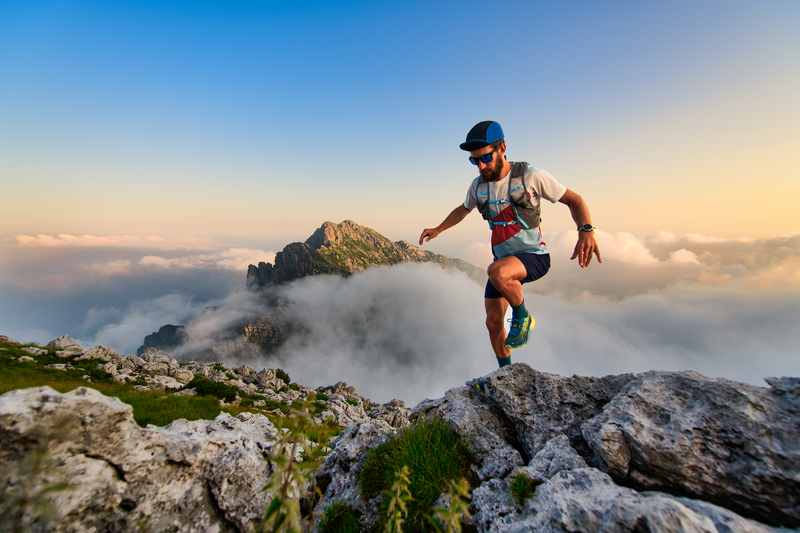
95% of researchers rate our articles as excellent or good
Learn more about the work of our research integrity team to safeguard the quality of each article we publish.
Find out more
REVIEW article
Front. Genet. , 23 August 2022
Sec. Epigenomics and Epigenetics
Volume 13 - 2022 | https://doi.org/10.3389/fgene.2022.949252
This article is part of the Research Topic Application of Epigenomics Data to Improve Human and Livestock Health View all 14 articles
Whether under anaerobic or aerobic conditions, glycolysis results in production of lactate. Increasing evidence suggests that lactate serves as a multifunctional signaling molecule that develops non-metabolic activities in addition to serving as a key metabolite to link glycolysis and oxidative phosphorylation. Histone posttranslational modification patterns (HPTMs) are essential epigenetic processes controlling a variety of biological activities. Proteomics based on mass spectrometry (MS) has been used to progressively reveal new HPTMs. Recent discoveries of histone lactylation modification mediated by lactate and subsequent research demonstrating its involvement in cancer, inflammation, lung fibrosis, and other conditions suggest that it plays a significant role in immune regulation and homeostasis maintenance. This review provides a brief overview of the complicated control of histone lactylation modification in both pathological and physiological conditions.
As part of the glycolysis process, lactate dehydrogenase (LDH) catalyzes a specific type of hydroxycarboxylic acid. It is produced by pyruvate breakdown in either anaerobic or aerobic conditions (Fletcher, 1907; Rogatzki et al., 2015). l-lactate and d-lactate are the two enantiomers of lactate that reside in the human body (Oh et al., 1979; Levitt and Levitt, 2020). The former is primarily found in human serum, whereas the latter comes from dietary intake. l-lactate is referred to in this article whenever the word lactate is not explicitly used. For quite a long time, lactate was thought to be a waste product of anaerobic glycolysis. However, emerging data suggest that lactate serves as a multifunctional bio-signaling molecule in addition to being a key metabolite connecting glycolysis and oxidative phosphorylation. On the one hand, lactate regulates intracellular and extracellular metabolic processes across the entire body. On the other hand, it also has a variety of biological effects, including anti-inflammation, immunological regulation, and gene expression, through receptors expressed in different cells and tissues (Ferguson et al., 2018; Brooks, 2020). Histone undergoes posttranslational alterations in both the C-terminal region and projecting N-terminal tails, which play a crucial role in histone modifications. Numerous histone modifications, including acetylation, methylation, and crotonylation, have been documented before the 2019 discovery of histone lactylation. Recent findings by Zhang et al. state that lactate contributed to epigenetic regulation of genes by lactylating histone lysine residues and that lactate was found to be a precursor to histone lysine lactylation (Kla), which stimulated gene transcription from chromatin (Zhang et al., 2019); these findings demonstrate lactylation’s critical role in immune regulation and homeostasis maintenance. Furthermore, lactylation modifications have primarily been studied on histones as of late, but we hypothesize that lactylation also occurs on non-histone proteins, similar to other modifications such as crotonylation. Additionally, the “YnLac” chemical reporter, which has an alkynyl functionalized bioorthogonal structure, may detect new lactylation modification sites in non-histone proteins (Sun et al., 2022). In addition, several microbes and plants have also been identified with lactylated global proteins, including non-histone (Gao et al., 2020; Zhang et al., 2021a; Meng et al., 2021; Sun et al., 2022). On the other hand, there are currently no investigations of non-histone lactylation in mammals, and additional research is required. In this review, we hope to share information on histone lactylation and discuss new findings that demonstrate how this process regulates a variety of pathological conditions.
Core histones (H2A, H2B, H3, and H4) and linker histones (H1 and H5), which are alkaline, positively charged proteins, are found in histones. The elementary unit of a chromosome is the nucleosome, which consists of a histone octamer with 200 bp of DNA wrapped around it. Histone posttranslational modification (PTM) is a part of epigenetics. The term “epigenetics” refers to changes in gene expression that are heritable during cell division but do not include changes in the DNA coding sequence. Through covalent modification, different acyl groups can be joined to the amino acid residues on histones. Acyl groups have a variety of impacts on how tightly histone and DNA are bound because of various covalent modification forces (Strahl and Allis, 2000; Tessarz and Kouzarides, 2014; Bowman and Poirier, 2015; Zhang et al., 2021b). This variation will be amplified during the gene expression process, finally resulting in different biological signals that cause transcriptional activation or gene silencing (Table 1). So, histone lactylation will result in different biological responses.
