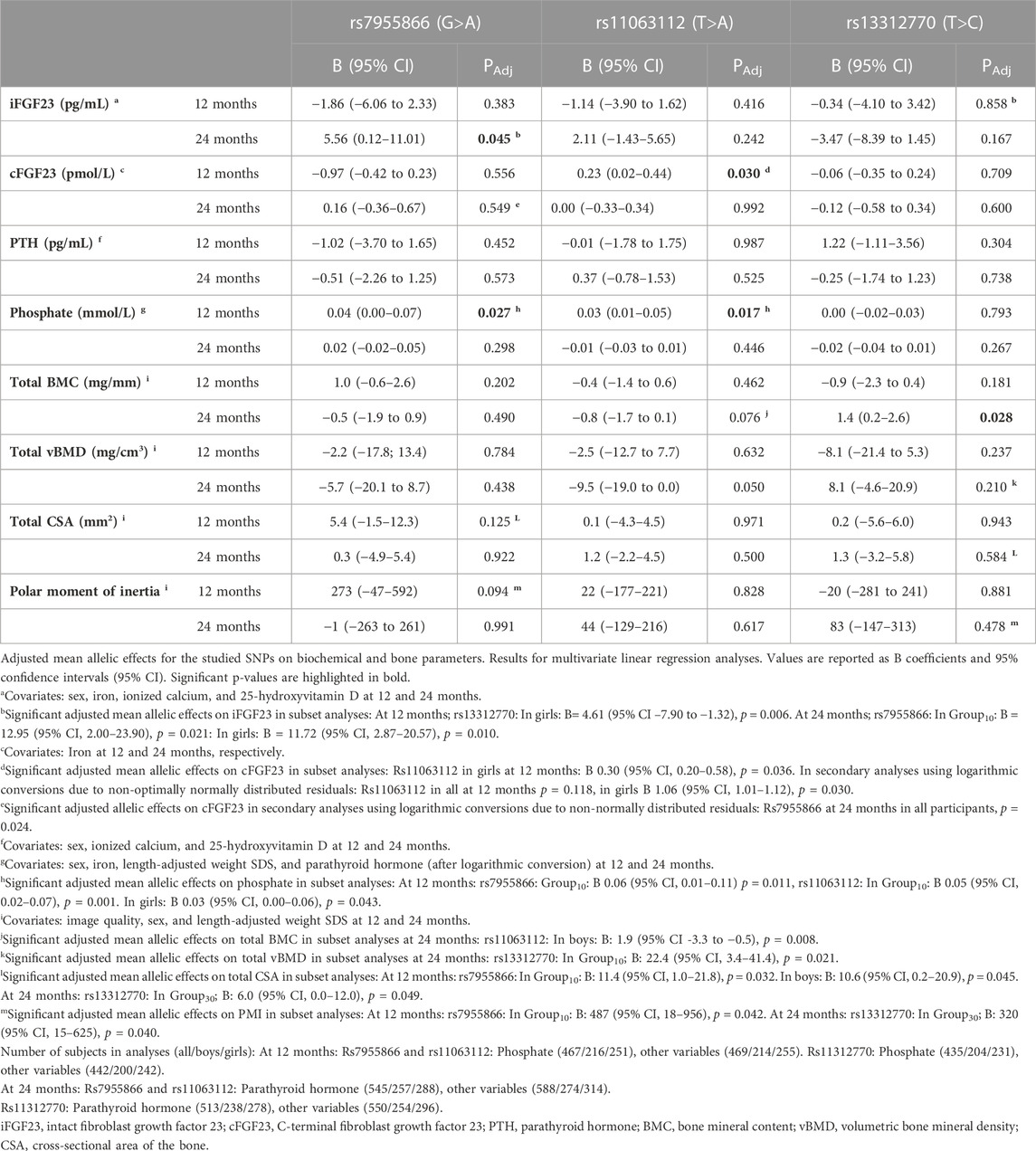
TABLE 6. Adjusted mean allelic effects of studied variants on biochemical and bone parameters at 12 and 24 months.
3.3.1 Biochemical parameters
Concentrations of 25-OHD modified iFGF23 and PTH in linear regression but did not affect other studied parameters. The genotype of the studied three SNPs did not affect 25-OHD, Ca ion, or iron concentrations (data not shown).
3.3.1.1 Associations with SNP1
The SNP1 genotype was associated with unadjusted cFGF23 in all participants and at both time points. SNP1 minor allele homozygotes (AA) had lower cFGF23 concentrations than major allele homozygotes (GG) at 12 and 24 months (p = 0.005 for both), and a similar association was observed for the corresponding haplotypes (GAT vs. AAT), significant at 12 months (p = 0.034) (Table 4). Genotype effects of SNP1 on the concentration of unadjusted cFGF23 were significant, also separately in boys and in Group30 at 12 and 24 months (p < 0.044 for all comparisons) (Table 4).
When examined by multivariate linear regression, using sex, iron, ionized calcium, and 25-OHD, as covariates, the adjusted mean allelic effects of SNP1 genotype in all participants were significant for iFGF23 at 24 months (B = 5.56; p = 0.045), and separately in Group10 (B = 12.95; p = 0.021) and in girls (B = 11.72; p = 0.010), with a shift from major (G) to minor (A) alleles, thus increasing iFGF23 (Table 6). As the residuals of cFGF23 did not show normal distribution when analyzed by the SNP1 genotype, secondary analyses were performed using logarithmic conversions of cFGF23 concentrations. The adjusted mean allelic effects for cFGF23 were significant in all participants at 24 months (p = 0.024), with a decrease in cFGF23 with the shift from major to minor SNP1 alleles.
