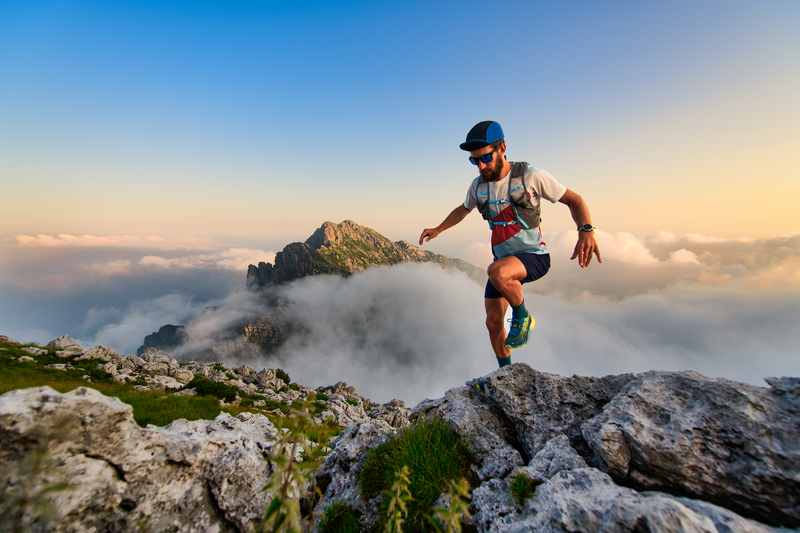
95% of researchers rate our articles as excellent or good
Learn more about the work of our research integrity team to safeguard the quality of each article we publish.
Find out more
ORIGINAL RESEARCH article
Front. Genet. , 23 January 2023
Sec. Evolutionary and Genomic Microbiology
Volume 14 - 2023 | https://doi.org/10.3389/fgene.2023.1009746
Sterol 14α-demethylase catalyzes lanosterol hydroxylation, which is one of the key reactions in the biosynthetic pathway of sterols. There is only one sterol 14α-demethylases gene named Erg11 in Saccharomyces cerevisiae genome. In this study, three sterol 14α-demethylases genes named AoErg11A, AoErg11B and AoErg11C were identified in Aspergillus oryzae genome through bioinformatics analysis. The function of these three genes were studied by yeast complementation, and the expression pattern/subcellular localization of these genes/proteins were detected. The results showed that the three AoErg11s were expressed differently at different growth times and under different abiotic stresses. All of the three proteins were located in endoplasmic reticulum. The AoErg11s could not restore the temperature-sensitive phenotype of S. cerevisiae erg11 mutant. Overexpression of the three AoErg11s affected both growth and sporulation, which may be due to the effect of AoErg11s on ergosterol content. Therefore, this study revealed the functions of three AoErg11s and their effects on the growth and ergosterol biosynthesis of A. oryzae, which may contribute to the further understanding of the ergosterol biosynthesis and regulation mechanism in this important filamentous fungus, A. oryzae.
Cytochrome P450 (CYP450), a class of monooxygenases, is a large family of self-oxidizing ferrous heme proteins (Rudolf et al., 2017) and it is named for its unique absorption peak at 450 nm when it binds with CO (Luthra et al., 2011). CYP450 was first discovered in the microsomes of rat liver cells in 1958 (Klingenberg, 1958). In recent years, researchers revealed that CYP450 widely exists in animals, plants and microorganisms (Elfaki et al., 2018), and it is one of the most abundant catalytic enzymes with the widest substrate spectrum in nature. CYP450 participates in the metabolism of endogenous and exogenous substances, including drugs and environmental compounds (Gao et al., 2017; Neunzig and Bernhardt, 2018) and has important effects on cytokines and thermoregulation (Ruparel et al., 2012). CYP450 is mainly distributed in endoplasmic reticulum (ER) and mitochondrial intima (El-Sherbeni and El-Kadi, 2014). As a terminal oxygenase, CYP450 participates in sterol biosynthesis and other processes in vivo.
Sterol 14α-demethylase (also named P45014DM, CYP51 or ERG11) belongs to the CYP450 superfamily and it is the only P450 enzyme that existed in all organisms including bacteria, fungi, lower eukaryotes, higher plants and mammals (Ghosh, 2017). CYP51 is a very important rate-limiting gene in S. cerevisiae, and a key enzyme in the sterol biosynthesis pathway (Jordá and Puig, 2020), catalyzing the substrate 14α-methyl hydroxylation to biosynthesis of sterol precursor (Ogris et al., 2021). Generally, the reaction is reported to occur in the ER; however, it is also reported this reaction might take place in mitochondria in Kinetoplastidae (Peña-Diaz et al., 2004). CYP51 is one of the key enzymes in the sterol biosynthesis pathway and final products of the pathway were different in different eukaryotes, such as cholesterol in animals, phytosterols in plants, and ergosterol in fungi. In most situations, sterols are important components of the plasma membrane or precursor of hormone (Zhang et al., 2019). Therefore, lacking this gene causes damage to membrane structure or function, which may eventually lead to the death of fungal, or affect plant growth and development process, or reducing animal the endogenous cholesterol synthesis, etc. Thus, CYP51 became important target for many antifungal drugs, herbicides and cholesterol-lowering drugs (Lepesheva and Waterman, 2011; Zhang et al., 2019; Kaluzhskiy et al., 2021).
