- 1Department of Oral Biology, University of Florida, Gainesville, FL, United States
- 2Department of Biological Sciences, Arkansas State University, Jonesboro, AR, United States
CRISPR-Cas is a bacterial immune system that restricts the acquisition of mobile DNA elements. These systems provide immunity against foreign DNA by encoding CRISPR spacers that help target DNA if it re-enters the cell. In this way, CRISPR spacers are a type of molecular tape recorder of foreign DNA encountered by the host microorganism. Here, we extracted ∼8,000 CRISPR spacers from a collection of over three hundred Streptococcus mutans genomes. Phage DNA is a major target of S. mutans spacers. S. mutans strains have also generated immunity against mobile DNA elements such as plasmids and integrative and conjugative elements. There may also be considerable immunity generated against bacterial DNA, although the relative contribution of self-targeting versus bona fide intra- or inter-species targeting needs to be investigated further. While there was clear evidence that these systems have acquired immunity against foreign DNA, there appeared to be minimal impact on horizontal gene transfer (HGT) constraints on a species-level. There was little or no impact on genome size, GC content and ‘openness’ of the pangenome when comparing between S. mutans strains with low or high CRISPR spacer loads. In summary, while there is evidence of CRISPR spacer acquisition against self and foreign DNA, CRISPR-Cas does not act as a barrier on the expansion of the S. mutans accessory genome.
Introduction
Streptococcus mutans is a Gram positive bacteria that inhabits the oral cavity and has a strong association with causing dental caries (tooth decay) (Lemos et al., 2019). It has a genome size of approximately 2 Mbp and an “open” pangenome, meaning that as more S. mutans genomes are sequenced new genes previously not associated with S. mutans are discovered (Song et al., 2013; Meng et al., 2017). These observations suggest that the organism is adaptable and able to acquire new traits over time. More broadly, it has been estimated that as a group 60% + of the pangenome of streptococci is acquired via horizontal gene transfer (HGT) (Richards et al., 2014). The ability of S. mutans to horizontally acquire DNA is likely greatly facilitated by competence development, a trait that is conserved (comRS, comX and late-competence genes) across the species (Khan et al., 2016). There are other important contributors to the genetic variability observed in S. mutans including residing in the oral microbiome which is likely a hotspot for HGT and significant pressure to adapt to new eco-niches. Regarding the last point, S. mutans strains have acquired traits, such as collagen-binding proteins, that have expanded its niche into cardiovascular diseases (Abranches et al., 2011).
In a recent publication we detected the presence of at least one CRISPR (clustered regularly interspaced short palindromic repeats)-Cas(CRISPR-associated protein) system in 95% of S. mutans strains from a collection of 477 genomes (Shields et al., 2020). CRISPR-Cas systems function as an adaptive immune system, incorporating DNA fragments from invading DNA (CRISPR spacers) that in concert with Cas endonucleases prevent future ‘re-infection’ of the invading DNA. CRISPR-Cas systems are organized into types and subtypes depending on the function of the Cas nuclease and the organization of the CRISPR-Cas loci. Type I (C and E subtypes) and Type II (A subtype) are most commonly identified in S. mutans and these systems recognize and cleave DNA. There are rare instances where Type III-A systems, that have specificity towards cleaving RNA (Kolesnik et al., 2021), are found in S. mutans (Shields et al., 2020). A substantial number of S. mutans strains encode both a Type I-C and a Type II-A CRISPR-Cas system, perhaps to help with viral escape from either system. The model S. mutans strain, UA159, carries Type II-A and Type I-C CRISPR-Cas loci. The Type II-A system is functional, is capable of interfering with plasmid transformation and has been repurposed for CRISPR interference and genome editing (Serbanescu et al., 2015; Gong et al., 2018; Shields et al., 2020). It is likely that the Type I-C system is not functional because of a truncated Cas1 protein (van der Ploeg, 2009; Serbanescu et al., 2015). Interference of both plasmid and phage DNA has also been shown in S. mutans P42S, with the Cas9 protein recognizing a different protospacer adjacent motif (PAM) domain compared with the UA159 Cas9 nuclease (Mosterd and Moineau, 2020). Some progress has been made exploring the functionality of these systems but there has been limited analysis of CRISPR spacers acquired by S. mutans. The most comprehensive study of S. mutans CRISPR spacers was conducted in 2009 from 29 individual strains (van der Ploeg, 2009). With our large collection of S. mutans genomes, and changes in CRISPR-Cas analysis over the last decade, we believe an updated analysis of CRISPR spacers is warranted.
The observation that S. mutans has a large pan-genome is in conflict with the high frequency of CRISPR-Cas systems across the species. These systems have primarily been considered as a phage defense mechanism. However, there is experimental evidence that CRISPR-Cas systems can limit other types of HGT (e.g., plasmid acquisition) in laboratory settings including in S. mutans (Serbanescu et al., 2015). Despite evidence that CRISPR-Cas systems protect bacteria from phage and other mobile genetic element infections in simplified systems, it remains unclear how much they contribute to the ecology and evolution of bacteria living in complex natural environments. When large genome datasets are studied there has been conflicting evidence of the impact of CRISPR-Cas on HGT in bacterial populations. In Pseudomonas aeruginosa CRISPR-Cas systems constrain HGT (Wheatley and MacLean, 2021) and there is evidence of immunity against antibiotic resistance genes in Enterococcus faecalis strains carrying CRISPR-Cas (Palmer and Gilmore, 2010). However, there is also evidence of no inhibition of HGT by CRISPR-Cas in other species (Gophna et al., 2015). The oral microbiome, specifically dental plaque, has a high CRISPR spacer load compared with other body sites (Münch et al., 2021), it is a dynamic environment and it is a large community of interacting microbes and phages. It therefore provides an excellent model for understanding the contribution of CRISPR-Cas in limiting HGT in complex microbial settings. Here, using S. mutans as a starting point, we will begin to address how impactful CRISPR-Cas is on genomic diversification in this complex ecosystem.
Methods
Genomic data
We have a collection of 477 S. mutans genomes that was curated by Vince Richards at Clemson University; sequencing files were downloaded from GenBank/NCBI and assembled. Genomes are available in. gbk format on the Github platform (https://github.com/theshieldslab/Streptococcus-mutans-CRISPR-Spacers-Analysis). For this study CRISPR spacers were isolated from 335 S. mutans genomes. Incomplete assemblies were evident in the other S. mutans genomes, likely because of Illumina based whole genome sequencing, leading to difficulty in identifying repeat regions (i.e., CRISPR spacer regions).
CRISPR spacer identification
Identification and data curation of the CRISPR systems found in our clinical isolates was conducted at the University of Florida HiPerGator cluster computer with the CRISPRCasFinder (Couvin et al., 2018) version 4.2.17 pipeline. The resulting output files, containing the predicted spacers, were then processed and summarized in several files containing CRISPR system types and subtype, list of genes, repeat sequences, count of repeat sequences, and spacers sequences. The spacer sequences were then labeled with their respective isolate name, contig ID where the spacers was predicted, and an additional counter number. All CRISPR spacer sequences were then parsed into a FASTA format file, which is available on the Github platform.
