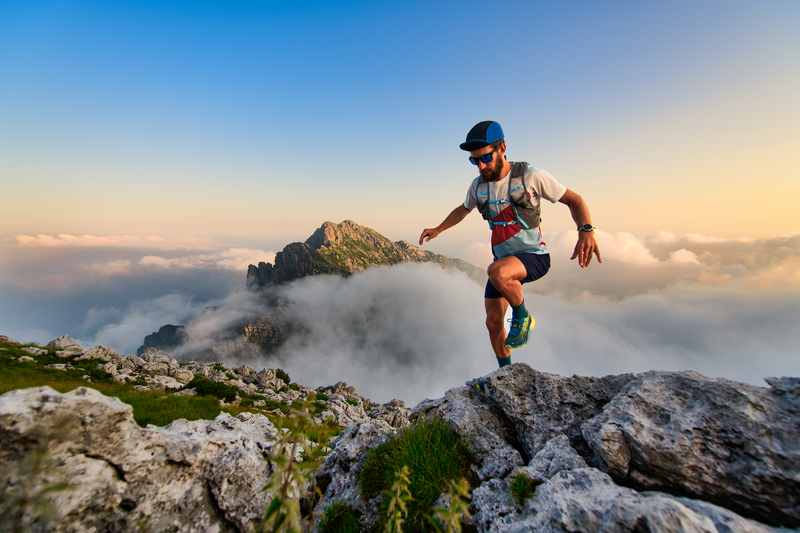
94% of researchers rate our articles as excellent or good
Learn more about the work of our research integrity team to safeguard the quality of each article we publish.
Find out more
REVIEW article
Front. Genet. , 19 August 2022
Sec. RNA
Volume 13 - 2022 | https://doi.org/10.3389/fgene.2022.916233
This article is part of the Research Topic The Role of Extracellular Vesicles in Diseases: shedding light on their role in cell-to-cell communication View all 7 articles
The primary cilium is a microtubule-based organelle that extends from a basal body at the surface of most cells. This antenna is an efficient sensor of the cell micro-environment and is instrumental to the proper development and homeostatic control of organs. Recent compelling studies indicate that, in addition to its role as a sensor, the primary cilium also emits signals through the release of bioactive extracellular vesicles (EVs). While some primary-cilium derived EVs are released through an actin-dependent ectocytosis and are called ectosomes (or large EVs, 350–500 nm), others originate from the exocytosis of multivesicular bodies and are smaller (small EVs, 50–100 nm). Ciliary EVs carry unique signaling factors, including protein markers and microRNAs (miRNAs), and participate in intercellular communication in different organism models. This review discusses the mechanism of release, the molecular features, and functions of EVs deriving from cilia, based on the existing literature.
Extracellular vesicles (EVs) are small elements released from the cell into the extracellular space and delimited by a lipid bilayer (Théry et al., 2018). Previously considered artefactual cellular debris (Cocucci et al., 2009), these particles are now recognized as potent means of intercellular communication through the transport of bioactive molecules, including proteins, lipids and non-coding RNA, from a donor to a recipient cell. Since the release of EVs and their molecular cargo is tightly associated with the patho-physiological status of the donor cells and tissues, there is an increasing interest in using EVs from bodily fluids in the non-invasive diagnosis of diseases. While the number of studies reporting on EVs has increased exponentially, the described diversity of these cellular particles has also expanded, rendering their classification difficult. According to the nomenclature established by the International Society of Extracellular Vesicles (ISEV), EVs are separated into two main categories: large EVs and small EVs. Large EVs (sometimes referred to as ectosomes, microvesicles or microparticles) are extracellular vesicles of 100–500 nm, generated by budding from the plasma membrane and released into the cell environment, while small EVs (also referred to as exosomes depending on their molecular markers) measure between 50 and 150 nm and are initially formed inside multivesicular bodies (MVB) before being released outside the cell by fusion of the MVB with the plasma membrane (Meldolesi, 2018). It is worth mentioning that this nomenclature is based chiefly on research on EVs deriving from the cellular cytoplasm, from human and mouse models. Over the past 10 years, a new class of EVs has come under the spotlight of leading laboratories that specialize in ciliary organelles: the ciliary EVs (Wood and Rosenbaum, 2015; Carter and Blacque, 2019; Nachury and Mick, 2019). While it is unclear what category ciliary EVs belong to, small and/or large ectosome-like EVs protruding from the base, the axonemal membrane, or the tip of cilia have been observed in multiple species (Table 1). The most compelling findings of ciliary EVs come from the studies of model organisms such as Chlamydomonas and Caenorhabditis elegans (for review, see Wood et al., 2013; Wang and Barr, 2018), where ciliary EVs participate in the control of mating behavior (Wang et al., 2014; Maguire et al., 2015; Garcia et al., 2018; Carter and Blacque, 2019). The present article provides an overview of the seminal knowledge on ciliary EVs that comes from organism models and puts emphasis on recent studies that delineate the features and mode of release of ciliary EVs from mammalian ciliated cells. Functional investigations into the contribution of ciliary EVs to intercellular communication and the control of biological systems in mammals are still lacking. However, we extend the current knowledge of ciliary EVs to discuss their potential role and use in the context of human diseases, including cilia-related diseases called ciliopathies.
TABLE 1. Microscopic analysis revealed the presence of (A) cilia deformations (e.g., bulb at the tip, membrane bud); (B) vesicles or vesicle-like structures associated with cilia (C) ciliary EVs generated at the ciliary tip (D) alongside the axoneme, or (E) the ciliary base.
The cell plasma membrane is the interface between the cell body and the cell environment that controls many cellular processes, including cell volume, motility, adhesion, and quorum sensing. A large variety of membrane protrusions exist to 1) increase the surface of exchanges between the internal and external cellular environment (e.g., microvilli, microplicae); 2) generate subcellular compartments enriched in receptors and signaling factors to sense the extracellular environment (e.g., primary cilia, filopodia); or 3) control the fluid turbulence in the cell surrounding environment (e.g., motile cilia). Beyond their classical role in cell sensing, accumulating evidence suggests that these membrane protrusions are cellular regions specialized in the release of EVs, which could mediate communication between cells (Rilla, 2021).