In Group10, minor allele homozygotes of SNP1 had highest unadjusted phosphate concentration (p = 0.012) at 12 months and lowest unadjusted PTH at both time points (p < 0.004 for both). Unadjusted PTH was also lowest in boys with minor allele homozygotes at 24 months (p = 0.027) (Table 4). The adjusted mean allelic effects on phosphate were significant at 12 months in all participants (B = 0.04; p = 0.027) and in Group10 (B = 0.06; p = 0.011). Minor alleles were associated with increasing phosphate concentrations (Table 6).
3.3.1.2 Associations with SNP2
Adjusted mean allelic effects of SNP2 on cFGF23 at 12 months were significant in all participants (B = 0.23; p = 0.030) and separately in girls (B = 0.30; p = 0.036), but not in boys. Association with phosphate at 12 months was also evident in all participants (B = 0.03; p = 0.017) and separately in girls (B = 0.03; p = 0.043), as well as in Group10 (B = 0.05; p = 0.001). In Group10, unadjusted phosphate at 12 months was also related to the genotype of SNP2 (p = 0.003), with heterozygotes (TA) having higher phosphate concentrations than major homozygotes (TT) (Table 6).
3.3.1.3 Associations with SNP3
In multivariate linear regression, the SNP3 genotype modified iFGF23 (B = −4.61, p = 0.006) in girls at 12 months, with a decrease in iFGF23 observed with the shift from major (T) to minor (C) alleles (Table 6).
3.3.1.4 Associations with haplotypes
Unadjusted haplotype analyses mirrored the differences found in SNP analyses, with differences in PTH concentrations observed in Group10 (p = 0.008) and those in phosphate observed in boys (p = 0.034) at 12 months (Table 4).
3.3.2 Bone parameters
The genotype of SNP1 and SNP2 did not affect unadjusted means or have significant adjusted mean allelic effects on pQCT-derived bone parameters when analyzed in all participants at 12 or 24 months.
3.3.2.1 Associations with SNP1
In Group10, the SNP1 genotype modified total BMC (p = 0.006), total CSA (p = 0.012), and PMI (p = 0.006) at 12 months, with minor homozygotes showing highest unadjusted means (Table 5). In multivariate linear regression, the SNP1 genotype correspondingly modified total CSA and PMI in Group10 (B = 11.4; p = 0.032 and B = 487; p = 0.042) and total CSA in boys (B = 10.6; p = 0.045) at 12 months, with an increase in means related to the shift from major to minor alleles (Table 6). In girls, minor allele homozygosity of SNP1 was also related to highest total vBMD (p < 0.001) at 24 months (Table 5).
3.3.2.2 Associations with SNP2
In subset analyses, the SNP2 genotype was associated with total BMC; minor allele homozygotes had lower means than major homozygotes in Group10 (p = 0.031) at 12 months, and in boys at both 12 and 24 months (p < 0.006 for both) (Table 5). In linear regression, a shift from major to minor alleles of SNP2 correspondingly decreased the total BMC in boys at 24 months (B-1.9; p = 0.008) (Table 6). At 24 months, major allele homozygosity of SNP2 was also related to highest unadjusted total vBMD in boys (p = 0.032) (Table 5).
3.3.2.3 Associations with SNP3
When studied in all participants, the genotype of SNP3 was significantly associated with total BMC (p = 0.005), total CSA (p = 0.037), and PMI (p = 0.036) at 24 months, with highest unadjusted means observed in heterozygotes (TC). In subset analyses, these associations were significant for total BMC in boys and in Group10 (p = 0.037 and p = 0.024, respectively) and for total CSA and PMI in Group30 (p = 0.005 for both) (Table 5). In all participants, significant adjusted mean allelic effects on bone parameters were similarly observed for SNP3, with a shift from major to minor alleles increasing the total BMC at 24 months (B = 1.4; p = 0.028). In Group10, SNP3 minor allele homozygotes had the highest unadjusted total vBMD (p = 0.045) (Table 5), and a shift from major to minor alleles of SNP3 increased total vBMD (B = 22.4; p = 0.021) at 24 months (Table 6). In Group30, minor allele carriers also had greater total CSA and PMI (B = 6.0; p = 0.049 and B = 320; p = 0.040, respectively) at 24 months (Table 6).
3.3.2.4 Associations with haplotype
In haplotype analyses, we did not observe effects on unadjusted bone parameters.
3.4 Genotype and temporal change in biochemical and bone parameters
3.4.1 Temporal change in biochemical parameters
Adjusted mean concentrations of biochemical parameters and results for linear mixed-model analyses are presented in Table 7 and Figure 4, respectively
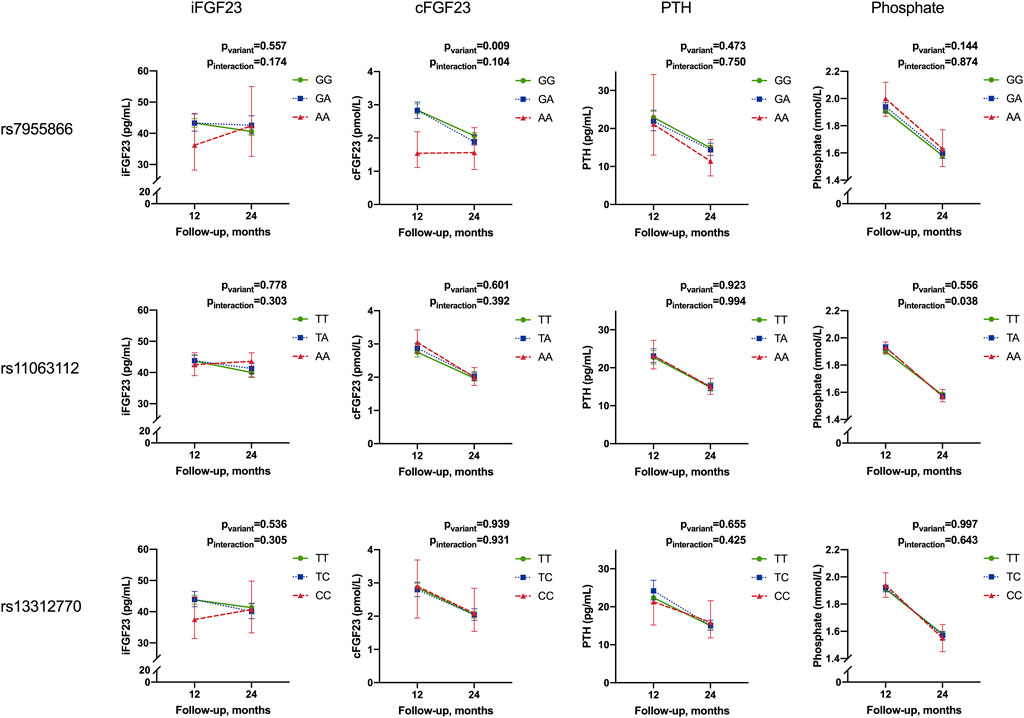
FIGURE 4. Temporal change in mean concentrations of intact FGF23, C-terminal FGF23, parathyroid hormone, and phosphate from 12 to 24 months by the genotype of studied SNPs. Results for linear mixed-model analyses of repeated measurements for differences in adjusted means (pvariant) and differences in temporal change between variants (i.e., interaction of the variant and temporal change (Pinteraction). Results are given as adjusted mean concentrations and 95% confidence intervals (95% CI). Means are adjusted for season, sex, and interaction of time and season; 25-hydroxyvitamin D and ionized calcium for iFGF23 and PTH; iron for iFGF23; cFGF23 and phosphate and length-adjusted weight standard deviation score for phosphate (iFGF23, intact fibroblast growth factor 23; cFGF23, C-terminal fibroblast growth factor 23; PTH, parathyroid hormone).