CYP51 was first purified from S. cerevisiae in 1984 (Yoshida and Aoyama, 1984). As it is an essential enzyme for ergosterol biosynthesis in fungus, the encoding gene was also named Erg11. Studies have shown that CYP51 is essential for the growth and development of animals, plants, fungi, etc. In mammals, it is a membrane-bound protein in liver microsomes that plays a crucial role in lipid metabolism, hormone synthesis and detoxification. For example, in mouse, inactivation of the liver CYP51 enzyme leads to the accumulation of lanosterol and 24, 25-dehydrolanosterol, causing progressive liver pathology (Lorbek et al., 2015; Urlep et al., 2017). Besides, CYP51 is also important for mammalian reproduction as sterols are required for activation of oocyte meiosis, and lanosterol 14α-demethylase (FF-MAS) and sterol 14-reductase (T-MAS) were able to reactivate meiosis in mouse oocytes in vitro (Byskov et al., 1995). In plants, CYP51 is essential for regulating plant growth and development. For example, Arabidopsis thaliana genome contains two cyp51 genes, cyp51A1 and cyp51A2. The cyp51A1 is expressed in root tissue, while cyp51A2 is ubiquitously expressed. Deletion of cyp51A2 showed various defects, such as hypocotyl dysplasia, short root, reduced cell elongation and seedling mortality (Kim et al., 2005). Besides, cyp51A2 mutants also show a defective stomatal development phenotype (Qian et al., 2013). In fungi genome, including S. cerevisiae, Candida albicans or Cryptococcus neoformans, it contains only one CYP51 gene. It has been shown that CYP51 gene is necessary for aerobic viability of yeast (Bard et al., 1993; Geber et al., 1995; Sanglard et al., 2003; Revankar et al., 2004). Most filamentous fungi contain multiple copies of CYP51 genes, and these genes usually showed functional redundancy. The deletion of all these CYP51 genes was fatal for the survival of the cell. For example, Aspergillus fumigatus genome contains two CYP51 genes named CYP51A and CYP51B. The single deletion mutant of these two genes showed no significant growth defects, while simultaneous deletion of both CYP51 genes is lethal (Roundtree et al., 2020). Moreover, CYP51A and CYP51B proteins are functionally compensatory. The expression of CYP51A was increased when CYP51B was absent, and vice versa (Hu et al., 2007). Similarly, Magnaporthe oryzae genome contains two CYP51 genes, CYP51A and CYP51B. Single deletion strains of CYP51A or CYP51B showed no differences in morphology from wild-type strains on CM medium, while simultaneous knockout of both genes is lethal (Yan et al., 2011). It is also revealed that the expression level of CYP51A is significantly increased in CYP51B mutant. Unlike the previous two, Aspergillus flavus genome contains three CYP51 genes, CYP51A, CYP51B and CYP51C, which CYP51A and CYP51B are the major expressed genes for 14α-demethylase activity, since CYP51C basal expression is very low or undetectable (Paul et al., 2018). CYP51A is the main gene responsible for drug resistance (Lucio et al., 2020), and CYP51B is a functionally redundant gene. Some authors have argued that CYP51C originated from the duplications of both genes, CYP51A or CYP51B depending on the species (Pérez-Cantero et al., 2020), and have proposed that CYP51 duplications are derived from an evolutionary mechanism controlling adaptation to azole toxicity (Hawkins et al., 2014; Dudakova et al., 2017).
However, the function of the CYP51/Erg11 (hereafter named Erg11) gene in A. oryzae, one of the most important filamentous fungi in industry, has been poorly studied. A. oryzae is a filamentous fungus approved by FDA and WHO for safe production. It has long been used not only in traditional food fermentation, brewing and condiment industries but also in modern biotechnology industries such as enzyme preparation and recombinant protein production (Merz et al., 2015; Wang et al., 2021). Unlike reported in yeast, our previous bioinformatics studies have shown that there are three genes encoding Erg11 in A. oryzae genome (Hu et al., 2019). However, the function of these three AoErg11s remains unclear. This study investigated the function of the three genes by yeast complementation and examined the expression patterns/subcellular localization of these AoErg11s encoding genes/proteins. Finally, the influence of overexpression of these AoErg11s on ergosterol synthesis was also examined. This study revealed the function of three Erg11s in A. oryzae and their effect on growth and ergosterol biosynthesis.
Neighbor-joining method was used to create the unrooted tree using MEGA-X, and MEME program was used to identify the conserved motifs of all proteins. The amino acid sequence used is as follows, sterol 14α-demethylase: A. flavus [KOC13200.1], A. flavus [KOC15064.1], A. flavus [KOC13803.1], A. niger (XP_001394224.1), A. niger (XP_001396151.2), A. nidulans (XP_659505.1), A. nidulans (XP_681552.1), S. cerevisiae (NP_011871.1), C. albicans [ADI76627.1], H. sapiens [BAG36881.1], C. imitator (XP_017392004.1), M. musculus (NP_064394.2), A. thaliana [OAP10887.1], A. thaliana (NP_172633.1), O. sativa (XP_015617432.1), Z. mays [PWA33212.1]. The accession numbers of A. oryzae sterol 14α-demethylase, AoErg11A–AoErg11C, are as follows: EIT83124.1, EIT73378.1 and EIT72345.1. The accession numbers are in parentheses.
The wild-type strain (A. oryzae 3.042 (CICC 40092)) obtained from China Center of Industry Culture Collection (Beijing, China) and the uridine/uracil auxotrophic (ΔpyrG) A. oryzae 3.042 strain constructed in our laboratory (Sun et al., 2019a) were used in this study. CD medium (2% glucose, 0.2% NaNO3, 0.1% KH2PO4, 0.05% MgSO4, 0.05% NaCl, 0.05% KCl, 0.002% FeSO4, 1.5% agar, pH 5.5) supplemented with uracil and uridine was used to collect A. oryzae 3.042 ΔpyrG conidia suspensions for Agrobacterium-mediated transformation. A. oryzae was cultured at 30°C for 72 h except where otherwise mentioned. The plasmid constructed with Escherichia coli DH5α was transformed into A. oryzae using Agrobacterium tumefaciens AGL1. Both E. coli and A. tumefaciens were cultured in Luria Bertani (LB) medium supplemented with appropriate antibiotics at 37°C and 28°C, respectively. All strains used in this study are shown in Table 1, and all plasmids used in this study are shown in Table 2.
The mycelia at different growth times or under different stress conditions were frozen in liquid nitrogen and crushed immediately after harvest. Total RNA was extracted using a fungal RNA kit (Omega Bio-tek, Norcross, GA, United States) and the cDNAs were synthesized using the Prime Script™ RT reagent kit (Perfect Real Time; Takara). The quality and concentration were determined using a NanoDrop ND-2000 spectrophotometer (Thermo Scientific, Wilmington, DE, United States). All qRT–PCR (quantitative reverse transcription-PCR) operations were performed using SYBR Premix Ex Taq (Takara, Japan) and CFX96 real-time PCR detection system (Bio-Rad, CA, United States). All experiments were repeated three times and the average was taken to calculate gene expression. The housekeeping gene encoding histone H4 as a normalization control (Maruyama et al., 2002), and the relative expression was calculated according to formula 2−ΔΔCT. The sequences of the primers used for qRT–PCR are shown in Table 3.
The erg11 mutant (Y40597) was purchased from EUROSCARF (http://www.euroscarf.de/index.php) and the BY4741 was used as wild-type control. The pYES2.0 vector (Rojas et al., 2011) with PGAL1 as promoter was used for yeast complementation. Using a one-step cloning kit (Vazyme Biotech Co., Ltd., China), the full-length coding sequence (CDS) of AoErg11s and AoErg11ΔSPs were fused into pYES2.0 vector digested with HindIII and EcoRI. Then, the constructed vectors were transformed into corresponding yeast mutants using yeast transformation kit II (Coolaber, Beijing, China). The erg11 mutants were randomly selected and identified by PCR using S. cerevisiae and A. oryzae ScErg11/AoErg11s-specific primer pairs (primer sequences are listed in Table 4). In temperature-sensitive tests, the control and transformants were grown on YPD (1% yeast extract, 2% peptone, 2% glucose, 1% AGAR) and YPG (1% yeast extract, 2% peptone, 2% galactose, 1% AGAR), respectively, and the phenotypes were evaluated at 30°C and 37°C. In addition, the control and transformants cultured in liquid YPD and liquid YPG for 2 days at 30°C were collected to determine the content of ergosterol.