Cilia are microtubule-based organelles that emerge from the cell surface to sense and control the extracellular microenvironment. Cilia are composed of an axoneme that extends from a basal body. A transition zone forms a structural gate with Y-shaped connectors between the basal body and the axoneme to control protein entry into the cilium, thus forming a separate subcellular compartment (Garcia-Gonzalo and Reiter, 2012; Li et al., 2012; Takao and Verhey, 2016). From this common archetype, different types of cilia can be distinguished based on their ultrastructure. For instance, the axoneme of motile cilia consists of nine pairs of microtubules surrounding two central singlet microtubules (Fisch and Dupuis-Williams, 2011; Brooks and Wallingford, 2014; Spassky and Meunier, 2017). Along the axoneme, dynein ATPase motor proteins ensure microtubule sliding responsible for ciliary beating, and the intraflagellar transport system (IFT) participates in ciliogenesis (Jordan and Pigino, 2021). The IFT is a critical molecular device for the assembly and maintenance of the primary cilium, allowing bidirectional movement between the base and tip of structural and signaling components. Organized into repeating structures called IFT trains, IFT-A and IFT-B protein complexes act as adapters between the cargoes to be moved and the molecular motors, kinesin-2 (anterograde motor) and dynein-2 (retrograde motor), respectively. In addition to these two types of IFT trains, the BBsome is an octameric complex of Bardet–Biedl syndrome (BBS) proteins that acts as an adapter between the IFT complexes and the cargoes, especially G protein-coupled enriched receptors (GPCRs) (Jordan and Pigino, 2021). Unlike motile cilia, primary cilia lack dynein arms and central microtubules, giving this type of cilium primarily sensory attributes rather than a mechanical beating function (Singla and Reiter, 2006; Satir, 2017; Spasic and Jacobs, 2017). While non-motile primary cilia are solitary organelles that protrude from most post-mitotic cells in pluricellular organisms (Satir et al., 2010; Smith et al., 2020), motile cilia are tufted on the surface of epithelial cells and are found in limited body locations, including the brain, the respiratory and reproductive tracts (Spassky and Meunier, 2017). In these particular regions, the whip-like motion of motile cilia generates fluid flow over the apical surface of the epithelium and is responsible for major physiological functions, e.g., flow of cerebrospinal fluid in the brain, clearing mucus from airways, transporting oocytes in the oviducts, and maintaining sperm in suspension in the efferent ductules to facilitate their concentration (Spassky and Meunier, 2017; Yuan et al., 2021;). Some motile cilia, such as the sperm flagellum or Chlamydomonas flagella, exist as solitary or paired organelles acting as a cell propellor (Silflow and Lefebvre, 2001; Miki and Clapham, 2013 Bertiaux and Bastin, 2020). In contrast to the stirring properties of motile cilia, non-motile primary cilia are highly potent chemo- and mechano-sensors of the extracellular environment. For instance, primary cilia control cell proliferation, differentiation, and polarity in response to fluid shear stress and/or the presence of agonists of different signaling pathways (e.g., Hedgehog, PDGF, Wnt) in the cell surrounding environment (Satir et al., 2010; Pala et al., 2017; Wheway et al., 2018; Anvarian et al., 2019).
Both non-motile primary cilia and motile cilia are mostly located at the apical pole of the cells and form a privileged surface of exchange between the cell cytosol and the extracellular environment. Over the past 15 years, microscopic studies performed on model organisms and mammalian cells have revealed the presence of cilia deformations (e.g., bulb at the tip, membrane bud) (Table 1A), vesicle-like elements associated with the cilium (Table 1B), which evoke the possible capacity of cilia to receive and/or emit ciliary EVs, or the presence of EVs released into the extracellular space (Table 1C-E). The detection of ciliary elements from different biological fluids has further supported the association between cilia and EVs (Marzesco et al., 2005; Dubreuil et al., 2007; Hogan et al., 2009). In addition to improving our comprehension of fundamental cellular biology, these findings open the possibility of applications for non-invasive diagnosis of ciliary dysfunctions and related diseases in humans (Mohieldin et al., 2021a). The release of EVs from motile cilia has mainly been studied in the unicellular green alga Chlamydomonas and the parasite Trypanosoma brucei. In the first organism, EVs are released from the tip and the base of the flagellum (references in Tables 1C,E), while in the second, they come from the nanotubes that bud from flagellar membrane (Szempruch et al., 2016b). To our knowledge, only one study reported the presence of EVs potentially deriving from motile cilia in vertebrates (Tarique et al., 2019). In this report on turtle efferent ductules, the authors used Transmission Electron Microscopy (TEM) to show the presence of EVs associated with motile ciliary axonemes and covered by the ciliary membrane. Additional studies are needed to validate this model and explore the possible role of these ciliary EVs in soma-sperm cell communication. In contrast, the role of primary cilia as a source of EVs in Caenorhabditis elegans ciliated sensory neurons and a large variety of ciliated cell lines has received greater study during the past years (for review, see Garcia et al., 2018; Carter and Blacque, 2019; Nachury and Mick, 2019). It appears that ciliary EVs can be generated from different sites of the cilium: at the ciliary tip (references in Table 1C), alongside the axoneme (references in Table 1D), or at the ciliary base, also called periciliary membrane compartment (PCMC) in C. elegans (references in Table 1E). While ciliary EVs and cytosolic EVs have different compositions and potentially different functions (Mohieldin et al., 2021b; Volz et al., 2021), very little is known about the differences between subpopulations of ciliary EVs from distinct regions of the primary cilia. The best-known example is in C. elegans. In this species, EVs released at the tips of primary cilia of sensory neurons are found in the surrounding extracellular environment, whereas EVs from the PCMC are released into the lumen surrounding the PCMC before being phagocytized by neighboring glial cells (Razzauti and Laurent, 2021). These two EVs subpopulations exert different functions (see below). In mammals, it remains to be determined whether these different origins are associated with different types of ciliary EVs with distinct compositions and functions, or differ according to the species or biological system studied.
According to functional studies performed thus far on model organisms and mammalian cells, ciliary EVs may participate in intercellular communication between cells or animals (Cao et al., 2015; Hoang-Minh et al., 2018; Razzauti and Laurent, 2021; Wang J. et al., 2021), or in the control of ciliary length and composition, notably through the discard of ciliary components (Long et al., 2016; Nager et al., 2017; Phua et al., 2017; Salinas et al., 2017; Akella et al., 2020; Razzauti and Laurent, 2021) (see below).