In 2019, Zhang et al. discovered that in human breast cancer cells, there was a mass shift which is 72.021Da on lysine residues by mass spectrometry (Zhang et al., 2019). Because this mass shift matched that produced by the attachment of a lactyl group to the ε-amino group of a lysine residue, the scientists suspected that it was caused by lactate. So, they created a synthetic peptide to see if it had any chemical characteristics with the peptide obtained in vivo that caused the mass change. Additionally, they carried out metabolic labeling studies with isotopic glucose and isotopic lactate to support it, which is in accordance with the conclusion of immunoblotting. The data showed that the unique histone modification known as “lysine lactylation” subsequently was derived directly from either exogenous or endogenous lactate. Zhang et al. made a crucial observation that lysine lactylation differed from lysine acetylation in its kinetics. Under the same conditions, lactylation occurs throughout 24 h, whereas acylations reach a stable state at 6 h. This demonstrates that lactylation and acetylation occur at different times. The scientists genetically removed LDH, which catalyzes the conversion of pyruvate to lactate, and discovered that lysine lactylation was completely abrogated (Zhang et al., 2019). This proved that the kinetics was only affected by lactate. All evidence signifies that lactylation is controlled by lactate and that the process takes longer than that of acetylation.
Specific enzymes or enzyme complexes known as “writers” and “erasers” control the addition and removal of histone acylations, activating transcriptional signals that are then read by effector proteins known as “readers” to influence downstream signal pathways and start various biological events (Strahl and Allis, 2000; Figlia et al., 2020). Therefore, lysine lactylation, a novel histone modification, should have some comparable components evolved for it to regulate gene expressions, just as the other histone modifications have a kit of their enzymes. The “writer” and “eraser” is a pair of enzymes with some opposing enzymatic activities to install or remove lactyl groups from modified lysine residues. The “reader” is a type of protein that specifically recognizes this modification and translates it into a variety of functional outcomes within the cell. Currently, it has been shown that “writer” and “eraser” are components of the histone acetyltransferases (HATs) and histone deacetylases (HDACs), respectively (Zhang et al., 2019; Moreno-Yruela et al., 2022a). Additionally, it should possess a class of substrates known as “lactyl-CoA”, which directly adds a lactyl group to lysine residues (Zhang et al., 2019). Although there is no evidence to support the presence of “lactyl-CoA”, in vivo, we hypothesize that the enzyme ACSS2, also known as acetyl-CoA synthase 2, may be able to produce “lactyl-CoA” since the enzymes found in lactylation are highly coincident with those in acetylation, and in acetylation, ACSS2 produces acetyl-CoA. Zhang et al. showed strong p53-dependent, p300-mediated H3 and H4 lactylation and a commensurate impact on transcription, indicating that p300, an acetyltransferase known for mediating histone lactylation, was responsible for lactate inductions of the histone lactylation (Zhang et al., 2019). After that, Moreno-Yruela, C. et al. demonstrated in vitro that two families of deacetylases, namely, HDAC1-3 (histone deacetylase 1–3) and SIRT1-3 (silent information regulator1-3) were lysine delactylases (Figlia et al., 2020; Moreno-Yruela et al., 2022a). HDACs are a subclass of lysine deacetylases that may cleave lactyllysine marks. There are 18 enzymes in total among two families of HDACs (Delcuve et al., 2012). They confirmed that HDACs 1 and 3 in cells had a higher impact on modified histone to remove the lactyl group since HDAC1-3 showed substantial activity toward lysine lactylation (Moreno-Yruela et al., 2022a). So far, we have determined that p300, HDAC1-3, and SIRT1-3 are responsible for setting up and removing histone lactylation. These results, above all, constitute a crucial step toward a thorough characterization of the regulatory components of this pathway, even if the substrate of lactylation has not yet been identified.
It is worth noting that lysine D-lactylation is said to occur by a non-enzymatic acyl transfer. Methylglyoxal is a reactive molecule produced during glycolysis. In the glyoxalase pathway, glyoxalase 1 traps it as D-lactylglutathionethen, and then glyoxalase 2 regenerates glutathione by releasing d-lactate. During the process, d-lactylglutathione is reactive to nucleophiles and can transfer the D-lactyl acyl group onto lysine residues (Gaffney et al., 2020). HDACs also cause the elimination of D-lactylation, even though no enzymes are involved in the acyl installation. D-lactylation and L-lactylation have differing catalytic efficiencies when removed by HDACs (Moreno-Yruela et al., 2022b). The varied distribution of HDACs may be the cause of this. In the cell nucleus, HDAC1–3 may target a few proteins that have been discovered to have D-lactylation modifications (Gaffney et al., 2020; Jennings et al., 2021). Additionally, SIRT2, which is largely found in the cytoplasm, is more likely to break down D-lactylation. However, there are still a lot of questions that require additional research.
Similarly, we use acetylation as a model to determine how lysine lactylation occurs (Figure 1). We hypothesize that cells have a “lactate clock” (Zhang et al., 2019). When exogenous or endogenous lactate accumulates to a certain quantity in cells, the “lactate clock” is activated and initiates lysine lactylation. Then, relevant enzymes start the process. First, the “writer” transfers “lactyl-CoA” as a substrate to histone lysine residues. This generates that the degree to which the changed histone binds to the lactyl group alters how tightly it binds to the DNA molecule, indirectly controlling the expression of genes. Then, the “erasers” arise to complete it, preventing histone lysine from having a lasting impact and stopping the whole cycle of histone lactylation. However, the aforementioned procedure is only capable of reaching the speculation stage; hence, more testing is required. Although the exact mechanism of histone lactylation is unknown, Zhang et al. have identified certain particular locations. From mouse bone marrow–derived macrophages (BMDMs) and human HeLa cells, they found 26 and 16 histone lactylation sites, respectively (Zhang et al., 2019). Additionally, some new sites has been found and all the sites will make it much easier for future researchers to analyze histone lactylation (Figure 2). Interestingly, a newly created predictor called FSL-Klanew may help forecast lactylation sites. According to the model’s inventor, the model can generate candidates for further experimental approaches in addition to being a cutting-edge tool for lactylation site profiles (Zhang et al., 2019; Jiang et al., 2021a).
