- Department of Biological Sciences, Faculty of Science, Kuwait University, Kuwait City, Kuwait
Introduction: Chronic heat stress during summer is a major challenge imposed by global warming. Chickens are more sensitive to heat stress than mammals because they lack sweat glands. Thus, chickens are more susceptible to heat stress during summer than other seasons. Induction of heat shock protein (HSP) genes is one of the primary defense mechanisms against heat stress. Tissue-specific responses exhibited by different classes of HSPs upon exposure to heat stress have been reported previously in different tissues including the heart, kidney, intestine, blood, and muscle, but not in the retina. Therefore, this study aimed to investigate the expression levels of HSP27, HSP40, HSP60, HSP70, and HSP90 in the retina under chronic heat stress.
Methods: This study was conducted during the summers of 2020 and 2021 in Kuwait. Chickens (Gallus gallus) were divided into control and heat-treated groups and sacrificed at different developmental stages. Retinas were extracted and analyzed by using Real Time quantitative Polymerase Chain Reaction (RT-qPCR).
Results: Our results from the summer of 2021 were similar to that from the summer of 2020, regardless of whether GAPDH or RPL5 was used as a gene normalizer. All five HSP genes were upregulated in the retina of 21-day-old heat-treated chickens and stayed upregulated until 35 days of age, with the exception of HSP40, which was downregulated. The addition of two more developmental stages in the summer of 2021 showed that at 14 days, all HSP genes were upregulated in the retina of heat-treated chickens. In contrast, at 28 days, HSP27 and HSP40 were downregulated, whereas HSP60, HSP70, and HSP90 were upregulated. Furthermore, our results showed that under chronic heat stress, the highest upregulation of HSP genes was seen at the earliest developmental stages.
Discussion: To the best of our knowledge, this is the first study to report the expression levels of HSP27, HSP40, HSP60, HSP70, and HSP90 in the retina under chronic heat stress. Some of our results match the previously reported expression levels of some HSPs in other tissues under heat stress. These results suggest that HSP gene expression can be used as a biomarker for chronic heat stress in the retina.
1 Introduction
Increases in global temperature pose severe environmental challenges. Heat stress is one of the most challenging environmental stresses imposed by global warming and the rise in global temperature. Heat stress occurs in two ways: 1) acute heat stress, which is characterized by a brief period of intense environmental temperatures and 2) chronic heat stress, which is characterized by prolonged high temperatures. Heat stress affects domestic animal production, especially in commercial poultry, causing massive economic losses (Temim et al., 2000; Das et al., 2016; Shehata et al., 2020; Nawaz et al., 2021).
Kuwait is a small country in the Arabian Gulf region known for its dry and hot desert climate with very high temperatures during summers. Kuwait’s extremely hot weather affects the desert and marine ecosystems, with daily maximum temperatures exceeding their long-term averages for several consecutive days (Nasrallah et al., 2004; Al-Awadhi et al., 2005). Summer in Kuwait lasts from the end of May until mid-September, with the hottest months being June–August (Sedaghat et al., 2021). Hence, chronic heat stress can be easily induced in Kuwait during the summer. In 2016, Asia’s highest temperature was recorded in Kuwait at 54.0°C (Alahmad et al., 2020). In 2021, Kuwait City recorded 53.2°C, the world’s highest recorded temperature in the summer of 2021. (https://gulfnews.com/world/gulf/kuwait/kuwait-registers-highest-temperature-on-earth-for-2021-1.80222855).
Chickens (Gallus gallus) are homoeothermic animals with a maintenance body temperature in the range of 41–42°C. Chickens lack sweat glands and are therefore more sensitive to high temperatures and heat stress than other animals (Murugesan et al., 2017; Cândido et al., 2020). During heat stress, metabolic heat increases, triggering hyperthermia (Nawaz et al., 2021), which is a combined effect of high environmental temperature and humidity (Olcina et al., 2019). Hyperthermic chickens are characterized by poor growth owing to damaged skeletal muscle cells and impaired protein synthesis. Additionally, they are immunocompromised and therefore, may suffer from fatal diseases (Shehata et al., 2020; Nawaz et al., 2021).
One of the primary defense mechanisms against hyperthermia is the activation of heat shock proteins (HSPs), also called “stress proteins”. HSPs are the major players in adaptive responses to heat stress and are considered the first line of protection for cells exposed to heat stress (Piri et al., 2016; Cedraz et al., 2017; Murugesan et al., 2017; Dubrez et al., 2020; Siddiqui et al., 2020a; Siddiqui et al., 2020b). HSPs are a large group of molecular chaperones that can block signaling cascades that affect cell death, protect essential signaling pathways needed for cell survival, or inhibit misfolded protein formation and aggregation. HSPs are classified into five families based on their molecular weight: HSP27, HSP60, HSP70, HSP90, and HSP110 (Bukau et al., 2000; Oshlag et al., 2013; Dubrez et al., 2020).
Previous studies on the effects of heat stress on tissue-specific responses in chickens have shown that HSP expression is induced upon exposure to acute and chronic heat stress. The expression levels of HSP70, HSP60, and HSP47 were significantly high in different sections of the small intestine of chickens (duodenum, ileum, and jejunum) upon exposure to acute heat stress (Siddiqui et al., 2020). Moreover, there were differences in the gene expression of HSPs in different regions of the chicken intestine. For example, the mRNA and protein expression of HSP70 and HSP90 increased in the ileum more than that in the jejunum upon exposure to heat stress. A study confirmed that the gastrointestinal tract of chickens is primarily responsive to heat stress (Varasteh et al., 2015). In another report, HSP70 and HSP90 genes were highly expressed in the muscle of chronic heat-stressed chickens when compared to the muscle of control chickens (Cedraz et al., 2017). Tissue-specific responses to acute and chronic heat stress have also been documented in the hearts and livers of chickens. For example, during chronic heat treatment, HSP70 mRNA levels increased in the heart, but HSP90 mRNA levels decreased. During acute heat challenge, the expression of HSP70 was highly increased in the liver, and HSP90 expression is increased by chronic heat treatment (Xie et al., 2014).
The retina is a sensory nerve membrane that lines the back of the eye to facilitate a connection between nerve impulses and the brain via the optic nerve. The chicken retina comprises neuronal cells that are precisely organized within defined layers. These neuronal cells and layers are conserved across all vertebrates (Yamagata et al., 2021). There are numerous studies on HSPs in retinal responses to eye diseases or other environmental stressors, and most of these studies have reported that HSPs protect the retina from damage. For example, HSPs have been shown to play a protective role against apoptosis in the retinas of diabetic retinopathy (Mohammad and Kowluru, 2011; Pinach et al., 2013; Reddy et al., 2013; Jo et al., 2014). Under hypoxic stress, HSP27 is upregulated in the retina and serves as a cytoprotective factor for preventing retinal ischemic damage (Whitlock et al., 2005). Moreover, upon exposure to bright light, HSP27 is shown to protect the photoreceptors by decreasing apoptosis of retinal cells (Chien et al., 2017). HSP70 also plays a neuroprotective role in retinal ganglion cells in hyperthermia, glaucoma, and hypertension (Piri et al., 2016).
Research on HSPs in the retinas of chickens exposed to heat stress is limited, but a few studies have demonstrated their roles in other organisms. For example, in one study, HSP70 mRNA and proteins were localized to the photoreceptor layer of the rat retina after brief exposure to whole-body hyperthermic treatment (Tytell et al., 1994). In another study, HSP70 mRNA levels increased in the retina of zebrafish after 30 min of heat shock induction at 37°C (Fujikawa et al., 2012). However, further studies are needed to explore the effects of HSPs in the retina in response to heat stress.
The objective of the present study was to determine the effect of chronic heat stress on the retina of chickens during summer season in Kuwait. This study was conducted over 2 years, during the summers of 2020 (August–September) and 2021(June–July), to assess the effect(s) of retinal expression of HSP27, HSP40, HSP60, HSP70, and HSP90 in chickens.
2 Materials and methods
2.1 Ethical approval
The chickens used in the present study were under the care and supervision of a poultry veterinarian at Kuwait University. The chickens were handled, maintained, and experimented with according to the “Instructions Guide” for the Care and Use of Laboratory Animals (National Research Council, 1996). The experimental procedures were approved by the Ethics Committee on Animal Use at Kuwait University and ECULA Ethical Committee for the Use of Laboratory Animals (DBS/IRB(ECULA)20-005).
2.2 Animals
In the present study, 68 local Kuwaiti chicks (Gallus gallus domesticus) were used across experiments conducted in the summers of 2020 and 2021. Newly hatched chicks (8–10 days old) were purchased from local farms in Kuwait and divided into two groups: the control (indoor) group, in which the temperature was maintained at 25°C, and the heat-treated (outdoor) group, in which the temperature fluctuated according to the outside temperature during summer in Kuwait (35–50°C). Temperatures were recorded thrice every day (morning, afternoon, and evening) (Figures 1, 2). The chicks were carefully observed for behavioral changes, especially in the outdoor group, to assess normal behavior (such as eating, breathing, and moving) and avoid sudden deaths caused by excessive heat. The chicks in both groups had full access to food and water at all times.
