- 1State Key Laboratory of Oral Diseases and National Clinical Research Center for Oral Diseases, West China Hospital of Stomatology, Sichuan University, Chengdu, China
- 2Department of Orthodontics, West China Hospital of Stomatology, Sichuan University, Chengdu, China
- 3Department of Temporomandibular Joint, West China Hospital of Stomatology, Sichuan University, Chengdu, China
- 4Hospital of Stomatology, Zunyi Medical University, Zunyi, China
Osteoarthritis (OA) is the most prevalent articular disease, especially in aged population. Caused by multi-factors (e.g., trauma, inflammation, and overloading), OA leads to pain and disability in affected joints, which decreases patients’ quality of life and increases social burden. In pathophysiology, OA is mainly characterized by cartilage hypertrophy or defect, subchondral bone sclerosis, and synovitis. The homeostasis of cell–cell communication is disturbed as well in such pro-inflammatory microenvironment, which provides clues for the diagnosis and treatment of OA. MicoRNAs (miRNAs) are endogenous non-coding RNAs that regulate various processes via post-transcriptional mechanisms. The miR-17-92 cluster is an miRNA polycistron encoded by the host gene called MIR17HG. Mature miRNAs generated from MIR17HG participate in biological activities such as oncogenesis, neurogenesis, and modulation of the immune system. Accumulating evidence also indicates that the expression level of miRNAs in the miR-17-92 cluster is tightly related to the pathological processes of OA, such as chondrocyte apoptosis, extracellular matrix degradation, bone remodeling, and synovitis. In this review, we aim to summarize the roles of the miR-17-92 cluster in the underlying molecular mechanism during the development and progression of OA and shed light on the new avenue of the diagnosis and treatment of OA.
Introduction
Osteoarthritis (OA) is the most prevalent joint disease, which is becoming an increasing social-economic problem worldwide (Bortoluzzi et al., 2018; Peat and Thomas, 2021). It has been estimated that over 3% people are troubled with symptomatic OA in the world (Katz et al., 2021). Clinically, to relieve pain and joint disability, two leading symptoms caused by inflammatory microenvironment and articular tissue destruction, a comprehensive plan for OA management is required (Jang et al., 2021; Sharma, 2021). Patient education and lifestyle change should be applied first if necessary (Hunter and Bierma-Zeinstra, 2019). Pharmaceutical therapy (e.g., nonsteroidal anti-inflammatory drugs (NSAIDs), hyaluronic acid, protease inhibitors, and bisphosphonates), via oral medication or intra-articular injection, could alleviate the pain and promote tissue repair to some extent (Conaghan et al., 2019; Jones et al., 2019; Latourte et al., 2020). If the lesions advance progressively and the aforementioned conservative methods do not work, total joint replacement might be the only choice currently (Ferguson et al., 2018; Price et al., 2018).
MicroRNAs (miRNAs) are a class of endogenous non-coding RNAs with about 22 nucleotides in length, which regulate the expression of genes via the post-transcriptional mechanism (Bartel, 2004). Numerous functions have been ascribed to miRNAs in various physiological and pathological activities during these decades (Liu et al., 2014; Galagali and Kim, 2020). For example, miR-125, mammalian ortholog of the first reported animal miRNA lin-4, was found to regulate cancers and cardiovascular and cerebrovascular diseases (Peng et al., 2021; Wang et al., 2021). The well-known let-7 was originally found in Caenorhabditis elegans by Reinhart et al. (2000), following which researchers identified that this miRNA is highly evolutionarily conserved across species and functions in tumor suppressing, organism development, and stem cell differentiation (Thornton and Gregory, 2012; Ventayol et al., 2014; Lee et al., 2016). The biogenesis of miRNA is a complicated and precisely regulated process (Lin and Gregory, 2015; Lee et al., 2016; Ali Syeda et al., 2020). In brief, primary miRNA (pri-miRNA) is first synthesized in the nucleus with a hairpin stem–loop structure. The Drosha/DGCR8 complex cleaves the pri-miRNA into a 60–70-nucleotide pre-miRNA, and exportin 5 subsequently translocates the pre-miRNA into cytoplasm. In cytoplasm, pre-miRNA is processed into a double-stranded RNA (dsRNA) with ∼22 base pairs in length by Dicer. One strand of the dsRNA is loaded on the Argonaute (AGO) to form an RNA-induced silencing complex (RISC), which has the potential to regulate various biological processes by binding to the 3′ untranslated region (3′UTR) of target genes. miRNAs regulate gene expression via two mechanisms: suppressing protein translation by inhibiting its initiation or elongation and promoting the cleavage or degradation of mRNAs (Saliminejad et al., 2019).
miR-17-92 is a classical polycistronic cluster, and its roles in tumor and normal development were extensively studied (Chen et al., 2013; Mogilyansky and Rigoutsos, 2013; Murphy et al., 2013). A recent study also reported the relationship between miR-17-92 and OA. For example, Kong et al. (2017) collected the plasma samples of OA patients and health control for miRNA detection and analysis. miR-19b-3p and miR-92a-3p, constituents of the miR-17-92 cluster, were among the eight top differentially expressed miRNAs. In the present review, we aim to summarize the current knowledge about the underlying molecular mechanism of the miR-17–92 cluster during the development and progression of OA and shed light on the new avenue of the diagnosis and treatment of OA.
The pathology of OA
Normally, articular cartilage coordinates with subchondral bone and synovial membrane to keep the joint in homeostasis (Zhen and Cao, 2014). The cartilage buffers external stress, while the subchondral bone transmits and absorbs the mechanical loading, and synovial fluid lubricates the joint and provides the avascular cartilage with nutrition and biological cues (Madry, 2010; Lories and Luyten, 2011). Considering their interplay under the physiological condition, it is natural to hold the belief that the progression of OA is not just questions about the cartilage. During the onset and progression of OA, loss of cartilage causes excessive loading on subchondral bone, which subsequently leads to bone lesions such as microfracture, necrosis, and fibrosis (Zhen and Cao, 2014). With the constant production and secretion of pro-inflammatory cytokines, nearby synovium, ligament, and even periarticular muscles will be affected (Katz et al., 2021).
Mechanistically, the apoptosis and hypertrophy of chondrocyte occur during OA progression, and the normal structure of the matrix is destructed by matrix metalloproteinases (MMPs), a disintegrin and metalloproteinase with thrombospondin motifs (ADAMTS); blood vessels from bone marrow invade through the osteochondral junction into the calcified cartilage zone, leading to endochondral osteogenesis (Karsdal et al., 2014; Goldring and Goldring, 2016). In subchondral bone, bone turnover is enhanced during the early OA stage, which causes the subchondral bone becoming thinner (Hu et al., 2021). During the late stage, growth factors like transforming growth factor beta (TGF-β) and bone morphogenetic proteins (BMPs), which promote matrix production and protect joints from damage under physiological conditions, would stimulate osteophyte production and subchondral bone sclerosis under such abnormal environment (Hu et al., 2021). In synovium, chemokines are secreted to promote monocyte/macrophage infiltration, which stimulates excessive proliferation of synovial fibroblasts and production of inflammatory cytokines (Kapoor et al., 2011; Sanchez-Lopez et al., 2022). The aforementioned pathological processes of OA have been sketched in Figure 1.
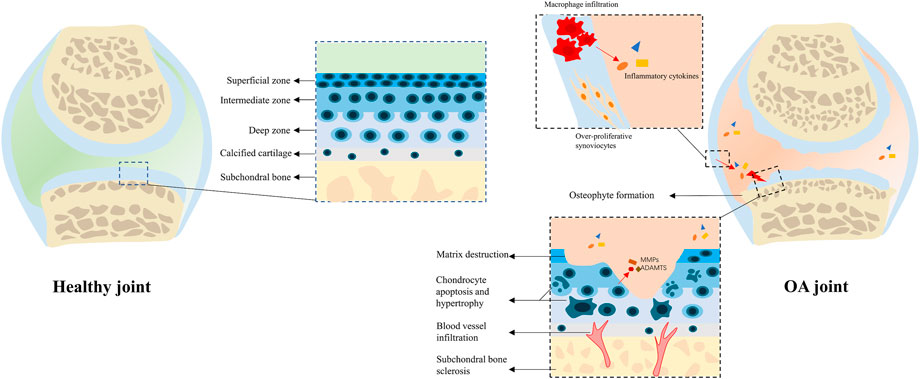
FIGURE 1. Physiological and pathological structure of the joint. In a healthy joint, the cartilage coordinates with subchondral bone, synovial membrane, and other peri-articular tissues to keep the joint in homeostasis. In an OA joint, chondrocyte hypertrophy and apoptosis occur with cartilage matrix degradation; subchondral bone changes including sclerosis, osteophyte formation, and blood vessel infiltration can also be observed; synoviocytes are over-proliferative, and plenty of macrophages infiltrate into the synovial membrane to secret pro-inflammatory cytokines, which deteriorate the microenvironment.
