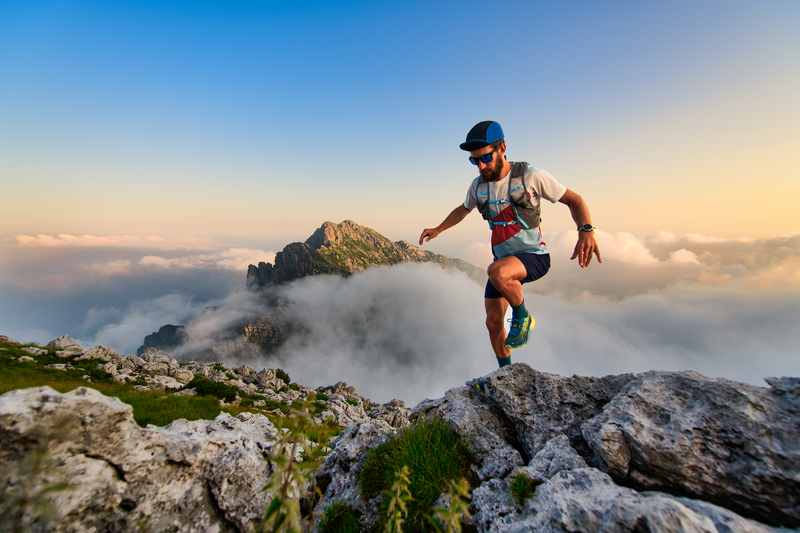
95% of researchers rate our articles as excellent or good
Learn more about the work of our research integrity team to safeguard the quality of each article we publish.
Find out more
BRIEF RESEARCH REPORT article
Front. Genet. , 22 June 2022
Sec. Genomics of Plants and the Phytoecosystem
Volume 13 - 2022 | https://doi.org/10.3389/fgene.2022.926955
This article is part of the Research Topic Breaking the Myth: Breeding for Stress Tolerance, Grain Yield, and Quality Traits Simultaneously by Diversifying the Narrow Genetic Base View all 42 articles
Wheat is one of the most important food crops worldwide. Even though wheat yields have increased considerably in recent years, future wheat production is predicted to face enormous challenges due to global climate change and new versions of diseases. CRISPR/Cas technology is a clean gene technology and can be efficiently used to target genes prone to biotic stress in wheat genome. Herein, the published research papers reporting the genetic factors corresponding to stripe rust, leaf rust, stem rust, powdery mildew, fusarium head blight and some insect pests were critically reviewed to identify negative genetic factors (Susceptible genes) in bread wheat. Out of all reported genetic factors related to these disease, 33 genetic factors (S genes) were found as negative regulators implying that their down-regulation, deletion or silencing improved disease tolerance/resistance. The results of the published studies provided the concept of proof that these 33 genetic factors are potential targets for CRISPR/Cas knockdowns to improve genetic tolerance/resistance against these diseases in wheat. The sequences of the 33 genes were retrieved and re-mapped on the latest wheat reference genome IWGSC RefSeq v2.1. Phylogenetic analysis revealed that pathogens causing the same type of disease had some common conserved motifs and were closely related. Considering the significance of these disease on wheat yield, the S genes identified in this study are suggested to be disrupted using CRISPR/Cas system in wheat. The knockdown mutants of these S genes will add to genetic resources for improving biotic stress resistance in wheat crop.
Wheat (Triticum aestivum L.) is grown in 89 countries and consumed by 2.5 billion people due to its dietary values (CGIAR, 2019). Each growing season, wheat is exposed to a wide range of diseases and pests that affect the crop yield (Gulnaz et al., 2019). Among biotic stresses, pathogenic fungi pose a serious threat to global wheat production. Stripe rust, stem rust, leaf rust, powdery mildew, and head blight are the primary diseases of wheat (Simón et al., 2021). Stripe rust has historically caused and continues to cause catastrophic losses in sensitive wheat cultivars globally (Gad et al., 2019). According to a recent estimate, 21.5% wheat yield losses are due to pests and diseases (Savary et al., 2019). Thus far, various breeding methods and biotechnological tools have exploited resistant genes (R genes) to breed biotic stress tolerant wheat varieties over different periods of time (Supplementary Figure S1). However, susceptible genes (S genes) are not yet explored to improve resistance against pests and diseases.