Concentrations of iFGF23, cFGF23, PTH, and phosphate decreased from 12 to 24 months in the study participants. Concentrations varied by season; iFGF23, cFGF23, and phosphate were highest in winter (p = 0.024, 0.036, and 0.037, respectively), while 25-OHD was lowest and PTH highest in spring (p = 0.010 and 0.002, respectively) (data not shown). The studied SNPs did not affect concentrations or temporal change of 25-OHD, Ca ion, or iron (data not shown).
In the whole cohort, genotype effects in linear mixed-model analyses were significant only for SNP1 and cFGF23 concentrations (p = 0.009), with lowest concentrations linked to minor allele homozygosity. However, although not relating with adjusted cross-sectional concentrations, the SNP2 genotype was found to be associated with a change in phosphate from 12 to 24 months, with a greatest decrease observed in minor allele homozygotes (pinteraction = 0.038) (Table 7; Figure 4).
In subset analyses, the SNP1 genotype was also associated with iFGF23 concentrations, but not a temporal change, in girls (pvariant = 0.041), with heterozygotes showing the greatest adjusted mean concentrations. In boys, minor allele homozygosity of SNP3 was related to greatest decrease in phosphate concentrations (pinteraction = 0.022) (Table 7).
The haplotype did not modify the temporal changes in iFGF23, cFGF23, PTH, or phosphate.
3.4.2 Temporal change in bone parameters
Adjusted mean concentrations of bone parameters and results for linear mixed-model analyses are presented in Table 8 and Figure 5. The means of bone parameters increased with age during follow-up. Total BMC, total CSA, and PMI were lowest in spring (p < 0.009), while total vBMD was the lowest in winter (p = 0.049) (data not shown).
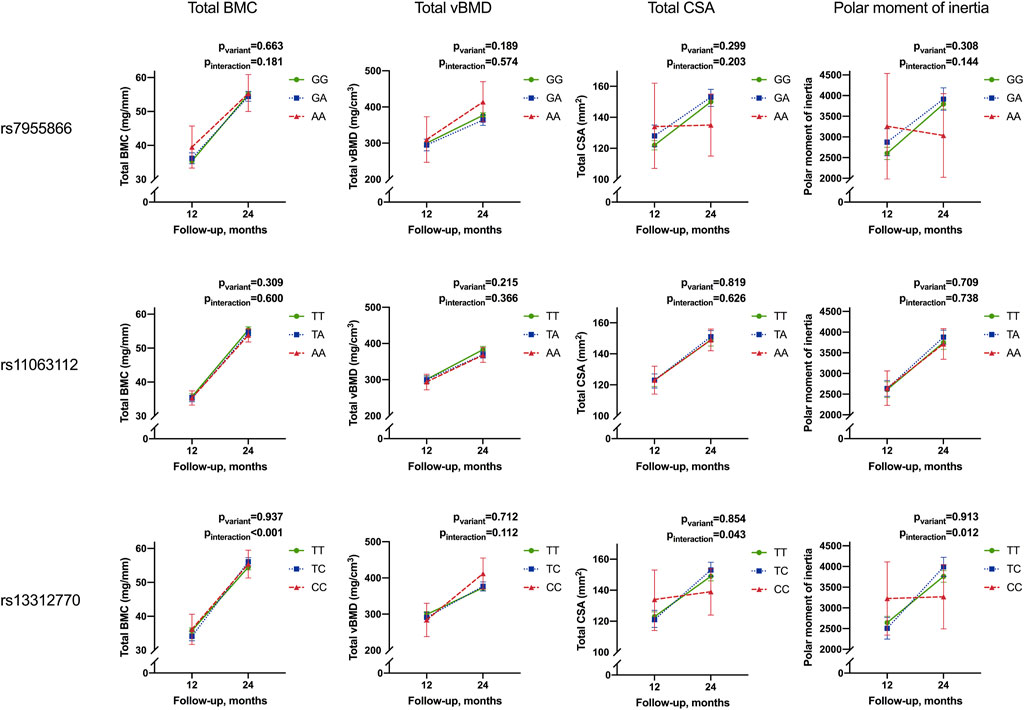
FIGURE 5. Temporal change in mean Total BMC, total vBMD, total CSA, and PMI from 12 to 24 months by the genotype of studied SNPs. Results for linear mixed-model analyses of repeated measurements for differences in adjusted means (pvariant) and differences in the temporal change between variants (i.e., interaction of the variant and temporal change (Pinteraction). Results are given as adjusted means and 95% confidence intervals (95% CI). Means are adjusted for season at follow-up and length-adjusted weight standard deviation score for all bone parameters and sex for total BMC, total CSA, and PMI (BMC, bone mineral content; vBMD, volumetric bone mineral density; CSA, cross-sectional area of the bone).