The miR-17-92 cluster and its biogenesis
The miR-17-92 cluster (also known as oncomir-1) is an important miRNA polycistron located in the non-protein-coding region of chromosome 13 open reading frame 25 (C13orf25), which is also referred to as the miR-17-92 host gene (MIR17HG) (Mogilyansky and Rigoutsos, 2013). There are six mature miRNAs generated from the miR-17-92 cluster transcript: miR-17, miR-18a, miR-19a, miR-20a, miR-19b-1, and miR-92a-1. These miRNAs were reported to regulate biological processes including oncogenesis, neurogenesis, and immune system modulation (Mendell, 2008; Osada and Takahashi, 2011; Baumjohann, 2018). The miR-106a-363 cluster (located on chromosome X) and miR-106b-25 cluster (located on chromosome 7) are two paralogs of the miR-17-92 cluster in the human genome (Petrocca et al., 2008; Gruszka and Zakrzewska, 2018). The former cluster contains six miRNAs: miR-106a, miR-18b, miR-20b, miR-19b-2, miR-92a-2, and miR-363. The latter one contains three miRNAs: miR-106b, miR-93, and miR-25. According to their seed sequence, miRNAs in these three clusters are categorized into four groups: the miR-17 family, the miR-18, the miR-19, and the miR-92 family (see Figures 2A,B).
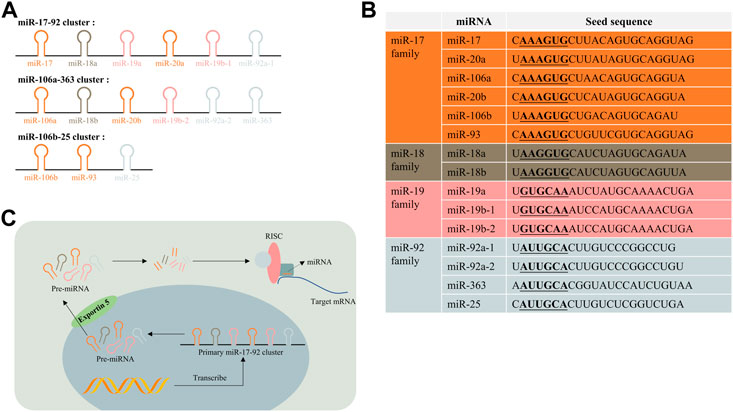
FIGURE 2. miR-17-92 cluster and their paralogs. (A) miR-17-92 cluster, miR-106a-363 cluster, and miR-106b-25 cluster are three paralogs in the human genome; (B) they can be classified into four subfamilies based on their seed sequence; (C) Biogenesis of the miR-17-92 cluster. RISC, RNA-induced silencing complex.
The biogenesis of the miR-17-92 cluster is the same as processes of other miRNAs, except there contains multiple stem–loop structures to form six miRNAs in a single transcript (Olive et al., 2010) (see Figure 2C). The production of these miRNAs could be regulated by a class of transcriptional factors. Members of the miR-17-92 and miR-106a-363 cluster were consistently observed to be upregulated when the transcription factor c-Myc was elevated in multiple cell types, and subsequent experiments validated that c-Myc could directly bind to miR-17-92 genomic locus-MIR17HG (O’Donnell et al., 2005). The amplification of another oncogenic gene n-Myc was discovered to upregulate miR-17-92 via transcription regulation (Schulte et al., 2008). Other transcription factors from the E2F transcription factor family (e.g., E2F1, 2, and 3) and ETS transcription factor family (e.g., Spi-1 and Fli-1) were reported to participate in the regulation of miR-17-92 biogenesis as well (Woods et al., 2007; Kayali et al., 2012). In addition, post-transcriptional factor like the tertiary structure of transcripts could also influence the biogenesis of miRNAs (Chaulk et al., 2011; Chakraborty et al., 2012). However, mechanisms of how transcription factor network and post-transcriptional factors control the biogenesis of the miR-17-92 cluster remains elucidated even with the knowledge mentioned previously.
miR-17-92 in cartilage degradation
Articular cartilage is composed of chondrocytes along with their surrounding matrix, which features the joints with the load-bearing characteristic (Guilak et al., 2018). However, the cartilage has weak ability to self-repair in response to chronic or acute pathological stimuli due to its shortage of blood supply (Makris et al., 2015; Robinson et al., 2016). Hence, proper interventions are necessary for cartilage regeneration when it is destructed. Exploring mechanisms of cartilage repair is a vital path for OA treatment (Goldring and Marcu, 2012). Interleukin-1beta (IL-1β) and lipopolysaccharide (LPS) are the two most common factors used for in vitro OA phenotype induction (Wang et al., 2019; Zhang H et al., 2021), and members of the miR-17-92 cluster were found to attenuate or promote OA progression in such an in vitro OA model. For example, miR-17a-5p, miR-19b-3p, and miR-92a-3p were reported to prevent OA progression in IL-1β-induced chondrocyte apoptosis and extracellular matrix (ECM) degradation (Mao et al., 2017a; Duan et al., 2019; Li Y et al., 2020). Under LPS induction, miR-20a inhibitor attenuated pro-inflammatory response of chondrocytes (Zhao and Gong, 2019). Based on current research, multiple signaling pathways (e.g., mitogen-activated protein kinase (MAPK) pathway, TGF-β pathway, Wnt/β-catenin, and nuclear factor kappa-B (NF-κB)) have been found to participate in the chondrocyte and ECM metabolism (van der Kraan, 2017; Choi et al., 2019; Shang et al., 2021; Li et al., 2022) (Table 1) (See Figure 3).
MAPK pathway
MAPK is a class of serine/threonine-protein kinases, including three main types of subfamily members: the extracellular-signal-regulated kinases (ERKs), the c-jun N-terminal kinase (JNKs), and the p38 kinases (Lee et al., 2020; Sinkala et al., 2021). They can transfer the extracellular signals into the nucleus via the MAP3K–MAP2K–MAPK cascade to participate in cellular activities like proliferation, differentiation, and apoptosis (Chen et al., 2018; Ostojic et al., 2021). It was reported that bone marrow stem cell-derived extracellular vesicles (BMSC-EVs) could alleviate the IL-1β-induced chondrocyte inflammatory phenotype (Zhang B et al., 2022), and such effect was further improved with the treatment of BMSC-EV generated in a hypoxic environment. RNA sequencing showed that 12 miRNAs were aberrantly expressed in hypoxia BMSC-EV, in which miR-18a was downregulated, indicating its involvement in exosome-treated OA progress, and Kyoto Encyclopedia of Genes and Genomes (KEGG) analysis showed that such progress may be mediated by the MAPK pathway (Zhang Y et al., 2022). Chen et al. (2017) found that lipopolysaccharide (LPS) promoted cellular injury and cytokine secretion of human articular chondrocytes, which was influenced by the lncHOTAIR-miR-17-3p-ETV1 network. Knockdown of HOTAIR and ETV1 or overexpression of miR-17-3p could reverse LPS-mediated apoptosis, and subsequent results verified that the ceRNA regulatory triad regulated such process through activating MAPK signaling (Chen et al., 2017).
TGF-β pathway
The TGF-β superfamily consists of factors including TGF-β, BMPs, and growth and differentiation factors (GDFs), which regulate gene expression in an SAMD-dependent or SMAD-independent way (Thielen et al., 2019; Cherifi et al., 2021). Accumulating evidence has shown that the TGF-β pathway is tightly related to the osteoarthritis progression and cartilage regeneration (MacFarlane et al., 2017; Wang C et al., 2020; Wang G et al., 2020). Lian et al. (2020) reported that miR-18a was significantly upregulated in IL-β-induced rat knee OA models; in in vitro experiments, hypertrophic genes like COL10A1 and MMP13 increased in the miR-18a overexpression group after 14 days of chondrogenic differentiation and 14 days of hypertrophic induction. As cartilage hypertrophy is a crucial step during OA progression, miR-18a has the potential to regulate OA progress negatively. Gene Ontology (GO) analysis indicated the underlying relationship between miR-18a and TGF-β; bioinformatics found that there existed binding sites of miR-18a in the 3′UTR of TGF-β1, SMAD2, and SMAD3, which was validated by RIP assay and luciferase reporter assay (Lian et al., 2020).
Wnt/β-catenin pathway
The nomination of Wnt comes from the integrase-1 gene in mice and the wingless gene in Drosophila. Wnt gene regulates biological activities via two distinctive ways (namely, canonical pathway and noncanonical pathway). The noncanonical pathway includes the Wnt-Ca2+ pathway and Wnt-atypical protein kinase C pathway. The canonical pathway is dependent on β-catenin, so it is also called as the Wnt/β-catenin pathway. With regard to the Wnt/β-catenin pathway, in the absence of the extracellular Wnt signal, a compound that is comprised of axis inhibition protein (AXIN), adenomatous polyposis coli (APC), glycogen synthase kinase-3 (GSK-3), and casein kinase 1 (CK1) constantly degraded cytoplasmic β-catenin via the ubiquitination mechanism; when the ligand Wnt binds to the transmembrane receptor, cytoplasmic β-catenin will be released from the destruction complex and regulates target genes via translocating into the nucleus (Zhou et al., 2017; Shang et al., 2021). Except for its roles in embryonic development and cancers, the Wnt/β-catenin pathway is also tightly related to bone remodeling and cartilage homeostasis (Lietman et al., 2018; Sun J et al., 2020). Hu et al. (2018) discovered the HOTAIR–miR-17-5p–FUT2 cascade in regulating matrix degradation and cell apoptosis in IL-1β-induced experimental OA models. Due to the correlation between FUT family and Wnt/β-catenin signaling, they made the hypothesis that FUT2 participated in the Wnt/β-catenin pathway. Results showed that FUT2 upregulation significantly increased phosphorylated GSK-3β (member of the β-catenin destruction complex) expression and nuclear β-catenin accumulation, which are the signs of Wnt/β-catenin activation.