The advent of CRISPR/Cas (clustered regularly interspaced short palindromic repeats-CRISPR-associated) system such as CRISPR/Cas9 and CRISPR/Cas12 for precise genome editing presents great scope of targeting S genes to improve economical traits in crops including wheat (Mubarik et al., 2021). As an allohexaploid, however, wheat has three closely linked sub genomes that were passed down from three homoeologous ancestors, with 2n = 6x = 42, AABBDD (Petersen et al., 2006). The A, B, and D genomes each contain three copies of a gene that is functionally redundant and complementary. As a result, it is extremely unlikely that natural selection or induced mutagenesis will result in the simultaneous mutation of genes in the human genomes A, B, and D. Consequently, compared to other cereals like rice and maize, wheat’s complicated polyploid structure has hampered the development of functional genomics and breeding (Char et al., 2017), and the failure to eliminate all of a gene’s duplicates may not necessarily result in phenotypic changes due to genome buffering. On the other hand, wheat’s genome is massive (∼17 Gb) and contains a high proportion of repetitive DNA (80%–90%), making targeted modifications extremely difficult. However, with the availability of novel Cas orthologues, gRNA design in the CRISPR/Cas systems has grown more flexible and can be easily created to target a variety of genes (Char and Yang 2020; Gürel et al., 2020).
To date, Cas9 and Cas12a, have been used for genome editing in wheat to create new alleles and disrupt gene’s function (Kefale & Getahun 2022). Due to their unique pros and cons, Cas9 and Cas12a have made the applications of CRISPR/Cas system highly versatile. Cas12a, has certain advantages over Cas9, in its ability to be used for multiplex genome editing and production of staggered DSB (double-stranded break), which promotes HDR (homology-directed repair) instead of NHEJ (non-homologous end joining).
Continuous improvement in genetic resources for biotic stress resistance is pre-requisite for sustained increase in yield potential of newly developing wheat varieties (Alemu, 2019). This report intends to provide a guide for exploiting S genes through CRISPR/Cas knockdowns to develop new genetic resources for breeding biotic stress resistant wheat varieties. The genetically stable knockdown-mutants the S genes could provide new genetic resources for enhancing biotic stress tolerance in future wheat varieties.
Plants have evolved a sophisticated immune system through co-evolution with diseases, while pathogens have developed counter-defense mechanisms. The pathogen-associated molecular patterns (PAMPs) such as bacterial flagellin or viral double-stranded RNAs are detected by PRRs on the cell surface, activating PAMP-triggered immunity (PTI). PTI causes dynamic changes in the defense-responsive transcriptome, reactive oxygen species (ROS) generation, and antimicrobial peptide/compound release in the apoplast (Jighly et al., 2016). Pathogens release virulence proteins or effectors and other poisons to reduce PTI (Huerta-Espino et al., 2011). These effector chemicals also change plant physiology to aid infection. Plants have evolved R genes that may detect effector activities and trigger effector triggered immunity (ETI). Strong defensive responses often cause localized cell death or hypersensitivity (Sharma et al., 2015; Alemu, 2019). S genes, on the other hand, are required for pathogen infection and consequently for suitable plant–pathogen interactions. They help in host identification and penetration, pathogen growth and spread, and negative modulation of immunological signals (Zaidi et al., 2018). While R genes are dominant, disease resistance offered by S genes is recessive and comes with a fitness penalty. S-gene-mediated disease resistance is pathogen specific when the damaged pathway is required for pathogen entry, penetration, or post-penetration. A suitable host surface state is essential for bacterial adhesion or fungal/oomycete spore germination prior to penetration. Pathogens enter their hosts in a variety of ways. Direct penetration through physical or chemical barriers, and indirect penetration through natural cell openings like stomata. Pathogens invade host cells after penetration by avoiding plant monitoring systems and/or dampening numerous levels of defense (Zaidi et al., 2018). In the same way, the target S gene involved in protracted or constitutive defense responses can be broad-spectrum. We use genome editing to target S-gene-mediated pathogen resistance.