The genotype of SNP1 or SNP2 did not modify the adjusted means or the increase in bone parameters in all participants. When stratified by the intervention group, the effects of SNP1 on total BMC, total CSA, and PMI were significant in Group10 (pvariant = 0.046, 0.021, and 0.024, respectively). Minor allele homozygotes had the greatest adjusted means of total BMC, total CSA, and PMI and the smallest increase in total CSA and PMI (pinteraction = 0.037 and 0.020, respectively) during follow-up (Table 8).
In boys, SNP2 major allele homozygotes had the greatest mean total BMC and vBMD (pvariant = 0.018 and 0.015, respectively) (Table 8).
SNP3 significantly modified the temporal change in total BMC, total CSA, and PMI in all participants (pinteraction <0.001, 0.043, and 0.012, respectively). Minor allele homozygotes had the smallest increase in total CSA and PMI, while heterozygotes showed the greatest increase in total BMC (Table 8; Figure 5).
The observed effect of the SNP3 genotype on increase in total BMC was also significant in Group10, and in girls and boys separately (pinteraction = 0.002, 0.012, and 0.029, respectively). The effect on total CSA and PMI was also significant separately in Group30 (pinteraction = 0.019 and 0.005, respectively) and in boys for the PMI (pinteraction = 0.034) (Table 8).
The haplotype effects on the temporal change in bone parameters were not significant.
4 Discussion
This study finds that, in healthy young children, common FGF23 SNPs rs7955866 (SNP1), rs11063112 (SNP2), and rs13312770 (SNP3) are associated with circulating FGF23 and phosphate concentrations and with pQCT-derived bone strength parameters, as well as with change in bone strength from 12 to 24 months. In all participants, SNP1 minor allele homozygosity was related to lower cFGF23 concentrations at both 12 and 24 months of age. SNP3 minor allele carriers had greater total BMC and showed greater increase in total BMC, but smaller increase in total CSA and PMI, from 12 to 24 months. In addition, the SNP2 genotype was associated with change in phosphate from 12 to 24 months, with the greatest decrease in concentrations observed in minor allele carriers. Further genotype associations were observed only in participants receiving standard vitamin D supplementation, although vitamin D intervention did not affect the studied bone strength or biochemical parameters, apart from 25-OHD and PTH. Concentrations of 25-OHD were not modified by the studied FGF23 SNP genotypes.
Previous GWAS studies in adults have found SNPs near FGF23, SLC34A1, and CASR to relate to differences in phosphate concentrations (Kestenbaum et al., 2010). While direct associations of FGF23 SNP2 or SNP3 with phosphate metabolism have not previously been described, minor allele homozygosity of SNP1 is related to lower phosphate concentrations in adult nephrolithiasis patients and a smaller cohort of school-aged children (Rendina et al., 2012; Pekkinen et al., 2015). SNPs linked to genes involved in vitamin D metabolism; cleavage and inactivation of FGF23 (PCSK9); and cell proliferation, inflammation, and apoptosis (HLA-DQA1, HLA-DQB1, and TGFB2, respectively) have, in turn, are associated with circulating FGF23 concentrations (White et al., 2001; Benet-Pagès et al., 2004; Robinson-Cohen et al., 2018; Chuang et al., 2020). Apart from confirming the associations of FGF23 genetic variation with phosphate metabolism in the studied age group, our study is, to our knowledge, the first to show a direct association between variation in the FGF23 gene and FGF23 concentrations.
In all participants, minor alleles were related to lower cFGF23 throughout follow-up (SNP1) and positive allelic effects on phosphate at 12 months of age (SNP1-2), but the greatest decrease in phosphate was observed from 12 to 24 months (SNP2 in all, SNP3 in boys). In analyses by sex, FGF23 minor allele carriers showed lowest iFGF23 in boys (SNP2) and negative allelic effects in girls (SNP3). The FGF23 genotype also notably affected bone strength and age-dependent change thereof. Minor alleles related to greater increase of total BMC, but smaller increase of total CSA and PMI, compared with major allele homozygotes, with heterozygotes having highest total BMC, CSA, and PMI at 24 months (SNP3). In contrast to SNP3, in boys, minor allele homozygosity of SNP2 was observed to be associated with lower total BMC at both 12 and 24 months, and lower total vBMD at 24 months.
The genotype-related differences in phosphate concentrations at 12 months differ from those previously reported in adult nephrolithiasis patients and a smaller cohort of school-aged children (Rendina et al., 2012; Pekkinen et al., 2015), with minor allele homozygosity relating to higher, instead of lower, phosphate concentration. We have previously found phosphate concentrations to markedly decrease from 12 to 24 months of age (Koljonen et al., 2021). In light of the age-related decrease in phosphate, which is greater in FGF23 minor allele carriers (SNP2-3), the different direction of genotype associations observed at 12 vs. 24 months of age, and the simultaneously decreasing FGF23 and PTH concentrations, the differing findings could be explained by age-specific regulation.