NF-κB pathway
RelA/p65, RelB, c-Rel, NF-κB1/p105, and NF-κB2/p100 are the five constituents of the NF-κB transcription factor family (Lepetsos et al., 2019). In the resting state, NF-κB dimers are inhibited via binding with NF-κB inhibitors (IκBs). When the NF-κB pathway is activated, IκBs are phosphorylated and degraded by IκB kinases (IKKs) to relieve NF-κB (Taniguchi and Karin, 2018). Finally, NF-κB is translocated into the nucleus to regulate downstream gene expression. The activation of NF-κB signaling provokes cartilage matrix degradation and chondrocyte hypertrophy via producing catabolic mediator (e.g., MMPs and aggrecanases) and inflammatory cytokines (e.g., IL-1β and tumor necrosis factor-alpha (TNF-α)) (Olivotto et al., 2015). Chen et al. (2017) reported that miR-17-3p mediated OA phenotype regulation via NF-κB signaling. From another research by Zhao and Gong (2019), it was found that IκBβ, a constituent of the IκB family, could be directly targeted by miR-20a and the inhibition of miR-20a could upregulate IκBβ, thus protecting chondrocytes via NF-κB signaling suppression.
Autophagy
Autophagy is a bio-protective mechanism in response to endogenous or exogenous stress (Xia et al., 2021). Intracellular components (e.g., pathogens, damaged organelles, and misfolded proteins) engulfed by autophagosomes are delivered to the lysosomal compartment for degradation and recycling (Dikic and Elazar, 2018). Three kinds of autophagy have been described currently (Mizushima and Levine, 2020; Kitada and Koya, 2021). Macroautophagy is the best understood type, where intracellular contents are first engulfed to form autophagosomes, which are fused with enzyme-enriched lysosomes to degrade contents in a selective or nonselective manner; microautophagy refers to the process in which cytoplasmic components are directly sequestered into lysosomes and chaperone-mediated autophagy selectively degrades proteins with the assistance of chaperone complexes (Mizushima and Levine, 2020; Kitada and Koya, 2021). The majority of human diseases like cancers, neurodegenerative disorders, and metabolism diseases are influenced by autophagy according to the research studies till date (Levy et al., 2017; Scrivo et al., 2018; Klionsky et al., 2021). Meanwhile, it was observed that autophagy was constantly activated under physiological articular cartilage while disturbed in aged and OA joints (Duarte, 2015; Li et al., 2016), hinting that they are tightly correlated.
Selective autophagy receptors mediate the selective degradation of aggregated proteins, dysfunctional mitochondria, and pathogens during macroautophagy. The deficiency of p62 (also referred to as sequestosome-1), a prototype autophagy receptor, leads to ubiquitinated protein accumulation and mitochondrial defects via impairing the selectivity of autophagy (Aparicio et al., 2020). Decreased miR-17-5p expression and suppressed autophagy were concurrently detected in the experimental mouse OA model (Li et al., 2018). The 3′-UTR of p62 was found to contain a conserved binding site with miR-17-5p, which was validated by luciferase reporter assay. However, deeper investigations on the underlying mechanisms of the miR-17-92/p62 axis and its effect on OA relief remain to be elucidated.
It was found that the miR-17-92 cluster was all decreased during ATDC5 cell chondrogenic differentiation (Xu et al., 2019). Among the six members, miR-20a showed the most significant inhibition trend on chondrogenesis. The mRFP-GFP-LC3 adenoviral vector was transfected into ATDC5 cells to trace the dynamic autophagic activities. At different induction time points (i.e., 24, 36, 48, and 72 h), increased red and yellow puncta in the chondrogenic group indicated the active state of autophagy, which can be blocked by miR-20a (Xu et al., 2019). Dozens of autophagy-related proteins were discovered in controlling the dynamics of membranes during autophagy (Xie et al., 2015). For instance, Atg5 along with Atg12/Atg16 is essential in autophagosome formation (Romanov et al., 2012; Sheng et al., 2018); Atg7 dysregulation significantly influenced the COL Ⅱ synthesis and secretion (Cinque et al., 2015). Xu et al. (2019) reported that disruption of Atg7 could reverse the promotion effect of miR-20a inhibitor on ATDC5 chondrogenic differentiation and autophagic vacuole production.
Histone modification
Histones are alkaline proteins that envelope DNA. Histone modification (e.g., acetylation, methylation, and phosphorylation) alters the conformation of chromatin and influences transcription of genes (Stoll et al., 2018; Zhang Y et al., 2021). Histone deacetylases (HDACs) were reported to regulate chondrogenesis and cartilage degradation via deacetylating nucleosomal histone (Carpio and Westendorf, 2016; Meng et al., 2018). miR-92a-3p and HDAC2 were aberrantly expressed during MSC chondrogenesis and OA progression, and the luciferase reporter assay confirmed their interaction (Mao et al., 2017b). Moreover, the acetylation of histone H3 on Col Ⅱ and aggrecan promoter was enhanced by miR-92a-5p, contributing to the process of cartilage protection. Enhancer of zeste homolog 2 (EZH2), a histone methyltransferase, was upregulated in OA cartilage (Li Z et al., 2020), hinting its vital roles in OA pathogenesis. However, such effect can be attenuated by upstream miR-17-5p.
Others
Long non-coding RNAs (lncRNAs) are a class of non-coding endogenous RNAs with over 200 nucleotides in length (Herman et al., 2022). Cytoplastic lncRNAs usually function as a miRNA sponge to regulate downstream target genes via classical ceRNA mechanisms (Thomson and Dinger, 2016). The roles of lncRNAs during OA-related ECM degradation and chondrocyte apoptosis were identified as well (Ghafouri-Fard et al., 2021). Hu et al. (2018) transfected chondrocytes with the HOTAIR pcDNA3.1 vector and showed that the overexpression of HOTAIR significantly promoted chondrocyte apoptosis and synthesis of cartilage-degrading enzymes (i.e., MMP13 and ADAMTS-5), while ECM-formation genes like COLⅡ and aggrecan were inhibited in the IL-1β-induced inflammatory environment. The addition of its target miR-17-5p could reverse such a pro-osteoarthritis trend via the FUT2-Wnt/β-catenin pathway. Another research discovered that the HOTAIR-miR-17-3p axis had the potential to influence LPS-induced OA (Chen et al., 2017). TUG1, an lncRNA related to cell apoptosis, was reported to target miR-17-5p (Li2 et al., 2020). Differentiation antagonizing non-protein coding RNA (DANCR) is an oncogenic lncRNA. Except for its vital roles in cancer progression, DANCR was reported to participate in stem cell differentiation. Li et al. (2021) found that si-DANCR could protect human chondrocytes from LPS-induced apoptosis, and miR-19a-3p was a vital mediator.
miR-17-92 in subchondral bone remodeling
Subchondral bone refers to the cortical plate and subchondral cancellous bone beneath the calcified cartilage (Burr and Gallant, 2012). Under physiological conditions, the viscoelastic characteristic of articular cartilage allows the maximum contact area to avoid stress concentration when mechanical stress is loaded, and the subchondral plate and cancellous bone play a role in stress transmission (Burr and Gallant, 2012). In the early stage of OA, the thickness of the subchondral plate is reduced as bone remodeling is accelerated. In the late stage, subchondral bone sclerosis (hallmark of OA progression) occurs, and the increase of stiffness and density will lead to stress concentration and exacerbate overlying cartilage deterioration in return (Hu et al., 2021).
Sun X et al. (2020) discovered that miR-29b was downregulated in BMSCs extracted from condylar subchondral bone of the OA mouse model, and knockdown of this miRNA significantly rescued the subchondral bone loss and cartilage degradation, which means miR-29b functions as a vital mediator during articular cartilage and subchondral bone physiopathological change. As for members of the miR-17-92 cluster, it was reported that the expression level of miR-17-3p was low during BMP2-induced osteogenesis. SRY-box transcription factor 6 (SOX6), a transcription factor related to alteration of bone mineral density, was discovered to contain binding sites of miR-17-3p (Chen et al., 2020). The promotion effect of miR-17-3p inhibitor on osteoblast differentiation could be partially reversed by si-SOX6, which shows that miR-17-3p–SOX6 might be the candidate molecular mechanism for OA subchondral bone treatment. However, mechanisms that show how the miR-17-92 cluster is involved in the changes of OA subchondral bone turnover and its communication with overlying cartilage are still obscure.
miR-17-92 in periarticular tissue destruction
Synovitis
Synovitis predominant with T cells characterizes the autoimmune disease—rheumatoid arthritis (RA) (Giannini et al., 2020). As for OA, synovitis featured by macrophage infiltration emerges with disease progression and subsequently deteriorates the articular microenvironment to aggravate cartilage and subchondral bone lesions (Sellam and Berenbaum, 2010; Mathiessen and Conaghan, 2017).