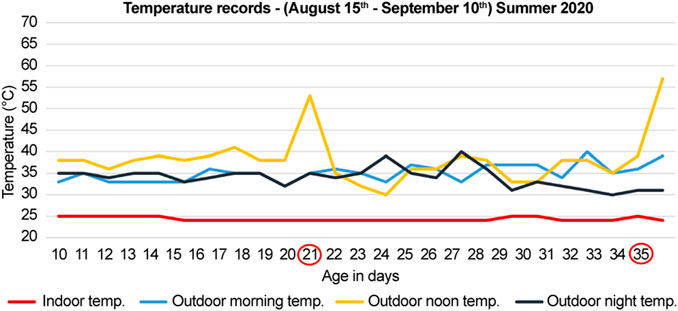
FIGURE 1. Temperature records for summer of 2020 (months of August and September). The documented temperature during the morning, noon, and night for the control (indoor) and heat-treated (outdoor) groups. The first group was sacrificed at 21-days, and the second group was sacrificed at 35-days (circled in red). The peaks indicate direct exposure to sun at noon for 10 min before sacrifice of the heat-treated chickens.
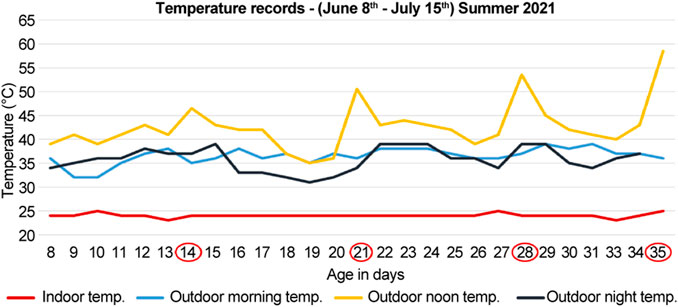
FIGURE 2. Temperature records for the summer of 2021 (months of June and July). The documented temperature during the morning, noon, and night for the control (indoor) and heat-treated (outdoor) groups. The first, second, third, and fourth groups were sacrificed at 14-, 21-, 28-, and 35-days (circled in red), respectively. The peaks indicate direct exposure to sun at noon for 10 min before sacrifice of the heat-treated chickens.
2.3 Distribution, number of chicks, and treatment end point
2.3.1 Summer of 2020
During the summer of 2020 (months of August and September), 28 chicks were procured and divided into two groups; each group had seven control (indoor) and seven heat-treated (outdoor) chicks. The first and second groups were sacrificed at 21- and 35-days, respectively (Figure 1). The number of deaths were recorded. The recorded mortality in each group ranged between 2–4 chicks. Therefore, three chicks were used in each group to standardize the number of chicks in each group.
The outdoor noon temperatures ranged from to 36–41°C, and for the indoor group, it was maintained at 25°C. Before sacrifice, the outdoor chickens were further exposed to the direct sun for 10 min at a temperature of 53°C in the 21-days old group and 57°C in the 35-days old group (Figure 1). The indoor groups were sacrificed indoors at 25°C at the same time (Figure 1).
2.3.2 Summer of 2021
During the summer of 2021 (months of June and July), 40 chicks were divided into four groups; each group had five control (indoor) and five heat-treated (outdoor) chicks. The first, second, third, and fourth groups were sacrificed at 14-, 21-, 28-, and 35-days, respectively (Figure 2). The number of deaths were recorded. The recorded mortality in each group ranged from 1–2 chicks. Therefore, three chicks were used in each group to standardize the number of chicks in each group.
The noon temperatures ranged from 39 to 45°C for the heat-treated group, and 25°C for the control group. Before sacrifice, the heat-treated chickens were further exposed to direct sun for 10 min at a temperature of 46.5°C for the 14-days old, 50.5°C for the 21-days old, 53.5°C for the 28-days old, and 58.5°C for 35-days old chicks (Figure 2). The control groups were sacrificed indoors at 25°C at the same time (Figure 2).
Chickens were sacrificed by cervical dislocation, their eyes were collected, and their retinas were excised and immediately processed for RNA extraction.
2.4 RNA extraction and cDNA synthesis
RNA was extracted using TRIzol reagent according to the manufacturer’s protocol (15596018, Invitrogen, Waltham, MA, United States) and then resuspended in 50 µL diethylpyrocarbonate-treated water. The quantity and quality of the RNA samples were checked using a Nanodrop 8,000 spectrophotometer (ND-8000-GL, Thermo Fisher Scientific, Inc., Waltham, MA, United States). Genomic DNA was removed using a TURBO DNA-free kit (AM 1907, Invitrogen). A cDNA reverse transcription kit was used to synthesize cDNA and oligo dT primers, according to the manufacturer’s protocol (4368814, Applied Biosystems, Waltham, MA, United States). The quantity and quality of cDNA samples were evaluated using a Nanodrop 8,000 spectrophotometer.
2.5 Reverse transcriptase PCR (RT-PCR)
Forward and reverse primers for the reference and HSP genes were designed using the primer design tool at NCBI (http://www.ncbi.nlm.nih.gov/tools/primer-blast/) and used to amplify approximately 150–200 bp products (Table 1). For polymerase chain reaction (PCR), Platinum™ Green Hot Start PCR 2X master mix (13001014, Invitrogen) was used according to the manufacturer’s protocol. The cDNA concentration for all developmental stages was maintained at 25 ng for all PCR reactions. The PCR conditions were as follows: initial denaturation at 94°C for 2 min; 35 cycles of denaturation at 94°C for 30 s, annealing at 55°C for 30 s, and extension at 72°C for 30 s; and final extension at 72°C for 5 min. For gel electrophoresis, 12 µL of each PCR reaction was loaded onto a 1.5% agarose gel and stained with SYBR safe DNA stain (S33102, Invitrogen).
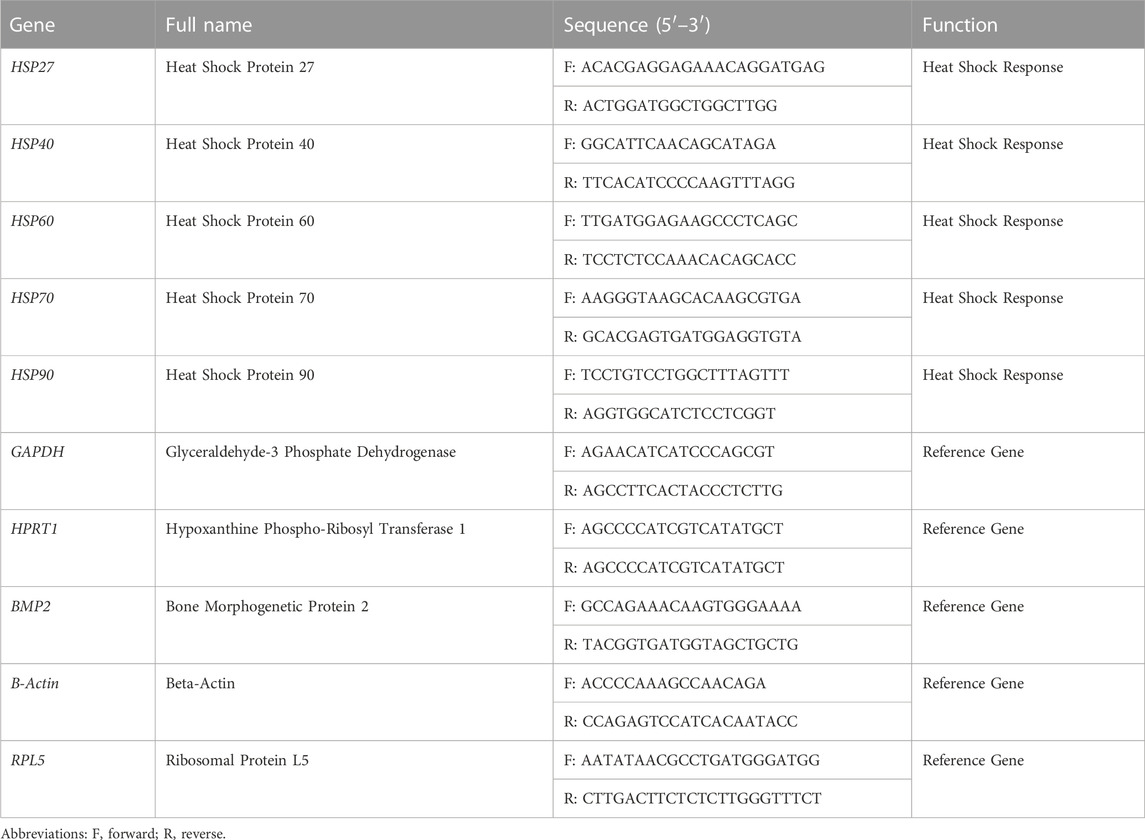
TABLE 1. Description of all primers related to Gallus gallus HSP genes and the reference genes used for RT-qPCR analysis.