FIGURE 1. Possible mechanism and roles of histone lactylation in some diseases. Lactate derives from the conversion of glucose by glycolysis, and it may synthesize lactyl-CoA, which is hitherto unclear with the ACSS2. Then, the lactyl group is transferred by p300 to Lys lactylations, leading to various physiopathological activities in different diseases. For example, in human cervical cancer and colitis, inflammation-related genes such as Arg1, Mmp9, Rtn4r, Tgm1, and Spsb4 are upregulated; in embryon, some glycolysis-related genes, including Hk2, Pgk1, Pfk1, Pkm, Eno1, and Ldha, are upregulated; in lung fibrosis, fibrosis-related genes such as Opn, Pdgfa, Thbs1, and Vegfa are upregulated; in ocular melanoma, two tumor suppressor genes, namely, Per1 and Tp53 are upregulated; In sepsis, Kla of HMGB1 is upregulated, contributing to the translocation of HMGB1; in AD, Kla of H4K12 is upregulated, resulting in microglial dysfunction. Abbreviations: ACSS2, synthetase short-chain family member 2; p300, an acetyltransferase; Arg1, arginase1; Mmp9, matrixmetalloproteinase9; Rtn4r, reticulon 4 receptor; Tgm1, transglutaminase 1; Spsb4, SPRY domain- and SOCS box-containing protein 3; Hk2, hexokinase 2; Pgk1, phosphoglycerate kinase1; Pfk1, phosphofructokinase-2; Pkm, pyruvate kinase M2; Eno1, enolase1; Ldha, lactate dehydrogenase; Opn, osteopontin; Pdgfa, platelet-derived growth factor A; Thbs 1, thrombospondin-1; Vegfa, vascular endothelial growth factor A; Per1, period circadian regulator 1; Tp53, tumor suppressor gene TP53; the dashed lines mean that the process has not been proved; The solid lines mean that the process has been proved.
FIGURE 2. Sites of histone lactylation in humans and mice. The lactylation sites identified on the four core histones, namely, H3, H4, H2A, and H2B. The different numbers represent the various sequences of lysine in histones. Some sites, such as H3K18, locate in both humans and mice, shown by both yellow circle icon with “H” and green circle icon with “M”. Others locate in only humans or mice, shown by yellow circle icon with “H” and green circle icon with “M”, respectively.
The numerous histone modifications are more or less connected because differences between them are fundamentally existent only in the modification groups. Therefore, we suspect that other types of histone modifications, especially acetylation, intersect with lactylation. Before the discovery of lactylation, acetylation and glucose metabolism were investigated. For instance, Wellen et al. discovered that the acetyl-CoA generated by ATP citrate lyase served as a bridge between the acetylation process and glucose metabolism (Wellen et al., 2009). Latham et al. later discovered that lactate may inhibit the activity of deacetylase, regulating gene expressions (Latham et al., 2012). Furthermore, it was found by Moussaieff et al. and Li, L et al. that glycolysis also influenced the co-factor and substrate levels of acetylation and that, in many glycolysis-dependent cells, acetyl-CoA typically altered simultaneously with lactate (Moussaieff et al., 2015; Li et al., 2020). There should be a connection between acetylation and lactylation. In fact, it is indeed the same. The histone lysine lactyltransferase, p300, was experimentally shown by Zhang et al. In addition, Moreno-Yruela, C et al. discovered that a portion of HDACs had a delactylating effect (Zhang et al., 2019; Moreno-Yruela et al., 2022a). Since acetylation and lactylation are regulated by both HATs and HDACs, and it is reasonable to assume that the two are connected. Although the link between lactylation and acetylation has not yet been defined, we can make assumptions based on several investigations (de Ruijter et al., 2003). For instance, lactate can increase the levels of lactylation and acetylation in macrophage HMGB1 (Yang et al., 2022a). Additionally, when glucose is used as a treatment, the levels of lactylation and acetylation both increase within a certain limit (Zhang et al., 2019). Mice that are exposed to cold also have higher levels of lactylation and acetylation (Lu et al., 2022). Lactylation and acetylation levels under hypoxia, however, differ depending on the type of cell; in Hela cells, lactylation levels increase, while acetylation levels decrease. In contrast, when lactylation levels increase in murine macrophages, there is no change in acetylation levels (Zhang et al., 2019). Therefore, we believe that it is unreasonable to simply categorize the relationship between lactylation and acetylation or other acylation modifications as synergistic or competitive because the changes in lactylation and acetylation occur differently in diverse cells and respond differently to various stimuli. Although no research has yet provided an explanation for the result, we may assume that they are related to the various subtypes of the pertinent enzymes. As is well-known, various tissues express different subtypes of HDACs differently (de Ruijter et al., 2003). It remains unclear if, even in the presence of the same stimulus, different distribution or activity of a specific subtype of HDACs in cells leads to asynchronous changes in lactylation and acetylation.
Other histone modifications, such as crotonylation and butyrylation, have also been discovered to be connected to lactylation. For instance, a recent discovery indicates that histone lactylation and crotonylation are crucial for epigenetic regulation of brain development. In this work, lactylation and crotonylation were shown to have a synergistic effect on the processes of neural differentiation and cell proliferation (Dai et al., 2022). Lactylation may also be related to butyrylation mediated by butyric acid because butyric acid contributes to an increased lactylation level of whole protein in Hela cells and may prevent lactylation via inhibiting a wide range of HDACs. Similar to this, we hypothesize that lactylation may be connected in some unknown ways to other histone modifications, including butyrylation, propionylation, succinylation, glutarylation, beta-hydroxybutyrylation, and 2-hydroxyisobutyrylation (Chen et al., 2007; Xie et al., 2012; Dai et al., 2014; Xie et al., 2016; Bao et al., 2019). But, we do not fully understand all of this right now; thus, it has to be investigated in further research.
HPTMs are involved in gene activation or gene silencing in cancer and inflammation (Allen et al., 2014; Benayoun et al., 2019; Evans et al., 2020). Belonging to HPTMs, lactylation is also intimately linked to numerous illnesses. An increasing amount of evidence points to the involvement of lactate-mediated histone lactylation in the pathophysiology of several systems. The function of histone lactylation in tumors, inflammation, embryogenesis, neuropathy, and pulmonary fibrosis will be discussed in the following sections.
