- Department of Ophthalmology, The Second Affiliated Hospital of Harbin Medical University, Harbin, China
Neovascularization is one of the many manifestations of ocular diseases, including corneal injury and vascular diseases of the retina and choroid. Although anti-VEGF drugs have been used to effectively treat neovascularization, long-term use of anti-angiogenic factors can cause a variety of neurological and developmental side effects. As a result, better drugs to treat ocular neovascularization are urgently required. There is mounting evidence that epigenetic regulation is important in ocular neovascularization. DNA methylation and histone modification, non-coding RNA, and mRNA modification are all examples of epigenetic mechanisms. In order to shed new light on epigenetic therapeutics in ocular neovascularization, this review focuses on recent advances in the epigenetic control of ocular neovascularization as well as discusses these new mechanisms.
1 Introduction
Angiogenesis (the formation of new blood vessels) is a physiological and pathological condition required for embryologic development, wound closure, degenerating tissues, and abnormal vascular proliferation. Corneal infection or injury, retinopathy of prematurity (ROP), proliferative diabetic retinopathy (PDR), and age-related macular degeneration (AMD) all-cause corneal neovascularization, retinal neovascularization (RNV), and choroidal neovascularization (CNV), respectively (Figure 1). Vision loss can occur suddenly and irreversibly in many disorders due to ocular neovascularization, or aberrant new blood vessels. Surgery, laser photocoagulation, hormone therapy, and anti-vascular endothelial growth factor (VEGF) medications are currently the main therapeutic options for DR. These therapies, meanwhile, have the potential to be harmful to one’s health or even life and can only temporarily relieve a condition’s symptoms rather than curing it entirely. As a result, it is critical for vision loss control and pharmacological treatment to investigate the molecular mechanisms of neovascularization.
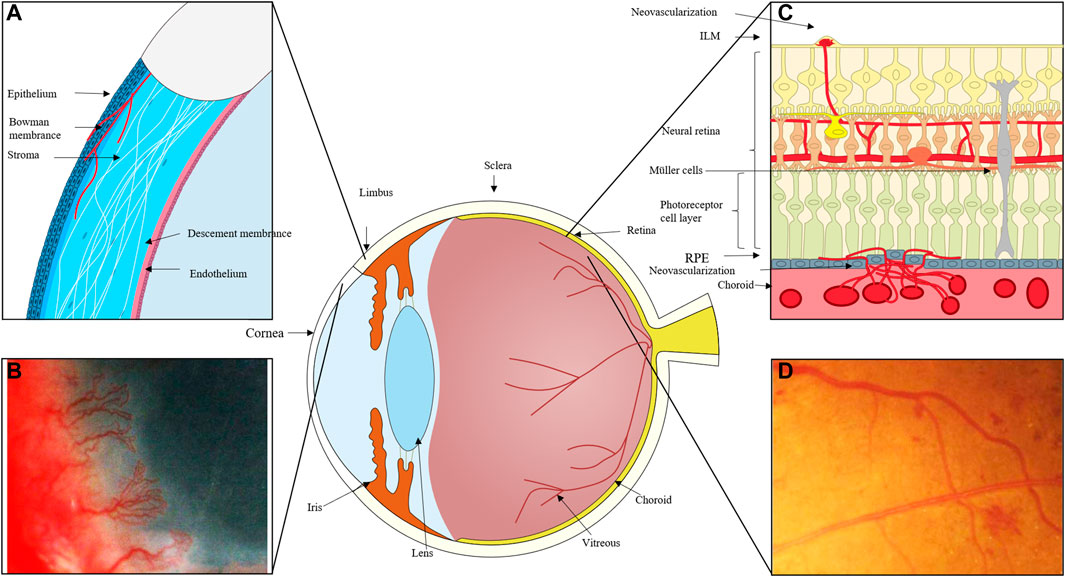
FIGURE 1. (A) The cornea can become damaged by inflammation, trauma, and other factors, and the process of corneal repair is accompanied by the development of neovascularization. New blood vessels emerge from the corneal limbal vessels, and the corneal endothelium proliferates and migrates, growing into the corneal stroma to create corneal neovascularization, which ultimately affects vision. (B) Massive corneal revascularisation, affecting the patient’s vision. (C) In cases of retinal inflammation, ischemia, and damage to retinal homeostasis, retinal microvascular endothelial cells migrate to the damaged area and, with the help of pro-angiogenic cells like Müller and microglia, secrete pro-angiogenic factors, eventually forming new neovascular cavities. In extreme cases, these neovascular cavities can even break through the inner boundary membrane and grow into the vitreous cavity. When the choroid is exposed to stimulating circumstances like hypoxia and ischemia, choroidal microvessels expand in the direction of these locations, occasionally piercing the RPE layer. (D) Fundus photography of neovascularisation in the eye.
2 Angiogenesis and neovascularization
Angiogenesis is one of the side effects of vascular injury. When the vasculature is exposed to negative factors, a variety of cells, including endothelial cells, neo-angiogenic immune cells, endothelial progenitor cells (EPCs), and others, become involved in angiogenesis. The newly developed and unstable vascular types are primarily twofold. On the one hand, endothelial cell proliferation, migration, and capillary tube development can attract circulating endothelial progenitor cells to a damaged region (Potente, Gerhardt, and Carmeliet 2011). Finally, the pericytes cover the endothelial cells, forming a solid vascular system. Similar processes of pathogenic neovascularization exist. Corneal neovascularization primarily arises from the corneal limbal vessels and grows into the layers of the cornea; retinal neovascularization frequently arises from the retinal vessels, breaks through the inner limiting membrane (ILM), and grows into the vitreous; neovascularization of the choroid occurs as a result of the formation of new blood vessels; often, choroidal neovascular membranes (CNVs) form when blood vessels from the choroid penetrate the retinal pigment epithelium and proliferate (Campochiaro, 2000; Nicholas and Mysore 2021). This paper focuses on the impact of dysregulation of epigenetic molecular mechanisms in endothelial cells on neovascularization because it is currently generally accepted that the mechanism of ocular neovascular disease is primarily related to the abnormal biological activity of ocular vascular endothelial cells.
3 Epigenetic modifications of ocular neovascularization
An epigenetic modification is a heritable transcriptional change that is not induced by DNA sequence changes (Felsenfeld 2014). Non-coding RNAs like miRNA, LncRNA, CircRNA, and transcription factors have been discovered to have a role in epigenetic regulation of gene transcription and protein modifications in recent years (Tough et al., 2016). Furthermore, as more and more RNA modifications are discovered and recognized, epigenetic regulation is becoming increasingly complex (Frye et al., 2018; Wiener and Schwartz 2021). Epigenetic alterations appear to play a key role in the regulation of ocular neovascularization, according to a growing body of research (Xu M et al., 2018; Lu et al., 2021). DNA methylation, covalent histone modification, noncoding RNA (ncRNA) regulation, and transcription factor regulatory network are the four major epigenetic mechanisms (Tough et al., 2016). DNA methylation (5-methylcytosine, 5mC) is one of the most common types. Histone alterations, such as acetylation and methylation, are important epigenetic factors. Through acetylation, methylation, phosphorylation, and ubiquitylation, the N-terminus of histones is now recognized as a common posttranslational alteration that controls chromatin status and, in turn, leads to aberrant gene regulation (Bates 2020). Studies have found that histone H2AX phosphorylation is essential for retinal neovascularization (Economopoulou et al., 2009). Non-coding RNAs (ncRNAs), including as miRNAs, lncRNAs, and circRNAs, regulate gene expression in a number of ways. A increasing body of evidence suggests that ncRNAs influence gene expression via histone modifications or a combination of histone modification and methylation (Slack and Chinnaiyan 2019).
DNA methylation, histone changes, including acetylation and methylation, and ncRNAs have all been linked to the development of neovascularization. The formation of lymphatic and endothelial arteries is connected to DNA methylation, histone 3 lysine 4 methylation (H3K4me), and histone 3 lysine 27 (H3K27me) (Tacconi et al., 2021). Furthermore, neovascularization is aided by the N6-methyladenosine (m6A) modification (Shan et al., 2020). The creation of a complex interaction network between non-coding RNAs and proteins, with a focus on common signaling pathways including VEGF, HIF, Notch, Wnt, and PI3K/AKT. We describe current advances in our understanding of angiogenesis regulatory mechanisms, including as DNA methylation, histone modifications, and the influence of noncoding RNAs on histone or DNA modifications, in this study. The function of epigenetic regulation in the creation of corneal, retinal, and choroidal neovascular membranes could lead to a new therapy option for neovascularization patients (Figure 2).
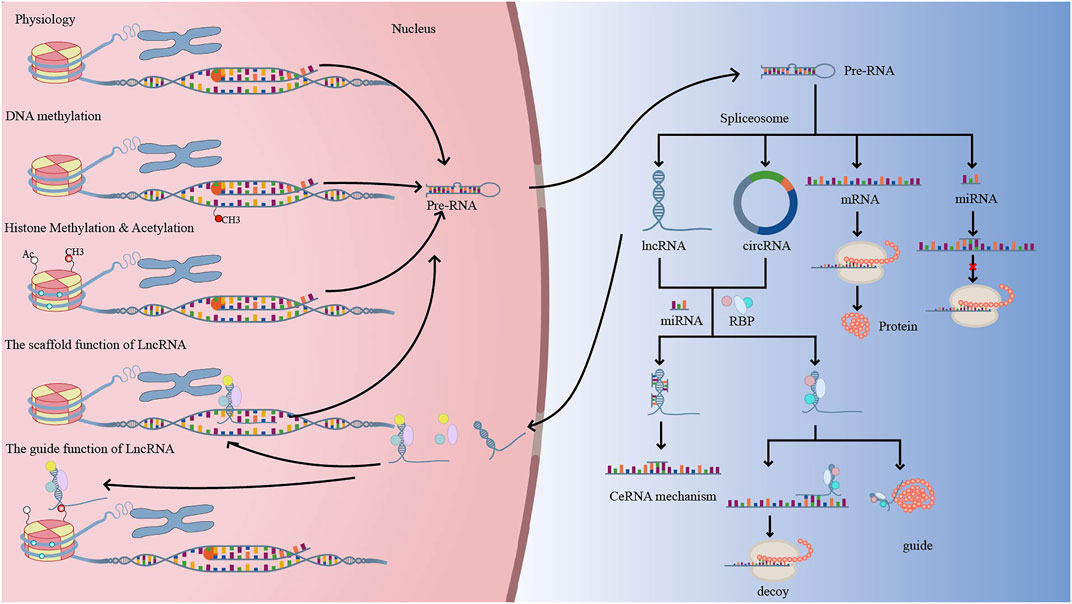
FIGURE 2. Under physiological conditions, DNA is translated into precursor RNA (pre-RNA), which exits the nucleus and is spliced into protein-coding RNA (mRNA) and non-coding RNA (miRNA, LncRNA, CircRNA, etc.). Normally, when DNA is methylated, pre-RNA transcription decreases, which in turn regulates genes related to neovascularization. LncRNA or CircRNA have similar mechanisms of action, with LncRNA acting as signal, decoy, guide, and scaffold to regulate gene transcription, translation, and post-translational modifications. Methylation and acetylation of histones can alter chromatin accessibility and facilitate transcriptional complexes into promoter regions, regulating gene transcription.