2.6 Real-time quantitative PCR (RT-qPCR)
RT-qPCR was performed using a Bio-Rad CFX96 Real-Time system (C1000 Touch Thermal Cycle, Bio-Rad, Singapore). Reactions were performed in a 10 µL reaction mixture containing 5 µL PowerUp SYBR Green Master-Mix 2X (A25779, Applied Biosystems), 2 µL of 10 ng cDNA, 1 µL of each specific primer (10 µM), and 1 µL of molecular-grade water. Gene amplification was performed in duplicates, and each PCR run was performed thrice. A negative control (ultrapure water) was used in each assay. The PCR conditions were as follows: 50°C for 2 min; 40 cycles of 95°C for 2 min, 95°C for 15 s, 57°C for 15 s, 72°C for 1 min; and an extra step of melting analysis for each sample at 65°C for 5 s and 95°C for 5 s.
2.7 Statistical analysis of target genes
The Ct value was computed using Bio-Rad CFX96 software to quantify mRNA for each gene, and the average Ct values were calculated for each sample group. The mRNA expression as the ΔCt value (Ct target gene − Ct reference gene) was calculated for each HSP gene. Subsequently, the normalized relative expression ∆∆Ct (∆Ct heat-treated sample − ∆Ct control sample) was calculated for each gene. The fold change 2−ΔΔCT was then obtained for each HSP gene. Finally, statistical analysis was performed by using Student’s t-test with GraphPad Prism version 9 (GraphPad Software Inc., San Diego, CA, United States) to obtain the p-value and standard deviation for expression of each HSP gene. Statistical significance was set at p < 0.05.
3 Results
3.1 Specificity and efficiency of the reference genes and the choice of a normalizer for the RT-qPCR
Five reference genes (B-actin, BMP2, GAPDH, HPRT1, and RPL5) were tested for their real-time amplification patterns in the control and heat-treated chickens. We found that GAPDH had the lowest and most consistent cycle threshold (Ct) values in the control and treated groups, followed by RPL5 and B-actin (Figure 3). BMP2 and HPRT1 had very high and variable Ct values in both the control and treated groups. The dissociation curves for GAPDH (Figure 4F), RPL5 (Figure 4J), and B-actin (Figure 4B) showed one peak, indicating primer specificity for the target genes. However, the dissociation curves for BMP2 (Figure 4D) and HPRT1 (Figure 4H) showed small peaks in addition to the main peak, indicating poor specificity of the primers for the target genes. The amplification curves for the five genes (Figures 4A, C, E, G, I) confirm that GAPDH, followed by RPL5 and then B-actin, had the best amplification patterns with consistent Ct values.
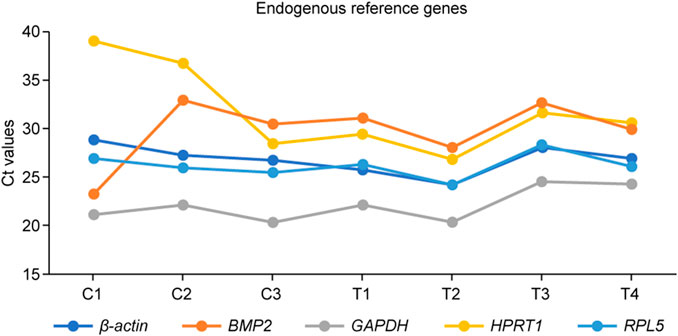
FIGURE 3. The threshold cycle (Ct) values for the five endogenous reference genes tested before use in the summer of 2021. C1–C3: retina collected from control (indoor) chicks, T1–T4: retina collected from heat-treated (outdoor) chicks.
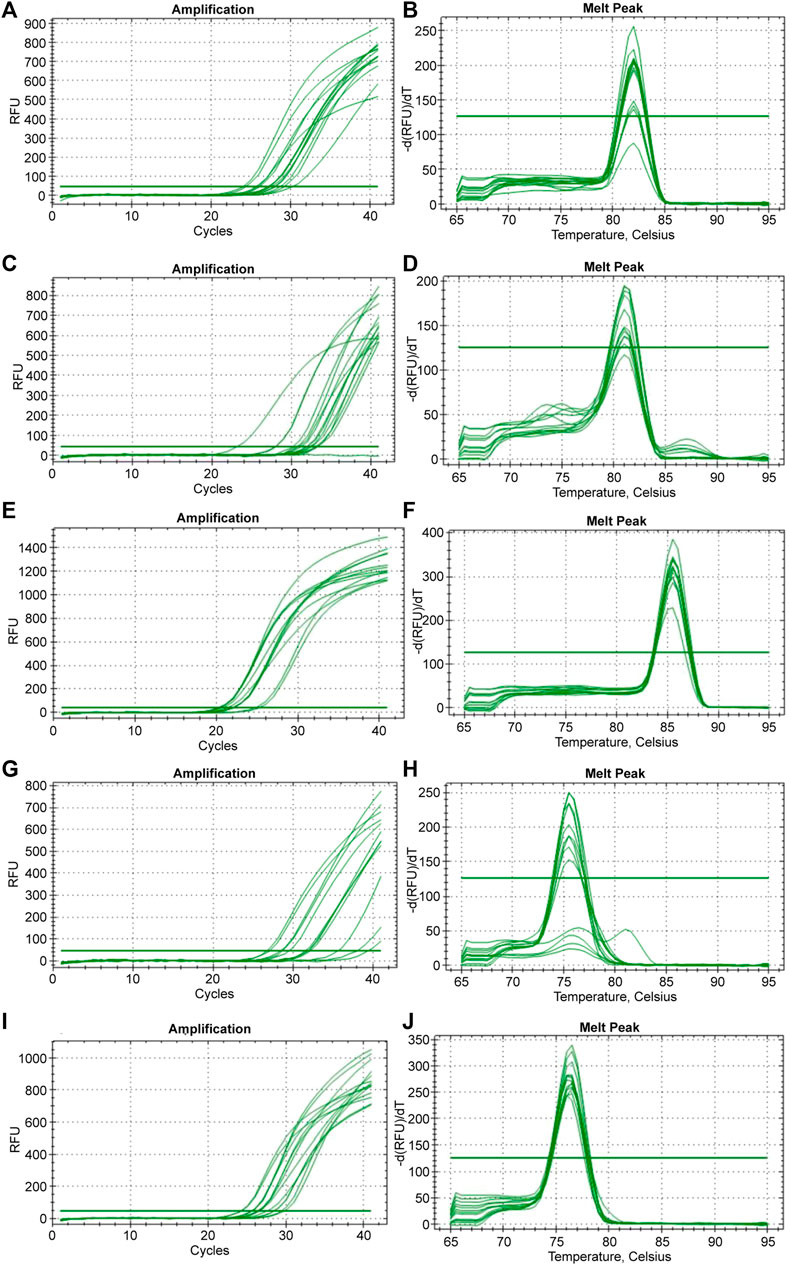
FIGURE 4. The amplification and dissociation of the efficiency test (melt peak) for the five reference genes tested in the study: (A, B) B-actin, (C, D) BMP2, (E, F) GAPDH, (G, H) HPRT1, and (I, J) RPL5.
Based on these results, GAPDH was used as a reference gene normalizer for all RT-qPCR-based gene expression analyses in the summer of 2020. RPL5 was chosen as a reference gene normalizer for all RT-qPCR-based gene expression analyses in the summer of 2021.
3.2 Relative expression of HSP genes in the summer of 2020
In the summer of 2020, at 21-days, all HSP genes were upregulated in the retinas of the heat-treated group when compared to that of the control group (Figure 5, light grey bars). The highest upregulation was observed for HSP70. At 35-days, all HSP genes were upregulated, except for HSP40, which was downregulated (Figure 5, gray and black striped bars). The highest upregulation was observed for HSP70.
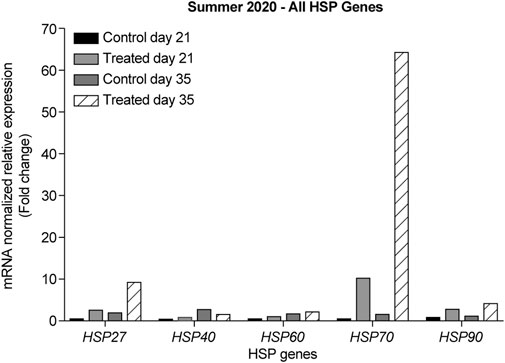
FIGURE 5. ΔΔ Ct of the relative normalized expression of HSP27, HSP40, HSP60, HSP70, and HSP90 against GAPDH in the control (indoor) vs. heat-treated (outdoor) chicks in the summer of 2020, using retinas from 21- and 35-days old chicks. (n = 3 in each group). HSP, heat shock protein.