The target S genes were selected based on critical review of research papers published from well-known labs. The genes/genetic factors whose absence, down regulation, reduced expression, silencing or loss of function mutation improved resistance against one or more than one of the diseases or insect pests were considered as S genes. Out of >100 genes reported to be associated with stripe rust, leaf rust, stem rust, powdery mildew and fusarium head blight, 33 were selected as S genes with strong concept of proof (Supplementary Table S1). The R genes or the genes without any functional concept of proof were not included in this mini review. Herein, 20 target S genes were selected for stripe rust. For powdery mildew, fusarium head blight, aphides and leaf rust, 4, 7, 1 and 1, target S genes were selected, respectively. Besides improving resistance to disease, the knockdowns of these S genes could cause some yield penalty or negatively affect some other agronomic traits. For example, the knockdown of TaNAC21/22 gene produces necrotic and shorter leaves which can lead significant yield penalty. The side effects of knockdowns of the S genes can be recovered by subsequent backcrossing with wild plants. The CRISPR/Cas knockdowns of these genes to develop null mutants will create valuable genetic resources for breeding against disease in wheat. Further, the orthologs of the selected S genes from Zea mays, Oryza sativa, were also searched to confirm their similar functions in maize and rice using NCBI-BLASTp (https://blast.ncbi.nlm.nih.gov) and phytozyme database (https://phytozome-next.jgi.doe.gov/). Based on their homology, complete sequence information of the selected 33 S genes were extracted from NCBI.
To map the selected S genes Wheat URGI (https://wheat-urgi.versailles. inrae. fr) (Appels et al., 2018) database was used. All the retrieved sequences were matched using public blast. Once the alignment was retrieved, chromosomes to be mapped were selected using IWGSC RefSeq v2.1. The map was constructed based on the highest similarity score and lowest E-values (Figure 1).
For multiple sequence alignment (MSA) of the selected S genes, Clustal W software (Thompson et al., 1994) was used (Supplementary Figure S2). The Molecular Evolutionary Genetics Analysis (MEGA) program is a computer tool that lets you to compare homologous genetic sequences from many classes or multi - gene families, with a focus on implying evolutionary links and patterns of DNA and protein evolution (Kumar et al., 2018). For phylogenetic analysis of the S genes MEGA software (Kumar et al., 2018) was used (Supplementary Figure S3).
All the coding sequences were retrieved from NCBI database and homology between the negative regulator genes was identified. Based on the phylogenetic analysis pathogens causing strip stripe rust, powdery mildew, leaf rust, and fusarium head blight were predicted to have common conserved motifs and to be closely related from evolution perspective.
The CRISPR/Cas9 genome-editing technique has made a big splash in plant genetics. It is the most sophisticated gene - editing system ever built due to its remarkable versatility in attacking any DNA sequence with the greatest specificity and modification effectiveness (100%). Furthermore, unlike typical transgene-carrying GMOs, CRISPR/Cas9 doesn’t really incorporate foreign genes into the plant genome, so genetically altered plants are not (yet) subject to legal constraints. Since its first use in plants 4 years ago, this approach has proven to be an innovative tool for increasing critical mating targets including yield, quality, herbicide tolerance, and biotic/abiotic stress resistance. This method is also utilized to change the patterns and architecture of plant inflorescences, as well as to manipulate gene expression through transcriptional regulation.
CRISPR-based genome editing and CRISPR, when combined with other breakthroughs such as the better transgenic technologies and generation of high-quality genome sequences, would propel rational design-based molecular breeding of polyploid wheat and functional genomics to the forefront of wheat biology. Gene-edited wheat and transgene-free, we believe, will play a crucial role in addressing environmental challenges while boosting maintainable agriculture. This is important to note that it is not a substitute for conventional breeding; rather, it is one of the strategies for speeding up wheat biology and developing wheat breeding programs.
Herein, 33 S genes were selected as potential targets for CRISPR/Cas9 knockdowns. The genetically stable knockdown mutants can be used as valuable parents for designing crosses to breed disease resistant cultivars in wheat. The MSA and phylogenic analysis of the selected genes revealed that the S genes related to a specific disease such as stripe rust share some common conserved motifs and have high sequence similarities. Using this information new S genes could be identified using relevant bioinformatics tools and validated in wet lab experiments.