Once released into the extracellular environment, ciliary EVs exert remote effects on recipient cells, as described in lower species such as Trypanosoma brucei, Chlamydomonas or Caenorhabditis elegans (Wood et al., 2013; Wang et al., 2014; Schorey et al., 2015; Szempruch et al., 2016a; Wang and Barr, 2018; Carter and Blacque, 2019; Luxmi and King, 2022). Trypanosoma brucei rhodesiense produces EVs containing serum resistance-associated protein (SRA), which confers resistance to human innate immunity, by neutralizing the circulating trypanosome lytic factors (TLF). Co-culture experiments have shown that EVs deriving from flagella in T.b. rhodesiense transfer SRA to T.b. brucei and confer resistance to TLF. For Chlamydomonas, flagella are an important source of EVs that are involved in different stages of their life cycle. Indeed, during the vegetative phase, the release of EVs containing an active enzyme (vegetative lytic enzyme protease) allows the digestion of the extracellular matrix (cell wall) of the sporangial cell and the release (“hatching”) of daughter cells (Wood et al., 2013). The authors confirmed the activity of the enzyme by exposing ciliary EVs isolated from wild type Chlamydomonas to sporangia from aflagellar IFT88 null mutants. While mutants were unable to hatch, ciliary EVs restored this function (Wood et al., 2013). Among the bioactive molecules transported by Chlamydomonas ciliary EVs, the membrane polypeptide SAG1-C65 may contribute to this mating success (Cao et al., 2015). Such a role of ciliary EVs in the control of reproductive functions is also observed in C. elegans, where ciliated sensory neurons release polycystin-containing EVs that affects mating related locomotory behaviors in males (Wang et al., 2014; Wang and Barr, 2018). As a consequence of mating, the male will also modulate the composition of EVs released into its surrounding environment, with an increase in the ratio of PKD-2 to CIL-7 EVs depending on the type of populations mated (isolated virgin males vs. mixed males) (Wang J. et al., 2021). In the cephalic sensory organ of C. elegans, ciliated neurons release EVs from PCMC that are phagocytosed by adjacent glial cells. This contributes to the maintenance of the biochemical integrity of the cilium. This intercellular communication mediated by ciliary EVs has been proposed to participate to the morphogenesis of this sensory organ (Akella et al., 2020; Razzauti and Laurent, 2021). Yet, in mammals, such a role for ciliary EVs in intercellular communication remains elusive. A homeostasis function for ciliary EVs has been suggested in the cardiovascular system, where a decrease in the number of circulating ciliary EVs is associated with abnormalities in blood pressure and heart rate (Mohieldin et al., 2020). In bbs4−/− and bbs8−/− mutants, where intraflagellar transport is interrupted, some of the proteins originally destined to be released through large ciliary EVs were found enriched in small EVs deriving from the cell cytoplasm, suggesting the existence of a tight connection between EVs released from different cell compartments (Volz et al., 2021). These protein- and miRNA-enriched EVs were able to modulate Wnt signaling in recipient cells (Volz et al., 2021), confirming their role in intercellular communication. Co-incubation of culture medium containing primary ciliary EVs from cancer cells with glioma cells increased their proliferation. This was confirmed by comparison with culture media from cells lacking primary cilia such as KIF3A−/− or IFT88−/− (Hoang-Minh et al., 2018).
Finally, while the role of the primary cilium as a signaling antenna is well known (Satir et al., 2010; Pala et al., 2017; Wheway et al., 2018; Anvarian et al., 2019), it may also be able to receive signals in the form of EVs or vesicle-like particles. While several microscopy studies have shown an association between EVs and primary cilia (Table 1B), in vitro incubation of isolated urinary PKD positives EVs with primary cilia from sections of rat biliary tree and IMCD3 cells confirmed this interaction, suggesting that the EV-ciliary interaction might also support intercellular communication (Hogan et al., 2009). For instance, EVs isolated from rat biliary tree and incubated with ciliated cholangiocytes decreased their proliferation through the disruption of the ERK signaling pathway and miR-15 A expression (Masyuk et al., 2010).
In BBS8 and osm-3 (anterograde motor kinesin 3) C. elegans mutants, the release of ciliary EV is increased at both the tip of the cilium and the PCMC (Akella et al., 2020; Razzauti and Laurent, 2021), where they serve as a safety valve to maintain the cilium composition. Similarly, when intraflagellar trafficking is blocked in mammalian cells, the number of activated GPCR-like receptors is regulated through their removal by ectocytosis, a mechanism that modulates cell signaling responses (Nager et al., 2017). In addition, by releasing EVs, the cell promotes cilium disassembly and re-entry into mitosis, which is confirmed by direct blockade of actin polymerization or knockdown of actin regulators (Phua et al., 2017; Wang et al., 2019). This disassembly of the cilium is especially important because targeted removal of intraflagellar transport components (e.g., ARL13B, IFT proteins, ICK) could concomitantly limit primary cilium elongation (Phua et al., 2017; Nakamura et al., 2020). More broadly, the control of cilium length could play an important role in the function of the cilium itself. For example, rod photoreceptors are sensory neurons whose length and function are highly dependent on their cilia. In 1969, Young and Bok showed that the discs at the end of the rod photoreceptors were continuously detached and phagocytosed by cells of the retinal pigment epithelium, thus ensuring the continuous renewal of the photoreceptor outer segment (Young and Bok, 1969). In contrast, the capacity for ectocytosis by the photoreceptor cilium is itself blocked during the cilium formation phase. Indeed, in absence of Peripherin-2 (RDS) in murine rds−/− mutants, the rod exhibits a higher capacity to release EVs, which prevents the formation of discs at the base of the outer segment (Salinas et al., 2017). Thus, the control of ciliary EVs release finely regulates the outer segment organization and turnover.