3.3 Relative expression of HSP genes in the summer of 2021
In the summer of 2021, at 14- and 21-days, all HSP genes were upregulated in the retinas of the heat-treated group when compared to that of the control group (Figures 6–10, Days 14 and 21). At 28 days, HSP27 and HSP40 were downregulated in the retinas of the heat-treated group compared to that of the control group. In contrast, at 28 days, the expression levels of HSP60, HSP70, and HSP90 were upregulated in the retinas of the heat-treated group when compared to that of the control group (Figures 6–10, Day 28). At 35 days, all HSP genes were upregulated, except for HSP40, which was downregulated (Figures 6–10, Day 35).
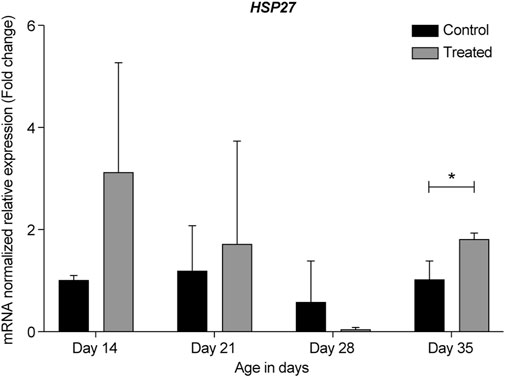
FIGURE 6. ΔΔ Ct of the relative normalized expression for HSP27 in the control (indoor) group and heat-treated (outdoor) chickens at 14-, 21-, 28-, and 35-days of age in the summer of 2021 (n = 3 in each group, *p < 0.05). HSP, heat shock protein.
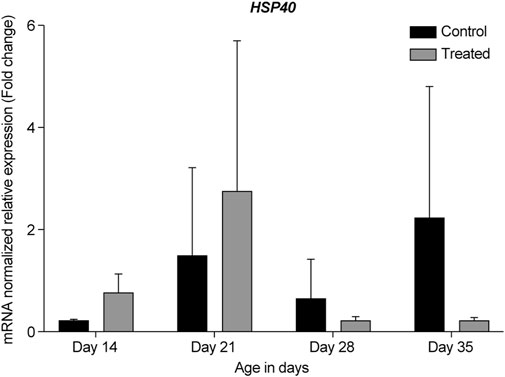
FIGURE 7. ΔΔ Ct of the relative normalized expression for HSP40 in the control (indoor) group and heat-treated (outdoor) chickens at 14-, 21-, 28-, and 35-days of age in the summer of 2021. (n = 3 in each group). HSP, heat shock protein.
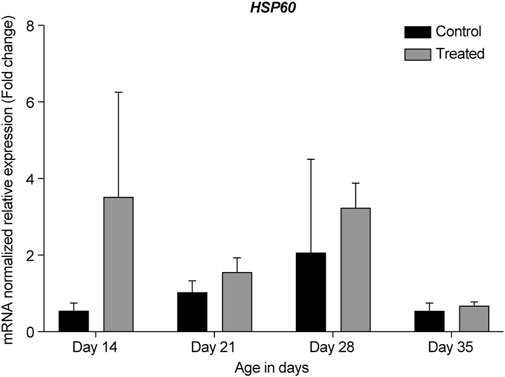
FIGURE 8. ΔΔ Ct of the relative normalized expression for HSP60 in the control (indoor) group and heat-treated (outdoor) chickens at 14-, 21-, 28-, and 35-days of age in the summer of 2021 (n = 3 in each group). HSP, heat shock protein.
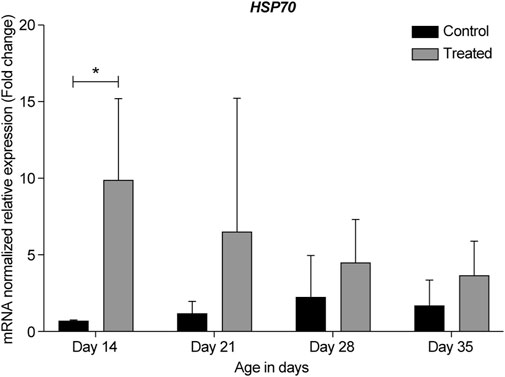
FIGURE 9. ΔΔ Ct of the relative normalized expression for HSP70 in the control (indoor) group and heat-treated (outdoor) chickens at 14-, 21-, 28-, and 35-days of age in the summer of 2021 (n = 3 in each group, *p < 0.05). HSP, heat shock protein.
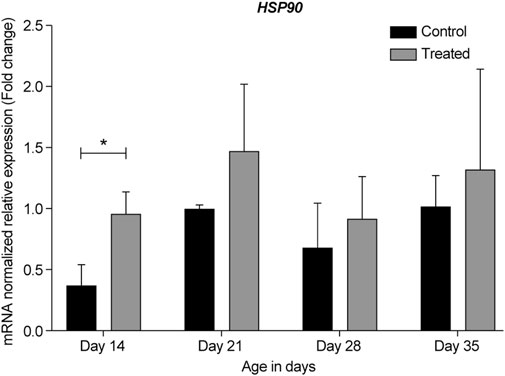
FIGURE 10. ΔΔ Ct of the relative normalized expression for HSP90 in the control (indoor) group and heat-treated (outdoor) chickens at 14-, 21-, 28-, and 35-days of age in the summer of 2021 (n = 3 in each group, *p < 0.05). HSP, heat shock protein.
The expression profile for HSP genes at 21- and 35-days in the summers of 2020 and 2021 was similar, regardless of the reference gene normalizer, which was GAPDH in the summer of 2020 and RPL5 in the summer of 2021. Moreover, a considerable upregulation of HSP27, HSP60, and HSP70 was observed in the earliest developmental stage, i.e., 14-day-old chicks (Figures 6, 8, 9, respectively).
4 Discussion
Extreme hot weather during summers is becoming a concern worldwide; the past 7 years have recorded the highest temperatures globally, and it is expected that upcoming years will continue to record high temperatures (Edmondson et al., 2022). The present study was conducted in Kuwait, a desert country with a hyper-arid climate, where temperatures frequently rise above 50°C during summer (Alahmad et al., 2020).
Extreme hot weather can induce chronic heat stress in all organisms. Heat stress triggers various adaptive physiological and cellular mechanisms, primarily the induction of HSPs to prevent hyperthermia, cellular damage, and death (Bouchama et al., 2017; Murugesan et al., 2017). Previous studies on the effects of heat stress on tissue-specific responses in chickens have demonstrated that exposure to heat stress severely affects different organs and tissues. The expression of HSPs is induced in various organs and tissues upon exposure to acute and chronic heat stress, such as the kidneys (Sikand et al., 2012), intestine (Varasteh et al., 2015; Siddiqui et al., 2020), muscle (Cedraz et al., 2017), heart, and liver (Xie et al., 2014). However, studies on the role of HSPs in the retinal response to heat stress are limited. Therefore, this study intended to determine the effect of chronic heat stress on the expression of HSP genes in the retina of chickens during the summer season.
In the summer of 2020, during the months of August and September, the morning, noon, and night temperatures ranged between, 33–39°C, 36–41°C, and 31–39°C, respectively (Figure 1). Likewise, in the summer of 2021, during the months of June and July, the temperatures ranged between 32 and 39°C in the morning, 39–45°C at noon, and 32–39°C in the evening (Figure 2). Therefore, the temperatures were similar in both years regardless of the different summer months in our study, except for the noon temperatures in 2021 summer, which were higher by approximately 3°–4°C than that of 2020 summer. Moreover, the mortality was unaffected by chronic heat stress in both years, as the number of recorded deaths was equal in both control groups and heat-treated groups in 2020 and 2021.
Before RT-qPCR analysis of retinal HSPs, the best reference gene as a normalizer for the experiment was tested and selected accordingly. GAPDH and B-actin are routinely used to normalize RT-qPCR data (Sikand et al., 2012). However, studies have suggested that the best reference gene may vary depending on the experimental conditions and tissue types used. Therefore, the reference gene selected should be suitable for the investigation of the tissue, and the study design (Dheda et al., 2004; Caracausi et al., 2017; Gromboni et al., 2020). Five endogenous reference genes were tested in this study: GAPDH (glyceraldehyde 3-phosphate dehydrogenase), B-actin (beta-actin), RPL5 (ribosomal protein L5), BMP2 (bone morphogenetic protein 2), and HPRT1 (hypoxanthine Phospho-Ribosyl transferase 1). A study on retinas from rats exposed to cyclic hyperoxia reported that HPRT1 was the best reference gene for retinal RT-qPCR (van Wijngaarden et al., 2007). A recent study aimed to identify the most stable reference genes for the normalization of target genes in the heart and liver of chickens exposed to heat stress and found that RPL5, and not B-actin or HPRT1, was the most stable reference gene for both heart and liver tissues (Gromboni et al., 2020).