3.1 DNA methylation in neovascularization
The covalent binding of a methyl group to the 5-position of cytosine in DNA happens frequently in sections bigger than 200bp with a high ratio of G + C-rich regions, resulting in the synthesis of 5-methylcytosine (5mC) (Hellebrekers, Griffioen, and van Engeland 2007). DNA methylation, which is performed by DNA methyltransferases (DNMTs), regulates chromatin structure and gene expression by transferring a methyl group from S-adenosylmethionine (SAM) to the fifth carbon of cytosine in CpG patterns (Hellebrekers, Griffioen, and van Engeland 2007; Shafabakhsh et al., 2019). DNMT1, DNMT3a and DNMT3b, and DNMT3L are the four main enzymes that mediate DNA methylation (Shafabakhsh et al., 2019). Distinct methyltransferases serve different purposes. DNMT1 is involved in DNA methylation maintenance, DNMT3A and DNMT3B are required for de novo methyltransferases, and DNMT3L interacts with DNMT3A and DNMT3B and dramatically increases their catalytic activity (Yuan et al., 2014). MBD, a DNA methylation binding protein, is principally engaged in DNMT’s execution of DNA methylation functions. MBD1, MBD2, MBD3 and MBD4 are the four methyl-CpG binding domain proteins (Banerjee and Bacanamwo 2010). MBD2, a methyl-CpG-binding protein that regulates gene expression and angiogenesis in vascular endothelium, is one of them. MBD2 regulates angiogenesis in a DNA methylation-dependent mechanism in response to tissue ischemia (Cooper and Keaney 2011). Furthermore, MBD2 can be coupled with a methylation area found in the promoters of endothelial nitric oxide synthase (eNOS) and vascular endothelial growth factor (VEGF) receptor 2 (VEGFR2) in vascular endothelial cells, reducing angiogenesis expression (Rao et al., 2011). Adenosine kinase (ADK) can also influence the methylation state of the VEGFR2 promoter region and promote neovascularization (Xu et al., 2017). In human umbilical artery DNMT3A has been shown to interact with E2F Transcription Factor 1 (E2F1) and control neovascularization-related genes such as the VEGF family, EPhinB2, and HEY2. However, the interaction of DNMT3A with E2F1 that modulates the gene’s transcriptional repression may be independent of the methyltransferase activity (Su et al., 2020).
The alteration of ocular neovascularization was caused by a DNA methylation mechanism (Table1). In an animal model of corneal alkali burn, corneal neovascularization is inhibited by a DNA demethylation alteration involving the PI3K/Akt/mTOR signaling pathways (Li J et al., 2021). The diabetic retinal vasculature is affected by abnormal DNA methylation modifications (Miao et al., 2020).The hypermethylated genes were mostly found in the PI3K/AKT signaling pathway and the extracellular matrix expression protein-related pathway, both of which are beneficial for neovascularization during PDR (Miao et al., 2020). Furthermore, in a hyperglycemic environment, IL-6 can enhance gene expression related with angiogenesis by lowering the protein levels of DNMT1 and DNMT3b (Balakrishnan et al., 2018). On the one hand, hypoxia increased HIF-1 binding site (HBS) methylation, decreased HIF-1 DNA binding, promoted erythropoietin expression, and induced revascularization growth (Wenger et al., 1998); on the other hand, hypoxia can induce HBS demethylation of the VEGF gene promoter, increase HIF-1 on the binding properties of VEGF gene promoters, promote hypoxic induced VEGF gene transactive and VEGF accumulation (Pisani et al., 2018). In neovascular AMD, or early AMD, DNA methylation has shifted (Oliver et al., 2015; Shen et al., 2020). Hypoxia boosted the synthesis of interleukin 17 receptor C (IL-17RC) mRNA in hypoxia-induced retinal pigment epithelium (RPE) cells by boosting the demethylation of the IL-17RC promoter; promoting choroidal neovascularization by a synergistic action of IL-17RC and VEGF (Alivand, Sabouni, and Soheili 2016).
3.2 Histone methylation regulates neovascularization
A DNA and protein polymer made primarily of histone proteins, chromatin is the physiological form of the eukaryotic genome. Chromatin remodeling and gene transcription are significantly influenced by histones and their post-translational modifications (Venkatesh and Workman 2015). The accessibility of chromatin may be directly impacted by histone modifications that change the chemistry of the residues that make up their changed forms. By altering the accessibility of RNA polymerase and transcription factors to genes, and notably promoters, chromatin compactness controls transcription at the level of the chromatin (Levy et al., 2017).Histone methyltransferases and demethylases regulate histone methylation. Specific histone tail methylation is frequently linked to transcriptional activation or repression (Choi et al., 2020). Trimethylation or dimethylation of H3K4, H3K36 and H3K79 is generally associated with gene activation, but histone H3 K9 methylation is generally associated with repression of gene expression (Chen et al., 2020). In association with polycomb complex, H3K27me3 acts as a repression histone mark (Agger et al., 2007).Histones can undergo demethylation as well as methylation, for example, H3K27me3 can be removed by the demethylase ubiquitously transcribed tetratricopeptide repeat X (UTX) (Agger et al., 2007). JHDM2A, JHDM1, Lid2, and the lysine demethylase (KDM) family are examples of proteins with the JmjC domain (Tsukada et al., 2006; Yamane et al., 2006; Li et al., 2008; Wang et al., 2017). Furthermore, non-dependent JMIC domain proteins, such as LSD1, can engage in the de-methylating modification of histones (Culhane and Cole 2007). These methyltransferase/demethylated enzymes, as well as other epigenetic genetic regulators, can play a role in neovascularization control (Table 2).
A number of histone changes are involved in the process of neovascularization. SETD8 is an H4K20me mono-methyltransferase. SETD8 was shown to have a role in angiogenesis in human umbilical vein endothelial cells (HUVEC) in part through influencing HES-1; in the meantime, SETD8 inhibitors suppressed abnormal neovascularization in the mouse oxygen induced retinopathy (OIR) model (Choi et al., 2020). Enhancer of zeste homolog 2 (EZH2), a major catalytic component of polycomb repressive complex 2 (PRC2), regulates the process of neovascularization by catalyzing the trimethylation of histone H3K27 (Ohtani et al., 2011). The expression of ROS-dependent activation of the Forkhead-box protein O3a (FoxO3a) pathway and P13K/AKT signaling is reduced when EZH2 is inhibited, lowering corneal neovascularization (Wan et al., 2020). PHD finger protein 8 (PHF8), a histone demethylase, may regulate HIF1 and H3K4me3 signaling to govern neovascularization. PHF8 upregulates HIF-1 and activates histone demethylase KDM3A in anoxic settings, which maintains H3K4me3 levels and upregulates HIF1 downstream target genes (Maina et al., 2017). VEGF stimulates angiogenesis by increasing the expression of EZH2 in endothelial cells (Lu et al., 2010). Furthermore, VEGF inhibited the production of EZH2 by downregulating miR-101 expression and therefore promoting angiogenesis (Smits et al., 2011). JARID1B occupies and decreases histone 3 lysine 4 methylation levels on the HOXA5 promoter, then inhibits HOXA5 production to retain angiogenic capacity (Fork et al., 2015). MLL is a histone H3 lysine 4 (H3K4) methyl transferase (HMT) that may methylate H3K4 at HOX9 promoters, increasing neovascularization via influencing endothelial cells sprout and migration (Diehl et al., 2007). Furthermore, LSD1-mediated demethylation of HIF-1a under hypoxic circumstances leads to HIF-1a stability, which promotes the establishment of neovascularization (Kim et al., 2016). In DR, LSD1 has a role in the neovascularization of endothelial cells, which is triggered by high blood glucose levels (Zhao et al., 2020). Increased expression of Jagged1 by histone lysine-specific demethylase 2A (KDM2A) might enhance neovascularization (Chen et al., 2016). JHDM1D (also known as KDM7A) is a histone demethylation enzyme that may change histone methylation levels and induce neovascularization by controlling the expression of VEGF-A (Osawa et al., 2011).
3.3 Histone acetylation in neovascularization
Histone acetyltransferase (HAT) and histone deacetylase (HDAC) regulate histone acetylation in a dynamic manner (Aikawa et al., 2006; Park and Kim 2020). Histone acetylation happens mostly on its histone tail, which can activate or repress gene transcription. When histone lysine is acetylated, the nucleosome’s histone structure changes from compact to loose, which increases RNA polymerase accessibility and hence increases gene expression (Park and Kim 2020). Histone deacetylation causes transcriptional repression by decreasing the DNA-histone link (Buysschaert et al., 2008). Histone acetylation is mostly related with the GNAT, MYST, and p300/CBP families (Xia et al., 2020). Histone deacetylation is primarily mediated by two protein families: the histone deacetylase family (HDAC1-11) and the Sirtuin protein family (SIRT1-7) (Portela and Esteller 2010; Park and Kim 2020). Dynamic modifications of histone acetylation have been shown to play an important role in neovascularization, with HDAC6, HDAC7, and HDAC9 being positive regulators of angiogenesis and enhancing endothelial cell angiogenesis, whereas HDAC5 inhibits endothelial cell sprouting by suppressing the expression of angiogenic guidance factors (Kaluza et al., 2013).
Histone acetyltransferase P300 dramatically increases MRTF-A transcriptional activity on VE-cadherin genes by increasing acetylation of H3K9, H3K14, and H4, hence promoting vascular development and angiogenesis (Shu et al., 2015). This is similar to previous studies in that neither SRF nor MRTF-A have intrinsic histone acetylase activity, but they can promote chromatin remodeling directly or indirectly through ribosomal reorganization; histone recruitment to p300/CBP can be promoted during chromatin remodeling, increasing the expression of angiogenesis-related genes (Hanna et al., 2009).The VEGF promoter region is efficiently upregulated by H3K9 acetylation, triggering VEGF-induced angiogenesis (Fu et al., 2014). Under high glucose conditions, histone acetyltransferases CBP/p300 and p/CAF acetylate histone H3 (K9, K14) and H4 (K8, K8, K12) and increase NF-κB expression, whereas NF-κB and HAT recruitment synergistically regulate chromatin remodeling and promote the expression of inflammation and angiogenesis-related genes (Miao et al., 2004; Aurora et al., 2010). Furthermore, in early DR, a high-glucose environment decreases the amount of Sirt6 in Müller cells while increasing acetylation of H3K56, resulting in reduced levels of neuroprotective factor (BDNF) protein and greater levels of VEGF protein, encouraging early neurodegenerative lesions and vascular dysfunction (Zorrilla-Zubilete et al., 2018). It was recently discovered that high levels of glycolysis in macrophages/microglia produce a lot of acetyl coenzyme a, which leads to histone acetylation and pathological retinal angiogenesis-associated glycolytic macrophages/microglia (PRAGM)-associated gene induction, which reprograms macrophages/microglia to an angiogenic phenotype and promotes retinal pathological angiogenesis (Liu C et al., 2020). HDAC controls the expression of HoxA9, which affects the expression of endothelial characteristic genes including endothelial nitric oxide synthase, VEGFR2 and VE-cadherin, promoting the differentiation of adult histocytes to endothelial cells and engaging in neovascularization. HoxA9 expression is decreased when HDAC inhibitors are employed, which decreases neovascularization (Kaluza et al., 2013).HDAC enhances the production of pro-angiogenic genes VEGF, β-FGF, and TGFβ1 in a corneal alkali burn model, promoting angiogenesis, whereas inhibitors limit neovascularization (Zhou et al., 2014a; Zhou et al., 2014b). The HDAC inhibitor AN7 inhibits CNV progression by antagonizing the deacetylation activity of class I and II HDAC, resulting in hyperacetylation of histones H3 and H4, as well as downregulating the production of pro-angiogenic factors VEGF and fibroblast growth factor (FGF-2) (Dahbash et al., 2019). These findings imply that histone acetylation regulation is involved in angiogenesis and that histone deacetylase inhibitors are employed to treat ocular neovascularization, although the molecular mechanisms of ocular neovascularization remain unknown.