CRISPR spacer target identification
Blastn was used to identify spacer targets from phage, integrative and conjugative elements (ICEs), plasmids, and S. mutans. Phage DNA sequences were downloaded from NCBI (ftp://ftp.ncbi.nih.gov/refseq/release/viral/). ICE sequences were downloaded from the ICE database ICEberg 2.0 (https://bioinfo-mml.sjtu.edu.cn/ICEberg2/download.html) (Liu et al., 2019). Plasmid sequences were downloaded from a database containing 10,892 complete and annotated plasmids (Brooks et al., 2019). In addition, known S. mutans plasmid sequences were compiled (available on the Github platform) (Zhou et al., 2001; Caufield et al., 2007; Rheinberg et al., 2013). Parameters for Blastn were set to 95% sequence coverage (qcovs) and 85% sequence identity (pident). These parameters are a reasonable threshold for identifying CRISPR targets but it is possible that CRISPR spacers meeting these criteria are not capable of DNA interference because of mismatches in the seed region, or because the foreign DNA has mismatches in the proto-spacer adjacent motif (PAM). Other parameters generated with the sequence results included the number of mismatches, the evalue and the subject strand (sstrand). All raw output data is available as. xlsx files on the Github platform.
Analysis of genome size, GC content and the pangenome
The data mining for gene clusters was conducted in a series of steps, starting with Prokka annotations for each clinical isolate genome (Seemann, 2014). Since all isolates belong to the Streptococcus genus, annotations with Prokka were defined within Bacteria and Streptococci for better gene predictions. Multilocus sequence typing (MLST) was conducted with Roary (Page et al., 2015), which is ideal for close non-divergent genomes. The final step was to generate the phylogenomic tree from the genome alignment file created by Roary. A maximum likelihood tree was drawn with the Phyml (Guindon and Gascuel, 2003; Hordijk and Gascuel, 2005) algorithm and plotted in R (R: a language and environment for statistical computing) with the GGtree (Yu et al., 2017) package. This work also included custom bash command lines in order to generate accurate measurements of GC content and genome size for all the isolates in our bank.
Results and discussion
CRISPR arrays and spacers are frequently detected in S. mutans isolates
Using CRISPRCasFinder (Couvin et al., 2018) across our genome database we identified a total of 8,078 CRISPR spacers of which 5,875 are unique sequences. Isolates with CRISPR arrays present carry an average of 23.9 spacers each, with strain smu174 carrying 129 spacers in total (on one array). By comparison, Streptococcus thermophilus strains carry an average of 33 spacers per cassette (Horvath et al., 2008). The majority of S. mutans strains carry one or two CRISPR arrays but there are rare instances of strains carrying 4 + (Figure 1A). Figure 1B shows a frequency distribution of CRISPR spacer load across all the isolates. CRISPR spacer acquisition is a balance between obtaining immunity against harmful sequences without diluting spacers (some which may provide inadequate immunity because of mutation) across the available Cas machinery (Garrett, 2021). Most CRISPR spacers were either 30-nt or 32–35-nt in length (Figure 1C), with these two groups likely arising from differences in Type I and Type II CRISPR spacer lengths. The average GC content of CRISPR spacers was 38.16%, which is above the average GC content of S. mutans strains (Figure 1D). This may indicate that foreign DNA encountered by S. mutans is on average of a higher GC content than the average S. mutans genome. By way of comparison, M102AD, an S. mutans infecting phage has GC content of 39.6%.
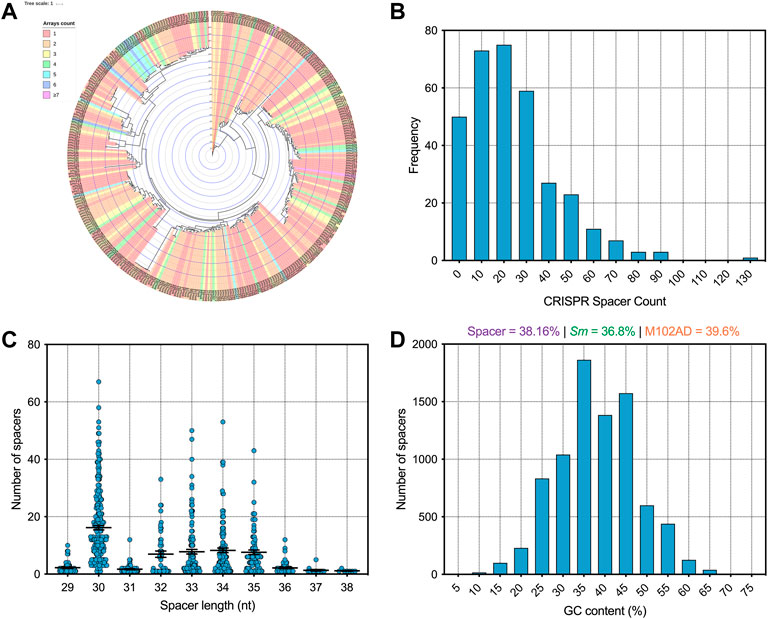
FIGURE 1. Analysis of S. mutans CRISPR spacers (A) A phylogenetic tree was generated using iTOL (Letunic and Bork, 2021) and strains were colored according to how many CRISPR spacer arrays were present on each strain genome (B) A frequency distribution of CRISPR spacer load across all the isolates (C) CRISPR spacers were grouped according to the length (nt) of the CRISPR spacers (D) A frequency distribution of CRISPR spacer GC content (percentage GC) was generated.
Targets of S. mutans CRISPR spacers
Having identified 8,078 spacers across our S. mutans genomes we next wanted to identify targets for these spacers. First, we characterized how many of these spacers target foreign DNA. This is the traditional target of CRISPR-Cas systems, and includes interference with phage, plasmid, integrative and conjugative elements (ICEs), and other bacterial DNA. In total, 1913 spacers (23.7%) mapped to these targets (Figure 2). Next, we will describe these targets in more detail for each sub-group of foreign DNA.
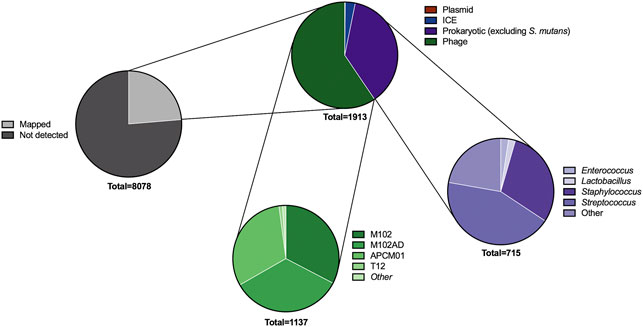
FIGURE 2. Predicted targets of CRISPR spacers. A series of pie charts shows the targets of spacers after searching across viral, plasmid, integrative and conjugative element, and bacterial DNA databases. The types of bacterial and phage DNA being targeted were further classified into genus (for bacteria) and strain (phage). These targets are not unique; for example, many of the CRISPR spacers targeting phage will target multiple phages if the phage gene is conserved.