The microenvironment of tumors is crucial to development and growth of malignancies. Various factors, including metabolites such as lactate, can affect it. Lactate is released by tumor cells and detected by macrophages through transporters found on those cells (Colegio et al., 2014; Chen et al., 2017; Jiang et al., 2021b). In the past, lactate was thought to be a biological marker of malignancy. For instance, Martinez-Z et al. discovered that the accumulation of lactate outside the tumor was closely related to both a shorter overall patient survival rate and a greater incidence of metastasis in tumor patients (Martínez-Zaguilán et al., 1996). The German scientist Otto Heinrich Warburg discovered the Warburg effect in the 20th century, which describes how most tumor cells have a high glucose absorption rate. Tumor cells produced and secreted significant quantities of lactate as a result of aggressive glycolysis occurring in aerobic conditions (Warburg, 1928). The Warburg effect hypothesis brought increased attention to the role of glycolysis in cancer. When lactate from a Warburg-type metabolism was discovered, Arg1 expression surged. It has even been associated with protumor in several systems and is also a marker for M2 macrophages (Colegio et al., 2014; Carmona-Fontaine et al., 2017; Arlauckas et al., 2018). In conclusion, it is not difficult to hypothesize that lactate-induced histone lactylation should be a crucial factor in the growth of tumors. Exact histone lysine lactylation was identified in mouse bone marrow–derived macrophages and HeLa cells. Research studies also demonstrated that lactylation could be enhanced under hypoxia, and intracellular or extracellular lactate could affect it (Zhang et al., 2019). The carcinogenic significance of histone lactylation in ocular melanoma was then shown by Jie Yu et al. They demonstrated that YTH domain family protein 2 (YTHDF2), an m6A (N6-methyladenosine) reader protein, is activated by histone lactylation, and this finding provided novel histone lactylation targets for treating ocular melanoma (Yu et al., 2021). It is to be note that it was the first time lactylation of histones was shown to promote oncogene expression and quicken tumor development, indicating that lactylation was involved in tumor development. In tumor immune escape, the cell types known as tumor-infiltrating myeloid cells (TIMs) play a significant role. Recently, Jia et al. discovered that lactylation also controls TIMs. Their findings demonstrated that lactate accumulated in the tumor microenvironment effectively increased methyltransferase-like 3 (METTL3) in TIMs via H3K18la. They discovered it was critical for boosting TIMs’ immunosuppressive abilities using lactylation-driven METTL3-mediated RNA m6A modification after identifying two lactylation modification sites in the zinc-finger domain of METTL3 (Xiong et al., 2022).
It was well-established that inflammation is essential for onset and development of many illnesses (Liu et al., 2017). Growing evidence has shown several molecular mechanisms, and now histone lactylation is also involved in the activation of inflammation (Chen et al., 2019a; Feng et al., 2019; Wenzel et al., 2019; Certo et al., 2021). As a result, histone lactylation may create novel therapeutic strategies for prevention and treatment of various illnesses focused on inflammation (Chen et al., 2019b; Wang et al., 2020a; Wang et al., 2020b; Sousa et al., 2020). Under various disease conditions, such as sepsis, cancer, chronic inflammation, and autoimmune diseases, it has been reported that lactate produced by aerobic glycolysis has immunosuppressive effects in local tissues (Sangsuwan et al., 2020). It has also been suggested that glycolysis at high rates may provide a way to meet the increased demand for biosynthetic precursors, which is used for pro-inflammatory protein synthesis after pro-inflammatory immune cell activation (Pucino et al., 2017). Macrophages can be classified as M1 or M2 functional phenotypes, which act in pro-inflammatory or anti-inflammatory capacities, respectively. As was previously reported, Zhang et al. demonstrated that lactate and histone lactylation significantly contributed to transformation of pro-inflammatory M1 macrophages into anti-inflammatory M2 macrophages (Zhang et al., 2019), and this was further supported in adipose tissue. According to other research, lactylation is a consequence rather than a cause of macrophage activation, but it occurs coincidently with an IL-6-and Arg1-dependent metabolic rewiring under inflammatory duress (Palsson-McDermott and O'Neill, 2013). Clinical trials have shown similar findings. In a study of 13 healthy volunteers and 35 critically ill patients with septic shock, Chu, X et al. found that H3K18la may reflect the severity of critical illness and presence of infection, suggesting that H3K18la may have a significant impact on the balance of inflammation in sepsis (Chu et al., 2021). In addition, Irizarry-C et al. showed that when lacking B-cell adapter for PI3K (BCAP), macrophages show impaired lactate production and also exhibit reduced histone lactylation and lower tissue repair gene expression, which results in blunting their reparative transition (Irizarry-Caro et al., 2020). In short, the fact that histone lactylation plays a role in the development of inflammation is affirmative, suggesting a novel avenue for treatment of inflammation.
Recently, it has also been claimed that lactylation affects embryogenesis. Yang et al. discovered that H3K18la, which is a reflection of histone lactylation in the endometrium, participated in remodeling uterine receptivity (Yang et al., 2022b). This finding provided a novel insight for developing potential clinical intervention strategies to increase pregnancy rates following both natural and assisted conception. The effects of hypoxia on the development of mouse pre-implantation embryos were later shown in vitro. In hypoxic conditions (2 percent O2), as compared to atmospheric oxygen content, the lactylation in embryos was significantly less (Yang et al., 2021). Additionally, hypoxia reduces the activity of LDHA, which reduces lactate production. Moreover, another study showed that the accumulation of H3K18la on germline and cleavage embryo genes promotes transcriptional elongation (Tian and Zhou, 2022). The authors found that adding lactate activated the germline genes and cleavage embryo genes in mouse embryonic stem cells (ESCs). So, they proposed that to enhance transcriptional elongation, cofactors are recruited through lactylation, which is stimulated by lactate.
Numerous studies have shown that astrocyte-derived lactate may be utilized to fuel neurons and can also serve as a signaling molecule by activating lactate receptors on the surface of neuronal cell membranes and changing how those receptors operate. In fact, lactylation, as with lactate, is also closely linked to neural activity. Hagihara et al. discovered that the degree of lactylation and lactate was correlated and that lactylation was controlled by neural excitation and social stress in brain cells (Hagihara et al., 2021). Social defeat stress and cerebral stimulation both increase brain lactate and lactylation levels, with the latter being a chronic process. After identifying 63 candidate lysine-lactylated proteins, additional research showed that lower social behavior is associated with higher histone H1la (Hagihara et al., 2021). A recent study found that lactylation had a role in the etiology of Alzheimer’s disease (AD). They discovered that the level of H4K12la was upregulated in Aβ plaque-adjacent microglia; thus, they hypothesized that this increased glycolysis/H4K12la/PKM2 positive feedback loop, which exacerbated microglial dysfunction in AD (Pan et al., 2022). So, this may be a possible therapy for AD.