3.4 Epigenetic modifications of non-coding RNAs and ocular neovascularization
These results in light of earlier research on the diversity of non-coding RNAs led to the classification of ncRNAs into two main groups: structural non-coding RNAs and regulatory non-coding RNAs.The rRNA and tRNA subgroups of structural non-coding RNAs.Regulatory non-coding RNAs are further divided into three classes, small, medium and long non-coding RNAs (Nagano and Fraser 2011). Small ncRNAs include miRNAs, tsRNAs, and piRNAs, but big ncRNAs are mostly subclasses like LncRNAs, CircRNAs, and pseudogenes. Furthermore, many more are categorized as medium non-coding RNA with a size between 50–200 nucleotides, including snoRNA, prompts, tiRNA, snRNA, and many more. (Dahariya et al., 2019; Slack and Chinnaiyan 2019). This research focuses on the interactions between these three RNAs and epigenetics as miRNA, LncRNA, and CircRNA are more extensively explored in ocular neovascularization.
3.4.1 Epigenetic modification of MiRNA and ocular neovascularization
MicroRNAs are a kind of small ncRNA with a length of around 22 nucleotides that are mostly generated through RNA pol II transcription (Slack and Chinnaiyan 2019; Liu Z et al., 2020). MiRNA regulates the expression of genes involved in angiogenesis by binding to the 3′UTR region in mRNA through its own 5′ end (Liu X et al., 2020). The emphasis of this paper is on the epigenetic control of miRNAs that are related with ocular neovascularization (Table 3).
3.4.1.1 MiR-126
MiR-126 governs the formation of different retinal vascular layers as well as ocular neovascularization (Zhao et al., 2015; Martinez and Peplow 2021). MiR-126-3p promotes immature capillary development by decreasing Sprouty-related protein1 (SPRED1) and phosphoinositol-3 kinase regulatory subunit 2 (PLK2) expression, boosting ERK phosphorylation, and modulating the mobility of endothelial cell (EC)-based membrane-like complex structures and stabilizing networks (Pitzler et al., 2016). Furthermore, MiR-126 promotes the differentiation of pluripotent stem cells into retinal ganglion stem cells and the release of VEGF, which in turn regulates retinal neovascularization (Ye et al., 2018). MiR-126 epigenetic regulation is also implicated in the process of neovascularization. The miR-126 promoter’s methylation is important in the control of neovascularization (Zhang et al., 2013; Xue et al., 2020). High hyperglycemia triggers human umbilical vein endothelial cell (HUVEC) endogenous H2S-DMNT1-miR-126 regulatory axis, causing endothelial cell migratory abnormalities (Xue et al., 2020). Histone methyltransferase (MMSET) can construct chromatin modification complexes by binding to the miR-126 promoter, KAP1 corepressor, and histone deacetylase, resulting in increased H3K9 trimethylation and reduced H3 acetylation and reducing miR-126 production (Min et al., 2013). According to the findings, epigenetic regulation involving miR-126 has the potential to be a molecular target for the therapy of ocular neovascularization in the future.
3.4.1.2 MiR-150
MiR-150 has been established in studies to perform a significant regulatory function in pathological neovascularization of the retina and choroid (Shen et al., 2008; Liu et al., 2016; Chen et al., 2019). MiR-150 targets stearoyl coenzyme A desaturase-2 in human peripheral blood mononuclear cells to coordinate macrophage-mediated inflammation and pathological angiogenesis in AMD in a VEGF-independent way (Lin et al., 2018). MiR-150 suppresses endothelial cell proliferation, migration, and tube formation while also specifically reducing the expression of different angiogenic regulators such as CXCR4, DLL4, and FZD4 in endothelial cells, hence limiting pathogenic ocular neovascularization (Liu et al., 2015). Mixed lineage leukemia gene (MLL) histone methylation transferase, which negatively controls miR-150 by preventing miR-150 precursors from being processed into mature miRNAs via the MYC/LIN28 functional axis (Jiang et al., 2012). Furthermore, it was discovered that the DNMT1 enzyme methylates CpG islands in the miR-150-5p gene promoter (Hu et al., 2019). These findings imply that miR-150 epigenetic control may play a role in the process of ocular neovascularization.
3.4.1.3 MiR-29
MIR-29b-3p has been demonstrated to adversely influence the production of VEGF A and platelet-derived growth factor B (PDGF B), as well as the proliferation and angiogenesis of retinal microvascular endothelial cells (Tang et al., 2020). Furthermore, in laser-induced choroidal neovascularization, activation of NF-κB suppressed MiR-29 expression and increased choroidal neovascularization development (Cai et al., 2014). In human cell lines, miR-29 regulates the gene expression of DNMT1, DNMT3a, Dnmt3b, SIRT1, SIRT3, and ROS, as well as overall DNA methylation and protein acetylation levels (Morita et al., 2013; Sonkar, Kay, and Choudhury 2019). MiR-29 reduce the abundance of H4K20me3 and the degree of DNA methylation, decreasing DNA damage repair and the expression of genomic signatures (Heid et al., 2017; Lyu et al., 2018). Furthermore, DNA methylation and histone changes can regulate miR-29 family control and hence neovascularization. HDAC4 silencing causes hyperacetylation of the miR-29b promoter, resulting in increased miR-29b expression (Amodio et al., 2016). These findings imply that molecular pathways involved in epigenetic interactions might cause abnormal miR-29 dysregulation and mediate angiogenesis.
3.4.1.4 MiR-200
MiR-200b/c protects human retinal microvascular endothelial cell (hRMECs) from high-glucose-induced dysfunction by decreasing vasohibin-2 (VASH2) and decreases endothelial cell migration and proliferation (Ding et al., 2017). Furthermore, hypoxia decreases miR-200b expression and increases Ets-1 expression in endothelial cells, promoting angiogenesis (Chan et al., 2011). The transcriptional regulation of microRNA-200 family members is regulated by DNMT1/DNMT-3A and histone methyltransferase (EZH2) (Ning et al., 2015). High glucose levels reduced the expression of the endothelial cell DNA methylation transferases DNMT1 and DNMT3A, resulting in miR-200b promoter hypermethylation and regulating neovascularization (Singh et al., 2017). Furthermore, high glucose stimulation inhibits miR-200b production by activating PRC2-mediated histone methylation, which lowers VEGF expression and increases retinal inflammation and neovascularization (Ruiz, Feng, and Chakrabarti 2015). MiR-200b is also a part of a complex regulatory network that includes LncRNAs and epigenetically linked proteins. Yes-associated protein (YAP1), which regulates the MALAT1/miR-200b-3p/VEGFA axis, may contribute to retinal neovascularization in DR lesions (Han et al., 2020).
3.4.1.5 MiR-21
MiR-21 is a critical regulator of ocular neovascularization (Guduric-Fuchs et al., 2012; Merrigan and Kennedy 2017; Vivanco-Rojas et al., 2020). By modulating the expression of its target protein Maspin, miR-21-5p affects the PI3K/AKT and ERK signaling pathways, and hence diabetic retinal neovascularization (Qiu et al., 2018). MiR-21 regulates and targets PPARa, promoting OIR retinal neovascularization and inflammatory responses (Chen et al., 2017). Chronic hypoxia and high glucose levels can cause abnormal methylation of the miR-21-5p promoter, which in turn controls eNOS production and causes endothelial dysfunction (Penaloza, Soto-Carrasco, and Krause 2020). Under hypoxic circumstances, intracellular Akt2 can control miR-21 production and hence neovascularization via NF-κB, CREB, and CBP/p300 binding to the miR-21 promoter and regional acetylation of histone H3K9 (Polytarchou et al., 2011).
3.4.1.6 Others
In mice, miR-24 suppresses laser-induced choroidal neovascularization (Zhou et al., 2014a). The study discovered that miR-24 is engaged in epigenetic control; UTX on chromosome X dramatically decreases methylation levels in the miR-24 promoter via histone H3K27 demethylase (KDM6) (Ni et al., 2019). However, it has to be shown if this epigenetic control is present in ocular neovascularization. MiR-34a also influences retinal angiogenesis via the Notch1/VEGF signaling pathway (Shi S et al., 2019). Furthermore, via modulating Sirt1 in hyperglycemia-stimulated microvascular endothelium, miR-34a might be implicated in neovascularization in diabetic vasculopathy (Arunachalam et al., 2016). These miRNAs play important physiological roles in neovascular regulation, and their involvement in the formation of complex networks of epigenetic regulation is becoming more apparent; understanding the mechanisms of formation of these abnormal miRNAs is critical for preventing and controlling neovascularization.
3.4.2 Epigenetic modifications of LncRNA and ocular neovascularization
LncRNA has a wide range of biological roles, including regulating pathological neovascularization of the cornea, retina, and choroidal disorders (Huang et al., 2015; Zhang C et al., 2020). Depending on their subcellular location, lncRNAs have diverse regulation mechanisms, according to studies. By binding to RNA-binding proteins, LncRNAs in the nucleus impact histone modifications, DNA methylation status, and maturation of certain precursor mRNAs, which ultimately govern gene transcription and protein production by regulatory transcription or post-transcriptional regulation (Xu Z et al., 2018). LncRNAs in the cytoplasm frequently pass through sponge target miRNAs and control intracellular signaling via competing endogenous RNAs (CeRNA) regulation mechanisms (Xu M et al., 2018; Ghafouri-Fard et al., 2020). The control of neovascularization relies heavily on these regulatory systems. The epigenetic regulatory mechanisms implicated in LncRNAs related with ocular neovascularization in recent years are the emphasis of the following paragraphs (Table 4).
3.4.2.1 NEAT1
In corneal, retinal, and choroidal neovascularization, long noncoding RNA nuclear enriched abundant transcript 1 (NEAT1) has been widely researched. By sponging with miR-377 and boosting the production of VEGFA, SIRT1, and BCL-XL, NEAT1 controls neovascularization (Zhou Y et al., 2019).Furthermore, miR-194-5p has been demonstrated to increase cell invasion and migration by inhibiting methylation of the NEAT1 promoter region by targeting the 3′ UTR of DNMT3A (Duan et al., 2020).
3.4.2.2 H19
In vascularized corneas, H19 is abundantly expressed. By targeting vascular endothelial growth factor-A, lncRNA H19 can increase ocular neovascularization (Sun et al., 2019). In addition, LncRNA H19 may have a role in angiogenesis epigenetic regulation. H19 can bind to EZH2, a histone methyltransferase. H19 improves EZH2’s ability to recruit methyl groups to the promoter region of the angiogenesis inhibitor gene vasopressor-1 (VASH1), inhibiting VASH1 expression and promoting angiogenesis (Yuan et al., 2019); H19 also promotes HIF-1 histone H3K4me3 methylation and increases HIF-1 expression by recruiting EZH2, speeding up fibroblast activation and angiogenesis regulation (Guo et al., 2018). H19 regulates endothelial cell dysfunction by raising histone acetylation upstream of miR-200 and controlling miR-200 production; it also regulates retinal neovascularization by acting on miR-200 in a non-dependent manner (Thomas et al., 2019).
3.4.2.3 HOTAIR
By influencing epigenetic processes such as histone methylation, acetylation, DNA methylation, and transcription factors, HOX transcript antisense intergenic RNA (HOTAIR) facilitates diabetic retinopathy development (Biswas et al., 2021). HOTAIR controls the status of chromatin and gene transcription by functioning as a scaffold for directed transcription and controlling histone methylation changes, among other things (Tsai et al., 2010). HOTAIR inhibits VE-calmodulin transcription while enhancing VEGFA transcription by binding to histone lysine specific demethylase 1 (LSD1) (Zhao et al., 2020). Furthermore, HOTAIR promotes angiogenesis by activating VEGFA transcription directly and upregulating VEGFA and Ang2 expression through glucose regulated protein 78 (Fu et al., 2016). Histone alterations also have a role in the regulation of lncRNA HOTAIR. Under hypoxic circumstances, the HOTAIR promoter is regulated by histone methyltransferase MLL1, histone acetylase p300, and HIF-1α, which are all abundant at the HOTAIR promoter (Bhan et al., 2017). Furthermore, JMJD6, a protein that has both lysyl hydroxylase and arginine demethylase capabilities, binds to the HOTAIR promoter region and stimulates HOTAIR expression through its lysyl hydroxylase/demethylase activity (Biswas A et al., 2018). According to these findings, HOTAIR may be involved in the genetic regulation of related gene expression in neovascularization.