Phage. Adaptive immunity against bacteriophage is a notable feature of CRISPR-Cas systems across bacteria. Consistent with this concept S. mutans CRISPR spacers mapped to 1,137 phage sequences, of which 531 were unique matches. A total of sixteen distinct phage species are targeted by S. mutans CRISPR spacers (Supplementary Table S1). For four of these phages S. mutans is the known host, whereas for the other phages the hosts are other Firmicutes bacteria; Staphylococci, Enterococci, Lactobacilli and Streptococci. For these phages, typically only one spacer aligned against a conserved target. We anticipate that rather than infecting S. mutans, these phage genes are present either as prophage elements or as orphan genes in S. mutans genomes. The four S. mutans infecting phage that S. mutans has acquired spacer immunity against are known as M102, M102AD, ɸAPCM01 and smHBZ8 (Delisle and Rostkowski, 1993; Delisle et al., 2012; Dalmasso et al., 2015; Zaken et al., 2021). These are the only S. mutans phage that have been genome sequenced and all belong to the Siphoviridae family of double-stranded DNA viruses; they are lytic phage. At least two other S. mutans phage, e10 and f1, are known to infect S. mutans but these strains have not been sequenced (Delisle and Rostkowski, 1993). The four sequenced S. mutans phage are highly related, particularly the first 20 Kbp (Figure 3). However, they each contain a variable region consisting of small open reading frames with unknown functions at the 3’ end of the genome (Figure 3). Spacers were mapped and viewed with a genome browser to determine if there is any bias in spacer acquisition to specific phage genes. Overall, it appears that immunity to the variable region is less commonly acquired, and this was most evident for M102 and M102AD.
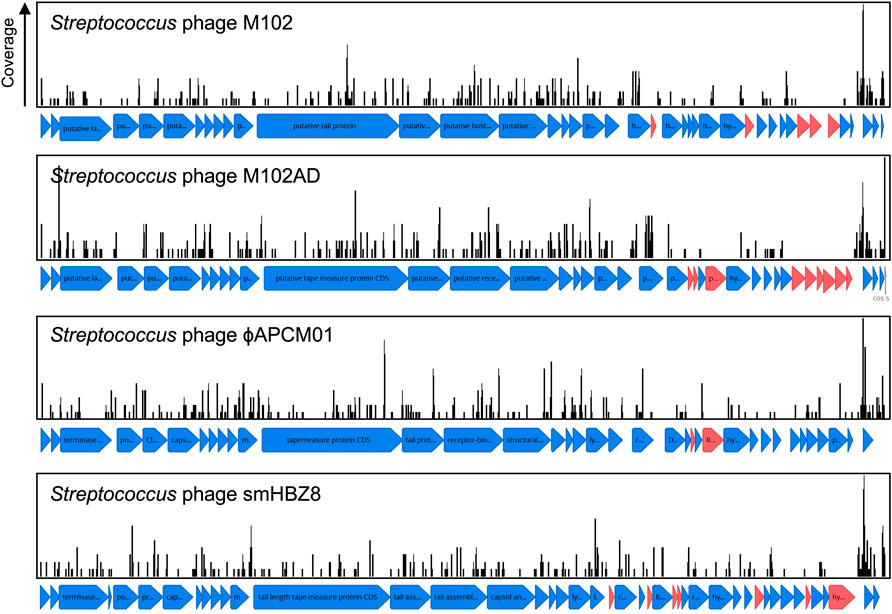
FIGURE 3. CRISPR spacer acquisition against S. mutans bacteriophage. CRISPR spacers were blasted against viral sequences downloaded from NCBI. CRISPR spacers mapped to four S. mutans infecting phage, M102 (371 spacers), M102AD (388 spacers), ɸAPCM01 (354 spacers) and smHBZ8 (362 spacers). These are the only S. mutans phage that have been genome sequenced and all belong to the Siphoviridae family of double-stranded DNA viruses. CRISPR spacers that mapped to phage sequences are shown as black lines, with the height of these lines indicating the total number of spacers that mapped to that specific region. Open reading frames for which no CRISPR spacers have been acquired against are colored red, and are more frequently located in a variable region of the phage genomes.
Isolation of S. mutans phage is rare, For example, ɸAPCM01 was the only S. mutans phage isolated from 85 saliva samples (Dalmasso et al., 2015) and smHBZ8 was the single S. mutans phage isolated from 254 samples (diverse sources; saliva, dental sewage, cariogenic dentin, extracted teeth, and dental plaque) (Zaken et al., 2021). To understand how important CRISPR-Cas is in explaining the low frequency of infective phage isolation we calculated the frequency of phage immunity spacers across our genome database. In total, 64% of S. mutans strains that carry CRISPR-Cas systems have phage targeting spacers (against M102AD or M102 or ɸAPCM01). The high frequency of CRISPR-Cas systems and anti-phage spacers would likely limit the efficacy of phage therapy in S. mutans.
Plasmids. CRISPR spacers are also naturally acquired against plasmid DNA (Palmer and Gilmore, 2010; Touchon et al., 2011). Acquisition of spacers against plasmids was very infrequently identified for S. mutans. In a database containing ∼12,000 sequenced plasmids (Brooks et al., 2019) only two spacers mapped. A spacer from smu333 mapped to pLF25067 carried by Lactobacillus fermentum (Aryantini et al., 2017) and a spacer from smu408 mapped to pSZ4 from Staphylococcus warneri (Cheng et al., 2013, 1). One strain, smu441, carries a spacer that maps (88% coverage) against a small 5.6 kbp ‘cryptic’ plasmid that is carried by ∼5% of S. mutans strains (Zhou et al., 2001, 140; Rheinberg et al., 2013).