All the gene overexpression experiments were performed using pEX2B (Nguyen et al., 2017), a binary vector with PamyB as the promoter. To construct the pEX2B-AoErg11s-DsRed and pEX2B-AoErg11ΔSPs-DsRed vectors, the CDS of AoErg11s and AoErg11ΔSPs were cloned into pEX2B linearized with AflII. There was only one enzyme restriction site EcoRV between the target gene and DsRed, encoding aspartic acid and isoleucine. The primers used in this study are listed in Table 4. All constructed vectors were transformed into A. tumefaciens AGL1. Then, the vectors were transformed into A. oryzae 3.042 ΔpyrG as previously reported (Sun et al., 2019a). At least three individual strains of each transformant were collected to determine the stability of the phenotype, and one of them was selected for the statistical data of three growth experiments. CD medium (with maltose), PDA medium (2% maltose, 20% potatoes, 1.5% agar) and DPY (2% maltose, 1% peptone, 0.5% Yeast Extract, 0.5% KH2PO4, 0.05% MgSO4, 1.5% agar, pH 5.5) was used to culture transformants for phenotypic analysis. Mycelia cultured with DPY medium were collected to determination of ergosterol content.
The AoErg11A-AoErg11C protein localization was predicted by iPSORT prediction website. The constructed pEX2B-AoErg11s-DsRed plasmid were transformed into A. oryzae 3.042 ΔpyrG for subcellular localization. The pEX2B vector with DsRed as the reporter gene was used as the control. ER localization protein AoClxA (Watanabe et al., 2007) and mitochondrial localization signal (MTS) (Mabashi et al., 2006) were used as ER localization markers and mitochondrial localization markers. pEX1-ptrA-GFP (Nguyen et al., 2017), with PgpdA as the promoter and GFP as the reporter gene, was used as the co-transformation vector. Then AoClxA and MTS were fused into the vector linearized with XhoI, to construct pEX1-ptrA-ClxA-GFP and pEX1-ptrA-MTS-GFP vectors. There was only one enzyme restriction site SnaBI between the target gene and GFP, encoding glycine and threonine. To study co-localization, GFP vector was transformed into pEX2B-AoErg11s-DsRed transformation strain. After the co-transformed strains were obtained, at least three strains were selected for observation, and the most obvious one was selected and cultured on CD medium (with maltose) for 72 h, and the mycelia were observed under 100 x oil microscope with Leica DM4000B microscope (GFP and DsRed fluorescent filter cube, 100 magnification). Primer sequences used to construct plastids are shown in Table 4.
The ergosterol extraction and determination were performed according to previously described methods (Huang et al., 2022). A. oryzae mycelia for 72 h were collected and freeze-dried in vacuum to constant weight, and then the mycelia were crushed into powder. Ergosterol was extracted with 50 mg dry powder, and 3 mL ethanolic potassium hydroxide (25 g KOH +35 mL ddH2O, 100% ethanol constant volume to 100 mL) was added to A. oryzae powder and vortexed for 1 min. Then incubated at 85°C in a water bath for 1.5 h. After cooling to room temperature, 3 mL of n-heptane (Sigma-Aldrich, St. Louis, MO, United States) and 1 mL of distilled water were added and vortexed for 3 min. The upper layer (n-heptane layer) was separated and stored at 20°C for 24 h before analysis by high performance liquid chromatography (HPLC). HPLC was performed with a Waters Alliance E2695-2489 UV/Vis detector HPLC (Milford, MA, United States) with the UV detector set at 282 nm on a Zorbax SB-C18 column. Methanol/water (95:5, V/V) was used as the mobile phase, and the elution rate was 1.5 mL/min. Ergosterol (Sigma-Aldrich) was used to calibrate the curves. Each experiment was repeated three times.
BLAST analysis of corresponding homologous proteins in A. oryzae were carried out in NCBI (http://www.ncbi.nlm.nih.gov/) by using S. cerevisiae Erg11p sequence as query condition and three homologous proteins (named AoErg11A–AoErg11C) were identified. The AoErg11A protein length is 524 amino acids, while AoErg11B and AoErg11C are both 513 amino acids. To obtain more information about these three AoErg11s, phylogenetic analyses of Erg11 in different organisms were conducted. As shown in Figure 1, the evolution of Erg11 in fungus, animals and plants is relative conserved. In fungus S. cerevisiae and C. albicans genome harbor only one Erg11, A. nidulans and A. niger harbor two Erg11, and A. flavus and A. oryzae harbor three Erg11 (Figure 1A). Motif analysis also showed that all of these Erg11s contain six conserved motifs (Figure 1B). Thus, the Erg11 is evolutionarily conserved across plants, animals and fungi.
FIGURE 1. Phylogenetic analysis and functional motifs prediction of Erg11s in different species. (A) Unrooted phylogenetic tree of Erg11s and homologous proteins in A. nidulans, A. niger, A. flavus, S. cerevisiae, C. albicans, H. sapiens, C. imitator, M. musculus, A. thaliana, O. sativa and Z. mays. The IDs of the sequences were included after the specie names in the figure. (B) All conserved motifs of the Erg11s were identified by the MEME program. Protein sequences are indicated by thin black line, and the conserved motifs are represented by different colored boxes.
To investigate the role of AoErg11s in A. oryzae growth, qRT–PCR was used to determine the expression pattern of AoErg11s at different growth times or under different growth conditions. As shown in Figure 2A, under normal conditions, the expression levels of AoErg11s differed at different growth times, and AoErg11B was the main expressed gene. The expression of AoErg11A at 48 h was 1/3 of that in 24 h; and at 72 h, it was 1.5 times of that in 24 h. The expression of AoErg11B showed high expression levels at 24 h and 72 h (about 10 times to that of AoErg11A) and relative low level at 48 h (about 1/5 of 24 h or 72 h). The expression of AoErg11C were very low at 24 h and 72 h: about 1/2000 and 1/1200 to the corresponding AoErg11A, while it almost showed no expression at 48 h.
FIGURE 2. Expression levels of AoErg11A–AoErg11C at different growth times and under different abiotic stresses on CD medium. (A) Expression of AoErg11A–AoErg11C at 24, 48, and 72 h of growth; (B–D) Expression of AoErg11A–AoErg11C under temperature, salt and ethanol (EtOH) stress conditions. Wild-type A. oryzae spores were cultured in CD agar medium alone or CD agar medium supplemented with NaCl or ethanol at 30°C (except for temperature stress). The mycelia were harvested at 24, 48 and 72 h to determine the mRNA levels of AoErg11A–AoErg11C at different growth times. For other stress experiments, mycelia were collected at 72 h. The expression levels of corresponding genes at 24 h, 30°C and 0% NaCl/ethanol was used as control. Values represent the mean ± SD of three independent experiments. Statistical analyses were performed by t-test of GraphPad (*, p < 0.05; **, p < 0.01). For each experimental group, the relative expression level was compared to the corresponding control.