3.4.2.4 MALAT1
Metastasis associated lung adenocarcinoma transcript 1 (MALAT1) is one of the most significant epigenetic regulators in diabetic retinopathy, and it regulates endothelial cell function and angiogenesis (Biswas S et al., 2018). MALAT1 promoter CpG island methylation is affected by high hyperglycemia, which affects endothelial cell function (Biswas A et al., 2018). MALAT1 regulates HIF-1 expression and interacts with nuclear transcription factors to induce neovascularization (Jayasuriya et al., 2020). MALAT1 is abundantly expressed in OIR mice’s retinas, and it promotes RNV development primarily via regulating the CCN1-Akt-VEGFA pathway (Wang et al., 2020). MALAT1 increases angiogenesis in ischemia-reperfusion damage via modulating the 15-lipoxygenase 1/STAT3 signaling pathway (Wang C et al., 2019). MALAT1 blocks the Wnt signaling pathway by suppressing CTNNB1 transcription and expression, which attracts methyltransferases to boost CTNNB1 promoter methylation, primarily in the CTNNB1 promoter region (Li et al., 2019). Histone modifications also have a role in MALAT1 expression. By binding to the promoter of the MALAT1 gene, resulting in the demethylation of histone H3K9 in the promoter region of the MALAT1 gene, the histone demethylase JMJD1A can boost MALAT1 expression, which stimulates cell proliferation and migration (Tee et al., 2014). In addition, JMJD2C protein entering the nucleus improved the -linked protein signaling pathway in colorectal cancer cells by lowering H3K9me3 and H3K36me3 histone methylation levels in the MALAT1 promoter region and upregulating MALAT1 expression (Wu et al., 2019). YAP1 may enhance neovascularization in diabetic retinopathy via the MALAT 1/MiR-200b-3p/VEGFA regulation axis in high-glucose-induced endothelial cells and high-fat diet-induced diabetic mice retina (Han et al., 2020). SIRT6 binds to the MALA T1 promoter region and reduces MALAT1 expression, allowing neovascularization to occur (Qin et al., 2019). However, the researchers did not investigate the impact of SIRT6’s deacetylase activity on MALAT1.
3.4.2.5 MEG3
There are several LncRNAs that inhibit neovascularization, in addition to the potential that the aforementioned LncRNAs promote neovascularization. Maternally expressed gene 3 (MEG3) reduces angiogenesis in vivo and in vitro by negatively influencing the notch system; lncRNA-Meg3 knockout mice had increased vascular endothelial growth factor pathway gene expression and microvessel density (Kumar and Goyal, 2017; Liu et al., 2017). Furthermore, it has been discovered that DNMT1 increases MEG3 promoter methylation by recruiting methyltransferases to inhibit MEG3 expression (He Y 2021 Mar 1). MEG3 inhibits histone methylation by interacting with EZH2 and lowering EZH2 and H3K27me3 recruitment to EN2 (Zhou R et al., 2020). TTR interacts with Poly(A)-binding protein cytoplasm 1 (PABPC1), which suppresses hREC proliferation and angiogenesis, to enhance lncRNA-MEG3 expression (Fan et al., 2019). These research discovered that MEG3-targeting epigenetic regulatory systems might be one of the treatment options for ocular neovascularization.
3.4.2.6 SNHG1 and SNHG7
In the DR, the lncRNA small nucleolar RNA host gene 1 (SNHG1) is abnormally expressed (Yang et al., 2021). SNHG1 enhances vascular endothelial cell proliferation and angiogenesis in TNF-stimulated HUVEC (Zhang et al., 2020a).SNHG1-mediated histone changes are also implicated in neovascularization. SNHG1 interacts directly with the polycomb repressor complex 2 (PRC2), which regulates cell growth and differentiation by regulating histone methylation of the Kruppel-like factor 2 (KLF2) promoter and cell cycle protein-dependent kinase inhibitor 2B (CDKN2B) in the nucleus (Xu Z et al., 2018; Li, Guo, and Wu 2020). By decreasing miR-543 and increasing Sirt1 expression, SNHG7 prevents high glucose-induced angiogenesis in human retinal endothelial cells (Ke et al., 2019). SNHG7 stimulates the Wnt/-catenin signaling pathway by inhibiting DKK1 production, which primarily controls H3K27 methylation levels by creating a complex with EZH2 in the DKK1 promoter region (Chi et al., 2020). These findings show that epigenetic control of SNHG7 or SNHG1 may play a role in neovascularization.
3.4.2.7 Others
Fendrr, a long noncoding RNA, enhances high glucose-induced proliferation and angiogenesis in human retinal endothelial cells, perhaps through regulating VEGF (Shi Y et al., 2019). Meanwhile, through the miRNA-214-3p/TET2 regulatory axis, LncRNA Fendrr may be involved in the modification of RASSF1 promoter methylation; however, TET2 is an ancient DNA demethylase, suggesting that LncRNA Fendrr indirectly mediates the involvement of DNA demethylases in neovascularization via the CeRNA mechanism (He Z 2018 Oct 15). Through changes in histone methylation, the lncRNA GATA6-AS interacts with the epigenetic regulator LOXL2 to modulate endothelial gene expression for angiogenesis (Neumann et al., 2018). These findings show that, whereas LncRNA-mediated DNA methylation, histone changes, and other modifications in ocular neovascularization have yet to be extensively reported, their precise mechanisms of action may be revealed in the near future.
3.5 CircRNA and ocular neovascularization
Circular RNA (circRNA) has been shown to be differentially expressed in corneal, retinal, and choroidal neovascularization, suggesting that it may regulate neovascularization by regulating intracellular signaling molecules such as extracellular matrix production, MAPK cascade signaling, and renin-angiotensin system pathways (Cao et al., 2019; Zhou Z et al., 2019; Zhang et al., 2020b; Liu C et al., 2020). CircRNA, like LncRNA, promotes angiogenesis mostly through the mechanism of sponge miRNA and regulates miRNA target genes. CircFndc3bk in endothelial-derived exosomes may play a crucial role in PDR neovascularization (Li X et al., 2021). CZBTB44 stimulates choroidal angiogenesis by functioning as a miR-578 sponge, decreasing miR-578 activity and increasing production of vascular endothelial growth factor A (VEGFA) and vascular cell adhesion molecule-1 (VCAM1) (Zhou Y et al., 2020). CIRCCRNA-0006896 promotes HUVEC migration and proliferation via the regulatory axis of microRNA-1264/DNMT1 (Wen et al., 2021). More regulatory mechanisms influencing epigenetic inheritance will be revealed in the future as CircRNAs are extensively investigated in ocular diseases.
3.6 M6A modifications are involved in ocular neovascularization
A rising number of studies have shown that RNA modifications, such as N6-methyladenosine (m6A) and 5-methylcytidine (m5C), play a significant role in epigenetic regulation illnesses, resulting in full base isomerization and aberrant alterations in RNA activity (Wiener and Schwartz 2021). M6A methylation changes are mediated by writer, eraser, and reader proteins, and these RNA modifying proteins (RMP) play critical roles in RNA stability and translation activity (Frye et al., 2018). Currently, m6A alterations are particularly prevalent in ocular neovascularization. The M6A writing protein METTL3 has been demonstrated to have a pro-angiogenic function in retinal and corneal neovascularization, with the major mechanism being to increase mRNA translation of LRP6 and DVL1 in a YTHDF1-dependent way (Yao et al., 2020). METTL3 knockdown hindered cell proliferation, migration, and capillary formation in HUVEC, with the major mechanism depending on its m6A writing enzyme activity to raise PHLPP2 levels and decrease mTOR phosphorylation, resulting in vascular defects and reduced AKT activation (Parial et al., 2021). Furthermore, FTO, a demethylase involved in m6A modification, has increased expression in sick corneal endothelial cells; increased FTO promotes low m6A methylation levels of the pro-angiogenic gene FAK, resulting in improved FAK stability and neovascularization (Shan et al., 2020). KAT1 can rely on histone acetylase activity to trigger YTHDF2 transcription, which can reduce ITGB1 mRNA stability via M6A modification and limit microvessel density and angiogenesis (Qi et al., 2021). Additionally, m6A mutations have a function in non-coding RNA regulation processes. METTL3, a m6A methyltransferase, enhances angiogenesis by boosting splicing of the precursor miR-143-3p, increasing miR-143-3p expression, and inhibiting VASH1 expression (Wang H et al., 2019). These findings imply that the RNA modification pathways involved in m6A in ocular neovascularization will be gradually elucidated in the near future.
4 Concluding remarks
The mechanisms of epigenetic changes such as DNA methylation, histone modifications, and non-coding RNA regulation in ocular neovascularization remain unknown. More in-depth study, however, will eventually reveal complex epigenetic regulatory processes in ocular neovascularization. The present regulatory network linking DNA methylation and RNA modifications of histones, noncoding RNAs, and m6A in ocular neovascularization, including epigenetics, is the subject of this study.
Author contributions
QH, XZ, BJ, and ZYZ contributed to the conception and design of the study. MHS is mainly responsible for drawing. BJ did compilation of the study. QH wrote the first draft of the manuscript and XZ edited it under the supervision of DS. All authors contributed to the article and approved the submitted version.
Funding
This work was funded by the National Natural Science Foundation of China (No.82171103).
Conflict of Interest
The authors declare that the research was conducted in the absence of any commercial or financial relationships that could be construed as a potential conflict of interest.
Publisher’s Note
All claims expressed in this article are solely those of the authors and do not necessarily represent those of their affiliated organizations, or those of the publisher, the editors and the reviewers. Any product that may be evaluated in this article, or claim that may be made by its manufacturer, is not guaranteed or endorsed by the publisher.