Kuo et al. (2019) reported that treating OA synovial fibroblasts (OASFs) with TGF-β1 diminished the synthesis of TNF-α, IL-1β, vascular endothelial growth factor (VEGF), and C–C motif chemokine ligand 2 (CCL2) and stimulated forkhead box O3 (FOXO3) expression, which means FOXO3 mediates the TGF-β1-stimulated anti-inflammation effect. Bioinformatics prediction and functional experiments convinced that miR-92a was a molecule upstream of FOXO3, indicating that the inhibition of miR-92a was essential for FOXO3 release and thus alleviated OASF inflammation. It was reported that the secretion of chemokines (e.g., CCL2/MCP-1 and CCL3/MIP-1α) decreased when synoviocytes were co-cultured with micro-fragmented adipose tissue (MF) (Shi et al., 2022). Given that chemokines are crucial factors mediating monocytes/macrophage infiltration and subsequent inflammatory cytokine production in synovial tissues and fluids (Raghu et al., 2017), the aforementioned results indicate the potential treatment effects of MF on OA synovitis. Mechanistically, miR-92a-3p was detected to be downregulated in TNF-α-treated synoviocytes, and knockdown of miR-92a-3p overturned the protective effect of co-cultured MF upon synovitis (Shi et al., 2022), which contradicts the results from Kuo et al. (2019). Hence, more high-quality evidence is required to illustrate the roles of miR-92a in synovitis. In the rat OA model from another researcher (Feng et al., 2022), upon injecting adeno-associated virus (AAV)–miR-18a-3p, the proliferation of synovial membrane cells was inhibited, accompanied by the reduction of inflammatory cells.
With the OA progression, pro-inflammatory cytokines could affect periarticular ligaments, which contributes to symptoms like pain and articular immobility (Katz et al., 2021). Li et al. (2017) reported the miRNA microarray results of knee anterior cruciate ligaments (ACLs) with or without OA, showing that OA progression significantly downregulated the expression of miR-18a in ACL.
miR-17-92 in OA treatment
Stem cell therapy
Tissue engineering is a novel technology aimed at different kinds of tissue repair and regeneration with the compound of seed cells, biocompatible materials, and bioactive molecules (Yang et al., 2017; Armiento et al., 2018). During the development of these years, its great success and huge potential attract more and more researchers.
The global change of miRNA expression during chondrogenesis of human adipose-derived stem cells (hADSCs) was detected by microarray chips (Zhang et al., 2012). Hundreds of differentially expressed miRNAs were found after chondrogenic differentiation, among which miR-92a was upregulated. However, the results of aforementioned research studies were not extended to in vivo validation, which obscures the roles of miR-92a in stem cell therapy for the treatment of OA.
Exogenous and endogenous miRNA promotion
Agomir/antagomir is usually used for promoting exogenous gene expression in experimental animal models. Agomir-17 injection reversed the high expression level of OA markers (i.e., MMP13, ADAMTS5, and nitric oxide synthase 2 (NOS2)) in mouse destabilization of the medial meniscus (DMM) model (Zhang B et al., 2022). Moreover, Lian et al. (2020) reported that in the combination of antagomir-18a and IL-1β inhibitor, better effects were observed on the suppression of chondrocyte hypertrophy and cartilage degradation than single anti-cytokine therapy, which means the injection of antagomir-18a sensitizes OA chondrocytes to anti-inflammatory cytokine and significantly promotes OA treatment.
From another research, AAV–miR-18a-3p was injected to promote exogenous miR-18a-3p expression in the rat knee OA model (Feng et al., 2022). Upon localized miR-18a-3p overexpression, the over-proliferation of synovial cells and the infiltration of inflammatory cells were significantly alleviated, and cytokines like IL-6 and PGE2, which are responsible for cartilage degradation and bone turnover deterioration, were in the low serum level in the miR-18a-3p group. To promote endogenous miR-17 expression, the intra-articular injection of GDF-5 was administered into the animal OA models (Zhang Y et al., 2022), which showed the significant effect of attenuating the knee OA phenotype.
Extracellular vesicle
Extracellular vesicles (EVs) are nanoparticles carrying bioactive molecules (e.g., proteins, mRNA, and miRNA) with a lipid bilayer structure, and EVs can be categorized into three subtypes based on their biogenesis processes and surface markers (namely, microvesicles, exosome, and apoptotic bodies) (van Niel et al., 2018; Mathieu et al., 2019). Their release and transfer play a role in intercellular communication, extensively participating in pathophysiological situations, such as cancer, metabolic diseases, and inflammatory responses (Akbar et al., 2019; Harrell et al., 2019; Walker et al., 2019). Meanwhile, EV-based therapy is an ideal substitute for MSC-based tissue regeneration due to its advantage of avoiding tumorigenesis and immunological rejection (Nagelkerke et al., 2021). For instance, exosomal miR-320c from human bone marrow MSCs (hBMSCs) reversed SOX9 downregulation and MMP13 upregulation in OA chondrocytes, and such effects were enhanced by exosomes from miR-320c-overexpressed hBMSCs (Sun et al., 2019).
MSC exosomes (MSC-Exos) with or without chondrogenic differentiation were collected for miRNA microarray (Mao et al., 2018). It was detected that 141 miRNAs were differentially expressed, among which miR-92a-3p was nearly eight-fold higher in the chondrogenic group. miR-92a-3p-overexpressed MSC-Exos (MSC-miR-92a-3p-Exos) were found to promote chondrocyte proliferation and MSC chondrogenesis. With MSC-miR-92a-3p-Exo injection on 7, 14, and 21 days, OA-related cartilage matrix degradation was significantly alleviated, while MMP13 expression was reduced compared to the OA mouse model without treatment.
Zhang B et al. (2022) isolated EVs secreted by bone marrow stem cells (BMSCs); subsequent validation confirmed that they were approximately 150-nm oval particles with positive EV surface marker expression. After uptake of BMSC-EVs, the OA phenotype of chondrocytes was surprisingly relieved, and EVs collected from BMSCs incubated in a hypoxic environment showed even better therapeutic efficacy on inflammatory chondrocytes. In the rat OA model, injection of BMSC-EVs protected joint from cartilage degradation, and OARSI scores in the hypoxia-EV group was significantly lower than those in OA and OA + normal EV groups, which indicates the excellent OA-treating function of hypoxia-preconditioned EVs (Zhang Y et al., 2022). To clarify the bioactive contents in these nanoparticles, miRNA profiling was implemented and miR-18a-3p was found as one of the downregulated miRNAs in hypoxia-EVs (Zhang B et al., 2022) (Table 2).
Conclusion
The onset and progression of OA are complex processes related to multiple tissues—cartilage, subchondral bone, synovium, and periarticular ligament. The pathological transformation of such tissues will affect each other, thus deteriorating the microenvironment in a vicious circle. miRNAs are one of the best understood mediators that epigenetically regulate physiological and pathological activities across species. As one miRNA could target a great number of downstream genes and a single mRNA could be complementarily blocked by various miRNAs, the multifaceted function and regulatory network would be complex even if functional redundancy exists. Although the participation of the miR-17-92 cluster in OA was extensively explored, the regulatory network construction of the six constituents remains a lot of work to perform.
As for the whole picture of the miR-17-92 cluster, several problems are still required for clarification. First, researchers up to date mostly focus on the roles of miR-17-92 in a single tissue of OA joint, and little is known about communication between different articular tissues (e.g., overlying cartilage and subchondral bone; synovium and cartilage), which is vital for joint homeostasis and equally important in joint diseases. Thus, more investigations are required to explore the function of the miR-17-92 cluster during such cross-talk in the future. Then, a well-designed in vivo experiment and a medication delivery system are still rare in miR-17-92-mediated treatment. In current in vivo research, the miR-17-92 cluster is usually delivered through viral vectors like AAVs; viral vectors have the advantage of excellent delivery efficiency, but they might cause tumorigenesis and immune rejection. To make it safe enough to deliver miR-17-92 into OA joint in human, developing ideal non-viral delivery systems could be a good choice. Moreover, there still exists contradiction concerning the roles of miR-17-92 in OA. One single miRNA could show reverse effect on the OA progression in different literature. For example, Kuo et al. (2019) reported that the inhibition of miR-92a could alleviate the inflammation of OA synovial fibroblasts, but another research discovered that miR-92a was downregulated in TNF-α-treated synoviocytes (Shi et al., 2022). The reason of such phenomena might be the differences in cell lineage and experimental conditions. To better illustrate the underlying mechanisms and current contradictions, more high-quality research studies about this topic are required in the future.
Author contributions
XH, XC, and ZZ: conception and literature search. XP, XH, and XX: manuscript writing and final approval. All authors contributed to the manuscript and approved the submitted version.
Funding
This work was supported by the National Natural Science Foundation of China (81900981), China Postdoctoral Science Foundation (2019M663530), Sichuan Science and Technology Program (2021YJ0149), Research and Development Foundation of West China Hospital of Stomatology (RD-02-202106), and Research Funding from West China School/Hospital of Stomatology Sichuan University (RCDWJS 2020-18).