Ergosterol has been reported to participate in the stress response in S. cerevisiae (Kodedová and Sychrová, 2015), therefore we also investigated the expression of AoErg11s under different stress conditions. A. oryzae was stressed by temperature, salt and ethanol. As shown in Figure 2B, under temperature stress, the expression levels of AoErg11A gradually increased as temperature increased from 22°C to 42°C. The expression levels of AoErg11B were almost the same at 30°C and 42°C, but decreased significantly at 22°C and 37°C, which was about 1/10 of that at 30°C. The expression of AoErg11C was tripled at 22°C and 37°C compared with 30°C, and it almost showed no expression under 42°C. Under salt treatment, the expression levels of AoErg11A were relative stable, but its expression level reduced to 40% of the control under 10% NaCl (Figure 2C). On the contrary, the expression levels of AoErg11B were significantly decreased (about 1/52, 1/37 and 1/35 of the control respectively) by salt treatment (Figure 2C). The expression levels of AoErg11C were about the same under 0%, 10% and 15% NaCl, while its expression level increased to about 4 times of the control under 5% NaCl (Figure 2C). Under ethanol stress, the expression of AoErg11A decreased by about half under 2% ethanol stress, while increased to over 2 times of the control under 4% ethanol stress. The expression of AoErg11B decreased to 8% and 40% of the control under 2% and 4% ethanol stress (Figure 2D). On the contrary, the expression of AoErg11C increased 50% under 2% ethanol stress and decreased 80% under 4% ethanol stress compared with the control (Figure 2D). In conclusion, A. oryzae possesses three Erg11 genes, and the expression of these three genes are differed at different growth times and under different abiotic stresses.
Sterol 14α-demethylase in S. cerevisiae has been reported to be localized in the ER (Jordá and Puig, 2020). However, studies on the subcellular localization of Erg11 homologues in filamentous fungal cells are limited. Bioinformatics prediction analysis showed that there were plant mitochondrial targeted amino acid sequences (MTS) in the N-terminus of AoErg11A, and signal peptide in the N-terminus of AoErg11B and AoErg11C by using iPSORT Prediction website (https://ipsort.hgc.jp/index.html) (Supplementary Figure S1). Therefore, DsRed or GFP was used as reporter protein to investigate the subcellular localization of AoErg11s in A. oryzae. The DsRed gene was fused into the C-terminal of AoErg11s, and the overexpression vectors were constructed with uridine/uracil auxotrophic as the selective marker. Meanwhile, ER (AoClxA-GFP)- and mitochondrial (MTS-GFP)-targeted GFP vectors were constructed with pyrithiamine as selective markers, as reported previously (Sun et al., 2019b). The AoErg11s overexpression strains were co-transformed with ER-targeted GFP vector and mitochondria-targeted GFP vector, respectively. Results showed that the fluorescence of AoErg11s overexpression strains had typical ring structures and were consistent with the ER-located GFP marker but not with mitochondria-located GFP marker (Figure 3A and Supplementary Figure S2). In addition, the signal peptides of AoErg11A, AoErg11B and AoErg11C were deleted to construct AoErg11AΔSP-DsRed, AoErg11BΔSP-DsRed and AoErg11CΔSP-DsRed vectors. Microscopic examination showed that red fluorescence signals of AoErg11ΔSPs overexpression strains were distributed in the cytoplasm uniformity (Figure 3B). Therefore, we concluded that AoErg11A–AoErg11C located in the ER mediated by their signal peptides, and the localization signal of the N-terminal of the protein is related to the localization of these proteins.
FIGURE 3. Subcellular localization of AoErg11s. (A) Co-localization of AoErg11s-DsRed with endoplasmic reticulum. The mycelia of A. oryzae 3.042 ΔpyrG were co-transformed with AoErg11s-DsRed and AoClxA-GFP vectors. Left to right: phase contrast, fluorescent image of DsRed, GFP, and merged image of DsRed, GFP and phase contrast. (B) The mycelium of A. oryzae 3.042 ΔpyrG transformed with AoErg11ΔSPs-DsRed. Left to right: phase contrast, fluorescent image of DsRed, and merged image of DsRed and phase contrast. The scale in the figure represents 5 um.
The S. cerevisiae erg11 mutant was reported to be temperature-sensitive and lethal under 37°C. Thus, erg11 mutant (Y40597) showing a temperature-sensitive phenotype was used in the yeast heterologous complementary assay. Full length CDS of three AoErg11s genes were fused into yeast expression vector (pYES2.0) and transformed into erg11 mutants. The ScErg11 gene was also transformed into the erg11 mutant as a positive control. As pYES2.0 contains a galactose-induced GAL1 promoter, phenotypes of all the transformants were observed on YPD (with glucose) and YPG (with galactose as an inducer) medium at 30°C and 37°C, respectively. The results showed that erg11 mutants were lethal at 37°C on YPD and YPG; AoErg11A/erg11—AoErg11C/erg11 could not restore the lethal phenotype of the erg11 mutants at 37°C while the control can restore the lethal phenotype (Figure 4A and Supplementary Figure S3). Moreover, we deleted the SP sequences of AoErg11A–AoErg11C to construct pYES2.0-AoErg11AΔSP, pYES2.0-AoErg11BΔSP and pYES2.0-AoErg11CΔSP vectors. And transforming them into the erg11 mutant. Similarly, AoErg11AΔSP–AoErg11CΔSP could not restore the temperature-sensitivity phenotype either (Figure 4B).
FIGURE 4. AoErg11s and AoErg11ΔSPs could not recover the phenotypes of the erg11 mutant of S. cerevisiae (A,B) Growth of wild type, erg11 mutant (Y40597), AoErg11s/erg11 transformants and AoErg11ΔSPs/erg11 transformants on YPD and YPG medium at 30°C and 37°C. (C,D) Measurement of ergosterol contents in all transformants, the control and transformants were cultured in liquid YPD and YPG for 2 days at 30°C, and the yeast were collected to determine the content of ergosterol. Statistical analyses were performed by t-test of GraphPad (*, p < 0.05; **, p < 0.01). The ergosterol content of each experimental group was compared with the pYes2.0/erg11 mutant in the corresponding medium. Values represent the mean ± SD of three independent experiments.
In addition, the ergosterol contents of the control and all transformants in 30°C liquid medium were measured. When the transformed genes were induced by YPG, the ergosterol content of AoErg11A/erg11 and AoErg11B/erg11 transformants were slightly increased compared with that in YPD, while it is still lower than control and the induction expression of AoErg11C in erg11 background showed no effects on the ergosterol content (Figure 4C). On the contrary, the induction of AoErg11AΔSP and AoErg11BΔSP in erg11 background slightly decreased ergosterol content, but the expression of AoErg11CΔSP increased the content of ergosterol, which was still lower than control (Figure 4D). Thus, it seems that AoErg11A/erg11, AoErg11B/erg11 and AoErg11CΔSP can partly restore the ergosterol content in erg11 mutant. However, the partial increment of ergosterol content is not enough to restore the temperature-sensitive phenotype of erg11 mutant.