Integrative conjugative plasmids
Integrative and conjugative elements (ICEs; also known as conjugative transposons) are another type of mobile genetic element that can transfer between bacterial species and strains (Johnson and Grossman, 2015). Sequence data for known ICEs was downloaded from ICEberg and used to check for CRISPR spacer acquisition against ICEs. In total, S. mutans has acquired fifty-eight spacers against ICEs. All but one of these spacers matches against TnSmu1, an uncharacterized putative ICE which is related to Tn916 but with significant modifications (Ajdić et al., 2002). In the model S. mutans strain UA159 TnSmu1 is a large 20 kbp region comprising of over twenty predicted genes (Ajdić et al., 2002). Most of the genes in this region are hypothetical but some are predicted to have integrative and conjugative functions (e.g. type IV secretion system components). When aligned to this region the CRISPR spacers qualitatively appear to have been generated to target the integrase and secretion system components instead of hypothetical regions (Figure 4). The functionality of TnSmu1 and whether it behaves like an ICE is unknown. However, acquisition of CRISPR spacers by strains of S. mutans could indicate that it may be horizontally acquired and strains are actively trying to avoid acquisition of the element. Certain strains of S. mutans may also have been repeatedly attacked by TnSmu1 as the isolate Smu179 has acquired five spacers that align to TnSmu1. In Pseudomonas aeruginosa CRISPR spacers often target ICE or conjugative transfer genes and this is associated with a lower prevalence of these systems in strains harboring CRISPR-Cas systems (Wheatley and MacLean, 2021). Prevention of the acquisition of these elements likely arises because of fitness costs associated with them. Our analysis of CRISPR-mediated immunity of ICEs is likely conservative as description and characterization of these elements is limited in S. mutans.
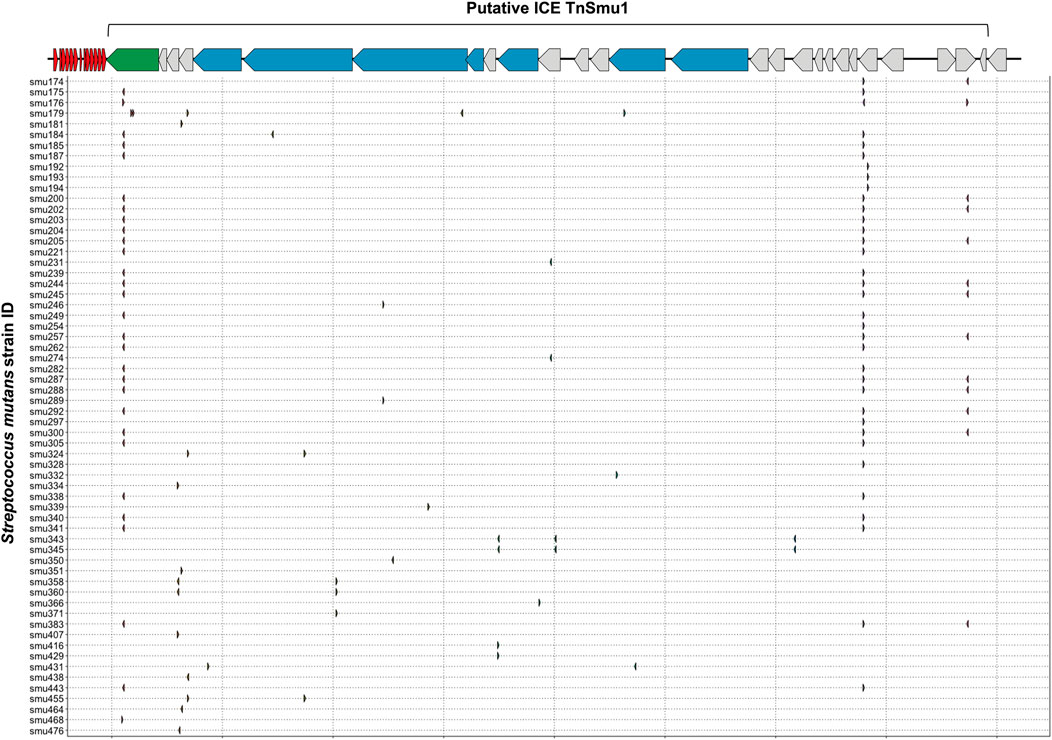
FIGURE 4. CRISPR spacer acquisition against the putative integrative and conjugative element TnSmu1. CRISPR spacers were blasted against ICE sequences downloaded from ICEberg. Multiple CRISPR spacers mapped to a putative S. mutans ICE known as TnSmu1. This element is approximately 20 Kbp and consists of twenty-eight genes. Most of the genes are hypothetical (grey) but some are annotated as putative T4SS components (blue) and one gene is annotated as an integrase (green). CRISPR spacers that mapped to this region are shown as small arrows, with a row for each S. mutans strain that has acquired spacers against the putative ICE.
S. mutans self- and strain-targeting CRISPR spacers
With the observation that S. mutans has gained immunity against inter-species transfer of mobile genetic elements we next wanted to explore if CRISPR spacers have been acquired more generally against S. mutans strain DNA. To do this, we searched for CRISPR spacer hits within genes encoded by S. mutans across all the genomes included in this study. A total of sixty-three annotated genes are the target of CRISPR spacers (Supplementary Table S2). In addition, six other genes with functional predictions (Supplementary Table S2) are also the target of CRISPR spacers. Lastly, S. mutans hypothetical proteins were also matched to S. mutans CRISPR spacer sequences. From this set of genes (excluding hypotheticals), 22 reside in the core genome (>99% of genomes), 4 are soft core genes (95–99% of genomes), 17 are shell genes (15–95% of genomes), and 20 are cloud genes (<15% of genomes) (Supplementary Table S2). CRISPR targets against S. mutans core genes are over-represented given that core genes only account for 5% of the S. mutans pangenome. This is compared with cloud genes which make up 85% of the pangenome. Notable S. mutans gene CRISPR targets include the clp system (Kajfasz et al., 2009), the gtfC glucosyltransferase (Hanada and Kuramitsu, 1988), the cell surface antigen spaP (Crowley et al., 1999), carbohydrate utilization systems (i.e., fruA and lacF) (Burne et al., 1999; Zeng et al., 2010) and several genes involved in DNA replication, repair or modification (i.e., dpnM, parE, radD, recF, repA, smc, ssb and topB). Several of the CRISPR spacers that target the S. mutans pangenome are likely targeting mobile DNA elements. For example, spacers against tyrosine recombinases (i.e., xerC and xerD), conjugal transfer protein traG, and the Int-Tn transposase from the ICE Tn916. To visualize the extent of CRISPR spacer acquisition against S. mutans genomes, spacers were mapped to two strains, UA159 and NN2025 (Figures 5A,B). The number of spacers that aligned within 10 kb regions varied, with notable hotspots against the genomes of both UA159 and NG8. These hotspot regions were TnSmu1 and repeat regions within the promoters of clpP (protein homeostasis), rexB (putative exonuclease) and a hypothetical protein.