Differences in the direction of genotype associations at 12 and 24 months are similarly observed for several bone parameters. Significant differences in temporal change, but not in cross-sectional adjusted means, as observed, for example., for SNP3 and total BMC, CSA, and PMI, are indicative of differences in genotype effects at the two studied time points. Growth velocity in infancy is greater in boys and decreases after 12 months of age (Kiviranta et al., 2016), while physical activity increases, including standing and onset of walking, that previously have been shown to be associated with bone strength in early childhood (Ireland et al., 2014). Observed differences between the sexes possibly reflect differences in growth velocity, as well as differences in FGF23 regulation previously observed between girls and boys in the studied age group (Holmlund-Suila et al., 2017; Enlund-Cerullo et al., 2020). We hypothesize that the differences in genotype effects, observed both for studied biochemical and bone parameters, potentially relate to age-specific differences in regulation, as well as to differences in growth phase and physical activity at 12 and 24 months.
The observed associations of the FGF23 genotype and cFGF23 concentrations in all participants could potentially indicate reduced expression of iFGF23, with correspondingly higher phosphate concentration as observed at 12 months, in minor allele carriers (SNP1), potentially related to altered binding to the FGF receptor and Klotho complex (Yamazaki et al., 2008; Rendina et al., 2012). The reduced expression of iFGF23 could be balanced by a decrease in cleavage, resulting in non-significant differences in iFGF23 by the genotype, but subsequently observed as a decrease in cFGF23 in minor allele carriers. Findings of minor allele genotype associations with low iFGF23 at 12 months observed in analyses by sex potentially support this hypothesis.
In limited adult studies, iFGF23 concentrations have been found to be negatively associated with BMD (Bouksila et al., 2019; Wang et al., 2020). Apart from its effects on phosphate metabolism, in vitro studies have suggested FGF23 expression to independently regulate bone formation (Wang et al., 2008). Inverse association of iFGF23 with BMD parameters has also recently been observed in a Mendelian randomization study exploring causal effects of FGF23 (Yokomoto-Umakoshi et al., 2021). As our study setting does not allow us to establish causality, the mechanisms underlying the observed differences between FGF23 genotype and bone strength and temporal change, thereof, require further studies. Based on earlier reports (Bouksila et al., 2019; Wang et al., 2020; Yokomoto-Umakoshi et al., 2021), the observed genotype effects on bone parameters could potentially correspond with effects on iFGF23 expression and concentrations. Our previous study in a smaller cohort of school-aged children observed associations of FGF23 diplotype and total hip BMD Z-scores (Pekkinen et al., 2015). The present study finds variation in FGF23 in infants to be primarily associated with differences in total BMC, total CSA, and PMI, i.e., measures of bone size, mass, and resistance to torsion, rather than with volumetric bone mineral density (total vBMD), for which associations are observed only in Group10 (SNP3).
Considering the tight interplay between vitamin D, phosphate, and bone metabolism, our study uniquely explores the effects of genetic variation in FGF23 in a vitamin D intervention setting. In our largely vitamin D sufficient study population, vitamin D intervention affected 25-OHD and PTH levels, as previously reported, but did not directly modify other biochemical or bone parameters (Rosendahl et al., 2018; Valkama et al., 2020). Some of our findings were, however, specific for participants receiving standard vitamin D supplementation (Group10). In contrast to our earlier study in school-aged children, FGF23 genotype did not modify 25-OHD, but in Group10, minor alleles associated with lower PTH (SNP1), a finding in line with our previous report (Pekkinen et al., 2015). As vitamin D intervention had a significant effect on PTH and 25-OHD concentration, it is plausible that the impact of higher vitamin D supplementation in Group30 did not allow detection of possible smaller effects of the SNP1 genotype on PTH and 25-OHD concentrations. We have previously found 25-OHD to modify iFGF23 in girls, while seasonal differences, possibly reflecting changes in vitamin D status, are associated with iFGF23 concentrations in boys (Enlund-Cerullo et al., 2020). In the present study, 25-OHD modified adjusted iFGF23 concentrations by the SNP1 genotype. Indirect effects of 25-OHD are possibly also reflected in the seasonal differences observed in bone parameters and iFGF23 and cFGF23 concentrations.
It is interesting to note that we observe positive effects on bone strength parameters for SNP1 only in participants receiving standard, but not high-dose, vitamin D supplementation. In Group10, minor allele homozygosity was related to higher total BMC, CSA, and PMI at 12 months and higher total BMC but lower CSA at 24 months, and smaller increase of total CSA and PMI from 12 to 24 months (SNP1), largely mirroring the findings in all participants (SNP3). As effects of SNP1 include potential differences in binding of FGF23 to FGF receptors and Klotho (Yamazaki et al., 2008; Rendina et al., 2012), it can be speculated that interaction with vitamin D and its metabolites is modified in minor allele carriers, possibly depending on 25-OHD and vitamin D supplementation dose. Further studies are needed to explore the interplay of vitamin D, FGF23, and genetic variation in FGF23 with bone strength and modeling in young children.
When considering observed associations between singular SNPs and phenotype, it is to be noted that associations may, in part, be due to linkage disequilibrium between studied and other genetic variants. In our study, potential differences in expression and post-translational modification of FGF23 through altered binding to the FGF receptor and Klotho and consequent differences in signaling (SNP1) (Yamazaki et al., 2008; Rendina et al., 2012) and possible differences in susceptibility to O-glycosylation (SNP2) may directly contribute to the observed differences in biochemical and bone parameters. The associations of SNP3 variation with bone parameters could possibly reflect minute effects on FGF23 function and PTH and phosphate concentrations, that were not statistically detectable.
The large cohort of healthy infants with uniform and extensive follow-up is a notable strength of this study, uniquely allowing us to examine genotype effects on temporal changes, in addition to cross-sectional associations, of the studied biochemical and bone parameters. Despite the cohort size, minor allele homozygotes of the studied SNPs, and consequently their haplotypes, were, however, rare, making detection of significant genotype effects challenging and arguably inconclusive, for example, for variants where heterozygotes show greatest means. It is possible that some genotype associations may have escaped detection, and larger studies are warranted to further examine the associations of FGF23 variation and mineral and bone metabolism. Although the intervention setting allowed us to examine the potential impact of different vitamin D supplementation doses, direct supplementation effects on, for example, 25-OHD and PTH concentrations may have made detection of smaller, FGF23 genotype-derived associations more challenging, especially in the higher-dose supplementation group. Analyses of 1,25-(OH)2D, urinary phosphate, creatinine, or calcium were unfortunately not conducted in this study.