The phenotypes of ER- and cytoplasm-located AoErg11s overexpressed strains were also investigated. All AoErg11s constructs (including AoErg11A-DsRed–AoErg11C-DsRed and AoErg11AΔSP-DsRed–AoErg11CΔSP-DsRed) were transformed into A. oryzae to obtain the overexpressed strains. Then, these transformants were cultured in CD, PDA and DPY medium (Figures 5A–C). The results showed that there were no significant differences in colony morphology and diameter of AoErg11A and AoErg11C between overexpressing strains and CK (Figures 5B,D), while the spore numbers in CD and PDA medium increased about 2 times compared with the control (Figure 5E). However, AoErg11B overexpressing strains showed significant differences in both colony diameter and spore numbers compared with the control (Figures 5D, E). The colony morphology, diameter and spore numbers of AoErg11AΔSP–AoErg11CΔSP overexpression strains were also detected (Figures 5F,G). Compared with the control, the colony morphology, diameter and spore numbers of these overexpression strains were significantly different (except AoErg11AΔSP overexpression strains in CD medium). In conclusion, overexpression of AoErg11s could affect the growth and sporulation of A. oryzae, and further indicates that the localization of AoErg11s is closely related to its function.
FIGURE 5. Phenotypes of AoErg11s and AoErg11ΔSPs overexpression strains. (A) The schematic of Figure B (upper) and Figure C (lower). (B,C) Colony morphologies of CK (wild-type A. oryzae transformed pEX2B vector), AoErg11s and AoErg11ΔSPs overexpression strains on CD, PDA and DPY medium after 72 h incubation (D–G) The relative concentration of spores and colony diameter of different transgenic strain colonies. Spore suspension of identical concentrations of different A. oryzae strains were plated on CD, PDA and DPY agar medium and incubated at 30°C for 72 h. Values represent the mean ± SD of three independent experiments. Statistical analyses were performed by t-test of GraphPad (*, p < 0.05; **, p < 0.01). Each experimental group was compared to the corresponding CK.
Since CYP51 is a key enzyme in the sterol synthesis pathway, therefore we detected the ergosterol content of all AoErg11s and AoErg11ΔSPs transgenic strains. As shown in Figure 6, compared with the control strain, the ergosterol content of AoErg11s overexpressing strains increased by 13%, 89% and 38%, while the ergosterol content of AoErg11ΔSPs overexpressing strains decreased by 34%, 6% and 13%, respectively. Ergosterol content was increased in all the AoErg11s overexpressed strains, indicating that AoErg11s gene expression was positively correlated with ergosterol synthesis. The ergosterol content of AoErg11ΔSPs overexpressing strains decreased slightly (except AoErg11AΔSP overexpressing strains), which indicated that AoErg11s localization was closely related to its function. It is consistent with the previous results obtained by subcellular localization. In conclusion, overexpression of AoErg11s disrupts the balance of ergosterol biosynthesis, thus affecting their cellular content.
FIGURE 6. Ergosterol contents of AoErg11s and AoErg11ΔSPs overexpression strains. The control and transformants were cultured in DPY for 3 days at 30°C and collected to determine the content of ergosterol. Values represent the mean ± SD of three independent experiments. Statistical analyses were performed by t-test of GraphPad (*, p < 0.05; **, p < 0.01). The ergosterol content of each experimental group was compared with CK.
Previous studies have reported that Erg11 is an important target enzyme for drug design (Liu et al., 2021). The azole antifungal drugs can bind with the hemoglobin at the active site of Erg11 (Hargrove et al., 2015), thus inhibiting the activity of Erg11, reducing the synthesis efficiency of ergosterol, causing varying degrees of damage to the integrity of the fungal plasma membrane, and suppressing the growth and reproduction of fungi. It has been found that the mutation or high expression of Erg11 gene is the main reason for the formation of drug resistance in fungi (Sun et al., 2014; Wei et al., 2015). Thus, a large number of studies focus on the discovery of Erg11 gene resistance mutation sites and the development of novel antifungal drugs with high efficiency and low toxicity. In this study, the function of three AoErg11s from A. oryzae, one of the most important industrial fungi, was analyzed. The results showed that: the three AoErg11s expressed differently at different growth times and under different abiotic stresses. Under normal conditions, AoErg11A and AoErg11B is the main expressed gene for sterol 14α-demethylase, AoErg11C is almost not expressed. But AoErg11C expression was significantly increased under stress conditions, which may indicate that AoErg11C plays an important role in A. oryzae response to abiotic stresses. All of these three proteins have a signal peptide in the N-terminal, which is localized in ER and distributed uniformly in the cytoplasm after excision of the signal peptide. AoErg11s can partially compensate the ergosterol content in S. cerevisiae erg11 mutant, but cannot completely restore the temperatures-sensitive lethal phenotype. Overexpression of these three AoErg11s can affect both growth and sporulation. The number of spores increased significantly after overexpression of AoErg11A and AoErg11C, while the number of spores decreased significantly after overexpression of AoErg11B. Thus, this study revealed the functions of three kinds of Erg11 in A. oryzae and their effects on the growth of A. oryzae and the biosynthesis of ergosterol, which may contribute to the further understanding of the ergosterol biosynthesis and regulation mechanism in this important filamentous fungus, A. oryzae.
Erg11 was first purified from S. cerevisiae (Yoshida and Aoyama, 1984) and has been found successively in animals (Rats) and plants (Sorghum bicolor), and is the most widely distributed member of the P450 family (Ghosh, 2017; Elfaki et al., 2018). Motif analysis showed that all of these Erg11s contained six conserved motifs. Therefore, Erg11 is evolutionarily conserved in plants, animals and fungi. There is only one Erg11 in S. cerevisiae genome, two Erg11s in A. niger and A. nidulans, while the third one (Erg11C) has evolved in A. flavus and A. oryzae genome. Previous studies have found that in A. flavus Erg11A and Erg11B are the main expression genes of 14α-demethylase activity, while the basic expression of Erg11C is very low or undetectable (Paul et al., 2018), which is consistent with our results. However, there are also differences between A. flavus and A. oryzae. Erg11A of A. flavus is the main gene leading to drug resistance (Lucio et al., 2020), Erg11B is a functionally redundant gene, and Erg11C is derived from the replication of Erg11A (Pérez-Cantero et al., 2020). Erg11C does not encode 14α-demethylase, but is essential for the complete virulence of fungus (Fan et al., 2013). In A. oryzae, the most important expression gene is AoErg11B (Figure 2), and AoErg11C is derived from the copy of AoErg11B (Figure 1), which plays an important role in A. oryzae response to abiotic stresses. We speculate that AoErg11A may be a functionally redundant gene. The expression of all three AoErg11 gene were relative higher at 24 and 72 h, but lower at 48 h. This may be due to the fact that at 24 h, the mycelia of A. oryzae is growing and expanding, which requires a large amount of ergosterol to synthesize a complete plasma membrane; at 48 h, the mycelia of A. oryzae was relative mature, there requirement of ergosterol was not as necessary as that of 24 h; at 72 h, the proliferation of A. oryzae requires a large amount of ergosterol to produce conidia. Our previous studies showed the function of ergosterol biosynthetic pathway genes such as Erg10 and Erg19 are conserved between S. cerevisiae and A. oryzae (Sun et al., 2019a; Sun et al., 2019b). In this study, yeast complementary experiment showed that AoErg11s could partially compensate for the ergosterol content in S. cerevisiae erg11 mutant, but it could not completely restore the temperature-sensitive lethal phenotype of S. cerevisiae erg11 deletion mutant. In addition, our previous study found that treatment of S. cerevisiae and A. oryzae with triazolone, an inhibitor targeting ERG11, inhibited the growth of S. cerevisiae, while it most has no impact on the germination and growth of A. oryzae (Hu et al., 2019). All these suggest that the function of the AoErg11 genes is more complex than that in S. cerevisiae. However, because of lacking phenotypes of knockout mutants, the function of these AoErg11s may not be fully uncovered. Further experiments to construct single, double and triple mutants of the three genes should be done to makes their function more explicit.