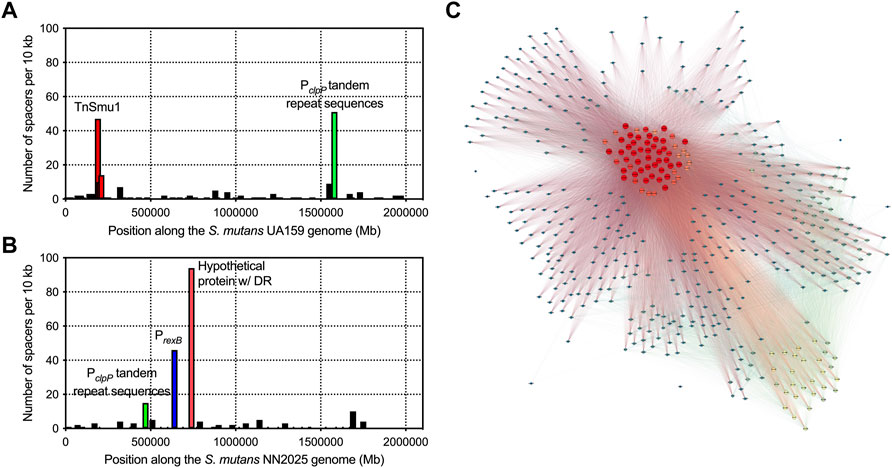
FIGURE 5. Acquisition of CRISPR spacers against S. mutans genes. To visualize CRISPR spacer acquisition against S. mutans genomes, spacers were mapped to two strains, UA159 (A) and NN2025 (B). Each graph shows the number of spacers that aligned within each 10 Kbp region of a S. mutans genome. There are notable hotspots that are highlighted with red, green and blue bars. A network diagram was constructed using Gephi (http://www.gephi.org) to visualize S. mutans strains that interact with other S. mutans strains on the basis of CRISPR spacer acquisition (C). Each node (circle) is a single S. mutans strain and a line between strains indicates a connection based on a CRISPR target being present on one of those strains. Strains with increased connections (targeting more strains with CRISPR spacers) are colored on a scale, with red nodes depicting strains with the most connections (CRISPR spacers targeting other S. mutans strains).
Inter-species CRISPR targeting was also visualized using network analysis (Figure 5C). This analysis showed that a minority of S. mutans strains (ca. 60 strains; red nodes in Figure 5C) were accounting for the majority of CRISPR spacer connections between S. mutans strains. These strains carry CRISPR spacers against highly conserved (core) S. mutans genes (Supplementary Table S2). We reasoned that this group of highly connected strains could actually be carrying self-targeting CRISPR spacers against highly conserved genes. Analysis of self-targeting revealed that ninety-four S. mutans strains carry self-targeting CRISPR spacers (Supplementary Table S3) and most of the highly connected nodes in the network analysis are strains that self-target. All of these strains contain the Cas genes required for functional CRISPR-Cas systems but it is unknown if these systems are inactivated through point mutations. Acquisition of self-targeting spacers would likely be detrimental and create pressure for inactivation of the CRISPR-Cas systems. Six out of the ninety-four self-targeting strains contain anti-CRISPR (Acr) proteins that could also account for a loss of functionality (Supplementary Table S3). In summary, S. mutans strains carry spacers that target S. mutans genes although the relative amount of intra-species-targeting versus only self-targeting is currently unknown.
Limited impact of CRISPR-Cas systems on S. mutans genome size and GC content
Analysis of S. mutans CRISPR spacers has shown potential immunity acquisition against phage, plasmid, ICE, and S. mutans DNA. Acquisition of spacers against these types of DNA should act to limit the rate of HGT in S. mutans. The association between CRISPR-Cas and its impact on HGT in bacteria is not clear. For example, P. aeruginosa genomes with CRISPR-Cas are significantly smaller than those lacking CRISPR-Cas which suggests that CRISPR-Cas impedes HGT (Wheatley and MacLean, 2021). However, a similar trend was not found for Staphylococcus aureus or Acinetobacter baumannii (Pursey et al., 2022). Here, we are using CRISPR spacer load as a measure of CRISPR-Cas activity. It has limitations because we cannot determine if the CRISPR arrays/systems are functional or defective. However, it is the most appropriate measure because it is unfeasible to test for genomic differences between strains with and without CRISPR-Cas systems because the majority of S. mutans strains have at least one CRISPR-Cas system. CRISPR spacer load, the number of CRISPR spacers per genome, has been used as a measure of CRISPR activity by others (Gophna et al., 2015). For our analysis, S. mutans strains were grouped by the total number of spacers they have acquired and for each group the median size of the genome was computed (Figure 6). We found that increased CRISPR spacers loads were associated with marginally increased genome sizes. Strains with 1–20 CRISPR spacers had a median genome size of 2.01 Mbp and strains with 40–60 CRISPR spacers had a median genome size of 2.05 Mbp (two-tailed t-test p < 0.01). Our analysis shows that increased CRISPR spacer acquisition does not lead to reduced genome size in S. mutans.
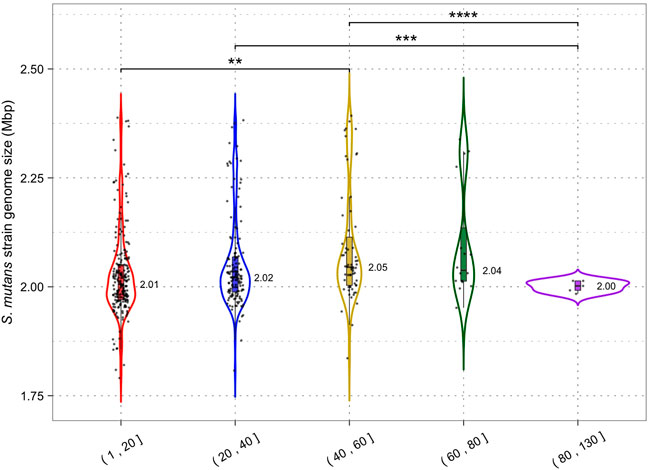
FIGURE 6. CRISPR activity and genome size. Genomes were grouped according to the number of CRISPR spacers present (1–20, 21–40, 41–60, 61–80 and 81+) and a violin plot was generated for each group. Median genome size (Mbp) is shown next to each violin plot; each dot is a single S. mutans strain/genome. Statistical significance between groups was calculated using a two-tailed t-test (** = p < 0.01; *** = p < 0.001).
By limiting HGT CRISPR-Cas could also affect the GC content of host genomes by reducing the acquisition of higher or lower GC foreign DNA. The average GC content across all S. mutans CRISPR spacers was 38.2% (Figure 1D) which may indicate that on average foreign DNA elements have higher GC content than the average S. mutans genome (36.8%). Phages that infect S. mutans, M102AD, M102, and SMHBZ8 have GC content of 39.6%, 39.21%, and 38.8% respectively (van der Ploeg, 2007; Delisle et al., 2012; Zaken et al., 2021). The GC content of S. mutans genomes was consistent for strains with 1–20 (36.80%), 20–40 (36.82%) and 40–60 (36.79%) CRISPR spacers (Figure 7). A significant difference in GC content was observed for strains with 60–80 (36.68%) CRISPR spacers compared with the 1–20 and 20–40 groupings (two-tailed t-test p <0.05). Although only nominally lower than the other groups, this might indicate a reduction in the acquisition of higher GC foreign DNA among strains with 60–80 CRISPR spacers.