Fibrosis is a common pathogenic characteristic of many illnesses, particularly interstitial lung disease, including pulmonary fibrosis. Pulmonary fibers may be stimulated by lactate that myofibroblasts and macrophages produce in the extracellular environment. To fulfill its non-metabolic activities, Cui et al. proposed that lactate generated by myofibroblasts may modify the pathogenic phenotype of alveolar macrophages. Then, they demonstrated that there was markedly elevated lactate in the conditioned media of transforming growth factor -β1 (TGF-1)–induced lung myofibroblasts and in the bronchoalveolar lavage fluids (BALFs) from animals with TGF-1-induced or bleomycin-induced lung fibrosis (Cui et al., 2021). Significantly, both the media and BALFs encouraged macrophages to produce pro-fibrotic mediators. Mechanistically, histone lactylation at the promoters of the pro-fibrotic genes in macrophages is boosted, which is consistent with the increase of lactate change in these cells. Histone lactylation and expression of pro-fibrotic genes were mediated by p300, as shown by the reduced levels of these processes in p300-knockdown macrophages (Cui et al., 2021). These discoveries provide fresh insight into the pathophysiology of the crucial role played by myofibroblast glycolysis in the etiology of lung fibrosis.
Most somatic cells contain lactate, and histone lactylation alterations may also be common in vivo. The impact of lactylation on gene expression is well-established. The mechanism of histone lactylation is still unclear. In our hypothesis, when lactate reaches a specific level under healthy or pathological circumstances, it causes lactylation of histones or non-histone to engage in various biochemical processes in the body, influencing the biological reactions of the organism. How it begins, whether substrate enzymes are present before the transfer, and how the body detects when lactylation should end are all yet unknown at present. We, thus, need to conduct additional investigation. As we already noted, lactylation has been shown to occur in some diseases such as tumors, inflammation, and so on. Whether there is lactinylation in other diseases in which lactate increases within a period of time needs further research. For example, when in ischemic and hypoxic disorders such as cardiac ischemia, cells may produce more lactate, resulting in lactylation. Also, in disorders such as rheumatoid arthritis, atherosclerotic plaques, poisoning, and intervertebral disc illnesses, it will happen, too (Cheng et al., 2022). In addition, lactate level also increases in certain physiological states such as exercises, and whether the lactylation levels change during this process (Plaza-Diaz et al., 2022) or whether the function is the same and lactylation level under physiological conditions and disease conditions is different are all worth exploring. Histone lactylation represents a novel face of histone posttranslational modifications, and more research studies are needed to unravel its mysteries. As previously mentioned, D-lactylation and L-lactylation have differing catalytic efficiencies when removed by HDACs (Gaffney et al., 2020; Moreno-Yruela et al., 2022b). This requires further research. Currently, non-histone modifications are poorly studied and deserve more attention. Last, we hope that this article will help readers and provide them with new research ideas.
LC and WH were responsible for the conception and design of the study. Y-MX, H-XH, M-TL, and T-TZ were responsible for data collection, analysis, and image processing. Y-MX wrote the manuscript, and XC revised the manuscript. Y-MX and H-XH were responsible for the final approval of the version to be submitted. All authors read and approved the final manuscript.
The authors are thankful for the support of the National Natural Science Foundation of China (No. 82170834, No. 81970676 and No. 81800741), the Office of Science Technology and Talent Work of Luzhou (No. 2020LZXNYDP02 and No.2021LZXNYD-G01) and the project of Affiliated Hospital of Southwest Medical University (No.11160).
The authors declare that the research was conducted in the absence of any commercial or financial relationships that could be construed as a potential conflict of interest.
All claims expressed in this article are solely those of the authors and do not necessarily represent those of their affiliated organizations, or those of the publisher, the editors, and the reviewers. Any product that may be evaluated in this article, or claim that may be made by its manufacturer, is not guaranteed or endorsed by the publisher.
Allen, B. G., Bhatia, S. K., Anderson, C. M., Eichenberger-Gilmore, J. M., Sibenaller, Z. A., Mapuskar, K. A., et al. (2014). Ketogenic diets as an adjuvant cancer therapy: History and potential mechanism. Redox Biol. 2, 963–970. doi:10.1016/j.redox.2014.08.002
Arlauckas, S. P., Garren, S. B., Garris, C. S., Kohler, R. H., Oh, J., Pittet, M. J., et al. (2018). Arg1 expression defines immunosuppressive subsets of tumor-associated macrophages. Theranostics 8 (21), 5842–5854. doi:10.7150/thno.26888
Bao, X., Liu, Z., Zhang, W., Gladysz, K., Fung, Y. M. E., Tian, G., et al. (2019). Glutarylation of histone H4 lysine 91 regulates chromatin dynamics. Mol. Cell 76 (4), 660–675. e9. doi:10.1016/j.molcel.2019.08.018
Benayoun, B. A., Pollina, E. A., Singh, P. P., Mahmoudi, S., Harel, I., Casey, K. M., et al. (2019). Remodeling of epigenome and transcriptome landscapes with aging in mice reveals widespread induction of inflammatory responses. Genome Res. 29 (4), 697–709. doi:10.1101/gr.240093.118
Bowman, G. D., and Poirier, M. G. (2015). Post-translational modifications of histones that influence nucleosome dynamics. Chem. Rev. 115 (6), 2274–2295. doi:10.1021/cr500350x
Brooks, G. A. (2020). Lactate as a fulcrum of metabolism. Redox Biol. 35, 101454. doi:10.1016/j.redox.2020.101454
Carmona-Fontaine, C., Deforet, M., Akkari, L., Thompson, C. B., Joyce, J. A., and Xavier, J. B. (2017). Metabolic origins of spatial organization in the tumor microenvironment. Proc. Natl. Acad. Sci. U. S. A. 114 (11), 2934–2939. doi:10.1073/pnas.1700600114
Certo, M., Elkafrawy, H., Pucino, V., Cucchi, D., Cheung, K. C. P., and Mauro, C. (2021). Endothelial cell and T-cell crosstalk: Targeting metabolism as a therapeutic approach in chronic inflammation. Br. J. Pharmacol. 178 (10), 2041–2059. doi:10.1111/bph.15002
Chen, D. Q., Feng, Y. L., Chen, L., Liu, J. R., Wang, M., Vaziri, N. D., et al. (2019). Poricoic acid A enhances melatonin inhibition of AKI-to-CKD transition by regulating Gas6/AxlNFκB/Nrf2 axis. Free Radic. Biol. Med. 134, 484–497. doi:10.1016/j.freeradbiomed.2019.01.046
Chen, L., Chen, D. Q., Liu, J. R., Zhang, J., Vaziri, N. D., Zhuang, S., et al. (2019). Unilateral ureteral obstruction causes gut microbial dysbiosis and metabolome disorders contributing to tubulointerstitial fibrosis. Exp. Mol. Med. 51 (3), 1–18. doi:10.1038/s12276-019-0234-2
Chen, P., Zuo, H., Xiong, H., Kolar, M. J., Chu, Q., Saghatelian, A., et al. (2017). Gpr132 sensing of lactate mediates tumor-macrophage interplay to promote breast cancer metastasis. Proc. Natl. Acad. Sci. U. S. A. 114 (3), 580–585. doi:10.1073/pnas.1614035114
Chen, Y., Sprung, R., Tang, Y., Ball, H., Sangras, B., Kim, S. C., et al. (2007). Lysine propionylation and butyrylation are novel post-translational modifications in histones. Mol. Cell. Proteomics 6 (5), 812–819. doi:10.1074/mcp.M700021-MCP200
Cheng, C., Xu, Z. Q., Yang, C., and Wu, X. H. (2022). Lactylation driven by lactate metabolism in the disc accelerates intervertebral disc degeneration: A hypothesis. Midlothian, Scotland: Medical Hypotheses, 159.