Previous studies have limited information on subcellular localization of Erg11 in species. In A. fumigatus, Erg11A and Erg11B were located in the ER (Roundtree et al., 2020). In M. oryzae, Erg11A was mainly located in the cytoplasm of mycelia and conidia (Yan et al., 2011). In this study, we through bioinformatics analysis found that there were plant MTS in the N-terminus of AoErg11A, and signal peptide in the N-terminus of AoErg11B and AoErg11C. Contrary to the predicted results, this study showed that AoErg11A- AoErg11C were located in the ER mediating by the signal peptide. We also noticed that the red fluorescence of AoErg11AΔSP–AoErg11CΔSP quenched very quickly (data not shown), indicating that the signal peptides of AoErg11s are not only required for their localization but also important for their homeostasis. Interestingly, the effects on ergosterol contents in ER and cytoplasm located AoErg11s over expression S. cerevisiae are very different. Phenotypes of overexpressed strains showed that AoErg11s could affect mycelia growth or sporulation. Similarly, overexpression of AoErg11s and AoErg11ΔSPs showed different effect on the phenotypes and ergosterol contents. Thus, the function of AoErg11 is closely related to its subcellular localization.
The datasets presented in this study can be found in online repositories. The names of the repository/repositories and accession number(s) can be found in the article/Supplementary Material.
ZH and BZ designed the manuscript. QJ wrote the manuscript. GL contribute to the statistical analysis of the data. KQ, YS, and HY participated in the collecting of data. GL and HL contributed to the revision of the manuscript.
This study was supported by Natural Science Foundation of Jiangxi Province (20192ACBL20012 and 20212BAB205001), National Natural Science Foundation of China (NSFC Grant NO. 31700068 and 32260009), the youth talent support program of Jiangxi Science and Technology Normal University (2019QNBJRC004), and Open Foundation of Hubei Key Laboratory of Edible Wild Plants Conservation and Utilization (EWPL202207).
The authors declare that the research was conducted in the absence of any commercial or financial relationships that could be construed as a potential conflict of interest.
All claims expressed in this article are solely those of the authors and do not necessarily represent those of their affiliated organizations, or those of the publisher, the editors and the reviewers. Any product that may be evaluated in this article, or claim that may be made by its manufacturer, is not guaranteed or endorsed by the publisher.
The Supplementary Material for this article can be found online at: https://www.frontiersin.org/articles/10.3389/fgene.2023.1009746/full#supplementary-material
Bard, M., Lees, N., Turi, T., Craft, D., Cofrin, L., Barbuch, R., et al. (1993). Sterol synthesis and viability of erg11 (cytochrome P450 lanosterol demethylase) mutations in Saccharomyces cerevisiae and Candida albicans. Lipids 28 (11), 963–967. doi:10.1007/BF02537115
Byskov, A. G., Andersen, C. Y., Nordholm, L., Thogersen, H., Xia, G., Wassmann, O., et al. (1995). Chemical structure of sterols that activate oocyte meiosis. Nature 374 (6522), 559–562. doi:10.1038/374559a0
Dudakova, A., Spiess, B., Tangwattanachuleeporn, M., Sasse, C., Buchheidt, D., Weig, M., et al. (2017). Molecular tools for the detection and deduction of azole antifungal drug resistance phenotypes in Aspergillus species. Clin. Microbiol. Rev. 30 (4), 1065–1091. doi:10.1128/CMR.00095-16
Elfaki, I., Mir, R., Almutairi, F., and Duhier, F. (2018). Cytochrome P450: polymorphisms and roles in cancer, diabetes and atherosclerosis. Asian Pac. J. Cancer Prev. 19 (8), 2057–2070. doi:10.22034/APJCP.2018.19.8.2057
El-Sherbeni, A., and El-Kadi, A. (2014). Characterization of arachidonic acid metabolism by rat cytochrome P450 enzymes: the involvement of CYP1As. Drug Metab. Dispos. Biol. fate Chem. 42 (9), 1498–1507. doi:10.1124/dmd.114.057836
Fan, J., Urban, M., Parker, J., Brewer, H., Kelly, S., Hammond-Kosack, K., et al. (2013). Characterization of the sterol 14α-demethylases of Fusarium graminearum identifies a novel genus-specific CYP51 function. New Phytol. 198 (3), 821–835. doi:10.1111/nph.12193
Gao, J., Tian, X., Zhou, J., Cui, M., Zhang, H., Gao, N., et al. (2017). From genotype to phenotype: cytochrome P450 2D6-mediated drug clearance in humans. Mol. Pharm. 14 (3), 649–657. doi:10.1021/acs.molpharmaceut.6b00920
Geber, A., Hitchcock, C., Swartz, J., Pullen, F., Marsden, K., Kwon-Chung, K., et al. (1995). Deletion of the Candida glabrata ERG3 and ERG11 genes: effect on cell viability, cell growth, sterol composition, and antifungal susceptibility. Antimicrob. Agents Chemother. 39 (12), 2708–2717. doi:10.1128/aac.39.12.2708
Ghosh, S. (2017). Triterpene structural diversification by plant cytochrome P450 enzymes. Front. Plant Sci. 8, 1886. doi:10.3389/fpls.2017.01886
Hargrove, T., Wawrzak, Z., Lamb, D., Guengerich, F., and Lepesheva, G. (2015). Structure-functional characterization of cytochrome P450 sterol 14α-demethylase (CYP51B) from Aspergillus fumigatus and molecular basis for the development of antifungal drugs. J. Biol. Chem. 290 (39), 23916–23934. doi:10.1074/jbc.M115.677310
Hawkins, N., Cools, H., Sierotzki, H., Shaw, M., Knogge, W., Kelly, S., et al. (2014). Paralog re-emergence: a novel, historically contingent mechanism in the evolution of antimicrobial resistance. Mol. Biol. Evol. 31 (7), 1793–1802. doi:10.1093/molbev/msu134