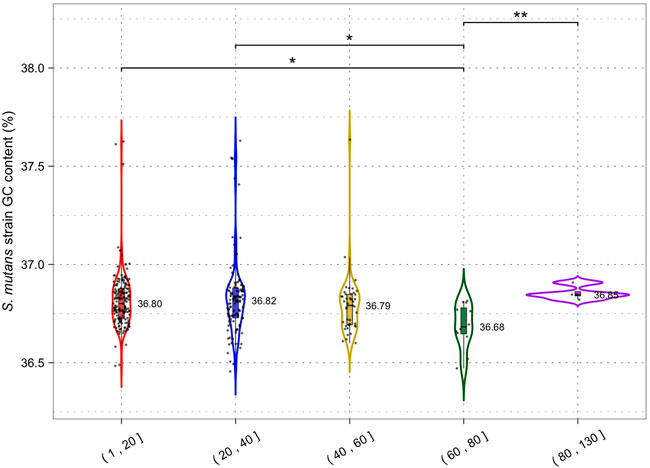
FIGURE 7. CRISPR activity and GC content. Genomes were grouped according to the number of CRISPR spacers present (1–20, 21–40, 41–60, 61–80 and 81+) and a violin plot was generated for each group. Median GC content (percentage GC) is shown next to each violin plot; each dot is a single S. mutans strain/genome. Statistical significance between groups was calculated using a two-tailed t-test (* = p <0.05; ** = p < 0.01).
CRISPR-Cas systems do not restrict the size of the S. mutans pangenome
To gain a greater insight into the impact of CRISPR-Cas systems on HGT we wanted to examine how CRISPR spacer load alters the size and distribution of the S. mutans pangenome. In our analysis including 335 S. mutans genomes, the pangenome size was calculated to include 15,062 genes. There were 822 genes in the core genome (>99% of genomes), 496 soft core genes (95–99% of genomes), 982 shell genes (15–95% of genomes), and 12,762 cloud genes (<15% of genomes). Next, pangenome analysis was conducted on two groups, one with a low CRISPR spacer load (1–20 spacers, average 9.36; 163 genomes) and another with a high CRISPR spacer load (21–129 spacers, average 37.93; 169 genomes). For both groups we generated pie charts which show the percentage of genes located in the different gene clusters (core; soft core; shell; cloud) (Figure 8). The low and high CRISPR spacer load groups have a similar percentage of genes located within the cloud genes gene cluster. Cloud genes are those that are most likely acquired via HGT. By this measure there is no limiting of HGT by CRISPR-Cas systems for S. mutans strains with either low or high CRISPR spacer loads.
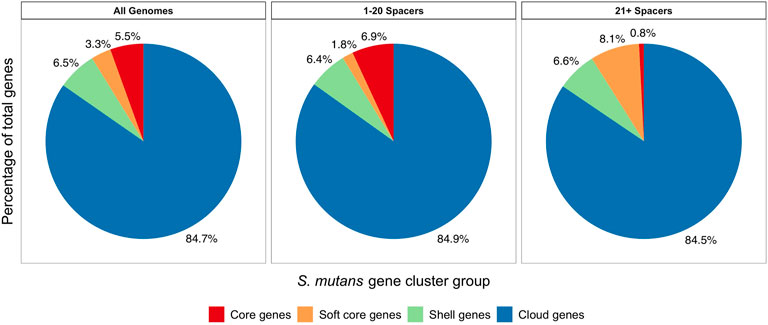
FIGURE 8. Pangenome analysis comparing low and high CRISPR spacer load S. mutans strains. Pangenome analysis was conducted on three groups. 1) all S. mutans genomes, 2) a group with low CRISPR spacer load (1–20 spacers, average 9.36; 163 genomes) and 3) a group with a high CRISPR spacer load (21–129 spacers, average 37.93; 169 genomes). For the three groups pie charts were generated which show the percentage of genes located in the different gene clusters (core, red; soft core, orange; shell, green; cloud, blue). Cloud genes (blue) are most likely acquired via HGT.
Conclusion
Here, we have generated a comprehensive ‘memory’ of DNA that has attacked a diverse panel of S. mutans isolates. Although there is considerable ‘dark matter’ (undetected CRISPR spacer sequences), phage are the main target of S. mutans spacers. Isolates have also generated immunity against mobile DNA elements such as plasmids and ICEs. There may also be considerable immunity generated against bacterial DNA, although the relative contribution of self-targeting versus bona fide intra- or inter-species targeting needs to be investigated further. Collectively, CRISPR-Cas systems and acquired spacers are abundant across all S. mutans strains. While there is clear evidence that these systems have acquired immunity against foreign DNA, there appears to be minimal impact on HGT constraints on a species-level. There was little or minimal impact on genome size, GC content and ‘openness’ of the pangenome. There are several possible explanations for why there is a minimal impact on HGT and these include 1) the systems have specificity for phage infection, 2) self-targeting has led to a loss of activity, 3) the systems are highly regulated to only acquire immunity under certain conditions, 4) competence acquired DNA is single stranded and the CRISPR-Cas systems are less active against ssDNA, 5) spacer load may be an inaccurate measure of CRISPR-Cas activity and 6) target or spacer DNA has changed so that immunity no longer functions. A deeper understanding of the impact of these systems on the biology and evolution of S. mutans will likely require functional studies and/or model systems for interrogating spacer acquisition.
Data availability statement
The datasets presented in this study can be found in online repositories. The names of the repository/repositories and accession number(s) can be found in the article/Supplementary Material.
Author contributions
AR and RS were involved in the investigation, data curation and revised the manuscript; AR performed the formal analysis and software development (implementation of code and supporting algorithms); RS was involved with funding acquisition, conceptualization of the study and drafted the manuscript.
Funding
This work was supported in part by the National Institute for Dental and Craniofacial Research (NIDCR) grant DE029882 awarded to RS.
Acknowledgments
We would like to thank Vince Richards (Clemson University) for providing us with the S. mutans genome files associated with this study.
Conflict of interest
The authors declare that the research was conducted in the absence of any commercial or financial relationships that could be construed as a potential conflict of interest.
Publisher’s note
All claims expressed in this article are solely those of the authors and do not necessarily represent those of their affiliated organizations, or those of the publisher, the editors and the reviewers. Any product that may be evaluated in this article, or claim that may be made by its manufacturer, is not guaranteed or endorsed by the publisher.