MT and AY: Collected the data, performed analysis and arranged the information about S genes MS: Conceived the idea and supervised the data collection and improved the draft writing MM and SK: wrote the first draft ML and CH: reviewed and improved the manuscript.
This work was supported by the Major special project of Yunnan Province (202102AE090003, 202102AE090014), and the National Key R&D Program of China (2021YFD1401000).
The authors declare that the research was conducted in the absence of any commercial or financial relationships that could be construed as a potential conflict of interest.
All claims expressed in this article are solely those of the authors and do not necessarily represent those of their affiliated organizations, or those of the publisher, the editors and the reviewers. Any product that may be evaluated in this article, or claim that may be made by its manufacturer, is not guaranteed or endorsed by the publisher.
The Supplementary Material for this article can be found online at: https://www.frontiersin.org/articles/10.3389/fgene.2022.926955/full#supplementary-material
Abou-Attia, M. A., Wang, X., Nashaat Al-Attala, M., Xu, Q., Zhan, G., and Kang, Z. (2016). TaMDAR6acts as a Negative Regulator of Plant Cell Death and Participates Indirectly in Stomatal Regulation during the Wheat Stripe Rust-Fungus Interaction. Physiol. Plant. 156 (3), 262–277. doi:10.1111/ppl.12355
Ahmed, S. M., Liu, P., Xue, Q., Ji, C., Qi, T., Guo, J., et al. (2017). TaDIR1-2, a Wheat Ortholog of Lipid Transfer Protein AtDIR1 Contributes to Negative Regulation of Wheat Resistance against Puccinia Striiformis F. Sp. Tritici. Front. Plant Sci. 8 (April). doi:10.3389/fpls.2017.00521
Alemu, G. (2019). Wheat Breeding for Disease Resistance: Review. Oajmb 4 (2), 1–10. doi:10.23880/oajmb-16000142
Appels, R., Eversole, K., Appels, R., Eversole, K., Feuillet, C., Keller, B., et al. (2018). Shifting the Limits in Wheat Research and Breeding Using a Fully Annotated Reference Genome. Science 361 (6403), aar7191. doi:10.1126/science.aar7191
CGIAR (Consultative Group for International Agricultural Research) (2019). Wheat in the WorldCGIAR Research Program on Wheat.
Char, S. N., Neelakandan, A. K., Nahampun, H., Frame, B., Main, M., Spalding, M. H., et al. (2017). An Agrobacterium -delivered CRISPR/Cas9 System for High-Frequency Targeted Mutagenesis in Maize. Plant Biotechnol. J. 15, 257–268. doi:10.1111/pbi.12611
Char, S. N., and Yang, B. (2020). Genome Editing in Grass Plants. aBIOTECH 1, 41–57. doi:10.1007/s42994-019-00005-x
Duan, X., Wang, X., Fu, Y., Tang, C., Li, X., Cheng, Y., et al. (2013). TaEIL1, a Wheat Homologue ofAtEIN3, Acts as a Negative Regulator in the Wheat-Stripe Rust Fungus Interaction. Mol. Plant Pathol. 14 (7), 728–739. doi:10.1111/mpp.12044
Fabre, F., Rocher, F., Alouane, T., Langin, T., and Bonhomme, L. (2020). Searching for FHB Resistances in Bread Wheat: Susceptibility at the Crossroad. Front. Plant Sci. 11 (June), 1–8. doi:10.3389/fpls.2020.00731
Feng, H., Duan, X., Zhang, Q., Li, X., Wang, B., Huang, L., et al. (2014a). The Target Gene of Tae-miR164, a Novel NAC Transcription Factor from the NAM Subfamily, Negatively Regulates Resistance of Wheat to Stripe Rust. Mol. Plant Pathol. 15, 284–296. doi:10.1111/mpp.12089