Hu, W., Sillaots, S., Lemieux, S., Davison, J., Kauffman, S., Breton, A., et al. (2007). Essential gene identification and drug target prioritization in Aspergillus fumigatus. PLoS Pathog. 3 (3), e24. doi:10.1371/journal.ppat.0030024
Hu, Z., Li, G., Sun, Y., Niu, Y., Ma, L., He, B., et al. (2019). Gene transcription profiling of Aspergillus oryzae 3.042 treated with ergosterol biosynthesis inhibitors. Braz. J. Microbiol. 50 (1), 43–52. doi:10.1007/s42770-018-0026-1
Huang, H., Niu, Y., Jin, Q., Qin, K., Wang, L., Shang, Y., et al. (2022). Identification of six thiolases and their effects on fatty acid and ergosterol biosynthesis in Aspergillus oryzae. Appl. Environ. Microbiol. 88 (6), e0237221. doi:10.1128/aem.02372-21
Jordá, T., and Puig, S. (2020). Regulation of ergosterol biosynthesis in Saccharomyces cerevisiae. Genes 11 (7), 795. doi:10.3390/genes11070795
Kaluzhskiy, L., Ershov, P., Yablokov, E., Shkel, T., Grabovec, I., Mezentsev, Y., et al. (2021). Human lanosterol 14-alpha demethylase (CYP51A1) Is a putative target for natural flavonoid luteolin 7,3'-disulfate. Mol. (Basel, Switz.) 26 (8), 2237. doi:10.3390/molecules26082237
Kim, H., Schaller, H., Goh, C., Kwon, M., Choe, S., An, C., et al. (2005). Arabidopsis cyp51 mutant shows postembryonic seedling lethality associated with lack of membrane integrity. Plant Physiol. 138 (4), 2033–2047. doi:10.1104/pp.105.061598
Klingenberg, M. (1958). Pigments of rat liver microsomes. Archives Biochem. Biophys. 75 (2), 376–386. doi:10.1016/0003-9861(58)90436-3
Kodedová, M., and Sychrová, H. (2015). Changes in the sterol composition of the plasma membrane affect membrane potential, salt tolerance and the activity of multidrug resistance pumps in Saccharomyces cerevisiae. PloS one 10 (9), e0139306. doi:10.1371/journal.pone.0139306
Lepesheva, G., and Waterman, M. (2011). Sterol 14alpha-demethylase (CYP51) as a therapeutic target for human trypanosomiasis and leishmaniasis. Curr. Top. Med. Chem. 11 (16), 2060–2071. doi:10.2174/156802611796575902
Liu, N., Abramyan, E., Cheng, W., Perlatti, B., Harvey, C., Bills, G., et al. (2021). Targeted genome mining reveals the biosynthetic gene clusters of natural product CYP51 inhibitors. J. Am. Chem. Soc. 143 (16), 6043–6047. doi:10.1021/jacs.1c01516
Lorbek, G., Perše, M., Jeruc, J., Juvan, P., Gutierrez-Mariscal, F., Lewinska, M., et al. (2015). Lessons from hepatocyte-specific Cyp51 knockout mice: impaired cholesterol synthesis leads to oval cell-driven liver injury. Sci. Rep. 5, 8777. doi:10.1038/srep08777
Lucio, J., Gonzalez-Jimenez, I., Rivero-Menendez, O., Alastruey-Izquierdo, A., Pelaez, T., Alcazar-Fuoli, L., et al. (2020). Point mutations in the 14-α sterol demethylase Cyp51A or Cyp51C could contribute to azole resistance in Aspergillus flavus Genes. Genes 11 (10), 1217. doi:10.3390/genes11101217
Luthra, A., Denisov, I., and Sligar, S. (2011). Spectroscopic features of cytochrome P450 reaction intermediates. Archives Biochem. Biophys. 507 (1), 26–35. doi:10.1016/j.abb.2010.12.008
Mabashi, Y., Kikuma, T., Maruyama, J., Arioka, M., and Kitamoto, K. (2006). Development of a versatile expression plasmid construction system for Aspergillus oryzae and its application to visualization of mitochondria. Biosci. Biotechnol. Biochem. 70 (8), 1882–1889. doi:10.1271/bbb.60052
Maruyama, J., Nakajima, H., and Kitamoto, K. (2002). Observation of EGFP-visualized nuclei and distribution of vacuoles in Aspergillus oryzae arpA null mutant. FEMS Microbiol. Lett. 206 (1), 57–61. doi:10.1111/j.1574-6968.2002.tb10986.x
Merz, M., Eisele, T., Berends, P., Appel, D., Rabe, S., Blank, I., et al. (2015). Flavourzyme, an enzyme preparation with industrial relevance: Automated nine-step purification and partial characterization of eight enzymes. J. Agric. Food Chem. 63 (23), 5682–5693. doi:10.1021/acs.jafc.5b01665
Neunzig, J., and Bernhardt, R. (2018). Effect of sulfonated steroids on steroidogenic cytochrome P450-dependent steroid hydroxylases. J. Steroid Biochem. Mol. Biol. 179, 3–7. doi:10.1016/j.jsbmb.2017.07.004
Nguyen, K., Ho, Q., Do, L., Mai, L., Pham, D., Tran, H., et al. (2017). A new and efficient approach for construction of uridine/uracil auxotrophic mutants in the filamentous fungus Aspergillus oryzae using Agrobacterium tumefaciens-mediated transformation. World J. Microbiol. Biotechnol. 33 (6), 107. doi:10.1007/s11274-017-2275-9
Ogris, I., Zelenko, U., Sosič, I., Gobec, M., Skubic, C., Ivanov, M., et al. (2021). Pyridylethanol (phenylethyl) amines are non-azole, highly selective Candida albicans sterol 14α-demethylase inhibitors. Bioorg. Chem. 106, 104472. doi:10.1016/j.bioorg.2020.104472
Paul, R., Rudramurthy, S., Dhaliwal, M., Singh, P., Ghosh, A., Kaur, H., et al. (2018). Magnitude of voriconazole resistance in clinical and environmental isolates of Aspergillus flavus and investigation into the role of multidrug efflux pumps. Antimicrob. Agents Chemother. 62 (11), 010222–e1118. doi:10.1128/AAC.01022-18
Peña-Diaz, J., Montalvetti, A., Flores, C., Constán, A., Hurtado-Guerrero, R., De Souza, W., et al. (2004). Mitochondrial localization of the mevalonate pathway enzyme 3-Hydroxy-3-methyl-glutaryl-CoA reductase in the Trypanosomatidae. Mol. Biol. Cell 15 (3), 1356–1363. doi:10.1091/mbc.e03-10-0720
Pérez-Cantero, A., López-Fernández, L., Guarro, J., and Capilla, J. (2020). Azole resistance mechanisms in Aspergillus: update and recent advances. Int. J. Antimicrob. Agents 55 (1), 105807. doi:10.1016/j.ijantimicag.2019.09.011
Qian, P., Han, B., Forestier, E., Hu, Z., Gao, N., Lu, W., et al. (2013). Sterols are required for cell-fate commitment and maintenance of the stomatal lineage in Arabidopsis. Plant J. Cell Mol. Biol. 74 (6), 1029–1044. doi:10.1111/tpj.12190