Supplementary material
The Supplementary Material for this article can be found online at: https://www.frontiersin.org/articles/10.3389/fgene.2022.997341/full#supplementary-material
References
Abranches, J., Miller, J. H., Martinez, A. R., Simpson-Haidaris, P. J., Burne, R. A., and Lemos, J. A. (2011). The collagen-binding protein Cnm is required for Streptococcus mutans adherence to and intracellular invasion of human coronary artery endothelial cells. Infect. Immun. 79, 2277–2284. doi:10.1128/IAI.00767-10
Ajdić, D., McShan, W. M., McLaughlin, R. E., Savić, G., Chang, J., Carson, M. B., et al. (2002). Genome sequence of Streptococcus mutans UA159, a cariogenic dental pathogen. Proc. Natl. Acad. Sci. U. S. A. 99, 14434–14439. doi:10.1073/pnas.172501299
Aryantini, N. P. D., Prajapati, J. B., Urashima, T., and Fukuda, K. (2017). Complete genome sequence of Lactobacillus fermentum MTCC 25067 (formerly TDS030603), a viscous exopolysaccharide-producing strain isolated from Indian fermented milk. Genome Announc. 5, e00091–17. doi:10.1128/genomeA.00091-17
Brooks, L., Kaze, M., and Sistrom, M. (2019). A curated, comprehensive database of plasmid sequences. Microbiol. Resour. Announc. 8, e01325–18. doi:10.1128/MRA.01325-18
Burne, R. A., Wen, Z. T., Chen, Y.-Y. M., and Penders, J. E. C. (1999). Regulation of expression of the fructan hydrolase gene of Streptococcus mutans GS-5 by induction and carbon catabolite repression. J. Bacteriol. 181, 2863–2871. doi:10.1128/JB.181.9.2863-2871.1999
Caufield, P. W., Saxena, D., Fitch, D., and Li, Y. (2007). Population structure of plasmid-containing strains of Streptococcus mutans, a member of the human indigenous biota. J. Bacteriol. 189, 1238–1243. doi:10.1128/JB.01183-06
Cheng, V. W. T., Zhang, G., Oyedotun, K. S., Ridgway, D., Ellison, M. J., and Weiner, J. H. (2013). Complete genome of the solvent-tolerant Staphylococcus warneri strain SG1. Genome Announc. 1, e0003813. doi:10.1128/genomeA.00038-13
Couvin, D., Bernheim, A., Toffano-Nioche, C., Touchon, M., Michalik, J., Néron, B., et al. (2018). CRISPRCasFinder, an update of CRISRFinder, includes a portable version, enhanced performance and integrates search for Cas proteins. Nucleic Acids Res. 46, W246–W251. doi:10.1093/nar/gky425
Crowley, P. J., Brady, L. J., Michalek, S. M., and Bleiweis, A. S. (1999). Virulence of a spaP mutant ofStreptococcus mutans in a gnotobiotic rat model. Infect. Immun. 67, 1201–1206. doi:10.1128/IAI.67.3.1201-1206.1999
Dalmasso, M., Haas, E. de, Neve, H., Strain, R., Cousin, F. J., Stockdale, S. R., et al. (2015). Isolation of a novel phage with activity against Streptococcus mutans biofilms. PLOS ONE 10, e0138651. doi:10.1371/journal.pone.0138651
Delisle, A. L., Guo, M., Chalmers, N. I., Barcak, G. J., Rousseau, G. M., and Moineau, S. (2012). Biology and genome sequence of Streptococcus mutans phage M102AD. Appl. Environ. Microbiol. 78, 2264–2271. doi:10.1128/AEM.07726-11
Delisle, A. L., and Rostkowski, C. A. (1993). Lytic bacteriophages of Streptococcus mutans. Curr. Microbiol. 27, 163–167. doi:10.1007/BF01576015
Garrett, S. C. (2021). Pruning and tending immune memories: Spacer dynamics in the CRISPR array. Front. Microbiol. 12, 664299. doi:10.3389/fmicb.2021.664299
Gong, T., Tang, B., Zhou, X., Zeng, J., Lu, M., Guo, X., et al. (2018). Genome editing in Streptococcus mutans through self-targeting CRISPR arrays. Mol. Oral Microbiol. 33, 440–449. doi:10.1111/omi.12247
Gophna, U., Kristensen, D. M., Wolf, Y. I., Popa, O., Drevet, C., and Koonin, E. V. (2015). No evidence of inhibition of horizontal gene transfer by CRISPR–Cas on evolutionary timescales. ISME J. 9, 2021–2027. doi:10.1038/ismej.2015.20
Guindon, S., and Gascuel, O. (2003). A simple, fast, and accurate algorithm to estimate large phylogenies by maximum likelihood. Syst. Biol. 52, 696–704. doi:10.1080/10635150390235520
Hanada, N., and Kuramitsu, H. K. (1988). Isolation and characterization of the Streptococcus mutans gtfC gene, coding for synthesis of both soluble and insoluble glucans. Infect. Immun. 56, 1999–2005. doi:10.1128/iai.56.8.1999-2005.1988
Hordijk, W., and Gascuel, O. (2005). Improving the efficiency of SPR moves in phylogenetic tree search methods based on maximum likelihood. Bioinformatics 21, 4338–4347. doi:10.1093/bioinformatics/bti713
Horvath, P., Romero, D. A., Coûté-Monvoisin, A.-C., Richards, M., Deveau, H., Moineau, S., et al. (2008). Diversity, activity, and evolution of CRISPR loci in Streptococcus thermophilus. J. Bacteriol. 190, 1401–1412. doi:10.1128/JB.01415-07
Johnson, C. M., and Grossman, A. D. (2015). Integrative and conjugative elements (ICEs): What they do and how they work. Annu. Rev. Genet. 49, 577–601. doi:10.1146/annurev-genet-112414-055018
Kajfasz, J. K., Martinez, A. R., Rivera-Ramos, I., Abranches, J., Koo, H., Quivey, R. G., et al. (2009). Role of clp proteins in expression of virulence properties of Streptococcus mutans. J. Bacteriol. 191, 2060–2068. doi:10.1128/JB.01609-08
Khan, R., Rukke, H. V., Høvik, H., Åmdal, H. A., Chen, T., Morrison, D. A., et al. (2016). Comprehensive transcriptome profiles of Streptococcus mutans UA159 map core streptococcal competence genes. mSystems 1, e00038–15. doi:10.1128/mSystems.00038-15
Kolesnik, M. V., Fedorova, I., Karneyeva, K. A., Artamonova, D. N., and Severinov, K. V. (2021). Type III CRISPR-cas systems: Deciphering the most complex prokaryotic immune system. Biochemistry. 86, 1301–1314. doi:10.1134/S0006297921100114
Lemos, J., Palmer, S., Zeng, L., Wen, Z., Kajfasz, J., Freires, I., et al. (2019). The Biology of Streptococcus mutans. Microbiol. Spectr. 7. doi:10.1128/microbiolspec.GPP3-0051–201810.1128/microbiolspec.GPP3-0051-2018
Letunic, I., and Bork, P. (2021). Interactive tree of life (iTOL) v5: an online tool for phylogenetic tree display and annotation. Nucleic Acids Res. 49, W293–W296. doi:10.1093/nar/gkab301
Liu, M., Li, X., Xie, Y., Bi, D., Sun, J., Li, J., et al. (2019). ICEberg 2.0: an updated database of bacterial integrative and conjugative elements. Nucleic Acids Res. 47, D660–D665. doi:10.1093/nar/gky1123
Meng, P., Lu, C., Zhang, Q., Lin, J., and Chen, F. (2017). Exploring the genomic diversity and cariogenic differences of Streptococcus mutans strains through pan-genome and comparative genome analysis. Curr. Microbiol. 74, 1200–1209. doi:10.1007/s00284-017-1305-z
Mosterd, C., and Moineau, S. (2020). Characterization of a type II-A CRISPR-cas system in Streptococcus mutans. mSphere 5, e00235–20. doi:10.1128/mSphere.00235-20
Münch, P. C., Franzosa, E. A., Stecher, B., McHardy, A. C., and Huttenhower, C. (2021). Identification of natural CRISPR systems and targets in the human microbiome. Cell Host Microbe 29, 94–106. doi:10.1016/j.chom.2020.10.010
Page, A. J., Cummins, C. A., Hunt, M., Wong, V. K., Reuter, S., Holden, M. T. G., et al. (2015). Roary: rapid large-scale prokaryote pan genome analysis. Bioinformatics 31, 3691–3693. doi:10.1093/bioinformatics/btv421
Palmer, K. L., and Gilmore, M. S. (2010). Multidrug-resistant Enterococci lack CRISPR-cas. mBio 1, e00227–10. doi:10.1128/mBio.00227-10
Pursey, E., Dimitriu, T., Paganelli, F. L., Westra, E. R., and van Houte, S. (2022). CRISPR-Cas is associated with fewer antibiotic resistance genes in bacterial pathogens. Philos. Trans. R. Soc. Lond. B Biol. Sci. 377, 20200464. doi:10.1098/rstb.2020.0464
R Core Team (2015). R: a language and environment for statistical computing Available at: https://www.gbif.org/tool/81287/r-a-language-and-environment-for-statistical-computing [Accessed April 12, 2022].