Feng, H., Liu, W., Zhang, Q., Wang, X., Wang, X., Duan, X., et al. (2014b). TaMDHAR4, a Monodehydroascorbate Reductase Gene Participates in the Interactions between Wheat and Puccinia Striiformis F. Sp. Tritici. Plant Physiology Biochem. 76, 7–16. doi:10.1016/j.plaphy.2013.12.015
Feng, H., Wang, Q., Zhao, X., Han, L., Wang, X., and Kang, Z. (2016). TaULP5 Contributes to the Compatible Interaction of Adult Plant Resistance Wheat Seedlings-Stripe Rust Pathogen. Physiological Mol. Plant Pathology 96, 29–35. doi:10.1016/j.pmpp.2016.06.008
Gad, M. A., Li, H., Alam, M. A., Sajjad, M., and Li, M. (2019). Geographical Distribution and Virulence Phenotypes of Puccinia Striiformis F. Sp. Tritici from Wheat in Yunnan, China. ScienceAsia 45 (6), 572–580. doi:10.2306/scienceasia1513-1874.2019.45.572
Gordon, C. S., Rajagopalan, N., Risseeuw, E. P., Surpin, M., Ball, F. J., Barber, C. J., et al. (2016). Characterization of triticum Aestivum Abscisic Acid Receptors and a Possible Role for These in Mediating Fusairum Head Blight Susceptibility in Wheat. PLoS ONE 11 (10), e0164996–23. doi:10.1371/journal.pone.0164996
Gulnaz, S., Zulkiffal, M., Sajjad, M., Ahmed, J., Musa, M., Abdullah, M., et al. (2019). Identifying Pakistani Wheat Landraces as Genetic Resources for Yield Potential, Heat Tolerance and Rust Resistance. Int. J. Agric. Biol. 21 (3), 520–526. doi:10.17957/IJAB/15.0924
Guo, J., Bai, P., Yang, Q., Liu, F., Wang, X., Huang, L., et al. (2013). Wheat Zinc Finger Protein TaLSD1, a Negative Regulator of Programmed Cell Death, Is Involved in Wheat Resistance against Stripe Rust Fungus. Plant Physiology Biochem. 71, 164–172. doi:10.1016/j.plaphy.2013.07.009
Guo, S., Zhang, Y., Zeng, P., Li, M., Zhang, Q., and Li, X. (2021). TaBln1 Negatively Regulates Wheat Resistance to Stripe Rust by Reducing Ca 2 + Influx, Plant Physiol. kiac112. doi:10.1093/plphys/kiac112
Gürel, F., Zhang, Y., Sretenovic, S., and Qi, Y. (2020). CRISPR-cas Nucleases and Base Editors for Plant Genome Editing. aBIOTECH 1, 74–87. doi:10.1007/s42994-019-00010-0
Hu, L.-q., Mu, J.-j., Su, P.-s., Wu, H.-y., Yu, G.-h., Wang, G.-p., et al. (2018). Multi-functional Roles of TaSSI2 Involved in Fusarium Head Blight and Powdery Mildew Resistance and Drought Tolerance. J. Integr. Agric. 17 (2), 368–380. doi:10.1016/S2095-3119(17)61680-0
Huai, B., Yang, Q., Wei, X., Pan, Q., Kang, Z., and Liu, J. (2020). TaSTP13 Contributes to Wheat Susceptibility to Stripe Rust Possibly by Increasing Cytoplasmic Hexose Concentration, BMC Plant Biol. 20(1):49. doi:10.1186/s12870-020-2248-2
Huerta-Espino, J., Singh, R. P., Germán, S., McCallum, B. D., Park, R. F., Chen, W. Q., et al. (2011). Global Status of Wheat Leaf Rust Caused by Puccinia Triticina. Euphytica 179 (1), 143–160. doi:10.1007/s10681-011-0361-x
Jaganathan, D., Ramasamy, K., Sellamuthu, G., Jayabalan, S., and Venkataraman, G. (2018a). CRISPR for Crop Improvement: An Update Review. Front. Plant Sci. 9 (July), 1–17. doi:10.3389/fpls.2018.00985
Jaganathan, D., Ramasamy, K., Sellamuthu, G., Jayabalan, S., and Venkataraman, G. (2018b). CRISPR for Crop Improvement: An Update Review. Front. Plant Sci. 9. 985. doi:10.3389/fpls.2018.00985
Jamil, S., Shahzad, R., Ahmad, S., Fatima, R., Zahid, R., Anwar, M., et al. (2020). Role of Genetics, Genomics, and Breeding Approaches to Combat Stripe Rust of Wheat. Front. Nutr. 7 (October), 1–12. doi:10.3389/fnut.2020.580715