Conflict of interest
The authors declare that the research was conducted in the absence of any commercial or financial relationships that could be construed as a potential conflict of interest.
Publisher’s note
All claims expressed in this article are solely those of the authors and do not necessarily represent those of their affiliated organizations, or those of the publisher, the editors, and the reviewers. Any product that may be evaluated in this article, or claim that may be made by its manufacturer, is not guaranteed or endorsed by the publisher.
References
Akbar, N., Azzimato, V., Choudhury, R. P., and Aouadi, M. (2019). Extracellular vesicles in metabolic disease. Diabetologia 62 (12), 2179–2187. doi:10.1007/s00125-019-05014-5
Ali Syeda, Z., Langden, S. S. S., Munkhzul, C., Lee, M., and Song, S. J. (2020). Regulatory mechanism of MicroRNA expression in cancer. Int. J. Mol. Sci. 21 (5), E1723. doi:10.3390/ijms21051723
Aparicio, R., Hansen, M., Walker, D. W., and Kumsta, C. (2020). The selective autophagy receptor SQSTM1/p62 improves lifespan and proteostasis in an evolutionarily conserved manner. Autophagy 16 (4), 772–774. doi:10.1080/15548627.2020.1725404
Armiento, A. R., Stoddart, M. J., Alini, M., and Eglin, D. (2018). Biomaterials for articular cartilage tissue engineering: Learning from biology. Acta Biomater. 65, 1–20. doi:10.1016/j.actbio.2017.11.021
Bartel, D. P. (2004). MicroRNAs: Genomics, biogenesis, mechanism, and function. Cell 116 (2), 281–297. doi:10.1016/s0092-8674(04)00045-5
Baumjohann, D. (2018). Diverse functions of miR-17-92 cluster microRNAs in T helper cells. Cancer Lett. 423, 147–152. doi:10.1016/j.canlet.2018.02.035
Bortoluzzi, A., Furini, F., and Scire, C. A. (2018). Osteoarthritis and its management - epidemiology, nutritional aspects and environmental factors. Autoimmun. Rev. 17 (11), 1097–1104. doi:10.1016/j.autrev.2018.06.002
Burr, D. B., and Gallant, M. A. (2012). Bone remodelling in osteoarthritis. Nat. Rev. Rheumatol. 8 (11), 665–673. doi:10.1038/nrrheum.2012.130
Carpio, L. R., and Westendorf, J. J. (2016). Histone deacetylases in cartilage homeostasis and osteoarthritis. Curr. Rheumatol. Rep. 18 (8), 52. doi:10.1007/s11926-016-0602-z
Chakraborty, S., Mehtab, S., Patwardhan, A., and Krishnan, Y. (2012). Pri-miR-17-92a transcript folds into a tertiary structure and autoregulates its processing. RNA 18 (5), 1014–1028. doi:10.1261/rna.031039.111
Chaulk, S. G., Thede, G. L., Kent, O. A., Xu, Z., Gesner, E. M., Veldhoen, R. A., et al. (2011). Role of pri-miRNA tertiary structure in miR-17∼92 miRNA biogenesis. RNA Biol. 8 (6), 1105–1114. doi:10.4161/rna.8.6.17410
Chen, H., Qi, J., Bi, Q., and Zhang, S. (2017). Expression profile of long noncoding RNA (HOTAIR) and its predicted target miR-17-3p in LPS-induced inflammatory injury in human articular chondrocyte C28/I2 cells. Int. J. Clin. Exp. Pathol. 10 (9), 9146–9157.
Chen, J., Huang, Z. P., Seok, H. Y., Ding, J., Kataoka, M., Zhang, Z., et al. (2013). mir-17-92 cluster is required for and sufficient to induce cardiomyocyte proliferation in postnatal and adult hearts. Circ. Res. 112 (12), 1557–1566. doi:10.1161/CIRCRESAHA.112.300658
Chen, N., Wu, D., Li, H., Liu, Y., and Yang, H. (2020). MiR-17-3p inhibits osteoblast differentiation by downregulating Sox6 expression. FEBS open bio 10 (11), 2499–2506. doi:10.1002/2211-5463.12979
Chen, X., Lv, Q., Ma, J., and Liu, Y. (2018). PLCγ2 promotes apoptosis while inhibits proliferation in rat hepatocytes through PKCD/JNK MAPK and PKCD/p38 MAPK signalling. Cell Prolif. 51 (3), e12437. doi:10.1111/cpr.12437
Cherifi, C., Monteagudo, S., and Lories, R. J. (2021). Promising targets for therapy of osteoarthritis: A review on the wnt and TGF-beta signalling pathways. Ther. Adv. Musculoskelet. Dis. 13, 1759720X211006959. doi:10.1177/1759720X211006959
Choi, M. C., Jo, J., Park, J., Kang, H. K., and Park, Y. (2019). NF-κB signaling pathways in osteoarthritic cartilage destruction. Cells 8 (7), E734. doi:10.3390/cells8070734
Cinque, L., Forrester, A., Bartolomeo, R., Svelto, M., Venditti, R., Montefusco, S., et al. (2015). FGF signalling regulates bone growth through autophagy. Nature 528 (7581), 272–275. doi:10.1038/nature16063
Conaghan, P. G., Cook, A. D., Hamilton, J. A., and Tak, P. P. (2019). Therapeutic options for targeting inflammatory osteoarthritis pain. Nat. Rev. Rheumatol. 15 (6), 355–363. doi:10.1038/s41584-019-0221-y
Dikic, I., and Elazar, Z. (2018). Mechanism and medical implications of mammalian autophagy. Nat. Rev. Mol. Cell Biol. 19 (6), 349–364. doi:10.1038/s41580-018-0003-4
Duan, L., Duan, D., Wei, W., Sun, Z., Xu, H., Guo, L., et al. (2019). MiR-19b-3p attenuates IL-1β induced extracellular matrix degradation and inflammatory injury in chondrocytes by targeting GRK6. Mol. Cell. Biochem. 459 (1-2), 205–214. doi:10.1007/s11010-019-03563-2
Duarte, J. H. (2015). Osteoarthritis: Autophagy prevents age-related OA. Nat. Rev. Rheumatol. 11 (12), 683. doi:10.1038/nrrheum.2015.145
Feng, X., Lu, J., Wu, Y., and Xu, H. (2022). MiR-18a-3p improves cartilage matrix remodeling and inhibits inflammation in osteoarthritis by suppressing PDP1. J. Physiol. Sci. 72 (1), 3. doi:10.1186/s12576-022-00827-3
Ferguson, R. J., Palmer, A. J., Taylor, A., Porter, M. L., Malchau, H., and Glyn-Jones, S. (2018). Hip replacement. Lancet 392 (10158), 1662–1671. doi:10.1016/S0140-6736(18)31777-X
Galagali, H., and Kim, J. K. (2020). The multifaceted roles of microRNAs in differentiation. Curr. Opin. Cell Biol. 67, 118–140. doi:10.1016/j.ceb.2020.08.015
Ghafouri-Fard, S., Poulet, C., Malaise, M., Abak, A., Mahmud Hussen, B., Taheriazam, A., et al. (2021). The emerging role of non-coding RNAs in osteoarthritis. Front. Immunol. 12, 773171. doi:10.3389/fimmu.2021.773171
Giannini, D., Antonucci, M., Petrelli, F., Bilia, S., Alunno, A., and Puxeddu, I. (2020). One year in review 2020: Pathogenesis of rheumatoid arthritis. Clin. Exp. Rheumatol. 38 (3), 387–397.