While the release of EVs has been observed from different membrane protrusions and cell types, the underlying mechanism of liberation remains largely unknown. The primary cilium, with its unique structural core and composition, is the best described source of protrusion-derived EVs. Primary cilia are assembled via IFT that traffics microtubule clusters and protein cargo along the axoneme. These structures are essential to ciliary genesis and functions and have long overshadowed the possible functions of actin within the primary cilium (Garcia et al., 2018; Brücker et al., 2020; Smith et al., 2020). In fact, microscopy studies have revealed the presence of F-actin along the axoneme and at the ciliary tip (Brücker et al., 2020; Smith et al., 2020) where it controls the release of EVs (Nager et al., 2017; Phua et al., 2017). For instance, live fluorescence imaging on mammalian cells showed that “decapitation” of the primary cilium tip was preceded by a transient actin polymerization at the site of excision (Phua et al., 2017). In addition, the use of actin poisons prevented the formation of EVs enriched in GPCRs such as neuropeptide Y receptor 2 (NPY2R), supporting a role for actin in the ciliary EV release process (Nager et al., 2017). Recently, a cryo-electron tomography (cryo-ET) study also revealed the presence of EVs in the vicinity of primary cilia isolated from MDCK-II cells. Among the EVs detected, 50% colocalized with actin filaments (Kiesel et al., 2020). The role of actin polymerization is further supported by the recruitment of actin-associated proteins such as Myosin 6, Drebrin, Cofilin-1, Rab7 to the site of EV release (Nager et al., 2017; Phua et al., 2017; Wang et al., 2019). Proteomic analyses confirmed the presence of many actin-related proteins in both the primary cilium and ciliary EVs (Ishikawa et al., 2012; Mick et al., 2015; Kohli et al., 2017; Mohieldin et al., 2021b; Volz et al., 2021), and the detection of these proteins is decreased in urinary EVs of nephronophthisis-related ciliopathy patients (Stokman et al., 2019). However, the functional link between actin-regulators and actin depolymerization inside the cilium requires further exploration. The local and transient actin polymerization occurring at the tip of primary cilia is thought to be coupled with a change in the phosphoinositide (PI-P) composition of the ciliary membrane. The primary cilium membrane of a quiescent cell is enriched in PI(4)P due to the presence of the ciliary phosphatase INPP5E (Inositol Polyphosphate-5-Phosphatase E) (Figure 1A), which converts PI(4,5)P2 to PI(4)P (Chávez et al., 2015; Garcia-Gonzalo et al., 2015). Following ciliary depletion of INPP5E after a proliferative signal, redistribution of PI(4,5)P2 into the cilium is observed (Phua et al., 2017). PI(4,5)P2-dependent actin regulators, including Cofilin-1, Fascin, Kras and SNX9 regulate actin polymerization and subsequent ciliary EV release and deciliation (Phua et al., 2017, 2018). Many molecular factors involved in microtubule stabilization and destabilization (Pugacheva et al., 2007; Plotnikova et al., 2015) can link growth signals, membrane events, and the evolution of actin and microtubule cytoskeletons. While this model provides an outline of the process leading to the release of ciliary EVs, other factors such as the mechanical fluid shear stress appears to be sufficient to generate ciliary EVs in 85% of cilia (Figure 1B) (Mohieldin et al., 2020). This process, confirmed by several simultaneous approaches (e.g., microscopy, proteomics), has been reproduced in endothelial cells (Mohieldin et al., 2021b). As primary cilia are sensory antennae exposed to bodily fluids and their turbulence, the implications of this shear force deserve special attention in future studies. Furthermore, recent research on mutants of proteins involved in IFT raises the possible implications of this transport in the release of ciliary EVs. In normal conditions, the combined action of BBSome, the small GTPase ARL6 and the activated GPCR sensor β-arrestin 2, sequesters the ciliary somatostatin receptor 3 (SSTR3) into the cell (Nager et al., 2017; Ye et al., 2018; Nachury and Mick, 2019). In knockdown or knockout models for BBSome, ARL6 or IFT-B component, retrograde IFT functions are impaired, and SSTR3 accumulates at the ciliary tip before undergoing ectocytosis. These studies therefore support the link existing between BBSome and IFT trains in the control of EV release (Nager et al., 2017; Wang W. et al., 2021; Jordan and Pigino, 2021). EV composition could provide information about the possible involvement of the IFT system in the genesis of ciliary EVs. Indeed, IFT-B is more abundant than IFT-A in EVs released from the primary cilium (Phua et al., 2017; Nakamura et al., 2020), and a defect in BBsome-mediated protein trafficking leads to a transfer of a portion of large EV-specific proteins to small EVs (Volz et al., 2021). Although the mutations of genes that encode IFT proteins lead to a wide variety of ciliopathies (Pigino, 2021), the direct relationship between these diseases and abnormal production of ciliary EVs has never been explored.
FIGURE 1. Model of ectocytosis from the mammal primary cilium. (A) The intraflagellar transport system (IFT) participates in the assembly and maintenance of the primary cilium through the transport of molecular cargos by IFT-A and IFT-B protein complexes. In quiescent cells, the primary ciliary membrane is enriched in PI(4)P due to the presence of the ciliary phosphatase INPP5E which converts PI(4,5)P2 to PI(4)P. (B) Following a proliferation growth signal (1), the ciliary phosphatase INPP5E is removed from the membrane, promoting the redistribution of the phosphoinositide PI(4,5)P2 in the primary cilium. These membrane changes allow the local polymerization of F-actin through the recruitment of actin-associated proteins, followed by the ectocytosis of ciliary EVs enriched in bioactive molecules. Although ectocytosis following actin polymerization was identified at the cilium tip, F-actin was also observed in the vicinity of ciliary EVs present along the axoneme membrane. The activated Neuropeptide Y receptor type 2 (NPY2R) uses ectocytosis as a constitutive way to exit the cilium (2). The mechanical force (3) generated by the surrounding fluid flow also promotes the release of ciliary EVs.
With the growing study of EVs in all bodily fluids, structural and biochemical characterization is needed to distinguish true vesicles from artefactual protein aggregates or cellular debris. For that purpose, consensual guidelines that define the optimal methodologies and relevant markers have been established for the study of EVs, based on the features of cytosolic-derived EVs from human and mouse species (Théry et al., 2018). While the definition of an unambiguous nomenclature to categorize EVs has been attempted, a lack of consensus on the definition of EV markers persists (Théry et al., 2018). This is in part due to the fact that the molecular composition of vesicles varies depending on the model organism/cell type studied, its patho-physiological state and the source/mode of release from the cell (Haraszti et al., 2016; Tricarico et al., 2016; Martínez and Andriantsitohaina, 2017). Extracellular vesicles deriving from cell protrusions, including primary cilia, appear as a new type of EVs that are associated with specific ciliary markers (Figure 2).