References
Agger, K., Cloos, P. A., Christensen, J., Pasini, D., Rose, S., Rappsilber, J., et al. (2007). 'UTX and JMJD3 are histone H3K27 demethylases involved in HOX gene regulation and development. Nature 449, 731–734. doi:10.1038/nature06145
Aikawa, Y., Nguyen, L. A., Isono, K., Takakura, N., Tagata, Y., Schmitz, M. L., et al. (2006). 'Roles of HIPK1 and HIPK2 in AML1- and p300-dependent transcription, hematopoiesis and blood vessel formation. EMBO J. 25, 3955–3965. doi:10.1038/sj.emboj.7601273
Alivand, M. R., Sabouni, F., and Soheili, Z. S. (2016). Probable chemical hypoxia effects on progress of CNV through induction of promoter CpG demethylation and overexpression of IL17RC in human RPE cells. Curr. Eye Res. 41, 1245–1254. doi:10.3109/02713683.2015.1095933
Amodio, N., Stamato, M. A., Gulla, A. M., Morelli, E., Romeo, E., Raimondi, L., et al. (2016). 'Therapeutic targeting of miR-29b/HDAC4 epigenetic loop in multiple myeloma. Mol. Cancer Ther. 15, 1364–1375. doi:10.1158/1535-7163.MCT-15-0985
Arunachalam, G., Lakshmanan, A. P., Samuel, S. M., Triggle, C. R., and Ding, H. (2016). Molecular interplay between microRNA-34a and Sirtuin1 in hyperglycemia-mediated impaired angiogenesis in endothelial cells: Effects of metformin. J. Pharmacol. Exp. Ther. 356, 314–323. doi:10.1124/jpet.115.226894
Aurora, A. B., Biyashev, D., Mirochnik, Y., Zaichuk, T. A., Sanchez-Martinez, C., Renault, M. A., et al. (2010). 'NF-kappaB balances vascular regression and angiogenesis via chromatin remodeling and NFAT displacement. Blood 116, 475–484. doi:10.1182/blood-2009-07-232132
Balakrishnan, A., Guruprasad, K. P., Satyamoorthy, K., and Manjunath, B. (2018). 'Interleukin-6 determines protein stabilization of DNA methyltransferases and alters DNA promoter methylation of genes associated with insulin signaling and angiogenesis. Lab. Invest. 98, 1143–1158. doi:10.1038/s41374-018-0079-7
Banerjee, S., and Bacanamwo, M. (2010). DNA methyltransferase inhibition induces mouse embryonic stem cell differentiation into endothelial cells. Exp. Cell Res. 316, 172–180. doi:10.1016/j.yexcr.2009.08.011
Bates, S. E. (2020). 'Epigenetic therapies for cancer. N. Engl. J. Med. 383, 650–663. doi:10.1056/NEJMra1805035
Bhan, A., Deb, P., Shihabeddin, N., Ansari, K. I., Brotto, M., and Mandal, S. S. (2017). 'Histone methylase MLL1 coordinates with HIF and regulate lncRNA HOTAIR expression under hypoxia. Gene 629, 16–28. doi:10.1016/j.gene.2017.07.069
Biswas, A., Shettar, A., Mukherjee, G., Kondaiah, P., and Desai, K. V. (2018). 'JMJD6 induces HOTAIR, an oncogenic lincRNA, by physically interacting with its proximal promoter. Biochem. J. 475, 355–371. doi:10.1042/BCJ20170664
Biswas, S., Feng, B., Chen, S., Liu, J., Aref-Eshghi, E., Gonder, J., et al. (2021). 'The long non-coding RNA HOTAIR is a critical epigenetic mediator of angiogenesis in diabetic retinopathy. Invest. Ophthalmol. Vis. Sci. 62, 20. doi:10.1167/iovs.62.3.20
Biswas, S., Thomas, A. A., Chen, S., Aref-Eshghi, E., Feng, B., Gonder, J., et al. (2018). 'MALAT1: An epigenetic regulator of inflammation in diabetic retinopathy. Sci. Rep. 8, 6526. doi:10.1038/s41598-018-24907-w
Buysschaert, I., Schmidt, T., Roncal, C., Carmeliet, P., and Lambrechts, D. (2008). 'Genetics, epigenetics and pharmaco-(epi)genomics in angiogenesis. J. Cell. Mol. Med. 12, 2533–2551. doi:10.1111/j.1582-4934.2008.00515.x
Cai, J., Yin, G., Lin, B., Wang, X., Liu, X., Chen, X., et al. (2014). Roles of NFκB-miR-29s-MMP-2 circuitry in experimental choroidal neovascularization. J. Neuroinflammation 11, 88. doi:10.1186/1742-2094-11-88
Campochiaro, P. A. (2000). Retinal and choroidal neovascularization. J. Cell. Physiol. 184, 301–310. doi:10.1002/1097-4652(200009)184:3<301::aid-jcp3>3.0.co;2-h
Cao, M., Zhang, L., Wang, J. H., Zeng, H., Peng, Y., Zou, J., et al. (2019). 'Identifying circRNA-associated-ceRNA networks in retinal neovascularization in mice. Int. J. Med. Sci. 16, 1356–1365. doi:10.7150/ijms.35149
Chan, Y. C., Khanna, S., Roy, S., and Sen, C. K. (2011). miR-200b targets Ets-1 and is down-regulated by hypoxia to induce angiogenic response of endothelial cells. J. Biol. Chem. 286, 2047–2056. doi:10.1074/jbc.M110.158790
Chen, Q. J., Cai, Q., Wang, M., Liu, J., Yang, J., Zhou, C., et al. (2018). Long Noncoding RNA NEAT1, Regulated by the EGFR Pathway, Contributes to Glioblastoma Progression Through the WNT/beta-Catenin Pathway by Scaffolding EZH2. Clin. Cancer Res. 24, 684–695.
Chen, J., Liang, X., Zhang, S., Wang, S., Garcia, S. P., Yan, P., et al. (2020). 'Two faces of bivalent domain regulate VEGFA responsiveness and angiogenesis. Cell Death Dis. 11, 75. doi:10.1038/s41419-020-2228-3
Chen, J. Y., Li, C. F., Chu, P. Y., Lai, Y. S., Chen, C. H., Jiang, S. S., et al. (2016). 'Lysine demethylase 2A promotes stemness and angiogenesis of breast cancer by upregulating Jagged1. Oncotarget 7, 27689–27710. doi:10.18632/oncotarget.8381
Chen, Q., Qiu, F., Zhou, K., Matlock, H. G., Takahashi, Y., Rajala, R. V. S., et al. (2017). Pathogenic role of microRNA-21 in diabetic retinopathy through downregulation of PPARα. Diabetes 66, 1671–1682. doi:10.2337/db16-1246
Chen, S., Yuan, M., Liu, Y., Zhao, X., Lian, P., Chen, Y., et al. (2019). 'Landscape of microRNA in the aqueous humour of proliferative diabetic retinopathy as assessed by next-generation sequencing. Clin. Exp. Ophthalmol. 47, 925–936. doi:10.1111/ceo.13554
Chi, C., Li, M., Hou, W., Chen, Y., Zhang, Y., and Chen, J. (2020). Long noncoding RNA SNHG7 activates wnt/β-catenin signaling pathway in cervical cancer cells by epigenetically silencing DKK1. Cancer biother. Radiopharm. 35, 329–337. doi:10.1089/cbr.2019.3004
Choi, D. K., Kim, Y. K., Park, S. W., Lee, H., Lee, S., Kim, S. A., et al. (2020). The histone lysine methyltransferase SETD8 regulates angiogenesis through HES-1 in human umbilical vein endothelial cells. Sci. Rep. 10, 12089. doi:10.1038/s41598-020-69103-x
Cooper, M. P., and Keaney, J. F. (2011). Epigenetic control of angiogenesis via DNA methylation. Circulation 123, 2916–2918. doi:10.1161/CIRCULATIONAHA.111.033092
Culhane, J. C., and Cole, P. A. (2007). 'LSD1 and the chemistry of histone demethylation. Curr. Opin. Chem. Biol. 11, 561–568. doi:10.1016/j.cbpa.2007.07.014
Dahariya, S., Paddibhatla, I., Kumar, S., Raghuwanshi, S., Pallepati, A., and Gutti, R. K. (2019). 'Long non-coding RNA: Classification, biogenesis and functions in blood cells. Mol. Immunol. 112, 82–92. doi:10.1016/j.molimm.2019.04.011
Dahbash, M., Sella, R., Megiddo-Barnir, E., Nisgav, Y., Tarasenko, N., Weinberger, D., et al. (2019). 'The histone deacetylase inhibitor AN7, attenuates choroidal neovascularization in a mouse model. Int. J. Mol. Sci. 20, E714. doi:10.3390/ijms20030714
Diehl, F., Rossig, L., Zeiher, A. M., Dimmeler, S., and Urbich, C. (2007). The histone methyltransferase MLL is an upstream regulator of endothelial-cell sprout formation. Blood 109, 1472–1478. doi:10.1182/blood-2006-08-039651
Ding, Y., Hu, Z., Luan, J., Lv, X., Yuan, D., Xie, P., et al. (2017). Protective effect of miR-200b/c by inhibiting vasohibin-2 in human retinal microvascular endothelial cells. Life Sci. 191, 245–252. doi:10.1016/j.lfs.2017.09.001
Dong, W. H., Zhang, C., Zhao, Y., Luo, , , and Chen, Y. (2021). Silencing of miR-150-5p ameliorates diabetic nephropathy by targeting SIRT1/p53/AMPK pathway. Front. Physiol. 12, 624989.
Duan, M. Y., Li, M., Tian, H., Tang, G., Yang, Y. C., and Peng, N. C. (2020). 'Down-regulation of lncRNA NEAT1 regulated by miR-194-5p/DNMT3A facilitates acute myeloid leukemia. Blood Cells Mol. Dis. 82, 102417. doi:10.1016/j.bcmd.2020.102417
Economopoulou, M., Langer, H. F., Celeste, A., Orlova, V. V., Choi, E. Y., Ma, M., et al. (2009). 'Histone H2AX is integral to hypoxia-driven neovascularization. Nat. Med. 15, 553–558. doi:10.1038/nm.1947
Fan, G., Gu, Y., Zhang, J., Xin, Y., Shao, J., Giampieri, F., et al. (2019). 'Transthyretin upregulates long non-coding RNA MEG3 by affecting PABPC1 in diabetic retinopathy. Int. J. Mol. Sci. 20, E6313. doi:10.3390/ijms20246313
Felsenfeld, G. (2014). 'A brief history of epigenetics. Cold Spring Harb. Perspect. Biol. 6, a018200. doi:10.1101/cshperspect.a018200
Fork, C., Gu, L., Hitzel, J., Josipovic, I., Hu, J., SzeKa Wong, M., et al. (2015). Epigenetic regulation of angiogenesis by JARID1B-induced repression of HOXA5. Arterioscler. Thromb. Vasc. Biol. 35, 1645–1652. doi:10.1161/ATVBAHA.115.305561
Frye, M., Harada, B. T., Behm, M., and He, C. (2018). RNA modifications modulate gene expression during development. Science 361, 1346–1349. doi:10.1126/science.aau1646
Fu, S. P., He, S. Y., Xu, B., Hu, C. J., Lu, S. F., Shen, W. X., et al. (2014). 'Acupuncture promotes angiogenesis after myocardial ischemia through H3K9 acetylation regulation at VEGF gene. PLoS One 9, e94604. doi:10.1371/journal.pone.0094604
Fu, W. M., Lu, Y. F., Hu, B. G., Liang, W. C., Zhu, X., Yang, H. D., et al. (2016). 'Long noncoding RNA Hotair mediated angiogenesis in nasopharyngeal carcinoma by direct and indirect signaling pathways. Oncotarget 7, 4712–4723. doi:10.18632/oncotarget.6731
Ghafouri-Fard, S., Shoorei, H., Mohaqiq, M., and Taheri, M. (2020). 'Non-coding RNAs regulate angiogenic processes. Vasc. Pharmacol. 133-134, 106778. doi:10.1016/j.vph.2020.106778
Guduric-Fuchs, Jasenka, O'Connor, Anna, Cullen, Angela, Harwood, Laura, Medina, Reinhold J., ChristinaO'Neill, L., et al. (2012). Deep sequencing reveals predominant expression of miR-21 amongst the small non-coding RNAs in retinal microvascular endothelial cells. J. Cell. Biochem. 113, 2098–2111. doi:10.1002/jcb.24084
Guo, J. R., Yin, L., Chen, Y. Q., Jin, X. J., Zhou, X., Zhu, N. N., et al. (2018). Autologous blood transfusion augments impaired wound healing in diabetic mice by enhancing lncRNA H19 expression via the HIF-1α signaling pathway. Cell Commun. Signal. 16, 84. doi:10.1186/s12964-018-0290-6
Han, N., Tian, W., Yu, N., and Yu, L. (2020). YAP1 is required for the angiogenesis in retinal microvascular endothelial cells via the inhibition of MALAT1-mediated miR-200b-3p in high glucose-induced diabetic retinopathy. J. Cell. Physiol. 235, 1309–1320. doi:10.1002/jcp.29047
Hanna, M., Liu, H., Amir, J., Sun, Y., Morris, S. W., Siddiqui, M. A., et al. (2009). 'Mechanical regulation of the proangiogenic factor CCN1/CYR61 gene requires the combined activities of MRTF-A and CREB-binding protein histone acetyltransferase. J. Biol. Chem. 284, 23125–23136. doi:10.1074/jbc.M109.019059
He, Y., Dan, Y., Gao, X., Huang, L., Lv, H., and Chen, J. (2021). . 'DNMT1-mediated lncRNA MEG3 methylation accelerates endothelial-mesenchymal transition in diabetic retinopathy through the PI3K/Akt/mTOR signaling pathway. Am. J. Physiol. Endocrinol. Metab. 320, E598–E608. doi:10.1152/ajpendo.00089.2020
He, Z., Wang, X., Huang, C., Gao, Y., Yang, C., Zeng, P., et al. (2018). . 'The FENDRR/miR-214-3P/TET2 axis affects cell malignant activity via RASSF1A methylation in gastric cancer. Am. J. Transl. Res. 10, 3211–3223.