Chu, X., Di, C., Chang, P., Li, L., Feng, Z., Xiao, S., et al. (2021). Lactylated histone H3K18 as a potential biomarker for the diagnosis and predicting the severity of septic shock. Front. Immunol. 12, 786666. doi:10.3389/fimmu.2021.786666
Colegio, O. R., Chu, N. Q., Szabo, A. L., Chu, T., Rhebergen, A. M., Jairam, V., et al. (2014). Functional polarization of tumour-associated macrophages by tumour-derived lactic acid. Nature 513 (7519), 559–563. doi:10.1038/nature13490
Cui, H. C., Xie, N., Banerjee, S., Ge, J., Jiang, D., Dey, T., et al. (2021). Lung myofibroblasts promote macrophage profibrotic activity through lactate-induced histone lactylation. Am. J. Respir. Cell Mol. Biol. 64 (1), 115–125. doi:10.1165/rcmb.2020-0360OC
Dai, L. Z., Peng, C., Montellier, E., Lu, Z., Chen, Y., Ishii, H., et al. (2014). Lysine 2-hydroxyisobutyrylation is a widely distributed active histone mark. Nat. Chem. Biol. 10 (5), 365–370. doi:10.1038/nchembio.1497
Dai, S.-K., Liu, P.-P., Li, X., Jiao, L.-F., Teng, Z.-Q., and Liu, C.-M. (2022). Dynamic profiling and functional interpretation of histone Kcr and Kla during neural development. Cambridge, England: Development.
de Ruijter, A. J., van Gennip, A. H., Caron, H. N., Kemp, S., and van Kuilenburg, A. B. P. (2003). Histone deacetylases (HDACs): Characterization of the classical HDAC family. Biochem. J. 370 (3), 737–749. doi:10.1042/BJ20021321
Delcuve, G. P., Khan, D. H., and Davie, J. R. (2012). Roles of histone deacetylases in epigenetic regulation: Emerging paradigms from studies with inhibitors. Clin. Epigenetics 4, 5. doi:10.1186/1868-7083-4-5
Evans, L. W., Stratton, M. S., and Ferguson, B. S. (2020). Dietary natural products as epigenetic modifiers in aging-associated inflammation and disease. Nat. Prod. Rep. 37 (5), 653–676. doi:10.1039/c9np00057g
Feng, Y. L., Cao, G., Chen, D. Q., Vaziri, N. D., Chen, L., Zhang, J., et al. (2019). Microbiome-metabolomics reveals gut microbiota associated with glycine-conjugated metabolites and polyamine metabolism in chronic kidney disease. Cell. Mol. Life Sci. 76 (24), 4961–4978. doi:10.1007/s00018-019-03155-9
Ferguson, B. S., Rogatzki, M. J., Goodwin, M. L., Kane, D. A., Rightmire, Z., and Gladden, L. B. (2018). Lactate metabolism: Historical context, prior misinterpretations, and current understanding. Eur. J. Appl. Physiol. 118 (4), 691–728. doi:10.1007/s00421-017-3795-6
Figlia, G., Willnow, P., and Teleman, A. A. (2020). Metabolites regulate cell signaling and growth via covalent modification of proteins. Dev. Cell 54 (2), 156–170. doi:10.1016/j.devcel.2020.06.036
Fletcher, W. M. (1907). Lactic acid in amphibian muscle. J. Physiol. 35 (4), 247–309. doi:10.1113/jphysiol.1907.sp001194
Gaffney, D. O., Jennings, E. Q., Anderson, C. C., Marentette, J. O., Shi, T., Schou Oxvig, A. M., et al. (2020). Non-enzymatic lysine lactoylation of glycolytic enzymes. Cell Chem. Biol. 27 (2), 206–213. doi:10.1016/j.chembiol.2019.11.005
Gao, M., Zhang, N., and Liang, W. (2020). Systematic analysis of lysine lactylation in the plant fungal pathogen botrytis cinerea. Front. Microbiol. 11, 594743. doi:10.3389/fmicb.2020.594743
Hagihara, H., Shoji, H., Otabi, H., Toyoda, A., Katoh, K., Namihira, M., et al. (2021). Protein lactylation induced by neural excitation. Cell Rep. 37 (2), 109820. doi:10.1016/j.celrep.2021.109820
Irizarry-Caro, R. A., McDaniel, M. M., Overcast, G. R., Jain, V. G., Troutman, T. D., and Pasare, C. (2020). TLR signaling adapter BCAP regulates inflammatory to reparatory macrophage transition by promoting histone lactylation. Proc. Natl. Acad. Sci. U. S. A. 117 (48), 30628–30638. doi:10.1073/pnas.2009778117
Jennings, E. Q., Ray, J. D., Zerio, C. J., Trujillo, M. N., McDonald, D. M., Chapman, E., et al. (2021). Sirtuin 2 regulates protein LactoylLys modifications. Chembiochem. 22 (12), 2102–2106. doi:10.1002/cbic.202000883
Jiang, J., Huang, D., Jiang, Y., Hou, J., Tian, M., Li, J., et al. (2021). Lactate modulates cellular metabolism through histone lactylation-mediated gene expression in non-small cell lung cancer. Front. Oncol. 11, 647559. doi:10.3389/fonc.2021.647559
Jiang, P. R., Ning, W., Shi, Y., Liu, C., Mo, S., Zhou, H., et al. (2021). FSL-kla: A few-shot learning-based multi-feature hybrid system for lactylation site prediction. Comput. Struct. Biotechnol. J. 19, 4497–4509. doi:10.1016/j.csbj.2021.08.013
Latham, T., Mackay, L., Sproul, D., Karim, M., Culley, J., Harrison, D. J., et al. (2012). Lactate, a product of glycolytic metabolism, inhibits histone deacetylase activity and promotes changes in gene expression. Nucleic Acids Res. 40 (11), 4794–4803. doi:10.1093/nar/gks066