Revankar, S., Fu, J., Rinaldi, M., Kelly, S., Kelly, D., Lamb, D., et al. (2004). Cloning and characterization of the lanosterol 14alpha-demethylase (ERG11) gene in Cryptococcus neoformans. Biochem. Biophys. Res. Commun. 324 (2), 719–728. doi:10.1016/j.bbrc.2004.09.112
Rojas, N., Ortiz, G., Baruque, D., Cavalitto, S., and Ghiringhelli, P. (2011). Production of heterologous polygalacturonase I from Aspergillus kawachii in Saccharomyces cerevisiae in batch and fed-batch cultures. J. Indust. Microbiol. Biotechnol. 38 (9), 1437–1447. doi:10.1007/s10295-010-0929-9
Roundtree, M., Juvvadi, P., Shwab, E., Cole, D., and Steinbach, W. (2020). Aspergillus fumigatus Cyp51A and Cyp51B proteins are compensatory in function and localize differentially in response to antifungals and cell wall inhibitors. Antimicrob. Agents Chemother. 64 (10), 007355–e820. doi:10.1128/AAC.00735-20
Rudolf, J., Chang, C., Ma, M., and Shen, B. (2017). Cytochromes P450 for natural product biosynthesis in streptomyces: sequence, structure, and function. Nat. Product. Rep. 34 (9), 1141–1172. doi:10.1039/c7np00034k
Ruparel, S., Green, D., Chen, P., and Hargreaves, K. (2012). The cytochrome P450 inhibitor, ketoconazole, inhibits oxidized linoleic acid metabolite-mediated peripheral inflammatory pain. Mol. Pain 8, 73. doi:10.1186/1744-8069-8-73
Sanglard, D., Ischer, F., Parkinson, T., Falconer, D., and Bille, J. (2003). Candida albicans mutations in the ergosterol biosynthetic pathway and resistance to several antifungal agents. Antimicrob. Agents Chemother. 47 (8), 2404–2412. doi:10.1128/aac.47.8.2404-2412.2003
Sun, X., Wang, K., Yu, X., Liu, J., Zhang, H., Zhou, F., et al. (2014). Transcription factor CCG-8 as a new regulator in the adaptation to antifungal azole stress. Antimicrob. Agents Chemother. 58 (3), 1434–1442. doi:10.1128/AAC.02244-13
Sun, Y., Niu, Y., He, B., Ma, L., Li, G., Tran, V., et al. (2019a). A dual selection marker transformation system Using Agrobacterium tumefaciens for the industrial Aspergillus oryzae 3.042. J. Microbiol. Biotechnol. 29 (2), 230–234. doi:10.4014/jmb.1811.11027
Sun, Y., Niu, Y., Huang, H., He, B., Ma, L., Tu, Y., et al. (2019b). Mevalonate diphosphate decarboxylase MVD/Erg19 is required for ergosterol biosynthesis, growth, sporulation and stress tolerance in Aspergillus oryzae. Front. Microbiol. 10, 1074. doi:10.3389/fmicb.2019.01074
Urlep, Ž., Lorbek, G., Perše, M., Jeruc, J., Juvan, P., Matz-Soja, M., et al. (2017). Disrupting hepatocyte Cyp51 from cholesterol synthesis leads to progressive liver injury in the developing Mouse and decreases RORC signalling. Sci. Rep. 7, 40775. doi:10.1038/srep40775
Wang, L., Hu, T., Jiang, Z., Yan, Q., and Yang, S. (2021). Efficient production of a novel alkaline cold-active phospholipase C from Aspergillus oryzae by molecular chaperon co-expression for crude oil degumming. Food Chem. 350, 129212. doi:10.1016/j.foodchem.2021.129212
Watanabe, T., Matsuo, I., Maruyama, J., Kitamoto, K., and Ito, Y. (2007). Identification and characterization of an intracellular lectin, calnexin, from Aspergillus oryzae using N-glycan-conjugated beads. Biosci. Biotechnol. Biochem. 71 (11), 2688–2696. doi:10.1271/bbb.70289
Wei, X., Zhang, Y., and Lu, L. (2015). The molecular mechanism of azole resistance in Aspergillus fumigatus: from bedside to bench and back. J. Microbiol. (Seoul, Korea) 53 (2), 91–99. doi:10.1007/s12275-015-5014-7
Yan, X., Ma, W., Li, Y., Wang, H., Que, Y., Ma, Z., et al. (2011). A sterol 14α-demethylase is required for conidiation, virulence and for mediating sensitivity to sterol demethylation inhibitors by the rice blast fungus Magnaporthe oryzae. Fungal Genet. Biol. 48 (2), 144–153. doi:10.1016/j.fgb.2010.09.005
Yoshida, Y., and Aoyama, Y. (1984). Yeast cytochrome P-450 catalyzing lanosterol 14 alpha-demethylation. I. Purification and spectral properties. J. Biol. Chem. 259 (3), 1655–1660. doi:10.1016/s0021-9258(17)43458-2
Keywords: Aspergillus oryzae, sterol 14α-demethylase, ergosterol, subcellular localization, function
Citation: Jin Q, Li G, Qin K, Shang Y, Yan H, Liu H, Zeng B and Hu Z (2023) The expression pattern, subcellular localization and function of three sterol 14α-demethylases in Aspergillus oryzae. Front. Genet. 14:1009746. doi: 10.3389/fgene.2023.1009746
Received: 02 August 2022; Accepted: 13 January 2023;
Published: 23 January 2023.
Edited by:
Yanchun Shao, Huazhong Agricultural University, ChinaReviewed by:
Somanon Bhattacharya, Stony Brook University, United StatesCopyright © 2023 Jin, Li, Qin, Shang, Yan, Liu, Zeng and Hu. This is an open-access article distributed under the terms of the Creative Commons Attribution License (CC BY). The use, distribution or reproduction in other forums is permitted, provided the original author(s) and the copyright owner(s) are credited and that the original publication in this journal is cited, in accordance with accepted academic practice. No use, distribution or reproduction is permitted which does not comply with these terms.
*Correspondence: Zhihong Hu, aHV6aGlob25nNDI2QDE2My5jb20=; Bin Zeng, WmVuZ3R4MDAxQGFsaXl1bi5jb20=
Disclaimer: All claims expressed in this article are solely those of the authors and do not necessarily represent those of their affiliated organizations, or those of the publisher, the editors and the reviewers. Any product that may be evaluated in this article or claim that may be made by its manufacturer is not guaranteed or endorsed by the publisher.
Research integrity at Frontiers
Learn more about the work of our research integrity team to safeguard the quality of each article we publish.