Our study presents novel associations of FGF23 and phosphate concentrations and bone strength with common SNPs of FGF23 in healthy infants. Our findings highlight the role of FGF23 in bone modeling, in particular effects on bone mineral content, cross-sectional area, and PMI, in growing young children. As simple measurements of FGF23 concentrations are subject to many confounding factors, our findings can help understand the role of FGF23 in bone metabolism and its temporal changes during early childhood.
Data availability statement
Data cannot be shared publicly because the data consists of sensitive patient data. More specifically the data consists of individual clinical data and individual genotypes for young children. Data are available from the Helsinki University Hospital's Institutional Data Access/Ethics Committee for researchers who meet the criteria for access to confidential data. Data availability contacts: OM, b3V0aS5tYWtpdGllQGhlbHNpbmtpLmZp.
Ethics statement
The studies involving human participants were reviewed and approved by The VIDI trial was approved by the Research Ethics Committee of the Hospital District of Helsinki and Uusimaa, Finland (107/13/03/03/2012). Written informed consent to participate in this study was provided by the participants' legal guardian/next of kin.
Author contributions
ME-C, SA, MP, and OM designed the study. SA and OM oversaw the study and provided facilities and reagents. ME-C, EH-S, SV, HH, and JR acquired the data, conducted follow-up visits, obtained venous blood samples, and participated in pQCT measurements. ME-C and MP planned and performed genotype analyses. ME-C analyzed the data and wrote the first draft of the manuscript. All authors contributed to the article and approved the submitted version.
Funding
The study was supported by grants from the Folkhälsan Research Foundation, the Finnish Medical Foundation, Victoriastiftelsen, the Orion Research Foundation, the Instrumentarium Science Foundation, the Paulo Foundation, the Päivikki and Sakari Sohlberg Foundation, the Juho Vainio Foundation, the Foundation of Finnish Pediatric Research in Finland, the Academy of Finland, the Sigrid Jusélius Foundation, the Swedish Research Council, the Novo Nordisk Foundation, Finska Läkaresällskapet, Stiftelsen Dorothea Olivia, Karl Walter och Jarl Walter Perkléns minne and State funding for university-level health research in Finland. Funding for publication was received from the Helsinki University Library.
Acknowledgments
The authors wish to express their utmost gratitude to all the participants of VIDI and their families. The authors also thank the personnel of the Kätilöopisto Maternity Hospital in Helsinki, the Pediatric Research Center and the Folkhälsan Research Center as well as laboratory technicians Laura Koljonen and Sari Lindén, and study nurses Sirpa Nolvi, Rhea Paajanen, Päivi Turunen, and Nea Boman for their invaluable contributions.
Conflict of interest
The authors declare that the research was conducted in the absence of any commercial or financial relationships that could be construed as a potential conflict of interest.
Publisher’s note
All claims expressed in this article are solely those of the authors and do not necessarily represent those of their affiliated organizations, or those of the publisher, the editors, and the reviewers. Any product that may be evaluated in this article, or claim that may be made by its manufacturer, is not guaranteed or endorsed by the publisher.
Supplementary material
The Supplementary Material for this article can be found online at: https://www.frontiersin.org/articles/10.3389/fgene.2023.1192368/full#supplementary-material
References
ADHR Consortium (2000). Autosomal dominant hypophosphataemic rickets is associated with mutations in FGF23. Nat. Genet. Nat. Publ. Group 26 (3), 345–348. doi:10.1038/81664
Agoro, R., Ni, P., Noonan, M. .L., and White, K. .E. (2020). Osteocytic FGF23 and its kidney function. Front. Endocrinol. (Lausanne) 11, 592. doi:10.3389/fendo.2020.00592
Araya, K., Fukumoto, S., Backenroth, R., Takeuchi, Y., Nakayama, K., Ito, N., et al. (2005). A novel mutation in fibroblast growth factor 23 gene as a cause of tumoral calcinosis. J. Clin. Endocrinol. Metab. 90 (10), 5523–5527. doi:10.1210/jc.2005-0301
Arnold, A., Dennison, E., Kovacs, C. .S., Mannstadt, M., Rizzoli, R., Brandi, M. .L., et al. (2021). Hormonal regulation of biomineralization. Nat. Rev. Endocrinol. Nat. Publ. Group 17 (5), 261–275. doi:10.1038/s41574-021-00477-2
Benet-Pagès, A., Lorenz-Depiereux, B., Zischka, H., White, K. .E., Econs, M. .J., and Strom, T. .M. (2004). FGF23 is processed by proprotein convertases but not by PHEX. Bone 35 (2), 455–462. doi:10.1016/j.bone.2004.04.002
Benet-Pagès, A., Orlik, P., Strom, T. .M., and Lorenz-Depiereux, B. (2005). An FGF23 missense mutation causes familial tumoral calcinosis with hyperphosphatemia. Hum. Mol. Genet. 14 (3), 385–390. doi:10.1093/hmg/ddi034
Bouksila, M., Mrad, M., Kaabachi, W., Kalai, E., Smaoui, W., Rekik, S., et al. (2019). Correlation of Fgf23 and balp with bone mineral density in hemodialysis patients. J. Med. Biochem. 38 (4), 418–426. doi:10.2478/jomb-2019-0002