Goldring, M. B., and Marcu, K. B. (2012). Epigenomic and microRNA-mediated regulation in cartilage development, homeostasis, and osteoarthritis. Trends Mol. Med. 18 (2), 109–118. doi:10.1016/j.molmed.2011.11.005
Goldring, S. R., and Goldring, M. B. (2016). Changes in the osteochondral unit during osteoarthritis: Structure, function and cartilage-bone crosstalk. Nat. Rev. Rheumatol. 12 (11), 632–644. doi:10.1038/nrrheum.2016.148
Gruszka, R., and Zakrzewska, M. (2018). The oncogenic relevance of miR-17-92 cluster and its paralogous miR-106b-25 and miR-106a-363 clusters in brain tumors. Int. J. Mol. Sci. 19 (3), E879. doi:10.3390/ijms19030879
Guilak, F., Nims, R. J., Dicks, A., Wu, C. L., and Meulenbelt, I. (2018). Osteoarthritis as a disease of the cartilage pericellular matrix. Matrix Biol. 71-72, 40–50. doi:10.1016/j.matbio.2018.05.008
Harrell, C. R., Jovicic, N., Djonov, V., Arsenijevic, N., and Volarevic, V. (2019). Mesenchymal stem cell-derived exosomes and other extracellular vesicles as new remedies in the therapy of inflammatory diseases. Cells 8 (12), E1605. doi:10.3390/cells8121605
Herman, A. B., Tsitsipatis, D., and Gorospe, M. (2022). Integrated lncRNA function upon genomic and epigenomic regulation. Mol. Cell 82 (12), 2252–2266. doi:10.1016/j.molcel.2022.05.027
Hu, J., Wang, Z., Shan, Y., Pan, Y., Ma, J., and Jia, L. (2018). Long non-coding RNA HOTAIR promotes osteoarthritis progression via miR-17-5p/FUT2/β-catenin axis. Cell Death Dis. 9 (7), 711. doi:10.1038/s41419-018-0746-z
Hu, W., Chen, Y., Dou, C., and Dong, S. (2021). Microenvironment in subchondral bone: Predominant regulator for the treatment of osteoarthritis. Ann. Rheum. Dis. 80 (4), 413–422. doi:10.1136/annrheumdis-2020-218089
Hunter, D. J., and Bierma-Zeinstra, S. (2019). Lancet 393 (10182), 1745–1759. doi:10.1016/S0140-6736(19)30417-9
Jang, S., Lee, K., and Ju, J. H. (2021). Recent updates of diagnosis, pathophysiology, and treatment on osteoarthritis of the knee. Int. J. Mol. Sci. 22 (5), 2619. doi:10.3390/ijms22052619
Jones, I. A., Togashi, R., Wilson, M. L., Heckmann, N., and Vangsness, C. T. (2019). Intra-articular treatment options for knee osteoarthritis. Nat. Rev. Rheumatol. 15 (2), 77–90. doi:10.1038/s41584-018-0123-4
Kapoor, M., Martel-Pelletier, J., Lajeunesse, D., Pelletier, J. P., and Fahmi, H. (2011). Role of proinflammatory cytokines in the pathophysiology of osteoarthritis. Nat. Rev. Rheumatol. 7 (1), 33–42. doi:10.1038/nrrheum.2010.196
Karsdal, M. A., Bay-Jensen, A. C., Lories, R. J., Abramson, S., Spector, T., Pastoureau, P., et al. (2014). The coupling of bone and cartilage turnover in osteoarthritis: Opportunities for bone antiresorptives and anabolics as potential treatments? Ann. Rheum. Dis. 73 (2), 336–348. doi:10.1136/annrheumdis-2013-204111
Katz, J. N., Arant, K. R., and Loeser, R. F. (2021). Diagnosis and treatment of hip and knee osteoarthritis: A review. JAMA 325 (6), 568–578. doi:10.1001/jama.2020.22171
Kayali, S., Giraud, G., Morle, F., and Guyot, B. (2012). Spi-1, Fli-1 and Fli-3 (miR-17-92) oncogenes contribute to a single oncogenic network controlling cell proliferation in friend erythroleukemia. PloS one 7 (10), e46799. doi:10.1371/journal.pone.0046799
Kitada, M., and Koya, D. (2021). Autophagy in metabolic disease and ageing. Nat. Rev. Endocrinol. 17 (11), 647–661. doi:10.1038/s41574-021-00551-9
Klionsky, D. J., Petroni, G., Amaravadi, R. K., Baehrecke, E. H., Ballabio, A., Boya, P., et al. (2021). Autophagy in major human diseases. EMBO J. 40 (19), e108863. doi:10.15252/embj.2021108863
Kong, R., Gao, J., Si, Y., and Zhao, D. (2017). Combination of circulating miR-19b-3p, miR-122-5p and miR-486-5p expressions correlates with risk and disease severity of knee osteoarthritis. Am. J. Transl. Res. 9 (6), 2852–2864.
Kuo, S. J., Liu, S. C., Huang, Y. L., Tsai, C. H., Fong, Y. C., Hsu, H. C., et al. (2019). TGF-β1 enhances FOXO3 expression in human synovial fibroblasts by inhibiting miR-92a through AMPK and p38 pathways. Aging 11 (12), 4075–4089. doi:10.18632/aging.102038
Latourte, A., Kloppenburg, M., and Richette, P. (2020). Emerging pharmaceutical therapies for osteoarthritis. Nat. Rev. Rheumatol. 16 (12), 673–688. doi:10.1038/s41584-020-00518-6
Lee, H., Han, S., Kwon, C. S., and Lee, D. (2016). Biogenesis and regulation of the let-7 miRNAs and their functional implications. Protein Cell 7 (2), 100–113. doi:10.1007/s13238-015-0212-y
Lee, S., Rauch, J., and Kolch, W. (2020). Targeting MAPK signaling in cancer: Mechanisms of drug resistance and sensitivity. Int. J. Mol. Sci. 21 (3), E1102. doi:10.3390/ijms21031102
Lepetsos, P., Papavassiliou, K. A., and Papavassiliou, A. G. (2019). Redox and NF-κB signaling in osteoarthritis. Free Radic. Biol. Med. 132, 90–100. doi:10.1016/j.freeradbiomed.2018.09.025
Levy, J. M. M., Towers, C. G., and Thorburn, A. (2017). Targeting autophagy in cancer. Nat. Rev. Cancer 17 (9), 528–542. doi:10.1038/nrc.2017.53
Li, B., Bai, L., Shen, P., Sun, Y., Chen, Z., and Wen, Y. (2017). Identification of differentially expressed microRNAs in knee anterior cruciate ligament tissues surgically removed from patients with osteoarthritis. Int. J. Mol. Med. 40 (4), 1105–1113. doi:10.3892/ijmm.2017.3086
Li, H., Miao, D., Zhu, Q., Huang, J., Lu, G., and Xu, W. (2018). MicroRNA-17-5p contributes to osteoarthritis progression by binding p62/SQSTM1. Exp. Ther. Med. 15 (2), 1789–1794. doi:10.3892/etm.2017.5622
Li, X. P., Wei, X., Wang, S. Q., Sun, G., Zhao, Y. C., Yin, H., et al. (2021). Differentiation antagonizing non-protein coding RNA knockdown alleviates lipopolysaccharide-induced inflammatory injury and apoptosis in human chondrocyte primary chondrocyte cells through upregulating miRNA-19a-3p. Orthop. Surg. 13 (1), 276–284. doi:10.1111/os.12845
Li Y, Y., Yuan, F., Song, Y., and Guan, X. (2020). miR-17-5p and miR-19b-3p prevent osteoarthritis progression by targeting EZH2. Exp. Ther. Med. 20 (2), 1653–1663. doi:10.3892/etm.2020.8887
Li, Y. S., Zhang, F. J., Zeng, C., Luo, W., Xiao, W. F., Gao, S. G., et al. (2016). Autophagy in osteoarthritis. Jt. bone spine 83 (2), 143–148. doi:10.1016/j.jbspin.2015.06.009
Li, Z., Dai, A., Yang, M., Chen, S., Deng, Z., and Li, L. (2022). p38MAPK signaling pathway in osteoarthritis: Pathological and therapeutic aspects. J. Inflamm. Res. 15, 723–734. doi:10.2147/JIR.S348491
Li Z, Z., Wang, J., and Yang, J. (2020). TUG1 knockdown promoted viability and inhibited apoptosis and cartilage ECM degradation in chondrocytes via the miR-17-5p/FUT1 pathway in osteoarthritis. Exp. Ther. Med. 20 (6), 154. doi:10.3892/etm.2020.9283
Lian, C., Tao, T., Su, P., Liao, Z., Wang, X., Lei, Y., et al. (2020). Targeting miR-18a sensitizes chondrocytes to anticytokine therapy to prevent osteoarthritis progression. Cell Death Dis. 11 (11), 947. doi:10.1038/s41419-020-03155-9
Lietman, C., Wu, B., Lechner, S., Shinar, A., Sehgal, M., Rossomacha, E., et al. (2018). Inhibition of Wnt/β-catenin signaling ameliorates osteoarthritis in a murine model of experimental osteoarthritis. JCI Insight 3 (3), 96308. doi:10.1172/jci.insight.96308
Lin, S., and Gregory, R. I. (2015). MicroRNA biogenesis pathways in cancer. Nat. Rev. Cancer 15 (6), 321–333. doi:10.1038/nrc3932
Liu, B., Li, J., and Cairns, M. J. (2014). Identifying miRNAs, targets and functions. Brief. Bioinform. 15 (1), 1–19. doi:10.1093/bib/bbs075
Lories, R. J., and Luyten, F. P. (2011). The bone-cartilage unit in osteoarthritis. Nat. Rev. Rheumatol. 7 (1), 43–49. doi:10.1038/nrrheum.2010.197