FIGURE 2. Markers and candidate markers of ciliary extracellular vesicles. With the increasing interest in ciliary EVs, the identification of specific markers is needed. Although ciliary EVs are mostly large EVs (ectosomes), classical markers of large EVs are not found in ciliary EVs, except for annexins and phosphatidylserine. The cross comparison of proteomic data from the primary cilium and ciliary EVs allowed the identification of several potential markers, in addition to those already described in the literature. References: 1Ishikawa et al., (2012), 2Dubreuil et al., (2007), 3Yoder et al., (2002), 4Pazour et al., (2002), 5Hogan et al., (2009), 6Nager et al., (2017), 7Phua et al., (2017), 8Mohieldin et al., (2021b), 9Volz et al., (2021), 10Mick et al., (2015), 11Théry et al., (2018)
While the number of studies performed on ciliary EVs is still limited, approaches have been developed to specifically identify ciliary markers. For instance, the comparative proteomic study of EVs isolated from ciliated wild-type (WT) and non-ciliated IFT88 knockout (KO) mouse endothelial cells underscored the different composition of ciliary and cytoplasmic EVs and identified specific markers of ciliary EVs (Mohieldin et al., 2021b). In this study, prevailing large EV markers such as actinin-1 and 4, MVP, and eEF2 (Mathivanan et al., 2010; Kowal et al., 2016; Théry et al., 2018) were not found in ciliary EVs (Mohieldin et al., 2021b). However, NADPH cytochrome P450 reductase (POR) was detected at the tips of primary cilia and enriched in ciliary EVs (Mohieldin et al., 2021b). POR participates in the peroxidation of membrane phospholipids, resulting in plasma membrane rupture in the context of cell death (Zou et al., 2020), but additional studies are needed to define the contribution of POR at the site of EV release within the primary cilium. Furthermore, the extent to which POR can be used as a universal marker of ciliary EVs remains to be established. In addition to POR, Prominin 1 (PROM1, also known as CD133) is a protein associated with different types of protrusions (e.g., filopodia, microvilli, primary cilium, and photoreceptor-specific outer segments) (Jászai et al., 2020) that is also found enriched in EVs derived from primary cilia and microvilli (Marzesco et al., 2005; Dubreuil et al., 2007). Similarly, Polycystin 1 (PC1) and Polycystin 2 (PC2) are two proteins that form a calcium-permeable ion channel in the membrane of the primary cilium, and are associated with a subpopulation of EVs found in urine (Pazour et al., 2002; Yoder et al., 2002; Hogan et al., 2009). The ADP ribosylation factor-like GTPase ARL13b (Nachury and Mick, 2019), a well-known marker of the primary cilium (Nager et al., 2017; Phua et al., 2017) and photoreceptors (Dilan et al., 2019), is used to label ciliary EVs (Table 1). Other compounds present at the ciliary membrane (e.g., GPCRs, INPP5E) are found concentrated in ciliary EVs following their release from the cilium tip (Nager et al., 2017; Phua et al., 2017; Nachury and Mick, 2019). Cross comparison of proteomics data performed on primary cilia organelles with those of ciliary EVs in mammals generated a list of potential markers (Ishikawa et al., 2012; Mick et al., 2015; Mohieldin et al., 2021; Volz et al., 2021), including Keratin-19, Nup155, Septin-10 and CD151. Among families of proteins and phospholipids (e.g., annexins and phosphatidylserine) that are used as EV markers (Rousseau et al., 2015; Théry et al., 2018; Rogers et al., 2020), annexin A8 has also been identified in ciliary EVs (Mohieldin et al., 2021b).
Apart from their protein composition, large and small EVs deriving from the cell cytosolic compartment are also enriched in other bioactive molecules, including small non-coding RNAs (sncRNAs) and lipids (Choi et al., 2013; Meldolesi, 2018; Volz et al., 2021) that participate in intercellular communication. While limited information is available regarding the molecular cargo transported by ciliary EVs and its fate, Volz et al. (2021) found an impaired sorting of bioactive molecules between subpopulations of EVs following ciliary dysfunction, pointing to the role of ciliary EVs in the modulation of cellular signaling.
The expanding field of research on EVs has entailed their characterization, the definition of a consensual nomenclature (Théry et al., 2018), and their functional analyses in distinct model systems and organisms (Yáñez-Mó et al., 2015). As carriers of the patho-physiological molecular signature of their cell of origin, EVs detected in all bodily fluids constitute attractive targets for non-invasive diagnosis of human pathologies (Kosaka et al., 2019). The growing interest in EVs derived from cellular protrusions, particularly ciliary EVs, opens the door to new fundamental questions: to what extent do ciliary EVs share common protein and lipid markers, cargo packaging mechanisms, features, and functions with cytosol derived EVs? Do they participate in the control of recipient cell functions through cellular crosstalk, as described in invertebrates and unicellular organisms? Or do they assist exclusively in the removal of bioactive compounds from the donor cell to control its function? Cilia are ubiquitous organelles important to the homeostatic control of biological systems, and their dysfunction causes ciliopathies. In addition, the primary cilium acts as a cellular brake upon cell division, and growing evidence points to its role in cancer initiation and progression (Izawa et al., 2015; Gerhardt et al., 2016; Eguether and Hahne, 2018). With these considerations in mind, additional investigations are needed to determine if the composition and number of ciliary EVs detected in bodily fluids can indicate the patho-physiological status of the cells, and if ciliary EVs could serve as new targets for the non-invasive diagnosis of cilia-related pathologies.
LV and CB wrote the manuscript. LV conceived the figures.
This work has been supported by a NSERC grant to CB and CRDSI- Fondation du CHU de Quebec fellowships to LV.