Levitt, M. D., and Levitt, D. G. (2020). Quantitative evaluation of D-lactate pathophysiology: New insights into the mechanisms involved and the many areas in need of further investigation. Clin. Exp. Gastroenterol. 13, 321–337. doi:10.2147/CEG.S260600
Li, L., Chen, K., Wang, T., Wu, Y., Xing, G., Chen, M., et al. (2020). Glis1 facilitates induction of pluripotency via an epigenome-metabolome-epigenome signalling cascade. Nat. Metab. 2 (9), 882–892. doi:10.1038/s42255-020-0267-9
Liu, Y. Z., Wang, Y. X., and Jiang, C. L. (2017). Inflammation: The common pathway of stress-related diseases. Front. Hum. Neurosci. 11, 316. doi:10.3389/fnhum.2017.00316
Lu, J., Fu, S., Dai, J., Hu, J., Li, S., Ji, H., et al. (2022). Integrated metabolism and epigenetic modifications in the macrophages of mice in responses to cold stress. J. Zhejiang Univ. Sci. B 23 (6), 461–480. doi:10.1631/jzus.B2101091
Martínez-Zaguilán, R., Seftor, E. A., Seftor, R. E., Chu, Y. W., Gillies, R. J., and Hendrix, M. J. (1996). Acidic pH enhances the invasive behavior of human melanoma cells. Clin. Exp. Metastasis 14 (2), 176–186. doi:10.1007/BF00121214
Meng, X., Baine, J. M., Yan, T., and Wang, S. (2021). Comprehensive analysis of lysine lactylation in rice (oryza sativa) grains. J. Agric. Food Chem. 69 (29), 8287–8297. doi:10.1021/acs.jafc.1c00760
Moreno-Yruela, C., Bæk, M., Monda, F., and Olsen, C. A. (2022). Chiral posttranslational modification to lysine ε-amino groups. Acc. Chem. Res. 55 (10), 1456–1466. doi:10.1021/acs.accounts.2c00115
Moreno-Yruela, C., Zhang, D., Wei, W., Bæk, M., Liu, W., Gao, J., et al. (2022). Class I histone deacetylases (HDAC1-3) are histone lysine delactylases. Sci. Adv. 8 (3), eabi6696. doi:10.1126/sciadv.abi6696
Moussaieff, A., Rouleau, M., Kitsberg, D., Cohen, M., Levy, G., Barasch, D., et al. (2015). Glycolysis-mediated changes in acetyl-CoA and histone acetylation control the early differentiation of embryonic stem cells. Cell Metab. 21 (3), 392–402. doi:10.1016/j.cmet.2015.02.002
Oh, M. S., Phelps, K. R., TraubeM., , Barbosa-Saldivar, J. L., Boxhill, C., and Carroll, H. J. (1979). D-lactic acidosis in a man with the short-bowel syndrome. N. Engl. J. Med. 301 (5), 249–252. doi:10.1056/NEJM197908023010505
Palsson-McDermott, E. M., and O'Neill, L. A. (2013). The Warburg effect then and now: From cancer to inflammatory diseases. Bioessays 35 (11), 965–973. doi:10.1002/bies.201300084
Pan, R. Y., He, L., Zhang, J., Liu, X., Liao, Y., Gao, J., et al. (2022). Positive feedback regulation of microglial glucose metabolism by histone H4 lysine 12 lactylation in Alzheimer's disease. Cell Metab. 34 (4), 634–648 e6. doi:10.1016/j.cmet.2022.02.013
Plaza-Diaz, J., Izquierdo, D., Torres-Martos, A., Baig, A. T., Aguilera, C. M., and Ruiz-Ojeda, F. J. (2022). Impact of physical activity and exercise on the epigenome in skeletal muscle and effects on systemic metabolism. Biomedicines 10 (1), 126. doi:10.3390/biomedicines10010126
Pucino, V., Bombardieri, M., Pitzalis, C., and Mauro, C. (2017). Lactate at the crossroads of metabolism, inflammation, and autoimmunity. Eur. J. Immunol. 47 (1), 14–21. doi:10.1002/eji.201646477
Rogatzki, M. J., Ferguson, B. S., Goodwin, M. L., and Gladden, L. B. (2015). Lactate is always the end product of glycolysis. Front. Neurosci. 9, 22. doi:10.3389/fnins.2015.00022
Sangsuwan, R., Thuamsang, B., Pacifici, N., Allen, R., Han, H., Miakicheva, S., et al. (2020). Lactate exposure promotes immunosuppressive phenotypes in innate immune cells. Cell. Mol. Bioeng. 13 (5), 541–557. doi:10.1007/s12195-020-00652-x
Sousa, L. P., Pinho, V., and Teixeira, M. M. (2020). Harnessing inflammation resolving-based therapeutic agents to treat pulmonary viral infections: What can the future offer to COVID-19? Br. J. Pharmacol. 177 (17), 3898–3904. doi:10.1111/bph.15164
Strahl, B. D., and Allis, C. D. (2000). The language of covalent histone modifications. Nature 403 (6765), 41–45. doi:10.1038/47412
Sun, Y., Chen, Y., and Peng, T. (2022). A bioorthogonal chemical reporter for the detection and identification of protein lactylation. Chem. Sci. 13 (20), 6019–6027. doi:10.1039/d2sc00918h
Tessarz, P., and Kouzarides, T. (2014). Histone core modifications regulating nucleosome structure and dynamics. Nat. Rev. Mol. Cell Biol. 15 (11), 703–708. doi:10.1038/nrm3890
Tian, Q., and Zhou, L. Q. (2022). Lactate activates germline and cleavage embryo genes in mouse embryonic stem cells. Cells 11 (3), 548. doi:10.3390/cells11030548
Wang, M., Hu, H. H., Chen, Y. Y., Chen, L., Wu, X. Q., and Zhao, Y. Y. (2020). Novel poricoic acids attenuate renal fibrosis through regulating redox signalling and aryl hydrocarbon receptor activation. Phytomedicine 79, 153323. doi:10.1016/j.phymed.2020.153323