Heid, J., Cencioni, C., Ripa, R., Baumgart, M., Atlante, S., Milano, G., et al. (2017). Age-dependent increase of oxidative stress regulates microRNA-29 family preserving cardiac health. Sci. Rep. 7, 16839. doi:10.1038/s41598-017-16829-w
Hellebrekers, D. M., Griffioen, A. W., and van Engeland, M. (2007). 'Dual targeting of epigenetic therapy in cancer. Biochim. Biophys. Acta 1775, 76–91. doi:10.1016/j.bbcan.2006.07.003
Hu, T., Chong, Y., Cai, B., Liu, Y., Lu, S., and Cowell, J. K. (2019). 'DNA methyltransferase 1-mediated CpG methylation of the miR-150-5p promoter contributes to fibroblast growth factor receptor 1-driven leukemogenesis. J. Biol. Chem. 294, 18122–18130. doi:10.1074/jbc.RA119.010144
Huang, J., Li, Y. J., Liu, J. Y., Zhang, Y. Y., Li, X. M., Wang, L. N., et al. (2015). 'Identification of corneal neovascularization-related long noncoding RNAs through microarray analysis. Cornea 34, 580–587. doi:10.1097/ICO.0000000000000389
Jayasuriya, R., Dhamodharan, U., Karan, A. N., Anandharaj, A., Rajesh, K., and Ramkumar, K. M. (2020). Role of Nrf2 in MALAT1/HIF-1α loop on the regulation of angiogenesis in diabetic foot ulcer. Free Radic. Biol. Med. 156, 168–175. doi:10.1016/j.freeradbiomed.2020.05.018
Jiang, X., Huang, H., Li, Z., Li, Y., Wang, X., Gurbuxani, S., et al. (2012). 'Blockade of miR-150 maturation by MLL-fusion/MYC/LIN-28 is required for MLL-associated leukemia. Cancer Cell 22, 524–535. doi:10.1016/j.ccr.2012.08.028
Kaluza, D., Kroll, J., Gesierich, S., Manavski, Y., Boeckel, J. N., Doebele, C., et al. (2013). Histone deacetylase 9 promotes angiogenesis by targeting the antiangiogenic microRNA-17-92 cluster in endothelial cells. Arterioscler. Thromb. Vasc. Biol. 33, 533–543. doi:10.1161/ATVBAHA.112.300415
Ke, Ning, Pi, Lian-Hong, Liu, Qing, and Chen, Lin (2019). 'Long noncoding RNA SNHG7 inhibits high glucose-induced human retinal endothelial cells angiogenesis by regulating miR-543/SIRT1 axis. Biochem. Biophys. Res. Commun. 514, 503–509. doi:10.1016/j.bbrc.2019.04.141
Kim, Y., Nam, H. J., Lee, J., Park, D. Y., Kim, C., Yu, Y. S., et al. (2016). Methylation-dependent regulation of HIF-1α stability restricts retinal and tumour angiogenesis. Nat. Commun. 7, 10347. doi:10.1038/ncomms10347
Klein, R. H., Stephens, D. N., Ho, H., Chen, J. K., Salmans, M. L., and Wang, W. (2017). Cofactors of LIM domains associate with estrogen receptor alpha to regulate the expression of noncoding RNA H19 and corneal epithelial progenitor cell function. J. Biol. Chem. 291, 13271–85.
Kumar, M. M., and Goyal, R. (2017). 'LncRNA as a therapeutic target for angiogenesis. Curr. Top. Med. Chem. 17, 1750–1757. doi:10.2174/1568026617666161116144744
Levy, E., Spahis, S., Bigras, J. L., Delvin, E., and Borys, J. M. (2017). 'The epigenetic machinery in vascular dysfunction and hypertension. Curr. Hypertens. Rep. 19, 52. doi:10.1007/s11906-017-0745-y
Li, F., Huarte, M., Zaratiegui, M., Vaughn, M. W., Shi, Y., Martienssen, R., et al. (2008). 'Lid2 is required for coordinating H3K4 and H3K9 methylation of heterochromatin and euchromatin. Cell 135, 272–283. doi:10.1016/j.cell.2008.08.036
Li, G. Q., Fang, Y. X., Liu, Y., Meng, F. R., Wu, X., Zhang, C. W., et al. (2019). 'MALAT1-Driven inhibition of Wnt signal impedes proliferation and inflammation in fibroblast-like synoviocytes through CTNNB1 promoter methylation in rheumatoid arthritis. Hum. Gene Ther. 30, 1008–1022. doi:10.1089/hum.2018.212
Li, J., Du, S., Shi, Y., Han, J., Niu, Z., Wei, L., et al. (2021). 'Rapamycin ameliorates corneal injury after alkali burn through methylation modification in mouse TSC1 and mTOR genes. Exp. Eye Res. 203, 108399. doi:10.1016/j.exer.2020.108399
Li, X., Wang, J., Qian, H., Wu, Y., Zhang, Z., Hu, Z., et al. (2021). 'Serum exosomal circular RNA expression profile and regulative role in proliferative diabetic retinopathy. Front. Genet. 12, 719312. doi:10.3389/fgene.2021.719312
Li, Z., Guo, X., and Wu, S. (2020). Epigenetic silencing of KLF2 by long non-coding RNA SNHG1 inhibits periodontal ligament stem cell osteogenesis differentiation. Stem Cell Res. Ther. 11, 435. doi:10.1186/s13287-020-01953-8
Lin, J. B., Moolani, H. V., Sene, A., Sidhu, R., Kell, P., Lin, J. B., et al. (2018). 'Macrophage microRNA-150 promotes pathological angiogenesis as seen in age-related macular degeneration. JCI Insight 3, 120157. doi:10.1172/jci.insight.120157
Liu, C. H., Huang, S., Britton, W. R., and Chen, J. (2020). 'MicroRNAs in vascular eye diseases. Int. J. Mol. Sci. 21, E649. doi:10.3390/ijms21020649
Liu, C. H., Sun, Y., Li, J., Gong, Y., Tian, K. T., Evans, L. P., et al. (2015). 'Endothelial microRNA-150 is an intrinsic suppressor of pathologic ocular neovascularization. Proc. Natl. Acad. Sci. U. S. A. 112, 12163–12168. doi:10.1073/pnas.1508426112
Liu, C. H., Wang, Z., Sun, Y., SanGiovanni, J. P., and Chen, J. (2016). Retinal expression of small non-coding RNAs in a murine model of proliferative retinopathy. Sci. Rep. 6, 33947. doi:10.1038/srep33947
Liu, J., Li, Q., Zhang, K. S., Hu, B., Niu, X., Zhou, S. M., et al. (2017). Downregulation of the long non-coding RNA Meg3 promotes angiogenesis after ischemic brain injury by activating Notch signaling. Mol. Neurobiol. 54, 8179–8190. doi:10.1007/s12035-016-0270-z
Liu, X., Zhang, L., Wang, J. H., Zeng, H., Zou, J., Tan, W., et al. (2020). 'Investigation of circRNA expression profiles and analysis of circRNA-miRNA-mRNA networks in an animal (mouse) model of age-related macular degeneration. Curr. Eye Res. 45, 1173–1180. doi:10.1080/02713683.2020.1722179
Liu, Z., Xu, J., Ma, Q., Zhang, X., Yang, Q., Wang, L., et al. (2020). 'Glycolysis links reciprocal activation of myeloid cells and endothelial cells in the retinal angiogenic niche. Sci. Transl. Med. 12, eaay1371. doi:10.1126/scitranslmed.aay1371
Lu, C., Han, H. D., Mangala, L. S., Ali-Fehmi, R., Newton, C. S., Ozbun, L., et al. (2010). Regulation of tumor angiogenesis by EZH2. Cancer Cell 18, 185–197. doi:10.1016/j.ccr.2010.06.016
Lu, J., Huang, Y., Zhang, X., Xu, Y., and Nie, S. (2021). 'Noncoding RNAs involved in DNA methylation and histone methylation, and acetylation in diabetic vascular complications. Pharmacol. Res. 170, 105520. doi:10.1016/j.phrs.2021.105520
Lu, J., Tan, T., Zhu, L., Dong, H., and Xian, R. (2020). Hypomethylation causes MIR21 overexpression in Tumors. Mol. Ther. Oncolytics 18, 47–57.