The authors declare that the research was conducted in the absence of any commercial or financial relationships that could be construed as a potential conflict of interest.
All claims expressed in this article are solely those of the authors and do not necessarily represent those of their affiliated organizations, or those of the publisher, the editors and the reviewers. Any product that may be evaluated in this article, or claim that may be made by its manufacturer, is not guaranteed or endorsed by the publisher.
Akella, J. S., Carter, S. P., Nguyen, K., Tsiropoulou, S., Moran, A. L., Silva, M., et al. (2020). Ciliary Rab28 and the BBSome negatively regulate extracellular vesicle shedding. eLife 9, e50580. doi:10.7554/eLife.50580
Anvarian, Z., Mykytyn, K., Mukhopadhyay, S., Pedersen, L. B., and Christensen, S. T. (2019). Cellular signalling by primary cilia in development, organ function and disease. Nat. Rev. Nephrol. 15, 199–219. doi:10.1038/s41581-019-0116-9
Bergman, K., Goodenough, U. W., Goodenough, D. A., Jawitz, J., and Martin, H. (1975). Gametic differentiation in Chlamydomonas reinhardtii. II. Flagellar membranes and the agglutination reaction. J. Cell Biol. 67, 606–622. doi:10.1083/jcb.67.3.606
Bertiaux, E., and Bastin, P. (2020). Dealing with several flagella in the same cell. Cell. Microbiol. 22, e13162. doi:10.1111/cmi.13162
Bloodgood, R. A., Woodward, M. P., and Salomonsky, N. L. (1986). Redistribution and shedding of flagellar membrane glycoproteins visualized using an anti-carbohydrate monoclonal antibody and concanavalin A. J. Cell Biol. 102, 1797–1812. doi:10.1083/jcb.102.5.1797
Brooks, E. R., and Wallingford, J. B. (2014). Multiciliated cells: a review. Curr. Biol. 24, R973–R982. doi:10.1016/j.cub.2014.08.047
Brücker, L., Kretschmer, V., and May-Simera, H. L. (2020). The entangled relationship between cilia and actin. Int. J. Biochem. Cell Biol. 129, 105877. doi:10.1016/j.biocel.2020.105877
Cao, M., Ning, J., Hernandez-Lara, C. I., Belzile, O., Wang, Q., Dutcher, S. K., et al. (2015). Uni-directional ciliary membrane protein trafficking by a cytoplasmic retrograde IFT motor and ciliary ectosome shedding. eLife 4, e05242. doi:10.7554/eLife.05242
Carter, S. P., and Blacque, O. E. (2019). Membrane retrieval, recycling and release pathways that organise and sculpt the ciliary membrane. Curr. Opin. Cell Biol. 59, 133–139. doi:10.1016/j.ceb.2019.04.007
Chacon-Heszele, M. F., Choi, S. Y., Zuo, X., Baek, J., Ward, C., Lipschutz, J. H., et al. (2014). The exocyst and regulatory GTPases in urinary exosomes. Physiol. Rep. 2, e12116. doi:10.14814/phy2.12116
Chávez, M., Ena, S., Van Sande, J., de Kerchove d’Exaerde, A., Schurmans, S., Schiffmann, S. N., et al. (2015). Modulation of ciliary phosphoinositide content regulates trafficking and sonic Hedgehog signaling output. Dev. Cell 34, 338–350. doi:10.1016/j.devcel.2015.06.016
Choi, D.-S., Kim, D.-K., Kim, Y.-K., and Gho, Y. S. (2013). Proteomics, transcriptomics and lipidomics of exosomes and ectosomes. PROTEOMICS 13, 1554–1571. doi:10.1002/pmic.201200329
Cocucci, E., Racchetti, G., and Meldolesi, J. (2009). Shedding microvesicles: artefacts no more. Trends Cell Biol. 19, 43–51. doi:10.1016/j.tcb.2008.11.003
Dentler, W. (2013). A role for the membrane in regulating Chlamydomonas flagellar length. PLOS ONE 8, e53366. doi:10.1371/journal.pone.0053366
Dilan, T. L., Moye, A. R., Salido, E. M., Saravanan, T., Kolandaivelu, S., Goldberg, A. F. X., et al. (2019). ARL13B, a Joubert Syndrome-Associated Protein, Is Critical for Retinogenesis and Elaboration of Mouse Photoreceptor Outer Segments. J. Neurosci. 39, 1347–1364. doi:10.1523/JNEUROSCI.1761-18.2018
Dubreuil, V., Marzesco, A.-M., Corbeil, D., Huttner, W. B., and Wilsch-Bräuninger, M. (2007). Midbody and primary cilium of neural progenitors release extracellular membrane particles enriched in the stem cell marker prominin-1. J. Cell Biol. 176, 483–495. doi:10.1083/jcb.200608137
Eguether, T., and Hahne, M. (2018). Mixed signals from the cell’s antennae: primary cilia in cancer. EMBO Rep. 19, e46589. doi:10.15252/embr.201846589
Fisch, C., and Dupuis-Williams, P. (2011). Ultrastructure of cilia and flagella – back to the future. Biol. Cell 103, 249–270. doi:10.1042/BC20100139
Garcia, G., Raleigh, D. R., and Reiter, J. F. (2018). How the ciliary membrane is organized inside-out to communicate outside-in. Curr. Biol. 28, R421–R434. doi:10.1016/j.cub.2018.03.010
Garcia-Gonzalo, F. R., and Reiter, J. F. (2012). Scoring a backstage pass: mechanisms of ciliogenesis and ciliary access. J. Cell Biol. 197, 697–709. doi:10.1083/jcb.201111146
Garcia-Gonzalo, F. R., Phua, S. C., Roberson, E. C., Garcia, G., Abedin, M., Schurmans, S., et al. (2015). Phosphoinositides regulate ciliary protein trafficking to modulate Hedgehog signaling. Dev. Cell 34, 400–409. doi:10.1016/j.devcel.2015.08.001
Gerhardt, C., Leu, T., Lier, J. M., and Rüther, U. (2016). The cilia-regulated proteasome and its role in the development of ciliopathies and cancer. Cilia 5, 14. doi:10.1186/s13630-016-0035-3
Haraszti, R. A., Didiot, M.-C., Sapp, E., Leszyk, J., Shaffer, S. A., Rockwell, H. E., et al. (2016). High-resolution proteomic and lipidomic analysis of exosomes and microvesicles from different cell sources. J. Extracell. Vesicles 5, 32570. doi:10.3402/jev.v5.32570
Hoang-Minh, L. B., Dutra-Clarke, M., Breunig, J., and Sarkisian, M. (2018). Glioma cell proliferation is enhanced in the presence of tumor-derived cilia vesicles. Cilia 7, 6. doi:10.1186/s13630-018-0060-5
Hogan, M. C., Manganelli, L., Woollard, J. R., Masyuk, A. I., Masyuk, T. V., Tammachote, R., et al. (2009). Characterization of PKD protein-positive exosome-like vesicles. J. Am. Soc. Nephrol. 20, 278–288. doi:10.1681/ASN.2008060564
Huang, F., Sirinakis, G., Allgeyer, E. S., Schroeder, L. K., Duim, W. C., Kromann, E. B., et al. (2016). Ultra-high resolution 3D imaging of whole cells. Cell 166, 1028–1040. doi:10.1016/j.cell.2016.06.016
Ishikawa, H., Thompson, J., Yates, J. R., and Marshall, W. F. (2012). Proteomic analysis of mammalian primary cilia. Curr. Biol. 22, 414–419. doi:10.1016/j.cub.2012.01.031