Wang, X., Antony, V., Wang, Y., Wu, G., and Liang, G. (2020). Pattern recognition receptor-mediated inflammation in diabetic vascular complications. Med. Res. Rev. 40 (6), 2466–2484. doi:10.1002/med.21711
Warburg, O. (1928). The chemical constitution of respiration ferment. Science 68 (1767), 437–443. doi:10.1126/science.68.1767.437
Wellen, K. E., Hatzivassiliou, G., Sachdeva, U. M., Bui, T. V., Cross, J. R., and Thompson, C. B. (2009). ATP-citrate lyase links cellular metabolism to histone acetylation. Science 324 (5930), 1076–1080. doi:10.1126/science.1164097
Wenzel, U. O., Bode, M., Kurts, C., and Ehmke, H. (2019). Salt, inflammation, IL-17 and hypertension. Br. J. Pharmacol. 176 (12), 1853–1863. doi:10.1111/bph.14359
Xie, Z. Y., Dai, J., Dai, L., Tan, M., Cheng, Z., Wu, Y., et al. (2012). Lysine succinylation and lysine malonylation in histones. Mol. Cell. Proteomics. 11 (5), 100–107. doi:10.1074/mcp.M111.015875
Xie, Z. Y., Zhang, D., Chung, D., Tang, Z., Huang, H., Dai, L., et al. (2016). Metabolic regulation of gene expression by histone lysine beta-hydroxybutyrylation. Mol. Cell 62 (2), 194–206. doi:10.1016/j.molcel.2016.03.036
Xiong, J., He, J., Zhu, J., Pan, J., Liao, W., Ye, H., et al. (2022). Lactylation-driven METTL3-mediated RNA m(6)A modification promotes immunosuppression of tumor-infiltrating myeloid cells. Mol. Cell 82 (9), 1660–1677 e10. doi:10.1016/j.molcel.2022.02.033
Yang, K., Fan, M., Wang, X., Xu, J., Wang, Y., Tu, F., et al. (2022). Lactate promotes macrophage HMGB1 lactylation, acetylation, and exosomal release in polymicrobial sepsis. Cell Death Differ. 29 (1), 133–146. doi:10.1038/s41418-021-00841-9
Yang, Q., Liu, J., Wang, Y., Zhao, W., Wang, W., Cui, J., et al. (2022). A proteomic atlas of ligand-receptor interactions at the ovine maternal-fetal interface reveals the role of histone lactylation in uterine remodeling. J. Biol. Chem. 298 (1), 101456. doi:10.1016/j.jbc.2021.101456
Yang, W., Wang, P., Cao, P., Wang, S., Yang, Y., Su, H., et al. (2021). Hypoxic in vitro culture reduces histone lactylation and impairs pre-implantation embryonic development in mice. Epigenetics Chromatin 14 (1), 57. doi:10.1186/s13072-021-00431-6
Yu, J., Chai, P., Xie, M., Ge, S., Ruan, J., Fan, X., et al. (2021). Histone lactylation drives oncogenesis by facilitating m(6)A reader protein YTHDF2 expression in ocular melanoma. Genome Biol. 22 (1), 85. doi:10.1186/s13059-021-02308-z
Zhang, D., Tang, Z., Huang, H., Zhou, G., Cui, C., Weng, Y., et al. (2019). Metabolic regulation of gene expression by histone lactylation. Nature 574 (7779), 575–580. doi:10.1038/s41586-019-1678-1
Zhang, N., Jiang, N., Yu, L., Guan, T., Sang, X., Feng, Y., et al. (2021). Protein lactylation critically regulates energy metabolism in the Protozoan parasite trypanosoma brucei. Front. Cell Dev. Biol. 9, 719720. doi:10.3389/fcell.2021.719720
Keywords: histone lactylation, epigenetic, gene transcription, inflammation, tumor, novel posttranslational modification, lactate
Citation: Xie Y, Hu H, Liu M, Zhou T, Cheng X, Huang W and Cao L (2022) The role and mechanism of histone lactylation in health and diseases. Front. Genet. 13:949252. doi: 10.3389/fgene.2022.949252
Received: 20 May 2022; Accepted: 18 July 2022;
Published: 23 August 2022.
Edited by:
Xiao Wang, Konge Larsen ApS, DenmarkReviewed by:
Linchong Sun, Guangdong Academy of Medical Sciences, ChinaCopyright © 2022 Xie, Hu, Liu, Zhou, Cheng, Huang and Cao. This is an open-access article distributed under the terms of the Creative Commons Attribution License (CC BY). The use, distribution or reproduction in other forums is permitted, provided the original author(s) and the copyright owner(s) are credited and that the original publication in this journal is cited, in accordance with accepted academic practice. No use, distribution or reproduction is permitted which does not comply with these terms.
*Correspondence: Wei Huang, aHVhbmd3ZWkxMjEyNTIwQDE2My5jb20=; Ling Cao, bHpjYW9saW5nQDE2My5jb20=aHR0cDovL2x6Y2FvbGluZ0AxNjMuY29t
Disclaimer: All claims expressed in this article are solely those of the authors and do not necessarily represent those of their affiliated organizations, or those of the publisher, the editors and the reviewers. Any product that may be evaluated in this article or claim that may be made by its manufacturer is not guaranteed or endorsed by the publisher.
Research integrity at Frontiers
Learn more about the work of our research integrity team to safeguard the quality of each article we publish.