Lyu, G., Guan, Y., Zhang, C., Zong, L., Sun, L., Huang, X., et al. (2018). 'TGF-beta signaling alters H4K20me3 status via miR-29 and contributes to cellular senescence and cardiac aging. Nat. Commun. 9, 2560. doi:10.1038/s41467-018-04994-z
Maina, P. K., Shao, P., Jia, X., Liu, Q., Umesalma, S., Marin, M., et al. (2017). Histone demethylase PHF8 regulates hypoxia signaling through HIF1α and H3K4me3. Biochim. Biophys. Acta. Gene Regul. Mech. 1860, 1002–1012. doi:10.1016/j.bbagrm.2017.07.005
Martinez, B., and Peplow, P. V. (2021). 'MicroRNAs in laser-induced choroidal neovascularization in mice and rats: Their expression and potential therapeutic targets. Neural Regen. Res. 16, 621–627. doi:10.4103/1673-5374.295271
Merrigan, S. L., and Kennedy, B. N. (2017). 'Vitamin D receptor agonists regulate ocular developmental angiogenesis and modulate expression of dre-miR-21 and VEGF. Br. J. Pharmacol. 174, 2636–2651. doi:10.1111/bph.13875
Miao, A., Lu, J., Wang, Y., Mao, S., Cui, Y., Pan, J., et al. (2020). 'Identification of the aberrantly methylated differentially expressed genes in proliferative diabetic retinopathy. Exp. Eye Res. 199, 108141. doi:10.1016/j.exer.2020.108141
Miao, F., Gonzalo, I. G., Lanting, L., and Natarajan, R. (2004). 'in vivo chromatin remodeling events leading to inflammatory gene transcription under diabetic conditions. J. Biol. Chem. 279, 18091–18097. doi:10.1074/jbc.M311786200
Min, D. J., Ezponda, T., Kim, M. K., Will, C. M., Martinez-Garcia, E., Popovic, R., et al. (2013). MMSET stimulates myeloma cell growth through microRNA-mediated modulation of c-MYC. Leukemia 27, 686–694. doi:10.1038/leu.2012.269
Morita, S., Horii, T., Kimura, M., Ochiya, T., Tajima, S., and Hatada, I. (2013). 'miR-29 represses the activities of DNA methyltransferases and DNA demethylases. Int. J. Mol. Sci. 14, 14647–14658. doi:10.3390/ijms140714647
Nagano, T., and Fraser, P. (2011). 'No-nonsense functions for long noncoding RNAs. Cell 145, 178–181. doi:10.1016/j.cell.2011.03.014
Neumann, P., Jae, N., Knau, A., Glaser, S. F., Fouani, Y., Rossbach, O., et al. (2018). 'The lncRNA GATA6-AS epigenetically regulates endothelial gene expression via interaction with LOXL2. Nat. Commun. 9, 237. doi:10.1038/s41467-017-02431-1
Ni, S., Luo, Z., Jiang, L., Guo, Z., Li, P., Xu, X., et al. (2019). 'UTX/KDM6A deletion promotes recovery of spinal cord injury by epigenetically regulating vascular regeneration. Mol. Ther. 27, 2134–2146. doi:10.1016/j.ymthe.2019.08.009
Nicholas, M. P., and Mysore, N. (2021). 'Corneal neovascularization. Exp. Eye Res. 202, 108363. doi:10.1016/j.exer.2020.108363
Ning, X., Shi, Z., Liu, X., Zhang, A., Han, L., Jiang, K., et al. (2015). 'DNMT1 and EZH2 mediated methylation silences the microRNA-200b/a/429 gene and promotes tumor progression. Cancer Lett. 359, 198–205. doi:10.1016/j.canlet.2015.01.005
Ohtani, K., Vlachojannis, G. J., Koyanagi, M., Boeckel, J. N., Urbich, C., Farcas, R., et al. (2011). Epigenetic regulation of endothelial lineage committed genes in pro-angiogenic hematopoietic and endothelial progenitor cells. Circ. Res. 109, 1219–1229. doi:10.1161/CIRCRESAHA.111.247304
Oliver, V. F., Jaffe, A. E., Song, J., Wang, G., Zhang, P., Branham, K. E., et al. (2015). Differential DNA methylation identified in the blood and retina of AMD patients. Epigenetics 10, 698–707. doi:10.1080/15592294.2015.1060388
Osawa, T., Muramatsu, M., Wang, F., Tsuchida, R., Kodama, T., Minami, T., et al. (2011). 'Increased expression of histone demethylase JHDM1D under nutrient starvation suppresses tumor growth via down-regulating angiogenesis. Proc. Natl. Acad. Sci. U. S. A. 108, 20725–20729. doi:10.1073/pnas.1108462109
Parial, R., Li, H., Li, J., Archacki, S., Yang, Z., Wang, I. Z., et al. (2021). 'Role of epigenetic m(6) A RNA methylation in vascular development: mettl3 regulates vascular development through PHLPP2/mTOR-AKT signaling. FASEB J. 35, e21465. doi:10.1096/fj.202000516RR
Park, S. Y., and Kim, J. S. (2020). 'A short guide to histone deacetylases including recent progress on class II enzymes. Exp. Mol. Med. 52, 204–212. doi:10.1038/s12276-020-0382-4
Penaloza, E., Soto-Carrasco, G., and Krause, B. J. (2020). 'MiR-21-5p directly contributes to regulating eNOS expression in human artery endothelial cells under normoxia and hypoxia. Biochem. Pharmacol. 182, 114288. doi:10.1016/j.bcp.2020.114288
Pisani, F., Cammalleri, M., Dal Monte, M., Locri, F., Mola, M. G., Nicchia, G. P., et al. (2018). 'Potential role of the methylation of VEGF gene promoter in response to hypoxia in oxygen-induced retinopathy: Beneficial effect of the absence of AQP4. J. Cell. Mol. Med. 22, 613–627. doi:10.1111/jcmm.13348
Pitzler, L., Auler, M., Probst, K., Frie, C., Bergmeier, V., Holzer, T., et al. (2016). 'miR-126-3p promotes matrix-dependent perivascular cell attachment, migration and intercellular interaction. Stem Cells 34, 1297–1309. doi:10.1002/stem.2308
Polytarchou, C., Iliopoulos, D., Hatziapostolou, M., Kottakis, F., Maroulakou, I., Struhl, K., et al. (2011). 'Akt2 regulates all Akt isoforms and promotes resistance to hypoxia through induction of miR-21 upon oxygen deprivation. Cancer Res. 71, 4720–4731. doi:10.1158/0008-5472.CAN-11-0365
Portela, A., and Esteller, M. (2010). Epigenetic modifications and human disease. Nat. Biotechnol. 28, 1057–1068. doi:10.1038/nbt.1685
Potente, M., Gerhardt, H., and Carmeliet, P. (2011). 'Basic and therapeutic aspects of angiogenesis. Cell 146, 873–887. doi:10.1016/j.cell.2011.08.039
Qi, Y., Yao, R., Zhang, W., and Cui, Q. (2021). 'KAT1 triggers YTHDF2-mediated ITGB1 mRNA instability to alleviate the progression of diabetic retinopathy. Pharmacol. Res. 170, 105713. doi:10.1016/j.phrs.2021.105713
Qin, W., Zhang, L., Li, Z., Xiao, D., Zhang, Y., Yang, H., et al. (2019). 'SIRT6-mediated transcriptional suppression of MALAT1 is a key mechanism for endothelial to mesenchymal transition. Int. J. Cardiol. 295, 7–13. doi:10.1016/j.ijcard.2019.07.082
Qiu, F., Tong, H., Wang, Y., Tao, J., Wang, H., and Chen, L. (2018). 'Inhibition of miR-21-5p suppresses high glucose-induced proliferation and angiogenesis of human retinal microvascular endothelial cells by the regulation of AKT and ERK pathways via maspin. Biosci. Biotechnol. Biochem. 82, 1366–1376. doi:10.1080/09168451.2018.1459179
Rao, X., Zhong, J., Zhang, S., Zhang, Y., Yu, Q., Yang, P., et al. (2011). 'Loss of methyl-CpG-binding domain protein 2 enhances endothelial angiogenesis and protects mice against hind-limb ischemic injury. Circulation 123, 2964–2974. doi:10.1161/CIRCULATIONAHA.110.966408
Ruiz, M. A., Feng, B., and Chakrabarti, S. (2015). 'Polycomb repressive complex 2 regulates MiR-200b in retinal endothelial cells: Potential relevance in diabetic retinopathy. PLoS One 10, e0123987. doi:10.1371/journal.pone.0123987
Shafabakhsh, R., Aghadavod, E., Ghayour-Mobarhan, M., Ferns, G., and Asemi, Z. (2019). 'Role of histone modification and DNA methylation in signaling pathways involved in diabetic retinopathy. J. Cell. Physiol. 234, 7839–7846. doi:10.1002/jcp.27844
Shan, K., Zhou, R. M., Xiang, J., Sun, Y. N., Liu, C., Lv, M. W., et al. (2020). 'FTO regulates ocular angiogenesis via m(6)A-YTHDF2-dependent mechanism. Exp. Eye Res. 197, 108107. doi:10.1016/j.exer.2020.108107
Shen, J., Yang, X., Xie, B., Chen, Y., Swaim, M., Hackett, S. F., et al. (2008). 'MicroRNAs regulate ocular neovascularization. Mol. Ther. 16, 1208–1216. doi:10.1038/mt.2008.104
Shen, Y., Li, M., Liu, K., Xu, X., Zhu, S., Wang, N., et al. (2020). 'Integrated bioinformatics analysis of aberrantly-methylated differentially-expressed genes and pathways in age-related macular degeneration. BMC Ophthalmol. 20, 119. doi:10.1186/s12886-020-01392-2
Shi, S., Jin, Y., Song, H., and Chen, X. (2019). 'MicroRNA-34a attenuates VEGF-mediated retinal angiogenesis via targeting Notch1. Biochem. Cell Biol. 97, 423–430. doi:10.1139/bcb-2018-0304
Shi, Y., Chen, C., Xu, Y., Liu, Y., Zhang, H., and Liu, Y. (2019). LncRNA FENDRR promotes high-glucose-induced proliferation and angiogenesis of human retinal endothelial cells. Biosci. Biotechnol. Biochem. 83, 869–875. doi:10.1080/09168451.2019.1569499
Shu, X. Z., Zhang, L. N., Zhang, R., Zhang, C. J., He, H. P., Zhou, H., et al. (2015). Histone acetyltransferase p300 promotes MRTF-A-mediates transactivation of VE-cadherin gene in human umbilical vein endothelial cells. Gene 563, 17–23. doi:10.1016/j.gene.2015.02.076
Singh, K., Pal, D., Sinha, M., Ghatak, S., Gnyawali, S. C., Khanna, S., et al. (2017). Epigenetic modification of MicroRNA-200b contributes to diabetic vasculopathy. Mol. Ther. 25, 2689–2704. doi:10.1016/j.ymthe.2017.09.009
Slack, F. J., and Chinnaiyan, A. M. (2019). The role of non-coding RNAs in oncology. Cell 179, 1033–1055. doi:10.1016/j.cell.2019.10.017
Smits, M., Mir, S. E., Nilsson, R. J., van der Stoop, P. M., Niers, J. M., Marquez, V. E., et al. (2011). 'Down-regulation of miR-101 in endothelial cells promotes blood vessel formation through reduced repression of EZH2. PLoS One 6, e16282. doi:10.1371/journal.pone.0016282
Sonkar, R., Kay, M. K., and Choudhury, M. (2019). 'PFOS modulates interactive epigenetic regulation in first-trimester human trophoblast cell line HTR-8/SVneo. Chem. Res. Toxicol. 32, 2016–2027. doi:10.1021/acs.chemrestox.9b00198
Su, K., Lin, N., Xie, S., Han, Y., Yang, Z., Zhang, H., et al. (2020). DNMT3A inhibits E2F1-induced arterial marker expression and impairs angiogenesis in human umbilical artery endothelial cells. Acta Biochim. Biophys. Sin. 52, 1236–1246. doi:10.1093/abbs/gmaa109
Sun, B., Ding, Y., Jin, X., Xu, S., and Zhang, H. (2019). Long non-coding RNA H19 promotes corneal neovascularization by targeting microRNA-29c. Biosci. Rep. 39, BSR20182394. doi:10.1042/BSR20182394
Tacconi, C., He, Y., Ducoli, L., and Detmar, M. (2021). Epigenetic regulation of the lineage specificity of primary human dermal lymphatic and blood vascular endothelial cells. Angiogenesis 24, 67–82. doi:10.1007/s10456-020-09743-9
Tang, W., Guo, J., Gu, R., Lei, B., Ding, X., Ma, J., et al. (2020). MicroRNA-29b-3p inhibits cell proliferation and angiogenesis by targeting VEGFA and PDGFB in retinal microvascular endothelial cells. Mol. Vis. 26, 64–75.
Tee, A. E., Ling, D., Nelson, C., Atmadibrata, B., Dinger, M. E., Xu, N., et al. (2014). The histone demethylase JMJD1A induces cell migration and invasion by up-regulating the expression of the long noncoding RNA MALAT1. Oncotarget 5, 1793–1804. doi:10.18632/oncotarget.1785
Thomas, A. A., Biswas, S., Feng, B., Chen, S., Gonder, J., and Chakrabarti, S. (2019). lncRNA H19 prevents endothelial-mesenchymal transition in diabetic retinopathy. Diabetologia 62, 517–530. doi:10.1007/s00125-018-4797-6
Tong, P., Peng, Q. H., Gu, L. M., Xie, W. W., and Li, W. J. (2019). LncRNA-MEG3 alleviates high glucose induced inflammation and apoptosis of retina epithelial cells via regulating miR-34a/SIRT1 axis. Exp. Mol. Pathol. 107, 102–09.