MacFarlane, E. G., Haupt, J., Dietz, H. C., and Shore, E. M. (2017). TGF-Beta family signaling in connective tissue and skeletal diseases. Cold Spring Harb. Perspect. Biol. 9 (11), a022269. doi:10.1101/cshperspect.a022269
Madry, H. (2010). The subchondral bone: A new frontier in articular cartilage repair. Knee Surg. Sports Traumatol. Arthrosc. 18 (4), 417–418. doi:10.1007/s00167-010-1071-y
Makris, E. A., Gomoll, A. H., Malizos, K. N., Hu, J. C., and Athanasiou, K. A. (2015). Repair and tissue engineering techniques for articular cartilage. Nat. Rev. Rheumatol. 11 (1), 21–34. doi:10.1038/nrrheum.2014.157
Mao, G., Wu, P., Zhang, Z., Zhang, Z., Liao, W., Li, Y., et al. (2017a). MicroRNA-92a-3p regulates aggrecanase-1 and aggrecanase-2 expression in chondrogenesis and IL-1β-induced catabolism in human articular chondrocytes. Cell. Physiol. biochem. 44 (1), 38–52. doi:10.1159/000484579
Mao, G., Zhang, Z., Hu, S., Zhang, Z., Chang, Z., Huang, Z., et al. (2018). Exosomes derived from miR-92a-3p-overexpressing human mesenchymal stem cells enhance chondrogenesis and suppress cartilage degradation via targeting WNT5A. Stem Cell Res. Ther. 9 (1), 247. doi:10.1186/s13287-018-1004-0
Mao, G., Zhang, Z., Huang, Z., Chen, W., Huang, G., Meng, F., et al. (2017b). MicroRNA-92a-3p regulates the expression of cartilage-specific genes by directly targeting histone deacetylase 2 in chondrogenesis and degradation. Osteoarthr. Cartil. 25 (4), 521–532. doi:10.1016/j.joca.2016.11.006
Mathiessen, A., and Conaghan, P. G. (2017). Synovitis in osteoarthritis: Current understanding with therapeutic implications. Arthritis Res. Ther. 19 (1), 18. doi:10.1186/s13075-017-1229-9
Mathieu, M., Martin-Jaular, L., Lavieu, G., and Thery, C. (2019). Specificities of secretion and uptake of exosomes and other extracellular vesicles for cell-to-cell communication. Nat. Cell Biol. 21 (1), 9–17. doi:10.1038/s41556-018-0250-9
Mendell, J. T. (2008). miRiad roles for the miR-17-92 cluster in development and disease. Cell 133 (2), 217–222. doi:10.1016/j.cell.2008.04.001
Meng, F., Li, Z., Zhang, Z., Yang, Z., Kang, Y., Zhao, X., et al. (2018). MicroRNA-193b-3p regulates chondrogenesis and chondrocyte metabolism by targeting HDAC3. Theranostics 8 (10), 2862–2883. doi:10.7150/thno.23547
Mizushima, N., and Levine, B. (2020). Autophagy in human diseases. N. Engl. J. Med. 383 (16), 1564–1576. doi:10.1056/NEJMra2022774
Mogilyansky, E., and Rigoutsos, I. (2013). The miR-17/92 cluster: A comprehensive update on its genomics, genetics, functions and increasingly important and numerous roles in health and disease. Cell Death Differ. 20 (12), 1603–1614. doi:10.1038/cdd.2013.125
Murphy, B. L., Obad, S., Bihannic, L., Ayrault, O., Zindy, F., Kauppinen, S., et al. (2013). Silencing of the miR-17∼92 cluster family inhibits medulloblastoma progression. Cancer Res. 73 (23), 7068–7078. doi:10.1158/0008-5472.CAN-13-0927
Nagelkerke, A., Ojansivu, M., van der Koog, L., Whittaker, T. E., Cunnane, E. M., Silva, A. M., et al. (2021). Extracellular vesicles for tissue repair and regeneration: Evidence, challenges and opportunities. Adv. Drug Deliv. Rev. 175, 113775. doi:10.1016/j.addr.2021.04.013
O'Donnell, K. A., Wentzel, E. A., Zeller, K. I., Dang, C. V., and Mendell, J. T. (2005). c-Myc-regulated microRNAs modulate E2F1 expression. Nature 435 (7043), 839–843. doi:10.1038/nature03677
Olive, V., Jiang, I., and He, L. (2010). mir-17-92, a cluster of miRNAs in the midst of the cancer network. Int. J. Biochem. Cell Biol. 42 (8), 1348–1354. doi:10.1016/j.biocel.2010.03.004
Olivotto, E., Otero, M., Marcu, K. B., and Goldring, M. B. (2015). Pathophysiology of osteoarthritis: Canonical NF-κB/IKKβ-dependent and kinase-independent effects of IKKα in cartilage degradation and chondrocyte differentiation. RMD Open 1 (1), e000061. doi:10.1136/rmdopen-2015-000061
Osada, H., and Takahashi, T. (2011). let-7 and miR-17-92: small-sized major players in lung cancer development. Cancer Sci. 102 (1), 9–17. doi:10.1111/j.1349-7006.2010.01707.x
Ostojic, J., Yoon, Y. S., Sonntag, T., Nguyen, B., Vaughan, J. M., Shokhirev, M., et al. (2021). Transcriptional co-activator regulates melanocyte differentiation and oncogenesis by integrating cAMP and MAPK/ERK pathways. Cell Rep. 35 (7), 109136. doi:10.1016/j.celrep.2021.109136
Peat, G., and Thomas, M. J. (2021). Osteoarthritis year in review 2020: Epidemiology & therapy. Osteoarthr. Cartil. 29 (2), 180–189. doi:10.1016/j.joca.2020.10.007
Peng, B., Theng, P. Y., and Le, M. T. N. (2021). Essential functions of miR-125b in cancer. Cell Prolif. 54 (2), e12913. doi:10.1111/cpr.12913
Petrocca, F., Vecchione, A., and Croce, C. M. (2008). Emerging role of miR-106b-25/miR-17-92 clusters in the control of transforming growth factor beta signaling. Cancer Res. 68 (20), 8191–8194. doi:10.1158/0008-5472.CAN-08-1768
Price, A. J., Alvand, A., Troelsen, A., Katz, J. N., Hooper, G., Gray, A., et al. (2018). Knee replacement. Lancet 392 (10158), 1672–1682. doi:10.1016/S0140-6736(18)32344-4
Raghu, H., Lepus, C. M., Wang, Q., Wong, H. H., Lingampalli, N., Oliviero, F., et al. (2017). CCL2/CCR2, but not CCL5/CCR5, mediates monocyte recruitment, inflammation and cartilage destruction in osteoarthritis. Ann. Rheum. Dis. 76 (5), 914–922. doi:10.1136/annrheumdis-2016-210426
Reinhart, B. J., Slack, F. J., Basson, M., Pasquinelli, A. E., Bettinger, J. C., Rougvie, A. E., et al. (2000). The 21-nucleotide let-7 RNA regulates developmental timing in Caenorhabditis elegans. Nature 403 (6772), 901–906. doi:10.1038/35002607
Robinson, W. H., Lepus, C. M., Wang, Q., Raghu, H., Mao, R., Lindstrom, T. M., et al. (2016). Low-grade inflammation as a key mediator of the pathogenesis of osteoarthritis. Nat. Rev. Rheumatol. 12 (10), 580–592. doi:10.1038/nrrheum.2016.136
Romanov, J., Walczak, M., Ibiricu, I., Schuchner, S., Ogris, E., Kraft, C., et al. (2012). Mechanism and functions of membrane binding by the Atg5-Atg12/Atg16 complex during autophagosome formation. EMBO J. 31 (22), 4304–4317. doi:10.1038/emboj.2012.278
Saliminejad, K., Khorram Khorshid, H. R., Soleymani Fard, S., and Ghaffari, S. H. (2019). An overview of microRNAs: Biology, functions, therapeutics, and analysis methods. J. Cell. Physiol. 234 (5), 5451–5465. doi:10.1002/jcp.27486
Sanchez-Lopez, E., Coras, R., Torres, A., Lane, N. E., and Guma, M. (2022). Synovial inflammation in osteoarthritis progression. Nat. Rev. Rheumatol. 18 (5), 258–275. doi:10.1038/s41584-022-00749-9
Schulte, J. H., Horn, S., Otto, T., Samans, B., Heukamp, L. C., Eilers, U. C., et al. (2008). MYCN regulates oncogenic MicroRNAs in neuroblastoma. Int. J. Cancer 122 (3), 699–704. doi:10.1002/ijc.23153
Scrivo, A., Bourdenx, M., Pampliega, O., and Cuervo, A. M. (2018). Selective autophagy as a potential therapeutic target for neurodegenerative disorders. Lancet. Neurol. 17 (9), 802–815. doi:10.1016/S1474-4422(18)30238-2
Sellam, J., and Berenbaum, F. (2010). The role of synovitis in pathophysiology and clinical symptoms of osteoarthritis. Nat. Rev. Rheumatol. 6 (11), 625–635. doi:10.1038/nrrheum.2010.159
Shang, X., Boker, K. O., Taheri, S., Hawellek, T., Lehmann, W., and Schilling, A. F. (2021). The interaction between microRNAs and the wnt/β-catenin signaling pathway in osteoarthritis. Int. J. Mol. Sci. 22 (18), 9887. doi:10.3390/ijms22189887
Sharma, L. (2021). Osteoarthritis of the knee. N. Engl. J. Med. 384 (1), 51–59. doi:10.1056/NEJMcp1903768
Sheng, Y., Song, Y., Li, Z., Wang, Y., Lin, H., Cheng, H., et al. (2018). RAB37 interacts directly with ATG5 and promotes autophagosome formation via regulating ATG5-12-16 complex assembly. Cell Death Differ. 25 (5), 918–934. doi:10.1038/s41418-017-0023-1