Our results showed that GAPDH, followed by RPL5, was the best gene normalizer for retinal tissues, as they had the lowest and most consistent Ct values in both control and heat-treated chickens (Figures 3, 4). Therefore, GAPDH was chosen as the normalizer for RT-qPCR in the summer of 2020, and RPL5 was chosen as the normalizer for RT-qPCR in the summer of 2021. Regardless of the reference gene normalizer used, we obtained a similar pattern of expression of HSP genes for the 21- and 35-day-old retinas in the summer of 2020 and 2021 (Figures 5–10), indicating that both GAPDH and RPL5 can be used as reference gene normalizers for retinal tissues in heat stress experiments.
HSPs are a superfamily of stress proteins that promote cell survival and protect cells from thermal damage. Many studies correlate the overexpression of some HSP genes with protection against stress (Staib et al., 2007). Stress stimuli, such as hyperthermia, hypothermia, ischemia, hypoxia, depletion of ATP, free radicals, desiccation, steroid hormones, and ethanol, induce the expression of HSPs (Piri et al., 2016; Bellini et al., 2017). The cytoprotective roles of HSPs are related to their molecular chaperone functions (Piri et al., 2016; Murugesan et al., 2017). Molecular chaperones suppress protein aggregation and refold proteins into their native conformations. The well-studied molecular chaperones belong to the HSP family (Li et al., 2009; Murugesan et al., 2017).
HSP27 is a small heat shock protein that acts as a molecular chaperone by maintaining the denaturation of the protein in a folding-competent state. Moreover, it protects cells from oxidative stress and heat shock (Rogalla et al., 1999). HSP27 regulates apoptosis under stressful conditions and acts as an antioxidant when cells are exposed to oxidation and chemical stress (Vidyasagar et al., 2012). In the rat retina, HSP27 is upregulated under ischemic conditions and acts as a cytoprotective factor that prevents retinal damage (Whitlock et al., 2005). HSP27 suppression protected photoreceptor cells of the retina from apoptosis and restored retinal function in a light-induced rat retinal regeneration model (Chien et al., 2017). HSP27 was also upregulated in the retina of rats subjected to various models of retinal ganglion cell injury (Chidlow et al., 2014).
Considering HSP27 expression in relation to heat stress, previous studies have focused on cell lines exposed to chronic heat stress. In cardiac cells of hamsters subjected to hyperthermic treatment, HSP27 expression increased two-fold as compared to untreated cells (Ferns et al., 2006). Similarly, in cardiac cells of goats subjected to heat treatment, an increase in HSP27 mRNA when compared to the control was noted (Parida et al., 2020). Furthermore, when neonatal rat primary myocardial cells were subjected to heat stress in vitro, HSP27 mRNA levels increased after prolonged exposure to heat (Tang et al., 2013).
To the best of our knowledge, this study is the first to report the expression of HSP27 in the retina after exposure to chronic heat stress. Our data from the summers of 2020 and 2021 in Kuwait showed that HSP27 was upregulated in 14-, 21-, and 35-days old retinas of the heat-treated group when compared to that of the control group. The most upregulation was seen at 14-days with almost a two-fold change; however, at 28-days, HSP27 expression was downregulated (Figures 5, 6). This result might indicate similar roles of HSP27 in protecting the retina from damage and apoptosis upon exposure to chronic heat stress. However, the reason underlying the decrease in the expression of HSP27 at 28-days of age is unclear, especially given that HSP27 expression was upregulated at 21-days and significantly upregulated at 35-days (p = 0.024).
It has been reported that the expression of some HSP genes can fluctuate under certain heat treatments. For example, αB-crystallin, a small HSP gene closely related to HSP27, is downregulated in the heart of rats after 20 min of heat exposure, followed by upregulation at 40 min, and then downregulation again at 60 min (Tang et al., 2014). Therefore, HSP27 expression may also fluctuate under heat-stress. Another reason may be owing to differences in the gene expression of HSPs in different regions of the same organ. For example, the expression levels of HSP70 and HSP90 were higher in the ileum than in the jejunum of chicken intestinal tissues (Varasteh et al., 2015). In another study, HSP27, HSP70, and HSP90 were differentially expressed in different areas of the central nervous system of scrapie-infected and control sheep (Serrano et al., 2011). Based on these studies, it can be suggested that at 28 days, the expression of HSP27 was downregulated in the retina but maybe it was upregulated in other eye regions, such as the cornea or optic nerve.
HSP60 is typically known as a mitochondrial chaperonin protein that works together with the co-chaperonin HSP10. This chaperonin complex is essential for newly imported mitochondrial protein folding. HSP60 and HSP10 have also been shown to localize in the extracellular space, cytosol, and nucleus (Meng et al., 2018; Caruso Bavisotto et al., 2020). Most studies on HSP60 in the retina have reported that its expression is associated with several retinal diseases, such as glaucoma (Tsai et al., 2019) and diabetic retinopathy (Mohammad and Kowluru, 2011). Herein, we observed an upregulation of HSP60 in the retina of chickens in the heat-treated group at all developmental stages when compared to that in the control group (Figures 5, 8). The highest upregulation was observed at 14-days, with almost a two-fold change when compared to the control group and the other developmental stages.
To the best of our knowledge, this study is the first to report the expression of HSP60 under chronic heat stress in the retina. Previous studies investigating acute heat stress in chickens reported an increase in HSP60 mRNA in different tissues; for example, HSP60 was upregulated in the hearts of heat-stressed chickens within a few hours of treatment (Yu et al., 2008; Cheng et al., 2016). Similar findings were observed in the duodenum, jejunum, and ileum of chickens (Siddiqui et al., 2020). These results indicate that an increased HSP60 level may be an important marker at the beginning of heat stress and acts as protective in adverse environments (Yu et al., 2008).
HSP70 is a well-studied molecular chaperone and is highly conserved in all organisms (Franklin et al., 2005; Li et al., 2009; Rauch and Gestwicki, 2014; Radons, 2016). Moreover, it is the most common gene to be studied based on its response to different stressors (Zhao et al., 2013). HSP70 has three structural domains, including an N-terminal domain (ATPase domain) that binds ATP molecules, a substrate-binding domain that binds unfolded proteins, and a C-terminal domain that acts as a lid for the cavity. HSP40, however, is a co-chaperone protein and contains a J-domain, which enables it to bind to and regulate the ATPase domain in HSP70, thereby activating it (Li et al., 2009). Moreover, HSP40 coordinates other co-chaperones in binding HSP70, such as the HSP70-interacting protein (Lackie et al., 2017).
Most retinal studies have focused on HSP70, but not HSP40. Earlier studies in hyperthermic rats found that HSP70 mRNA was induced in the photoreceptor layer of the retina (Tytell et al., 1994). Later studies found that a brief period of hyperthermia in cultured cells and in the whole animal effectively induced HSP70 expression in the retina and significantly decreased photoreceptor degeneration in animals exposed to bright light (Piri et al., 2016).
Our data showed that under chronic heat stress, HSP70 was upregulated throughout all developmental stages, 14-, 21-, 28-, and 35-days compared to the control (Figures 5, 9). The highest upregulation was observed at the earliest age (p = 0.039). HSP40 was upregulated at the early stages, 14- and 21-days, but was downregulated at 28- and 35-days (Figures 5, 7). Our results may suggest that HSP40 is needed in the retina as a co-chaperone to activate HSP70 during the early stages of development; however, as retinal cells mature (recover or regenerate) within 28- and 35-days, there is no need for HSP40 expression, and HSP70 can function as the main chaperone independently.
Previous studies have reported that the expression of some HSP genes fluctuates across the developmental stages. For example, when 36 members of HSP family were examined in normal and abnormal development of embryonic hindlimbs, the RT-qPCR results showed that HSP27 and HSP40 expression levels changed from embryonic days 12–18 (Yan et al., 2015). As our chicks were developing from days 14–35, it could be possible that HSP27 and HSP40 were also differentially expressed throughout the different developmental stages. This may explain the sudden downregulation of HSP27 and HSP40 expression at 28-days, followed by the upregulation of HSP27 at 35 days, while the expression of HSP40 remained downregulated (Figures 6, 7).
HSP90 is one of the most abundant cellular chaperones and is involved in many cellular processes, such as folding, stability, maturation, maintenance, and degradation of several proteins. Additionally, it is the most conserved HSP that is essential for protection against heat shock response under normal and stress conditions (Retzlaff et al., 2009; Kanamaru et al., 2014; Hoter et al., 2018). In primate retina, HSP90 mRNA and protein are constitutively expressed at high levels in all layers of the retina and play a role in homeostasis. The retinal ganglion cells highly express HSP90 (Bernstein et al., 2001; Kanamaru et al., 2014). Prolonged inhibition of HSP90 has been shown to cause photoreceptor cell death (Zhou et al., 2013; Kanamaru et al., 2014). HSP90 and its inhibitors have been shown to prevent retinal degeneration in models of retinitis pigmentosa and age-related macular degeneration; therefore, they have been used as potential therapeutic agents for retinal diseases (Aguilà and Cheetham, 2016).