Tough, D. F., Tak, P. P., Tarakhovsky, A., and Prinjha, R. K. (2016). 'Epigenetic drug discovery: Breaking through the immune barrier. Nat. Rev. Drug Discov. 15, 835–853. doi:10.1038/nrd.2016.185
Tsai, M. C., Manor, O., Wan, Y., Mosammaparast, N., Wang, J. K., Lan, F., et al. (2010). Long noncoding RNA as modular scaffold of histone modification complexes. Science 329, 689–693. doi:10.1126/science.1192002
Tsukada, Y., Fang, J., Erdjument-Bromage, H., Warren, M. E., Borchers, C. H., Tempst, P., et al. (2006). 'Histone demethylation by a family of JmjC domain-containing proteins. Nature 439, 811–816. doi:10.1038/nature04433
Venkatesh, S., and Workman, J. L. (2015). 'Histone exchange, chromatin structure and the regulation of transcription. Nat. Rev. Mol. Cell Biol. 16, 178–189. doi:10.1038/nrm3941
Vivanco-Rojas, O., Garcia-Bermudez, M. Y., Iturriaga-Goyon, E., Rebollo, W., Buentello-Volante, B., Magana-Guerrero, F. S., et al. (2020). Corneal neovascularization is inhibited with nucleolin-binding aptamer, AS1411. Exp. Eye Res. 193, 107977. doi:10.1016/j.exer.2020.107977
Wan, S. S., Pan, Y. M., Yang, W. J., Rao, Z. Q., and Yang, Y. N. (2020). 'Inhibition of EZH2 alleviates angiogenesis in a model of corneal neovascularization by blocking FoxO3a-mediated oxidative stress. FASEB J. 34, 10168–10181. doi:10.1096/fj.201902814RRR
Wang, C., Qu, Y., Suo, R., and Zhu, Y. (2019). Long non-coding RNA MALAT1 regulates angiogenesis following oxygen-glucose deprivation/reoxygenation. J. Cell. Mol. Med. 23, 2970–2983. doi:10.1111/jcmm.14204
Wang, H., Deng, Q., Lv, Z., Ling, Y., Hou, X., Chen, Z., et al. (2019). 'N6-methyladenosine induced miR-143-3p promotes the brain metastasis of lung cancer via regulation of VASH1. Mol. Cancer 18, 181. doi:10.1186/s12943-019-1108-x
Wang, W., Sidoli, S., Zhang, W., Wang, Q., Wang, L., Jensen, O. N., et al. (2017). 'Abnormal levels of histone methylation in the retinas of diabetic rats are reversed by minocycline treatment. Sci. Rep. 7, 45103. doi:10.1038/srep45103
Wang, Y., Wang, X., Wang, Y. X., Ma, Y., and Di, Y. (2020). 'Effect and mechanism of the long noncoding RNA MALAT1 on retinal neovascularization in retinopathy of prematurity. Life Sci. 260, 118299. doi:10.1016/j.lfs.2020.118299
Wen, Y., Chun, Y., Lian, Z. Q., Yong, Z. W., Lan, Y. M., Huan, L., et al. (2021). circRNA0006896miR1264DNMT1 axis plays an important role in carotid plaque destabilization by regulating the behavior of endothelial cells in atherosclerosis. Mol. Med. Rep. 23, 311. doi:10.3892/mmr.2021.11950
Wenger, R. H., Kvietikova, I., Rolfs, A., and Camenisch, G. (1998). 'Oxygen-regulated erythropoietin gene expression is dependent on a CpG methylation-free hypoxia-inducible factor-1 DNA-binding site. Eur. J. Biochem. 253, 771–777. doi:10.1046/j.1432-1327.1998.2530771.x
Wiener, D., and Schwartz, S. (2021). The epitranscriptome beyond m(6)A. Nat. Rev. Genet. 22, 119–131. doi:10.1038/s41576-020-00295-8
Wu, X., Li, R., Song, Q., Zhang, C., Jia, R., Han, Z., et al. (2019). 'JMJD2C promotes colorectal cancer metastasis via regulating histone methylation of MALAT1 promoter and enhancing beta-catenin signaling pathway. J. Exp. Clin. Cancer Res. 38, 435. doi:10.1186/s13046-019-1439-x
Xia, C., Tao, Y., Li, M., Che, T., and Qu, J. (2020). 'Protein acetylation and deacetylation: An important regulatory modification in gene transcription (Review). Exp. Ther. Med. 20, 2923–2940. doi:10.3892/etm.2020.9073
Xu, M., Chen, X., Lin, K., Zeng, K., Liu, X., Pan, B., et al. (2018). 'The long noncoding RNA SNHG1 regulates colorectal cancer cell growth through interactions with EZH2 and miR-154-5p. Mol. Cancer 17, 141. doi:10.1186/s12943-018-0894-x
Xu, Y., Wang, Y., Yan, S., Zhou, Y., Yang, Q., Pan, Y., et al. (2017). 'Intracellular adenosine regulates epigenetic programming in endothelial cells to promote angiogenesis. EMBO Mol. Med. 9, 1263–1278. doi:10.15252/emmm.201607066
Xu, Z. M., Huang, F., and Huang, W. Q. (2018). 'Angiogenic lncRNAs: A potential therapeutic target for ischaemic heart disease. Life Sci. 211, 157–171. doi:10.1016/j.lfs.2018.09.022
Xue, W. L., Chen, R. Q., Zhang, Q. Q., Li, X. H., Cao, L., Li, M. Y., et al. (2020). Hydrogen sulfide rescues high glucose-induced migration dysfunction in HUVECs by upregulating miR-126-3p. Am. J. Physiol. Cell Physiol. 318, C857–C869. doi:10.1152/ajpcell.00406.2019
Yamane, K., Toumazou, C., Tsukada, Y., Erdjument-Bromage, H., Tempst, P., Wong, J., et al. (2006). 'JHDM2A, a JmjC-containing H3K9 demethylase, facilitates transcription activation by androgen receptor. Cell 125, 483–495. doi:10.1016/j.cell.2006.03.027
Yang, J., Yang, K., Meng, X., Liu, P., Fu, Y., and Wang, Y. (2021). Silenced SNHG1 inhibited epithelial-mesenchymal transition and inflammatory response of ARPE-19 cells induced by high glucose. J. Inflamm. Res. 14, 1563–1573. doi:10.2147/JIR.S299010
Yao, M. D., Jiang, Q., Ma, Y., Liu, C., Zhu, C. Y., Sun, Y. N., et al. (2020). 'Role of METTL3-dependent N(6)-methyladenosine mRNA modification in the promotion of angiogenesis. Mol. Ther. 28, 2191–2202. doi:10.1016/j.ymthe.2020.07.022
Ye, L., Peng, Y., Mo, J., and Yao, Y. (2018). MiR-126 enhances VEGF expression in induced pluripotent stem cell-derived retinal neural stem cells by targeting spred-1. Int. J. Clin. Exp. Pathol. 11, 1023–1030.
Yuan, F. L., Li, X., Xu, R. S., Jiang, D. L., and Zhou, X. G. (2014). 'DNA methylation: Roles in rheumatoid arthritis. Cell biochem. Biophys. 70, 77–82. doi:10.1007/s12013-014-9913-8
Yuan, Z., Bian, Y., Ma, X., Tang, Z., Chen, N., and Shen, M. (2019). 'LncRNA H19 knockdown in human amniotic mesenchymal stem cells suppresses angiogenesis by associating with EZH2 and activating vasohibin-1. Stem Cells Dev. 28, 781–790. doi:10.1089/scd.2019.0014
Zhang, C., Hu, J., and Yu, Y. (2020). 'CircRNA is a rising star in researches of ocular diseases. Front. Cell Dev. Biol. 8, 850. doi:10.3389/fcell.2020.00850
Zhang, L., Zeng, H., Wang, J. H., Zhao, H., Zhang, B., Zou, J., et al. (2020a). 'Altered long non-coding RNAs involved in immunological regulation and associated with choroidal neovascularization in mice. Int. J. Med. Sci. 17, 292–301. doi:10.7150/ijms.37804
Zhang, L., Zhang, Q., Lv, L., Jianhua, Z., Ting, C., and Wu, Y. (2020b). 'LncRNA SNHG1 regulates vascular endothelial cell proliferation and angiogenesis via miR-196a. J. Mol. Histol. 51, 117–124. doi:10.1007/s10735-020-09862-z
Zhang, Y., Wang, X., Xu, B., Wang, B., Wang, Z., Liang, Y., et al. (2013). Epigenetic silencing of miR-126 contributes to tumor invasion and angiogenesis in colorectal cancer. Oncol. Rep. 30, 1976–1984. doi:10.3892/or.2013.2633
Zhao, D., Zhao, Y., Wang, J., Wu, L., Liu, Y., Zhao, S., et al. (2020). 'Long noncoding RNA Hotair facilitates retinal endothelial cell dysfunction in diabetic retinopathy. Clin. Sci. 134, 2419–2434. doi:10.1042/CS20200694
Zhao, R., Hou, W., Zhang, Z., Qian, L., and Jiang, L. (2015). 'Differential expression of mir-1 26 and vascular endothelial growth factor in retinal cells of metabolic acidosis-induced neonatal rats. J. Nanosci. Nanotechnol. 15, 2088–2093. doi:10.1166/jnn.2015.9230
Zhou, H., Jiang, S., Chen, J., Ren, X., Jin, J., and Su, S. B. (2014b). 'Largazole, an inhibitor of class I histone deacetylases, attenuates inflammatory corneal neovascularization. Eur. J. Pharmacol. 740, 619–626. doi:10.1016/j.ejphar.2014.06.019
Zhou, H., Jiang, S., Chen, J., and Su, S. B. (2014a). 'Suberoylanilide hydroxamic acid suppresses inflammation-induced neovascularization. Can. J. Physiol. Pharmacol. 92, 879–885. doi:10.1139/cjpp-2014-0117
Zhou, Q., Anderson, C., Zhang, H., Li, X., Inglis, F., Jayagopal, A., et al. (2014). 'Repression of choroidal neovascularization through actin cytoskeleton pathways by microRNA-24. Mol. Ther. 22, 378–389. doi:10.1038/mt.2013.243
Zhou, R. M., Shi, L. J., Shan, K., Sun, Y. N., Wang, S. S., Zhang, S. J., et al. (2020). 'Circular RNA-ZBTB44 regulates the development of choroidal neovascularization. Theranostics 10, 3293–3307. doi:10.7150/thno.39488
Zhou, Y. F., Shi, L. J., Yao, J., Sun, Y. N., Shan, K., Jiang, Q., et al. (2019). Microarray analysis of circRNA expression pattern in corneal neovascularization. Cornea 38, 1443–1449. doi:10.1097/ICO.0000000000002089
Zhou, Y., Yang, H., Xia, W., Cui, L., Xu, R., Lu, H., et al. (2020). 'LncRNA MEG3 inhibits the progression of prostate cancer by facilitating H3K27 trimethylation of EN2 through binding to EZH2. J. Biochem. 167, 295–301. doi:10.1093/jb/mvz097
Zhou, Z. W., Zheng, L. J., Ren, X., Li, A. P., and Zhou, W. S. (2019). 'LncRNA NEAT1 facilitates survival and angiogenesis in oxygen-glucose deprivation (OGD)-induced brain microvascular endothelial cells (BMECs) via targeting miR-377 and upregulating SIRT1, VEGFA, and BCL-XL. Brain Res. 1707, 90–98. doi:10.1016/j.brainres.2018.10.031
Keywords: ocular neovascular, epigenetic regulation, non-coding RNAs, RNA modification, DNA methylation, histone modifications
Citation: Hu Q, Zhang X, Sun M, jiang B, Zhang Z and Sun D (2022) Potential epigenetic molecular regulatory networks in ocular neovascularization. Front. Genet. 13:970224. doi: 10.3389/fgene.2022.970224
Received: 20 June 2022; Accepted: 02 August 2022;
Published: 02 September 2022.
Edited by:
Trygve Tollefsbol, University of Alabama at Birmingham, United StatesReviewed by:
Lin Cheng, The University of Iowa, United StatesIgnacio Fernando Hall, University of Edinburgh, United Kingdom
Copyright © 2022 Hu, Zhang, Sun, jiang, Zhang and Sun. This is an open-access article distributed under the terms of the Creative Commons Attribution License (CC BY). The use, distribution or reproduction in other forums is permitted, provided the original author(s) and the copyright owner(s) are credited and that the original publication in this journal is cited, in accordance with accepted academic practice. No use, distribution or reproduction is permitted which does not comply with these terms.
*Correspondence: Dawei Sun, sun.dawei@hotmail.com