Chuang, G.-T., Liu, P.-H., Chyan, T.-W., Huang, C.-H., Huang, Y.-Y., Lin, C.-H., et al. (2020). Genome-wide association study for circulating fibroblast growth factor 21 and 23. Sci. Rep. 10 (1), 14578. Nature Publishing Group. doi:10.1038/s41598-020-71569-8
Enlund-Cerullo, M., Hauta-Alus, H., Valkama, S., Rosendahl, J., Andersson, S., Mäkitie, O., et al. (2020). Fibroblast growth factor 23 concentrations and modifying factors in children from age 12 to 24 months. Bone 141, 115629. doi:10.1016/j.bone.2020.115629
Erben, R. .G., and Andrukhova, O. (2017). FGF23-Klotho signaling axis in the kidney. Bone 100, 62–68. doi:10.1016/j.bone.2016.09.010
Gohil, A., and Imel, E. .A. (2019). FGF23 and associated disorders of phosphate wasting. Pediatr. Endocrinol. Rev. 17 (1), 17–34. doi:10.17458/per.vol17.2019.gi.fgf23anddisordersphosphate
Helve, O., Viljakainen, H., Holmlund-Suila, E., Rosendahl, J., Hauta-Alus, H., Enlund-Cerullo, M., et al. (2017). Towards evidence-based vitamin D supplementation in infants: vitamin D intervention in infants (VIDI) - study design and methods of a randomised controlled double-blinded intervention study. BMC Pediatr. Biomed. Cent. 17 (1), 91. doi:10.1186/s12887-017-0845-5
Holmlund-Suila, E., Enlund-Cerullo, M., Valkama, S., Hauta-Alus, H., Rosendahl, J., Helve, O., et al. (2017). Sex and iron modify fibroblast growth factor 23 concentration in 1-year-old children. J. Clin. Endocrinol. Metab. 102 (12), 4526–4533. doi:10.1210/jc.2017-01211
Holmlund-Suila, E., Viljakainen, H., Ljunggren, O., Hytinantti, T., Andersson, S., and Mäkitie, O. (2016). Fibroblast growth factor 23 concentrations reflect sex differences in mineral metabolism and growth in early infancy. Horm. Res. Paediatr. 85 (4), 232–241. Karger Publishers. doi:10.1159/000443988
International HapMap Consortium (2005). A haplotype map of the human genome. Nat. Nat. Publ. Group 437 (7063), 1299–1320. doi:10.1038/nature04226
Ireland, A., Rittweger, J., Schönau, E., Lamberg-Allardt, C., and Viljakainen, H. (2014). Time since onset of walking predicts tibial bone strength in early childhood. Bone 68, 76–84. doi:10.1016/j.bone.2014.08.003
Kestenbaum, B., Glazer, N. .L., Köttgen, A., Felix, J. .F., Hwang, S.-J., Liu, Y., et al. (2010). Common genetic variants associate with serum phosphorus concentration. J. Am. Soc. Nephrol. 21 (7), 1223–1232. doi:10.1681/ASN.2009111104
Kiviranta, P., Kuiri-Hänninen, T., Saari, A., Lamidi, M.-L., Dunkel, L., and Sankilampi, U. (2016). Transient postnatal gonadal activation and growth velocity in infancy. Pediatrics 138 (1), e20153561. doi:10.1542/peds.2015-3561
Koljonen, L., Enlund-Cerullo, M., Hauta-Alus, H., Holmlund-Suila, E., Valkama, S., Rosendahl, J., et al. (2021). Phosphate concentrations and modifying factors in healthy children from 12 to 24 months of age. J. Clin. Endocrinol. Metab. 106, 2865–2875. doi:10.1210/clinem/dgab495
Lederer, E. (2014). Regulation of serum phosphate. J Physiol. John Wiley Sons, Ltd 592 (18), 3985–3995. doi:10.1113/jphysiol.2014.273979
Lek, M., Karczewski, K. .J., Minikel, E. .V., Samocha, K. .E., Banks, E., Fennell, T., et al. (2016). Analysis of protein-coding genetic variation in 60,706 humans. Nature 536 (7616), 285–291. Nature Publishing Group. doi:10.1038/nature19057
Martin, A., David, V., and Quarles, L. .D. (2012). Regulation and function of the FGF23/klotho endocrine pathways. Physiol Rev. Am. Physiological Soc. 92 (1), 131–155. Bethesda, MD. doi:10.1152/physrev.00002.2011
Pekkinen, M., Laine, C. .M., Mäkitie, R., Leinonen, E., Lamberg-Allardt, C., Viljakainen, H., et al. (2015). FGF23 gene variation and its association with phosphate homeostasis and bone mineral density in Finnish children and adolescents. Bone 71, 124–130. doi:10.1016/j.bone.2014.10.013
Quarles, L. .D. (2012). Role of FGF23 in vitamin D and phosphate metabolism: Implications in chronic kidney disease. Exp. Cell. Res. 318 (9), 1040–1048. doi:10.1016/j.yexcr.2012.02.027
Rendina, D., Esposito, T., Mossetti, G., De Filippo, G., Gianfrancesco, F., Perfetti, A., et al. (2012). A functional allelic variant of the FGF23 gene is associated with renal phosphate leak in calcium nephrolithiasis. J. Clin. Endocrinol. Metab. 97 (5), E840–E844. doi:10.1210/jc.2011-1528
Robinson-Cohen, C., Bartz, T. .M., Lai, D., Ikizler, T. .A., Peacock, M., Imel, E. .A., et al. (2018). Genetic variants associated with circulating fibroblast growth factor 23. J Am Soc Nephrol. Am. Soc. Nephrol. 29 (10), 2583–2592. doi:10.1681/ASN.2018020192
Rosendahl, J., Valkama, S., Holmlund-Suila, E., Enlund-Cerullo, M., Hauta-Alus, H., Helve, O., et al. (2018). Effect of higher vs standard dosage of vitamin D3 supplementation on bone strength and infection in healthy infants: A randomized clinical trial. JAMA Pediatr. 172 (7), 646–654. doi:10.1001/jamapediatrics.2018.0602
Rothe, H., Brandenburg, V., Haun, M., Kollerits, B., Kronenberg, F., Ketteler, M., et al. (2017). Ecto-5' -Nucleotidase CD73 (NT5E), vitamin D receptor and FGF23 gene polymorphisms may play a role in the development of calcific uremic arteriolopathy in dialysis patients - data from the German Calciphylaxis Registry. Public Libr. Sci. 12 (2), e0172407. doi:10.1371/journal.pone.0172407
Sisuproject (2018). Sequencing initiative suomi project (SISu). [SISu v4.1, October, 2018]. Available at: http://sisuproject.fi.