Izawa, I., Goto, H., Kasahara, K., and Inagaki, M. (2015). Current topics of functional links between primary cilia and cell cycle. Cilia 4, 12. doi:10.1186/s13630-015-0021-1
Jacoby, M., Cox, J. J., Gayral, S., Hampshire, D. J., Ayub, M., Blockmans, M., et al. (2009). INPP5E mutations cause primary cilium signaling defects, ciliary instability and ciliopathies in human and mouse. Nat. Genet. 41, 1027–1031. doi:10.1038/ng.427
Jászai, J., Thamm, K., Karbanová, J., Janich, P., Fargeas, C. A., Huttner, W. B., et al. (2020). Prominins control ciliary length throughout the animal kingdom: New lessons from human prominin-1 and zebrafish prominin-3. J. Biol. Chem. 295, 6007–6022. doi:10.1074/jbc.RA119.011253
Jordan, M. A., and Pigino, G. (2021). The structural basis of intraflagellar transport at a glance. J. Cell Sci. 134, jcs247163. doi:10.1242/jcs.247163
Kiesel, P., Viar, G. A., Tsoy, N., Maraspini, R., Gorilak, P., Varga, V., et al. (2020). The molecular structure of mammalian primary cilia revealed by cryo-electron tomography. Nat. Struct. Mol. Biol. 27, 1115–1124. doi:10.1038/s41594-020-0507-4
Kohli, P., Höhne, M., Jüngst, C., Bertsch, S., Ebert, L. K., Schauss, A. C., et al. (2017). The ciliary membrane-associated proteome reveals actin-binding proteins as key components of cilia. EMBO Rep. 18, 1521–1535. doi:10.15252/embr.201643846
Kosaka, N., Kogure, A., Yamamoto, T., Urabe, F., Usuba, W., Prieto-Vila, M., et al. (2019). Exploiting the message from cancer: the diagnostic value of extracellular vesicles for clinical applications. Exp. Mol. Med. 51, 31–39. doi:10.1038/s12276-019-0219-1
Kowal, J., Arras, G., Colombo, M., Jouve, M., Morath, J. P., Primdal-Bengtson, B., et al. (2016). Proteomic comparison defines novel markers to characterize heterogeneous populations of extracellular vesicle subtypes. Proc. Natl. Acad. Sci. U. S. A. 113, E968–E977. doi:10.1073/pnas.1521230113
Li, S., Fernandez, J.-J., Marshall, W. F., and Agard, D. A. (2012). Three-dimensional structure of basal body triplet revealed by electron cryo-tomography. EMBO J. 31, 552–562. doi:10.1038/emboj.2011.460
Long, H., Zhang, F., Xu, N., Liu, G., Diener, D. R., Rosenbaum, J. L., et al. (2016). Comparative analysis of ciliary membranes and ectosomes. Curr. Biol. 26, 3327–3335. doi:10.1016/j.cub.2016.09.055
Luxmi, R., and King, S. M. (2022). Cilia-derived vesicles: an ancient route for intercellular communication. Semin. Cell Dev. Biol. doi:10.1016/j.semcdb.2022.03.014
Maguire, J. E., Silva, M., Nguyen, K. C. Q., Hellen, E., Kern, A. D., Hall, D. H., et al. (2015). Myristoylated CIL-7 regulates ciliary extracellular vesicle biogenesis. Mol. Biol. Cell 26, 2823–2832. doi:10.1091/mbc.E15-01-0009
Martínez, M. C., and Andriantsitohaina, R. (2017). Extracellular vesicles in metabolic syndrome. Circ. Res. 120, 1674–1686. doi:10.1161/CIRCRESAHA.117.309419
Marzesco, A.-M., Janich, P., Wilsch-Bräuninger, M., Dubreuil, V., Langenfeld, K., Corbeil, D., et al. (2005). Release of extracellular membrane particles carrying the stem cell marker prominin-1 (CD133) from neural progenitors and other epithelial cells. J. Cell Sci. 118, 2849–2858. doi:10.1242/jcs.02439
Masyuk, A. I., Huang, B. Q., Ward, C. J., Gradilone, S. A., Banales, J. M., Masyuk, T. V., et al. (2010). Biliary exosomes influence cholangiocyte regulatory mechanisms and proliferation through interaction with primary cilia. Am. J. Physiol. Gastrointest. Liver Physiol. 299, G990–G999. doi:10.1152/ajpgi.00093.2010
Mathivanan, S., Ji, H., and Simpson, R. J. (2010). Exosomes: Extracellular organelles important in intercellular communication. J. Proteomics 73, 1907–1920. doi:10.1016/j.jprot.2010.06.006
Meldolesi, J. (2018). Exosomes and ectosomes in intercellular communication. Curr. Biol. 28, R435–R444. doi:10.1016/j.cub.2018.01.059
Mick, D. U., Rodrigues, R. B., Leib, R. D., Adams, C. M., Chien, A. S., Gygi, S. P., et al. (2015). Proteomics of primary cilia by proximity labeling. Dev. Cell 35, 497–512. doi:10.1016/j.devcel.2015.10.015
Miki, K., and Clapham, D. E. (2013). Rheotaxis guides mammalian sperm. Curr. Biol. 23, 443–452. doi:10.1016/j.cub.2013.02.007
Mohieldin, A. M., Pala, R., Sherpa, R. T., Alanazi, M., Alanazi, A., Shamloo, K., et al. (2020). Proteomic identification reveals the role of ciliary extracellular-like vesicle in cardiovascular function. Adv. Sci. 7, 1903140. doi:10.1002/advs.201903140
Mohieldin, A. M., Alachkar, A., Yates, J., and Nauli, S. M. (2021a). Novel biomarkers of ciliary extracellular vesicles interact with ciliopathy and Alzheimer’s associated proteins. Commun. Integr. Biol. 14, 264–269. doi:10.1080/19420889.2021.2017099
Mohieldin, A. M., Pala, R., Beuttler, R., Moresco, J. J., Yates, J. R., Nauli, S. M., et al. (2021b). Ciliary extracellular vesicles are distinct from the cytosolic extracellular vesicles. J. Extracell. Vesicles 10, e12086. doi:10.1002/jev2.12086
Nachury, M. V., and Mick, D. U. (2019). Establishing and regulating the composition of cilia for signal transduction. Nat. Rev. Mol. Cell Biol. 20, 389–405. doi:10.1038/s41580-019-0116-4
Nager, A. R., Goldstein, J. S., Herranz-Pérez, V., Portran, D., Ye, F., Garcia-Verdugo, J. M., et al. (2017). An actin Network dispatches ciliary GPCRs into extracellular vesicles to modulate signaling. Cell 168, 252–263.e14. doi:10.1016/j.cell.2016.11.036
Nakamura, K., Noguchi, T., Takahara, M., Omori, Y., Furukawa, T., Katoh, Y., et al. (2020). Anterograde trafficking of ciliary MAP kinase–like ICK/CILK1 by the intraflagellar transport machinery is required for intraciliary retrograde protein trafficking. J. Biol. Chem. 295, 13363–13376. doi:10.1074/jbc.RA120.014142
O’Hagan, R., Silva, M., Nguyen, K. C. Q., Zhang, W., Bellotti, S., Ramadan, Y. H., et al. (2017). Glutamylation regulates transport, specializes function, and sculpts the structure of cilia. Curr. Biol. 27, 3430–3441.e6. doi:10.1016/j.cub.2017.09.066
Pala, R., Alomari, N., and Nauli, S. M. (2017). Primary cilium-dependent signaling mechanisms. Int. J. Mol. Sci. 18, 2272. doi:10.3390/ijms18112272