Jighly, A., Alagu, M., Makdis, F., Singh, M., Singh, S., Emebiri, L. C., et al. (2016). Genomic Regions Conferring Resistance to Multiple Fungal Pathogens in Synthetic Hexaploid Wheat. Mol. Breed. 36 (Issue 9). doi:10.1007/s11032-016-0541-4
Kage, U., Yogendra, K. N., and Kushalappa, A. C. (2017). TaWRKY70 Transcription Factor in Wheat QTL-2DL Regulates Downstream Metabolite Biosynthetic Genes to Resist Fusarium Graminearum Infection Spread within Spike. Sci. Rep. 7. doi:10.1038/srep42596
Kefale, H., and Getahun, S. (2022). Gene Editing Improves the Agronomic Important Traits of Wheat. CRISPR-Cas9 and Cas12/Cpf1. Editor M. Ansari, Wheat [Working Title], London: IntechOpen. doi:10.5772/intechopen.103867
Kong, L., Liu, Y., Wang, X., and Chang, C. (2020). Insight into the Role of Epigenetic Processes in Abiotic and Biotic Stress Response in Wheat and Barley. Ijms 21 (4), 1480–1515. doi:10.3390/ijms21041480
Kumar, S., Stecher, G., Li, M., Knyaz, C., and Tamura, K. (2018). MEGA X: Molecular Evolutionary Genetics Analysis across Computing Platforms. Mol. Biol. Evol. 35 (6), 1547–1549. doi:10.1093/molbev/msy096
Li, S., Zhang, C., Li, J., Yan, L., Wang, N., and Xia, L. (2021). Present and Future Prospects for Wheat Improvement through Genome Editing and Advanced Technologies. Plant Commun. 2 (Issue 4), 100211. doi:10.1016/j.xplc.2021.100211
Liu, P., Duan, Y., Liu, C., Xue, Q., Guo, J., Qi, T., et al. (2018). The Calcium Sensor TaCBL4 and its Interacting Protein TaCIPK5 Are Required for Wheat Resistance to Stripe Rust Fungus. J. Exp. Bot. 69 (18), 4443–4457. doi:10.1093/jxb/ery227
Mamun, M., Tang, C., Sun, Y., Islam, M., Liu, P., Wang, X., et al. (2018). Wheat Gene TaATG8j Contributes to Stripe Rust Resistance. Ijms 19 (6), 1666. doi:10.3390/ijms19061666
Miché, L., Battistoni, F., Gemmer, S., Belghazi, M., and Reinhold-Hurek, B. (2018). Host-dependent Expression of Rhizobium Leguminosarum Bv. Viciae Hydrogenase Is Controlled at Transcriptional and Post-transcriptional Levels in Legume Nodules. Mol. Plant-Microbe Interact. 19 (5). doi:10.1094/MPMI
Mubarik, M. S., Khan, S. H., and Sajjad, M. (2021). “Key Applications of CRISPR/Cas for Yield and Nutritional Improvement,” in CRISPR Crops. Editors A. Ahmad, S. H. Khan, and Z. Khan (Singapore: Springer). doi:10.1007/978-981-15-7142-8_7
Nalam, V. J., Alam, S., Keereetaweep, J., Venables, B., Burdan, D., Lee, H., et al. (2015). Facilitation of Fusarium Graminearum Infection by 9-Lipoxygenases in Arabidopsis and Wheat. Mol. Plant Microbe Interact. 28 (10), 1142–1152. doi:10.1094/mpmi-04-15-0096-r
Petersen, G., Seberg, O., Yde, M., and Berthelsen, K. (2006). Phylogenetic relationships of Triticum and Aegilops and evidence for the origin of the A, B, and D genomes of common wheat (Triticum aestivum). Mol. Phylogenet. Evol. 39, 70–82. doi:10.1016/j.ympev.2006.01.023
Savary, S., Willocquet, L., Pethybridge, S. J., Esker, P., McRoberts, N., and Nelson, A. (2019). The Global Burden of Pathogens and Pests on Major Food Crops. Nat. Ecol. Evol. 3, 430–439. doi:10.1038/s41559-018-0793-y
Sharma, I., Tyagi, B. S., Singh, G., Venkatesh, K., and Gupta, O. P. (2015). Enhancing Wheat Production - A Global Perspective. Indian J. Agric. Sci. 85 (1), 3–13.