Saari, A., Sankilampi, U., Hannila, M.-L., Kiviniemi, V., Kesseli, K., and Dunkel, L. (2011). New Finnish growth references for children and adolescents aged 0 to 20 years: Length/height-for-age, weight-for-length/height, and body mass index-for-age. Ann Med. Taylor Francis 43 (3), 235–248. doi:10.3109/07853890.2010.515603
Schwantes-An, T.-H., Liu, S., Stedman, M., Decker, B. .S., Wetherill, L., Edenberg, H. .J., et al. (2019). Fibroblast growth factor 23 genotype and cardiovascular disease in patients undergoing hemodialysis. Am. J. Nephrol. Karger Publ. 49 (2), 125–132. doi:10.1159/000496060
Shimada, T., Kakitani, M., Yamazaki, Y., Hasegawa, H., Takeuchi, Y., Fujita, T., et al. (2004). Targeted ablation of Fgf23 demonstrates an essential physiological role of FGF23 in phosphate and vitamin D metabolism. J Clin Invest. Am. Soc. Clin. Investigation 113 (4), 561–568. doi:10.1172/JCI19081
Shimada, T., Mizutani, S., Muto, T., Yoneya, T., Hino, R., Takeda, S., et al. (2001). Cloning and characterization of FGF23 as a causative factor of tumor-induced osteomalacia. Proc. Natl. Acad. Sci. U. S. A. 98 (11), 6500–6505. doi:10.1073/pnas.101545198
Shimada, T. (2007). Possible role of the FGF23-Klotho axis in bone mineralization. Clin. Calcium 17 (10), 1548–1553.
Takashi, Y., Fukumoto, S., and Matsumoto, T. (2018). [Bone-derived hormones and their systemic regulation]. Clin. Calcium 28 (1), 9–15.
Valkama, S., Holmlund-Suila, E., Ireland, A., Hauta-Alus, H., Enlund-Cerullo, M., Rosendahl, J., et al. (2020). Peripheral quantitative computed tomography (pQCT) in 12- and 24-month-old children - practical aspects and descriptive data. Bone 141, 115670. doi:10.1016/j.bone.2020.115670
Wang, H., Yoshiko, Y., Yamamoto, R., Minamizaki, T., Kozai, K., Tanne, K., et al. (2008). Overexpression of fibroblast growth factor 23 suppresses osteoblast differentiation and matrix mineralization in vitro. J. Bone Min. Res. 23 (6), 939–948. John Wiley and Sons, Ltd. doi:10.1359/jbmr.080220
Wang, Y., Wang, H., and Chen, P. (2020). Higher fibroblast growth factor 23 levels are causally associated with lower bone mineral density of heel and femoral neck: Evidence from two-sample mendelian randomization analysis. Front. Public Health. Front. 8, 467. doi:10.3389/fpubh.2020.00467
White, K. .E., Carn, G., Lorenz-Depiereux, B., Benet-Pages, A., Strom, T. .M., and Econs, M. .J. (2001). Autosomal-dominant hypophosphatemic rickets (ADHR) mutations stabilize FGF-23. Kidney Int. 60 (6), 2079–2086. doi:10.1046/j.1523-1755.2001.00064.x
Yamazaki, Y., Tamada, T., Kasai, N., Urakawa, I., Aono, Y., Hasegawa, H., et al. (2008). Anti-FGF23 neutralizing antibodies show the physiological role and structural features of FGF23. J Bone Min. Res. John Wiley Sons, Ltd 23 (9), 1509–1518. doi:10.1359/jbmr.080417
Yokomoto-Umakoshi, M., Umakoshi, H., Miyazawa, T., Ogata, M., Sakamoto, R., and Ogawa, Y. (2021). Investigating the causal effect of fibroblast growth factor 23 on osteoporosis and cardiometabolic disorders: A mendelian randomization study. Bone 143, 115777. doi:10.1016/j.bone.2020.115777
Keywords: FGF23, genetic variation, vitamin D, bone strength, infants (0–24 months)
Citation: Enlund-Cerullo M, Holmlund-Suila E, Valkama S, Hauta-alus H, Rosendahl J, Andersson S, Pekkinen M and Mäkitie O (2023) Variation in the fibroblast growth factor 23 (FGF23) gene associates with serum FGF23 and bone strength in infants. Front. Genet. 14:1192368. doi: 10.3389/fgene.2023.1192368
Received: 23 March 2023; Accepted: 27 April 2023;
Published: 22 May 2023.
Edited by:
Maritha J. Kotze, National Health Laboratory Service, South AfricaReviewed by:
Liping Xiao, UCONN Health, United StatesKonstantin Kulebyakin, Lomonosov Moscow State University, Russia
Nobuaki Ito, The University of Tokyo Hospital, Japan
Copyright © 2023 Enlund-Cerullo, Holmlund-Suila, Valkama, Hauta-alus, Rosendahl, Andersson, Pekkinen and Mäkitie. This is an open-access article distributed under the terms of the Creative Commons Attribution License (CC BY). The use, distribution or reproduction in other forums is permitted, provided the original author(s) and the copyright owner(s) are credited and that the original publication in this journal is cited, in accordance with accepted academic practice. No use, distribution or reproduction is permitted which does not comply with these terms.
*Correspondence: Maria Enlund-Cerullo, bWFyaWEuZW5sdW5kQGhlbHNpbmtpLmZp
†ORCID: Maria Enlund-Cerullo, orcid.org/0000-0001-9057-1840