Herein, we found that under chronic heat stress, similar to HSP70, HSP90 expression in the retina was upregulated in the heat-treated groups at all developmental stages when compared to the same developmental stages in the control group (Figures 5, 10). Furthermore, similar to that for HSP70, the highest upregulation was seen at the earliest age, i.e., at 14-days (p = 0.036).
The similar pattern of expression of HSP70 and HSP90 observed in our study was not surprising as many studies have linked the chaperone function of these two proteins. For example, the C-terminal HSP70-interacting protein is a co-chaperone for both HSP70 and HSP90 (Lackie et al., 2017). Moreover, recent studies have found that under stressful conditions, heat shock factor-1 dissociates from HSP90 to induce HSP70 expression, which leads to the correct refolding of misfolded proteins and/or their clearance to alleviate cell stress (Chaudhury et al., 2021). Additionally, our results on HSP70 and HSP90 expression under chronic heat stress in the chicken retina are consistent with their reported expression under heat stress in other tissues, such as the heart, liver, kidney, blood, intestine, and muscle (Yu et al., 2008; Lei et al., 2009; Xie et al., 2014; Varasteh et al., 2015; Cedraz et al., 2017).
In chickens, approximately 90% of the retina is produced 1 week before hatching. Therefore, chickens have fully functional retinas after hatching (Fischer and Reh, 2000; Fischer, 2005). A zone of cells at the retinal margin of postnatal chicks, called the circumferential marginal zone (CMZ), which contributes to the postnatal growth of the retina, has been identified and characterized (Fischer and Reh, 2000; Miles and Tropepe, 2021). Previous studies have shown that the addition of new cells to the edge of the retina continues for at least 3 weeks after hatching, and after this time period, fewer cells are added to this region. These findings indicate that the proliferation of progenitor cells and the addition of new cells to the retina in chickens decrease with increasing age (Fischer and Reh, 2000; Fischer, 2005). Earlier studies have also shown that some HSP genes are essential for normal retinal development and functional maturation of retinal cells at the embryonic and adult stages (Kojima et al., 1996; Sakai et al., 2003; Lee et al., 2006; Zhao et al., 2006).
Consistent with the findings of previous studies, our results showed that the highest upregulation of HSP genes (HSP27, HSP60, and HSP70) was at the earliest developmental stages of the retina (at 14-days) when compared to other developmental stages (Figures 6, 8, 9 respectively). We hypothesize that this elevated expression may serve dual functions in the earliest developmental stages of the retina to not only protect existing cells from damage, but also to promote regeneration, development, and maturation of new retinal cells in the CMZ region.
In conclusion, the present study was a two-year study that provided reproducible results on the expression of HSP genes under chronic heat stress. To the best of our knowledge, this is the first study to report the expression levels of HSP27, HSP40, HSP60, HSP70, and HSP90 in the retina under chronic heat stress. Our data was consistent with the previously reported expression levels of some of these HSPs in other tissues, but not in the retina. The consistent increase in the expression of HSPs in the retina suggests their role as biomarkers for chronic heat stress. It is expected that the induction of HSPs in the retina during chronic heat stress is required to protect retinal cells from damage and degeneration. This study focused only on mRNA expression. Protein analysis is needed to confirm the function of HSPs in the retina under heat stress. Furthermore, it would be interesting to conduct the same study under acute heat stress and compare the expression of HSPs under both chronic and acute heat stress.
Data availability statement
The original contributions presented in the study are included in the article/Supplementary Material, further inquiries can be directed to the corresponding author.
Ethics statement
The animal study was reviewed and approved by Ethics Committee on Animal Use at Kuwait University and ECULA Ethical Committee for the Use of Laboratory Animals (DBS/IRB(ECULA)20-005).
Author contributions
NB was the primary investigator on the project. NB and TA contributed to conception and design of the study. TA and FA performed the experiments and the statistical analysis. NB prepared and wrote the first draft of the manuscript. TA and AA contributed to manuscript revision. All authors read and approved the submitted version.
Funding
This project was funded by the Research Sector at Kuwait University (Project SL01/20).
Acknowledgments
The authors acknowledge the General Facility Project (GS 01/ 02) at the Biotechnology Center of Kuwait University for the use of the ABI 3130xI Genetic Analyzer and Bio-Rad CFX96 Real-Time System and the General Facility Project (GS 03/01) at the Research Sector Project Unit for the use of liquid nitrogen generator. The authors would also like to acknowledge Zahraa Almomen for participating in sample collection and RNA extraction.
Conflict of interest
The authors declare that the research was conducted in the absence of any commercial or financial relationships that could be construed as a potential conflict of interest.
Publisher’s note
All claims expressed in this article are solely those of the authors and do not necessarily represent those of their affiliated organizations, or those of the publisher, the editors and the reviewers. Any product that may be evaluated in this article, or claim that may be made by its manufacturer, is not guaranteed or endorsed by the publisher.
References
Aguilà, M., and Cheetham, M. E. (2016). Hsp90 as a potential therapeutic target in retinal disease. Adv. Exp. Med. Biol. 854, 161–167. doi:10.1007/978-3-319-17121-0_22
Al-Awadhi, J. M., Al-Helal, A., and Al-Enezi, A. (2005). Sand drift potential in the desert of Kuwait. J. Arid. Environ. 63, 425–438. doi:10.1016/j.jaridenv.2005.03.011
Alahmad, B., Tomasso, L. P., Al-Hemoud, A., James, P., and Koutrakis, P. (2020). Spatial distribution of land surface temperatures in Kuwait: Urban heat and cool islands. Int. J. Environ. Res. Public Health. 17, 2993. doi:10.3390/ijerph17092993
Bellini, S., Barutta, F., Mastrocola, R., Imperatore, L., Bruno, G., and Gruden, G. (2017). Heat shock proteins in vascular diabetic complications: Review and future perspective. Int. J. Mol. Sci. 18, 2709. doi:10.3390/ijms18122709
Bernstein, S. L., Russell, P., Wong, P., Fishelevich, R., and Smith, L. E. (2001). Heat shock protein 90 in retinal ganglion cells: Association with axonally transported proteins. Vis. Neurosci. 18, 429–436. doi:10.1017/s0952523801183094
Bouchama, A., Aziz, M. A., Mahri, S. A., Gabere, M. N., Dlamy, M. A., Mohammad, S., et al. (2017). A model of exposure to extreme environmental heat uncovers the human transcriptome to heat stress. Sci. Rep. Sci. Rep. 7, 9429. doi:10.1038/s41598-017-09819-5
Bukau, B., Deuerling, E., Pfund, C., and Craig, E. A. (2000). Getting newly synthesized proteins into shape. Cell 101, 119–122. doi:10.1016/S0092-8674(00)80806-5