Shi, B., Wang, J., Gao, H., Yang, Q., Wang, Y., Day, B., et al. (2021). The Small GTP-Binding Protein TaRop10 Interacts with TaTrxh9 and Functions as a Negative Regulator of Wheat Resistance against the Stripe Rust. Plant Sci. 309 (May), 110937. doi:10.1016/j.plantsci.2021.110937
Simón, M. R., Börner, A., and Struik, P. C. (2021). Editorial: Fungal Wheat Diseases: Etiology, Breeding, and Integrated Management. Front. Plant Sci. 12, 671060. doi:10.3389/fpls.2021.671060
Soni, N., Altartouri, B., Hegde, N., Duggavathi, R., Nazarian-Firouzabadi, F., and Kushalappa, A. C. (2021). TaNAC032 Transcription Factor Regulates Lignin-Biosynthetic Genes to Combat Fusarium Head Blight in Wheat. Plant Sci. 304 (September 2020), 110820. doi:10.1016/j.plantsci.2021.110820
Su, P., Zhao, L., Li, W., Zhao, J., Yan, J., Ma, X., et al. (2021). Integrated Metabolo-Transcriptomics and Functional Characterization Reveals that the Wheat Auxin Receptor TIR1 Negatively Regulates Defense against Fusarium Graminearum. J. Integr. Plant Biol. 63 (2), 340–352. doi:10.1111/jipb.12992
Su, Z., Bernardo, A., Tian, B., Chen, H., Wang, S., Ma, H., et al. (2019). A Deletion Mutation in TaHRC Confers Fhb1 Resistance to Fusarium Head Blight in Wheat. Nat. Genet. 51 (7), 1099–1105. doi:10.1038/s41588-019-0425-8
Thompson, J. D., Higgins, D. G., and Gibson, T. J. (1994). CLUSTAL W: Improving the Sensitivity of Progressive Multiple Sequence Alignment through Sequence Weighting, Position-specific Gap Penalties and Weight Matrix Choice. Nucl. Acids Res. 22 (22), 4673–4680. doi:10.1093/nar/22.22.4673
Tolerance, B. S., Improvement, C., Zhang, Z, and Xu, P. (2019) Transcription Factors Associated with Abiotic and Biotic Stress Tolerance and Their Potential for Crops Improvement, 10(10):771. doi:10.3390/genes10100771
Van Eck, L., Schultz, T., Leach, J. E., Scofield, S. R., Peairs, F. B., Botha, A.-M., et al. (2010). Virus-induced Gene Silencing of WRKY53 and an Inducible Phenylalanine Ammonia-Lyase in Wheat Reduces Aphid Resistance. Plant Biotechnol. J. 8 (9), 1023–1032. doi:10.1111/j.1467-7652.2010.00539.x
Wang, B., Wei, J., Song, N., Wang, N., Zhao, J., and Kang, Z. (2018). A Novel Wheat NAC Transcription factor,TaNAC30, Negatively Regulates Resistance of Wheat to Stripe Rust. J. Integr. Plant Biol. 60 (5), 432–443. doi:10.1111/jipb.12627
Wang, F., Lin, R., Feng, J., Chen, W., Qiu, D., and Xu, S. (2015). TaNAC1 Acts as a Negative Regulator of Stripe Rust Resistance in Wheat, Enhances Susceptibility to Pseudomonas syringae, and Promotes Lateral Root Development in Transgenic Arabidopsis thaliana. Front. Plant Sci. 6 (February), 1–17. doi:10.3389/fpls.2015.00108
Wang J, J., Tao, F., Tian, W., Guo, Z., Chen, X., Xu, X., et al. (2017). The Wheat WRKY Transcription Factors TaWRKY49 and TaWRKY62 Confer Differential High-Temperature Seedling-Plant Resistance to Puccinia Striiformis F. Sp. Tritici. PLoS ONE 12 (7), e0181963. doi:10.1371/journal.pone.0181963
Wang X, X., Wang, Y., Liu, P., Ding, Y., Mu, X., Liu, X., et al. (2017). TaRar1 Is Involved in Wheat Defense against Stripe Rust Pathogen Mediated by YrSu. Front. Plant Sci. 8 (February), 1–13. doi:10.3389/fpls.2017.00156