Rheinberg, A., Swierzy, I. J., Nguyen, T. D., Horz, H.-P., and Conrads, G. (2013). Cryptic Streptococcus mutans 5.6-kb plasmids encode a toxin–antitoxin system for plasmid stabilization. J. Oral Microbiol. 5, 19729. doi:10.3402/jom.v5i0.19729
Richards, V. P., Palmer, S. R., Pavinski Bitar, P. D., Qin, X., Weinstock, G. M., Highlander, S. K., et al. (2014). Phylogenomics and the dynamic genome evolution of the genus Streptococcus. Genome Biol. Evol. 6, 741–753. doi:10.1093/gbe/evu048
Seemann, T. (2014). Prokka: rapid prokaryotic genome annotation. Bioinformatics 30, 2068–2069. doi:10.1093/bioinformatics/btu153
Serbanescu, M. A., Cordova, M., Krastel, K., Flick, R., Beloglazova, N., Latos, A., et al. (2015). Role of the Streptococcus mutans CRISPR-cas systems in immunity and cell physiology. J. Bacteriol. 197, 749–761. doi:10.1128/JB.02333-14
Shields, R. C., Walker, A. R., Maricic, N., Chakraborty, B., Underhill, S. A. M., and Burne, R. A. (2020). Repurposing the Streptococcus mutans CRISPR-cas9 system to understand essential gene function. PLoS Pathog. 16, e1008344. doi:10.1371/journal.ppat.1008344
Song, L., Wang, W., Conrads, G., Rheinberg, A., Sztajer, H., Reck, M., et al. (2013). Genetic variability of mutans streptococci revealed by wide whole-genome sequencing. BMC Genomics 14, 430. doi:10.1186/1471-2164-14-430
Touchon, M., Charpentier, S., Clermont, O., Rocha, E. P. C., Denamur, E., and Branger, C. (2011). CRISPR distribution within the Escherichia coli species is not suggestive of immunity-associated diversifying selection. J. Bacteriol. 193, 2460–2467. doi:10.1128/JB.01307-10
van der Ploeg, J. R. (2009). Analysis of CRISPR in Streptococcus mutans suggests frequent occurrence of acquired immunity against infection by M102-like bacteriophages. Microbiol. Read. Engl.) 155, 1966–1976. doi:10.1099/mic.0.027508-0
van der Ploeg, J. R. (2007). Genome sequence of Streptococcus mutans bacteriophage M102. FEMS Microbiol. Lett. 275, 130–138. doi:10.1111/j.1574-6968.2007.00873.x
Wheatley, R. M., and MacLean, R. C. (2021). CRISPR-Cas systems restrict horizontal gene transfer in Pseudomonas aeruginosa. ISME J. 15, 1420–1433. doi:10.1038/s41396-020-00860-3
Yu, G., Smith, D. K., Zhu, H., Guan, Y., and Lam, T. T.-Y. (2017). ggtree: an r package for visualization and annotation of phylogenetic trees with their covariates and other associated data. Methods Ecol. Evol. 8, 28–36. doi:10.1111/2041-210X.12628
Zaken, H. B., Kraitman, R., Coppenhagen-Glazer, S., Khalifa, L., Alkalay-Oren, S., Ben-Gal, G., et al. (2021). Isolation and characterization of anti-Streptococcus mutans phage as a possible treatment agent for caries. doi:10.20944/preprints202104.0045.v1
Zeng, L., Das, S., and Burne, R. A. (2010). Utilization of lactose and galactose by Streptococcus mutans: Transport, toxicity, and carbon catabolite repression. J. Bacteriol. 192, 2434–2444. doi:10.1128/JB.01624-09
Keywords: Streptococcus mutans, CRISPR, horizontal gene transfer, integrative and conjugative element, pangenome, CRISPR-Cas
Citation: Walker AR and Shields RC (2022) Investigating CRISPR spacer targets and their impact on genomic diversification of Streptococcus mutans. Front. Genet. 13:997341. doi: 10.3389/fgene.2022.997341
Received: 18 July 2022; Accepted: 17 August 2022;
Published: 15 September 2022.
Edited by:
David W. Ussery, University of Arkansas for Medical Sciences, United StatesReviewed by:
Kesavan Babu, UT Southwestern Medical Center, United StatesKurt Selle, Locus Biosciences Inc., United States
Copyright © 2022 Walker and Shields. This is an open-access article distributed under the terms of the Creative Commons Attribution License (CC BY). The use, distribution or reproduction in other forums is permitted, provided the original author(s) and the copyright owner(s) are credited and that the original publication in this journal is cited, in accordance with accepted academic practice. No use, distribution or reproduction is permitted which does not comply with these terms.
*Correspondence: Robert C. Shields, cnNoaWVsZHNAYXN0YXRlLmVkdQ==
†ORCID: Alejandro R. Walker, orcid.org/0000-0002-5839-6584; Robert C. Shields, orcid.org/0000-0002-8823-9504