Cândido, M. G. L., Tinôco, I. F. F., Albino, L. F. T., Freitas, L. C. S. R., Santos, T. C., Cecon, P. R., et al. (2020). Effects of heat stress on pullet cloacal and body temperature. Poult. Sci. 99, 2469–2477. doi:10.1016/j.psj.2019.11.062
Caracausi, M., Piovesan, A., Antonaros, F., Strippoli, P., Vitale, L., and Pelleri, M. C. (2017). Systematic identification of human housekeeping genes possibly useful as references in gene expression studies. Mol. Med. Rep. 16, 2397–2410. doi:10.3892/mmr.2017.6944
Caruso Bavisotto, C., Alberti, G., Vitale, A. M., Paladino, L., Campanella, C., Rappa, F., et al. (2020). Hsp60 post-translational modifications: Functional and pathological consequences. Front. Mol. Biosci. 7, 95. doi:10.3389/fmolb.2020.00095
Cedraz, H., Gromboni, J. G. G., Garcia, A. A. P. J., Farias Filho, R. V., Souza, T. M., Oliveira, E. R., et al. (2017). Heat stress induces expression of HSP genes in genetically divergent chickens. PLOS ONE 12, e0186083. doi:10.1371/journal.pone.0186083
Chaudhury, S., Keegan, B. M., and Blagg, B. S. J. (2021). The role and therapeutic potential of Hsp90, Hsp70, and smaller heat shock proteins in peripheral and central neuropathies. Med. Res. Rev. 41, 202–222. doi:10.1002/med.21729
Cheng, Y., Sun, J., Chen, H., Adam, A., Tang, S., Kemper, N., et al. (2016). Expression and location of HSP60 and HSP10 in the heart tissue of heat-stressed rats. Exp. Ther. Med. 12, 2759–2765. doi:10.3892/etm.2016.3650
Chidlow, G., Wood, J. P., and Casson, R. J. (2014). Expression of inducible heat shock proteins Hsp27 and Hsp70 in the visual pathway of rats subjected to various models of retinal ganglion cell injury. PLOS ONE 9, e114838. doi:10.1371/journal.pone.0114838
Chien, C. C., Huang, C. J., Tien, L. T., Cheng, Y. C., Ke, C. Y., and Lee, Y. J. (2017). Suppression of HSP27 restores retinal function and protects photoreceptors from apoptosis in a light-induced retinal degeneration animal model. Invest. Ophthalmol. Vis. Sci. 58, 3107–3117. doi:10.1167/iovs.16-21007
Das, R., Sailo, L., Verma, N., Bharti, P., Saikia, J., Imtiwati, R., et al. (2016). Impact of heat stress on health and performance of dairy animals: A review. Vet. World. 9, 260–268. doi:10.14202/vetworld.2016.260-268
Dheda, K., Huggett, J. F., Bustin, S. A., Johnson, M. A., Rook, G., and Zumla, A. (2004). Validation of housekeeping genes for normalizing RNA expression in real-time PCR. BioTechniques 37, 112–114, 116, 118-119. doi:10.2144/04371RR03
Dubrez, L., Causse, S., Borges Bonan, N., Dumétier, B., and Garrido, C. (2020). Heat-shock proteins: Chaperoning DNA repair. Oncogene 39, 516–529. doi:10.1038/s41388-019-1016-y
Edmondson, D., Conroy, D., Romero-Canyas, R., Tanenbaum, M., and Czajkowski, S. (2022). Climate change, behavior change and health: A multidisciplinary, translational and multilevel perspective. Transl. Behav. Med. 12, 503–515. doi:10.1093/tbm/ibac030
Ferns, G., Shams, S., and Shafi, S. (2006). Heat shock protein 27: Its potential role in vascular disease. Int. J. Exp. Pathol. 87, 253–274. doi:10.1111/j.1365-2613.2006.00484.x
Fischer, A. J. (2005). Neural regeneration in the chick retina. Prog. Retin. Eye Res. 24, 161–182. doi:10.1016/j.preteyeres.2004.07.003
Fischer, A. J., and Reh, T. A. (2000). Identification of a proliferating marginal zone of retinal progenitors in postnatal chickens. Dev. Biol. 220, 197–210. doi:10.1006/dbio.2000.9640
Franklin, T. B., Krueger-Naug, A. M., Clarke, D. B., Arrigo, A. P., and Currie, R. W. (2005). The role of heat shock proteins Hsp70 and Hsp27 in cellular protection of the central nervous system. Int. J. Hyperth. 21, 379–392. doi:10.1080/02656730500069955
Fujikawa, C., Nagashima, M., Mawatari, K., and Kato, S. (2012). HSP70 gene expression in the zebrafish retina after optic nerve injury: A comparative study under heat shock stresses. Adv. Exp. Med. Biol. 723, 663–668. doi:10.1007/978-1-4614-0631-0_84
Gromboni, J. G. G., Cedraz de Oliveira, H., Botelho Diniz Marques, D., Amândio Pinto Garcia Junior, A., Vasconcelos Farias Filho, R., Fernando Gromboni, C., et al. (2020). Influence of heat stress on reference genes stability in heart and liver of two chickens genotypes. PLOS ONE 15, e0228314. doi:10.1371/journal.pone.0228314
Hoter, A., El-Sabban, M. E., and Naim, H. Y. (2018). The HSP90 family: Structure, regulation, function, and implications in health and disease. Int. J. Mol. Sci. 19, 2560. doi:10.3390/ijms19092560
Jo, D. H., An, H., Chang, D. J., Baek, Y. Y., Cho, C. S., Jun, H. O., et al. (2014). Hypoxia-mediated retinal neovascularization and vascular leakage in diabetic retina is suppressed by HIF-1α destabilization by SH-1242 and SH-1280, novel hsp90 inhibitors. J. Mol. Med. (Berl.) 92, 1083–1092. doi:10.1007/s00109-014-1168-8
Kanamaru, C., Yamada, Y., Hayashi, S., Matsushita, T., Suda, A., Nagayasu, M., et al. (2014). Retinal toxicity induced by small-molecule Hsp90 inhibitors in beagle dogs. J. Toxicol. Sci. 39, 59–69. doi:10.2131/jts.39.59
Kojima, M., Hoshimaru, M., Aoki, T., Takahashi, J. B., Ohtsuka, T., Asahi, M., et al. (1996). Expression of heat shock proteins in the developing rat retina. Neurosci. Lett. 205, 215–217. doi:10.1016/0304-3940(96)12406-x
Lackie, R. E., Maciejewski, A., Ostapchenko, V. G., Marques-Lopes, J., Choy, W. Y., Duennwald, M. L., et al. (2017). The Hsp70/Hsp90 chaperone machinery in neurodegenerative diseases. Front. Neurosci. 11, 254. doi:10.3389/fnins.2017.00254
Lee, J., Kim, H., Lee, J. M., and Shin, T. (2006). Immunohistochemical localization of heat shock protein 27 in the retina of pigs. Neurosci. Lett. 406, 227–231. doi:10.1016/j.neulet.2006.07.067
Lei, L., Yu, J., and Bao, E. (2009). Expression of heat shock protein 90 (Hsp90) and transcription of its corresponding mRNA in broilers exposed to high temperature. Br. Poult. Sci. 50, 504–511. doi:10.1080/00071660903110851
Li, J., Qian, X., and Sha, B. (2009). Heat shock protein 40: Structural studies and their functional implications. Protein Pept. Lett. 16, 606–612. doi:10.2174/092986609788490159
Meng, Q., Li, B. X., and Xiao, X. (2018). Toward developing chemical modulators of Hsp60 as potential therapeutics. Front. Mol. Biosci. 5, 35. doi:10.3389/fmolb.2018.00035
Miles, A., and Tropepe, V. (2021). Retinal stem cell ‘retirement plans’: Growth, regulation and species adaptations in the retinal ciliary marginal zone. Int. J. Mol. Sci. 22, 6528. doi:10.3390/ijms22126528
Mohammad, G., and Kowluru, R. A. (2011). Novel role of mitochondrial matrix metalloproteinase-2 in the development of diabetic retinopathy. Invest. Ophthalmol. Vis. Sci. 52, 3832–3841. doi:10.1167/iovs.10-6368
Murugesan, S., Ullengala, R., and Amirthalingam, V. (2017). “Heat shock protein and thermal stress in chicken,” in Heat shock proteins in veterinary medicine and sciences. Editors A. A. A. Asea, and P. Kaur (New York: Springer), 179–193. doi:10.1007/978-3-319-73377-7_6
Nasrallah, H. A., Nieplova, E., and Ramadan, E. (2004). Warm season extreme temperature events in Kuwait. J. Arid. Environ. 56, 357–371. doi:10.1016/S0140-1963(03)00007-7
National Research Council, (1996). Guide for the Care and Use of Laboratory Animals. Washington DC, USA: The National Academies Press.