Yang, Q., Huai, B., Lu, Y., Cai, K., Guo, J., Zhu, X., et al. (2020). A Stripe Rust Effector Pst18363 Targets and Stabilises TaNUDX23 that Promotes Stripe Rust Disease. New Phytol. 225 (2), 880–895. doi:10.1111/nph.16199
Zaidi, S. S.-e. -A., Mukhtar, M. S., and Mansoor, S. (2018). Genome Editing: Targeting Susceptibility Genes for Plant Disease Resistance. Trends Biotechnol. 36 (9), 898–906. doi:10.1016/j.tibtech.2018.04.005
Zaman, Q. U., Li, C., Cheng, H., and Hu, Q. (2019). Genome Editing Opens a New Era of Genetic Improvement in Polyploid Crops. Crop J. 7 (2), 141–150. doi:10.1016/j.cj.2018.07.004
Zhang, B., Hua, Y., Wang, J., Huo, Y., Shimono, M., Day, B., et al. (2017). Ta ADF 4 , an Actin-Depolymerizing Factor from Wheat, Is Required for Resistance to the Stripe Rust Pathogen Puccinia Striiformis F. Sp. Tritici. Plant J. 89 (6), 1210–1224. doi:10.1111/tpj.13459
Zhang, N., Yuan, S., Zhao, C., Park, R. F., Wen, X., Yang, W., et al. (2020). TaNAC35 Acts as a Negative Regulator for Leaf Rust Resistance in a Compatible Interaction between Common Wheat and Puccinia Triticina. Mol. Genet. Genomics 296, 279–287. doi:10.1007/s00438-020-01746-x
Zhang, X.-m., Zhang, Q., Pei, C.-l., Li, X., Huang, X.-l., Chang, C.-y., et al. (2018). TaNAC2 Is a Negative Regulator in the Wheat-Stripe Rust Fungus Interaction at the Early Stage. Physiological Mol. Plant Pathology 102, 144–153. doi:10.1016/j.pmpp.2018.02.002
Keywords: S genes, crispr/cas, stripe rust, leaf rust, powdery mildew, biotic stress, wheat, genetic resources
Citation: Taj M, Sajjad M, Li M, Yasmeen A, Mubarik MS, Kaniganti S and He C (2022) Potential Targets for CRISPR/Cas Knockdowns to Enhance Genetic Resistance Against Some Diseases in Wheat (Triticum aestivum L.). Front. Genet. 13:926955. doi: 10.3389/fgene.2022.926955
Received: 23 April 2022; Accepted: 10 May 2022;
Published: 22 June 2022.
Edited by:
Karansher Singh Sandhu, Bayer Crop Science (United States), United StatesReviewed by:
Pranav Dawar, Texas Tech University, United StatesCopyright © 2022 Taj, Sajjad, Li, Yasmeen, Mubarik, Kaniganti and He. This is an open-access article distributed under the terms of the Creative Commons Attribution License (CC BY). The use, distribution or reproduction in other forums is permitted, provided the original author(s) and the copyright owner(s) are credited and that the original publication in this journal is cited, in accordance with accepted academic practice. No use, distribution or reproduction is permitted which does not comply with these terms.
*Correspondence: Muhammad Sajjad, bXVoYW1tYWQuc2FqamFkQGNvbXNhdHMuZWR1LnBr; Mingju Li, bGlseTY5NjE4QDE2My5jb20=
Disclaimer: All claims expressed in this article are solely those of the authors and do not necessarily represent those of their affiliated organizations, or those of the publisher, the editors and the reviewers. Any product that may be evaluated in this article or claim that may be made by its manufacturer is not guaranteed or endorsed by the publisher.
Research integrity at Frontiers
Learn more about the work of our research integrity team to safeguard the quality of each article we publish.