Shi, Z., He, J., He, J., and Xu, Y. (2022). Micro-fragmented adipose tissue regulated the biological functions of osteoarthritis synoviocytes by upregulating MiR-92a-3p expression. Tissue Cell 74, 101716. doi:10.1016/j.tice.2021.101716
Sinkala, M., Nkhoma, P., Mulder, N., and Martin, D. P. (2021). Integrated molecular characterisation of the MAPK pathways in human cancers reveals pharmacologically vulnerable mutations and gene dependencies. Commun. Biol. 4 (1), 9. doi:10.1038/s42003-020-01552-6
Stoll, S., Wang, C., and Qiu, H. (2018). DNA methylation and histone modification in hypertension. Int. J. Mol. Sci. 19 (4), E1174. doi:10.3390/ijms19041174
Sun, H., Hu, S., Zhang, Z., Lun, J., Liao, W., and Zhang, Z. (2019). Expression of exosomal microRNAs during chondrogenic differentiation of human bone mesenchymal stem cells. J. Cell. Biochem. 120 (1), 171–181. doi:10.1002/jcb.27289
Sun J, J. L., Yan, J. F., Yu, S. B., Zhao, J., Lin, Q. Q., and Jiao, K. (2020). MicroRNA-29b promotes subchondral bone loss in TMJ osteoarthritis. J. Dent. Res. 99 (13), 1469–1477. doi:10.1177/0022034520937617
Sun X, X., Zhang, R., Chen, H., Du, X., Chen, S., Huang, J., et al. (2020). Fgfr3 mutation disrupts chondrogenesis and bone ossification in zebrafish model mimicking CATSHL syndrome partially via enhanced Wnt/β-catenin signaling. Theranostics 10 (16), 7111–7130. doi:10.7150/thno.45286
Taniguchi, K., and Karin, M. (2018). NF-κB, inflammation, immunity and cancer: Coming of age. Nat. Rev. Immunol. 18 (5), 309–324. doi:10.1038/nri.2017.142
Thielen, N. G. M., van der Kraan, P. M., and van Caam, A. P. M. (2019). TGFβ/BMP signaling pathway in cartilage homeostasis. Cells 8 (9), E969. doi:10.3390/cells8090969
Thomson, D. W., and Dinger, M. E. (2016). Endogenous microRNA sponges: Evidence and controversy. Nat. Rev. Genet. 17 (5), 272–283. doi:10.1038/nrg.2016.20
Thornton, J. E., and Gregory, R. I. (2012). How does Lin28 let-7 control development and disease? Trends Cell Biol. 22 (9), 474–482. doi:10.1016/j.tcb.2012.06.001
van der Kraan, P. M. (2017). The changing role of TGFβ in healthy, ageing and osteoarthritic joints. Nat. Rev. Rheumatol. 13 (3), 155–163. doi:10.1038/nrrheum.2016.219
van Niel, G., D'Angelo, G., and Raposo, G. (2018). Shedding light on the cell biology of extracellular vesicles. Nat. Rev. Mol. Cell Biol. 19 (4), 213–228. doi:10.1038/nrm.2017.125
Ventayol, M., Vinas, J. L., Sola, A., Jung, M., Brune, B., Pi, F., et al. (2014). miRNA let-7e targeting MMP9 is involved in adipose-derived stem cell differentiation toward epithelia. Cell Death Dis. 5, e1048. doi:10.1038/cddis.2014.2
Walker, S., Busatto, S., Pham, A., Tian, M., Suh, A., Carson, K., et al. (2019). Extracellular vesicle-based drug delivery systems for cancer treatment. Theranostics 9 (26), 8001–8017. doi:10.7150/thno.37097
Wang, B. W., Jiang, Y., Yao, Z. L., Chen, P. S., Yu, B., and Wang, S. N. (2019). Aucubin protects chondrocytes against IL-1β-induced apoptosis in vitro and inhibits osteoarthritis in mice model. Drug Des. devel. Ther. 13, 3529–3538. doi:10.2147/DDDT.S210220
Wang C, C., Yue, H., Huang, W., Lin, X., Xie, X., He, Z., et al. (2020). Cryogenic 3D printing of heterogeneous scaffolds with gradient mechanical strengths and spatial delivery of osteogenic peptide/TGF-β1 for osteochondral tissue regeneration. Biofabrication 12 (2), 025030. doi:10.1088/1758-5090/ab7ab5
Wang G, G., Chen, S., Xie, Z., Shen, S., Xu, W., Chen, W., et al. (2020). TGFβ attenuates cartilage extracellular matrix degradation via enhancing FBXO6-mediated MMP14 ubiquitination. Ann. Rheum. Dis. 79 (8), 1111–1120. doi:10.1136/annrheumdis-2019-216911
Wang, Y., Tan, J., Wang, L., Pei, G., Cheng, H., Zhang, Q., et al. (2021). MiR-125 family in cardiovascular and cerebrovascular diseases. Front. Cell Dev. Biol. 9, 799049. doi:10.3389/fcell.2021.799049
Woods, K., Thomson, J. M., and Hammond, S. M. (2007). Direct regulation of an oncogenic micro-RNA cluster by E2F transcription factors. J. Biol. Chem. 282 (4), 2130–2134. doi:10.1074/jbc.C600252200
Xia, H., Green, D. R., and Zou, W. (2021). Autophagy in tumour immunity and therapy. Nat. Rev. Cancer 21 (5), 281–297. doi:10.1038/s41568-021-00344-2
Xie, Y., Kang, R., Sun, X., Zhong, M., Huang, J., Klionsky, D. J., et al. (2015). Posttranslational modification of autophagy-related proteins in macroautophagy. Autophagy 11 (1), 28–45. doi:10.4161/15548627.2014.984267
Xu, R., Wei, Y., Yin, X., Shi, B., and Li, J. (2019). miR-20a suppresses chondrogenic differentiation of ATDC5 cells by regulating Atg7. Sci. Rep. 9 (1), 9243. doi:10.1038/s41598-019-45502-7
Yang, J., Zhang, Y. S., Yue, K., and Khademhosseini, A. (2017). Cell-laden hydrogels for osteochondral and cartilage tissue engineering. Acta Biomater. 57, 1–25. doi:10.1016/j.actbio.2017.01.036
Zhang B, B., Tian, X., Qu, Z., Hao, J., and Zhang, W. (2022). Hypoxia-preconditioned extracellular vesicles from mesenchymal stem cells improve cartilage repair in osteoarthritis. Membr. (Basel) 12 (2), 225. doi:10.3390/membranes12020225
Zhang H, H., Ge, J., and Lu, X. (2021). CircFADS2 is downregulated in osteoarthritis and suppresses LPS-induced apoptosis of chondrocytes by regulating miR-195-5p methylation. Arch. Gerontol. Geriatr. 96, 104477. doi:10.1016/j.archger.2021.104477
Zhang Y, Y., Li, S., Jin, P., Shang, T., Sun, R., Lu, L., et al. (2022). Dual functions of microRNA-17 in maintaining cartilage homeostasis and protection against osteoarthritis. Nat. Commun. 13 (1), 2447. doi:10.1038/s41467-022-30119-8
Zhang Y, Y., Sun, Z., Jia, J., Du, T., Zhang, N., Tang, Y., et al. (2021). Overview of histone modification. Adv. Exp. Med. Biol. 1283, 1–16. doi:10.1007/978-981-15-8104-5_1
Zhang, Z., Kang, Y., Zhang, Z., Zhang, H., Duan, X., Liu, J., et al. (2012). Expression of microRNAs during chondrogenesis of human adipose-derived stem cells. Osteoarthr. Cartil. 20 (12), 1638–1646. doi:10.1016/j.joca.2012.08.024
Zhao, H., and Gong, N. (2019). miR-20a regulates inflammatory in osteoarthritis by targeting the IκBβ and regulates NK-κB signaling pathway activation. Biochem. Biophys. Res. Commun. 518 (4), 632–637. doi:10.1016/j.bbrc.2019.08.109
Zhen, G., and Cao, X. (2014). Targeting TGFβ signaling in subchondral bone and articular cartilage homeostasis. Trends Pharmacol. Sci. 35 (5), 227–236. doi:10.1016/j.tips.2014.03.005
Keywords: osteoarthritis, miRNA, chondrocyte apoptosis, extracellular matrix degradation, bone remodeling, synovitis
Citation: Pan X, Cen X, Xiong X, Zhao Z and Huang X (2022) miR-17-92 cluster in osteoarthritis: Regulatory roles and clinical utility. Front. Genet. 13:982008. doi: 10.3389/fgene.2022.982008
Received: 06 July 2022; Accepted: 15 November 2022;
Published: 29 November 2022.
Edited by:
Sandeep Kumar, Emory University, United StatesReviewed by:
Jiao Kai, Fourth Military Medical University, ChinaKittisak Buddhachat, Naresuan University, Thailand
Copyright © 2022 Pan, Cen, Xiong, Zhao and Huang. This is an open-access article distributed under the terms of the Creative Commons Attribution License (CC BY). The use, distribution or reproduction in other forums is permitted, provided the original author(s) and the copyright owner(s) are credited and that the original publication in this journal is cited, in accordance with accepted academic practice. No use, distribution or reproduction is permitted which does not comply with these terms.
*Correspondence: Zhihe Zhao, emh6aGFvQHNjdS5lZHUuY24=; Xinqi Huang, eHFodWFuZ0BzY3UuZWR1LmNu