Nawaz, A. H., Amoah, K., Leng, Q. Y., Zheng, J. H., Zhang, W. L., and Zhang, L. (2021). Poultry response to heat stress: Its physiological, metabolic, and genetic implications on meat production and quality including strategies to improve broiler production in a warming world. Front. Vet. Sci. 8, 699081. doi:10.3389/fvets.2021.699081
Olcina, G., Crespo, C., Timón, R., Mjaanes, J. M., and Calleja-González, J. (2019). Core temperature response during the marathon portion of the ironman world championship (Kona-Hawaii). Front. Physiol. 10, 1469. doi:10.3389/fphys.2019.01469
Oshlag, J. Z., Devasthanam, A. S., and Tomasi, T. B. (2013). Mild hyperthermia enhances the expression and induces oscillations in the Dicer protein. Int. J. Hyperth. 29, 51–61. doi:10.3109/02656736.2012.753471
Parida, S., Mishra, S. R., Mishra, C., Dalai, N., Mohapatra, S., Mahapatra, A. P. K., et al. (2020). Impact of heat stress on expression kinetics of HSP27 in cardiac cells of goats. Biol. Rhythm Res. 51, 925–933. doi:10.1080/09291016.2018.1564578
Pinach, S., Burt, D., Berrone, E., Barutta, F., Bruno, G., Porta, M., et al. (2013). Retinal heat shock protein 25 in early experimental diabetes. Acta Diabetol. 50, 579–585. doi:10.1007/s00592-011-0346-1
Piri, N., Kwong, J. M., Gu, L., and Caprioli, J. (2016). Heat shock proteins in the retina: Focus on HSP70 and alpha crystallins in ganglion cell survival. Prog. Retin. Eye Res. 52, 22–46. doi:10.1016/j.preteyeres.2016.03.001
Radons, J. (2016). The human HSP70 family of chaperones: Where do we stand? Cell Stress Chaperones 21, 379–404. doi:10.1007/s12192-016-0676-6
Rauch, J. N., and Gestwicki, J. E. (2014). Binding of human nucleotide exchange factors to heat shock protein 70 (Hsp70) generates functionally distinct complexes in vitro. J. Biol. Chem. 289, 1402–1414. doi:10.1074/jbc.M113.521997
Reddy, V. S., Raghu, G., Reddy, S. S., Pasupulati, A. K., Suryanarayana, P., and Reddy, G. B. (2013). Response of small heat shock proteins in diabetic rat retina. Invest. Ophthalmol. Vis. Sci. 54, 7674–7682. doi:10.1167/iovs.13-12715
Retzlaff, M., Stahl, M., Eberl, H. C., Lagleder, S., Beck, J., Kessler, H., et al. (2009). Hsp90 is regulated by a switch point in the C-terminal domain. EMBO Rep. 10, 1147–1153. doi:10.1038/embor.2009.153
Rogalla, T., Ehrnsperger, M., Preville, X., Kotlyarov, A., Lutsch, G., Ducasse, C., et al. (1999). Regulation of Hsp27 oligomerization, chaperone function, and protective activity against oxidative stress/tumor necrosis factor alpha by phosphorylation. J. Biol. Chem. 274, 18947–18956. doi:10.1074/jbc.274.27.18947
Sakai, M., Sakai, H., Nakamura, Y., Fukuchi, T., and Sawaguchi, S. (2003). Immunolocalization of heat shock proteins in the retina of normal monkey eyes and monkey eyes with laser-induced glaucoma. Jpn. J. Ophthalmol. 47, 42–52. doi:10.1016/s0021-5155(02)00627-5
Sedaghat, A., Sabati, M., Alkhatib, F., Amir Abbas Oloomi, S., Sabri, F., Salem, H., et al. (2021). Climate change and thermo-solar patterns of office buildings with/without window films in extreme hot-arid climate of Kuwait. J. Sol. Energy. 217, 354–374. doi:10.1016/j.solener.2021.02.051
Serrano, C., Bolea, R., Lyahyai, J., Filali, H., Varona, L., Marcos-Carcavilla, A., et al. (2011). Changes in HSP gene and protein expression in natural scrapie with brain damage. Vet. Res. 42, 13. doi:10.1186/1297-9716-42-13
Shehata, A. M., Saadeldin, I. M., Tukur, H. A., and Habashy, W. S. (2020). Modulation of heat-shock proteins mediates chicken cell survival against thermal stress. Anim. (Basel) 10, 2407. doi:10.3390/ani10122407
Siddiqui, S. H., Kang, D., Park, J., Khan, M., and Shim, K. (2020a). Chronic heat stress regulates the relation between heat shock protein and immunity in broiler small intestine. Sci. Rep. Sci. Rep. 10, 18872. doi:10.1038/s41598-020-75885-x
Siddiqui, H. S., Kang, D., Park, J., Choi, H. W., and Shim, K. (2020b). Acute heat stress induces the differential expression of heat shock proteins in different sections of the small intestine of chickens based on exposure duration. Anim. (Basel) 10, 1234. doi:10.3390/ani10071234
Sikand, K., Singh, J., Ebron, J. S., and Shukla, G. C. (2012). Housekeeping gene selection advisory: glyceraldehyde-3-phosphate dehydrogenase (GAPDH) and β-actin are targets of miR-644a. PLOS ONE 7, e47510. doi:10.1371/journal.pone.0047510
Staib, J. L., Quindry, J. C., French, J. P., Criswell, D. S., and Powers, S. K. (2007). Increased temperature, not cardiac load, activates heat shock transcription factor 1 and heat shock protein 72 expression in the heart. Am. J. Physiol. Regul. Integr. Comp. Physiol. 292, R432–R439. doi:10.1152/ajpregu.00895.2005
Tang, S., Buriro, R., Liu, Z., Zhang, M., Ali, I., Adam, A., et al. (2013). Localization and expression of Hsp27 and αB-crystallin in rat primary myocardial cells during heat stress in vitro. PLOS ONE 8, e69066. doi:10.1371/journal.pone.0069066
Tang, S., Lv, Y., Chen, H., Adam, A., Cheng, Y., Hartung, J., et al. (2014). Comparative analysis of αB-crystallin expression in heat-stressed myocardial cells in vivo and in vitro. PLOS ONE 9, e86937. doi:10.1371/journal.pone.0086937
Temim, S., Chagneau, A. M., Peresson, R., and Tesseraud, S. (2000). Chronic heat exposure alters protein turnover of three different skeletal muscles in finishing broiler chickens fed 20 or 25% protein diets. J. Nutr. 130, 813–819. doi:10.1093/jn/130.4.813
Tsai, T., Grotegut, P., Reinehr, S., and Joachim, S. C. (2019). Role of heat shock proteins in glaucoma. Int. J. Mol. Sci. 20, 5160. doi:10.3390/ijms20205160
Tytell, M., Barbe, M. F., and Brown, I. R. (1994). Induction of heat shock (stress) protein 70 and its mRNA in the normal and light-damaged rat retina after whole body hyperthermia. J. Neurosci. Res. 38, 19–31. doi:10.1002/jnr.490380105
van Wijngaarden, P., Brereton, H. M., Coster, D. J., and Williams, K. A. (2007). Stability of housekeeping gene expression in the rat retina during exposure to cyclic hyperoxia. Mol. Vis. 13, 1508–1515.
Varasteh, S., Braber, S., Akbari, P., Garssen, J., and Fink-Gremmels, J. (2015). Differences in susceptibility to heat stress along the chicken intestine and the protective effects of galacto-oligosaccharides. PLOS ONE 10, e0138975. doi:10.1371/journal.pone.0138975
Vidyasagar, A., Wilson, N. A., and Djamali, A. (2012). Heat shock protein 27 (HSP27): Biomarker of disease and therapeutic target. Fibrogenes. Tissue Repair 5, 7. doi:10.1186/1755-1536-5-7
Whitlock, N. A., Agarwal, N., Ma, J. X., and Crosson, C. E. (2005). Hsp27 upregulation by HIF-1 signaling offers protection against retinal ischemia in rats. Invest. Ophthalmol. Vis. Sci. 46, 1092–1098. doi:10.1167/iovs.04-0043
Xie, J., Tang, L., Lu, L., Zhang, L., Xi, L., Liu, H. C., et al. (2014). Differential expression of heat shock transcription factors and heat shock proteins after acute and chronic heat stress in laying chickens (Gallus gallus). PLOS ONE 9, e102204. doi:10.1371/journal.pone.0102204
Yamagata, M., Yan, W., and Sanes, J. R. (2021). A cell atlas of the chick retina based on single-cell transcriptomics. eLife 10, e63907. doi:10.7554/eLife.63907
Yan, Z., Wei, H., Ren, C., Yuan, S., Fu, H., Lv, Y., et al. (2015). Gene expression of Hsps in normal and abnormal embryonic development of mouse hindlimbs. Hum. Exp. Toxicol. 34, 563–574. doi:10.1177/0960327114555927
Yu, J., Bao, E., Yan, J., and Lei, L. (2008). Expression and localization of Hsps in the heart and blood vessel of heat-stressed broilers. Cell Stress Chaperones 13, 327–335. doi:10.1007/s12192-008-0031-7
Zhao, F. Q., Zhang, Z. W., Wang, C., Zhang, B., Yao, H. D., Li, S., et al. (2013). The role of heat shock proteins in inflammatory injury induced by cold stress in chicken hearts. Cell Stress Chaperones 18, 773–783. doi:10.1007/s12192-013-0429-8
Zhao, J., Yoneda, M., Inoue, Y., Kakizaki, H., Ohno-Jinno, A., Kataoka, T., et al. (2006). Expression profile of heat shock protein 108 during retinal development in the chick. Neurosci. Lett. 397, 10–14. doi:10.1016/j.neulet.2005.11.045
Keywords: chronic heat stress, HSP genes, chicken, retina, RT-qPCR
Citation: Bastaki NK, Albarjas TA, Almoosa FA and Al-Adsani AM (2023) Chronic heat stress induces the expression of HSP genes in the retina of chickens (Gallus gallus). Front. Genet. 14:1085590. doi: 10.3389/fgene.2023.1085590
Received: 09 December 2022; Accepted: 24 March 2023;
Published: 03 April 2023.
Edited by:
Giuseppe Passarino, University of Calabria, ItalyReviewed by:
Pietro Gareri, Dipartimento delle Cure Primarie, ASP Catanzaro, ItalyPaolina Crocco, University of Calabria, Italy
Copyright © 2023 Bastaki, Albarjas, Almoosa and Al-Adsani. This is an open-access article distributed under the terms of the Creative Commons Attribution License (CC BY). The use, distribution or reproduction in other forums is permitted, provided the original author(s) and the copyright owner(s) are credited and that the original publication in this journal is cited, in accordance with accepted academic practice. No use, distribution or reproduction is permitted which does not comply with these terms.
*Correspondence: Nasmah K. Bastaki, bmFzbWFoLmJhc3Rha2lAa3UuZWR1Lmt3