Pampliega, O., Orhon, I., Patel, B., Sridhar, S., Díaz-Carretero, A., Beau, I., et al. (2013). Functional interaction between autophagy and ciliogenesis. Nature 502, 194–200. doi:10.1038/nature12639
Pazour, G. J., San Agustin, J. T., Follit, J. A., Rosenbaum, J. L., and Witman, G. B. (2002). Polycystin-2 localizes to kidney cilia and the ciliary level is elevated in orpk mice with polycystic kidney disease. Curr. Biol. 12, R378–R380. doi:10.1016/S0960-9822(02)00877-1
Phua, S. C., Chiba, S., Suzuki, M., Su, E., Roberson, E. C., Pusapati, G. V., et al. (2017). Dynamic remodeling of membrane composition drives cell cycle through primary cilia excision. Cell 168, 264–279.e15. doi:10.1016/j.cell.2016.12.032
Phua, S. C., Nihongaki, Y., and Inoue, T. (2018). Autonomy declared by primary cilia through compartmentalization of membrane phosphoinositides. Curr. Opin. Cell Biol. 50, 72–78. doi:10.1016/j.ceb.2018.01.008
Pigino, G. (2021). Intraflagellar transport. Curr. Biol. 31, R530–R536. doi:10.1016/j.cub.2021.03.081
Plotnikova, O. V., Seo, S., Cottle, D. L., Conduit, S., Hakim, S., Dyson, J. M., et al. (2015). INPP5E interacts with AURKA, linking phosphoinositide signaling to primary cilium stability. J. Cell Sci. 128, 364–372. doi:10.1242/jcs.161323
Pugacheva, E. N., Jablonski, S. A., Hartman, T. R., Henske, E. P., and Golemis, E. A. (2007). HEF1-dependent Aurora A activation induces disassembly of the primary cilium. Cell 129, 1351–1363. doi:10.1016/j.cell.2007.04.035
Razzauti, A., and Laurent, P. (2021). Ectocytosis prevents accumulation of ciliary cargo in C. elegans sensory neurons. eLife 10, e67670. doi:10.7554/eLife.67670
Rilla, K. (2021). Diverse plasma membrane protrusions act as platforms for extracellular vesicle shedding. J. Extracell. Vesicles 10, e12148. doi:10.1002/jev2.12148
Rogers, M. A., Buffolo, F., Schlotter, F., Atkins, S. K., Lee, L. H., Halu, A., et al. (2020). Annexin A1–dependent tethering promotes extracellular vesicle aggregation revealed with single–extracellular vesicle analysis. Sci. Adv. 6, eabb1244. doi:10.1126/sciadv.abb1244
Rousseau, M., Belleannee, C., Duchez, A.-C., Cloutier, N., Levesque, T., Jacques, F., et al. (2015). Detection and quantification of microparticles from different cellular lineages using flow cytometry. Evaluation of the impact of secreted phospholipase A2 on microparticle assessment. PLoS One 10, e0116812. doi:10.1371/journal.pone.0116812
Salinas, R. Y., Pearring, J. N., Ding, J.-D., Spencer, W. J., Hao, Y., Arshavsky, V. Y., et al. (2017). Photoreceptor discs form through peripherin-dependent suppression of ciliary ectosome release. J. Cell Biol. 216, 1489–1499. doi:10.1083/jcb.201608081
Satir, P., Pedersen, L. B., and Christensen, S. T. (2010). The primary cilium at a glance. J. Cell Sci. 123, 499–503. doi:10.1242/jcs.050377
Schorey, J. S., Cheng, Y., Singh, P. P., and Smith, V. L. (2015). Exosomes and other extracellular vesicles in host–pathogen interactions. EMBO Rep. 16, 24–43. doi:10.15252/embr.201439363
Silflow, C. D., and Lefebvre, P. A. (2001). Assembly and motility of eukaryotic cilia and flagella. Lessons from Chlamydomonas reinhardtii. Plant Physiol. 127, 1500–1507. doi:10.1104/pp.010807
Silva, M., Morsci, N., Nguyen, K. C. Q., Rizvi, A., Rongo, C., Hall, D. H., et al. (2017). Cell-specific α-tubulin isotype regulates ciliary microtubule ultrastructure, intraflagellar transport, and extracellular vesicle biology. Curr. Biol. 27, 968–980. doi:10.1016/j.cub.2017.02.039
Singer, D., Thamm, K., Zhuang, H., Karbanová, J., Gao, Y., Walker, J. V., et al. (2019). Prominin‐1 controls stem cell activation by orchestrating ciliary dynamics. EMBO J. 38, e99845. doi:10.15252/embj.201899845
Singla, V., and Reiter, J. F. (2006). The primary cilium as the cell’s antenna: Signaling at a sensory organelle. Science 313, 629–633. doi:10.1126/science.1124534
Smith, C. E. L., Lake, A. V. R., and Johnson, C. A. (2020). Primary cilia, ciliogenesis and the actin cytoskeleton: A little less resorption, A little more actin please. Front. Cell Dev. Biol. 8, 622822. doi:10.3389/fcell.2020.622822
Spasic, M., and Jacobs, C. R. (2017). Primary cilia: Cell and molecular mechanosensors directing whole tissue function. Semin. Cell Dev. Biol. 71, 42–52. doi:10.1016/j.semcdb.2017.08.036
Spassky, N., and Meunier, A. (2017). The development and functions of multiciliated epithelia. Nat. Rev. Mol. Cell Biol. 18, 423–436. doi:10.1038/nrm.2017.21
Stokman, M. F., Bijnsdorp, I. V., Schelfhorst, T., Pham, T. V., Piersma, S. R., Knol, J. C., et al. (2019). Changes in the urinary extracellular vesicle proteome are associated with nephronophthisis-related ciliopathies. J. Proteomics 192, 27–36. doi:10.1016/j.jprot.2018.07.008
Sun, S., Fisher, R. L., Bowser, S. S., Pentecost, B. T., and Sui, H. (2019). Three-dimensional architecture of epithelial primary cilia. Proc. Natl. Acad. Sci. U. S. A. 116, 9370–9379. doi:10.1073/pnas.1821064116
Szempruch, A. J., Dennison, L., Kieft, R., Harrington, J. M., and Hajduk, S. L. (2016a). Sending a message: extracellular vesicles of pathogenic protozoan parasites. Nat. Rev. Microbiol. 14, 669–675. doi:10.1038/nrmicro.2016.110
Szempruch, A. J., Sykes, S. E., Kieft, R., Dennison, L., Becker, A. C., Gartrell, A., et al. (2016b). Extracellular vesicles from trypanosoma brucei mediate virulence factor transfer and cause host anemia. Cell 164, 246–257. doi:10.1016/j.cell.2015.11.051
Takao, D., and Verhey, K. J. (2016). Gated entry into the ciliary compartment. Cell. Mol. Life Sci. 73, 119–127. doi:10.1007/s00018-015-2058-0
Tarique, I., Liu, Y., Bai, X., Haseeb, A., Yang, P., Huang, Y., et al. (2019). Characterization of extracellular vesicles from cilia and epithelial cells of ductuli efferentes in a turtle (pelodiscus sinensis). Animals. 9, 888. doi:10.3390/ani9110888
Théry, C., Witwer, K. W., Aikawa, E., Alcaraz, M. J., Anderson, J. D., Andriantsitohaina, R., et al. (2018). Minimal information for studies of extracellular vesicles 2018 (MISEV2018): a position statement of the international society for extracellular vesicles and update of the MISEV2014 guidelines. J. Extracell. Vesicles 7, 1535750. doi:10.1080/20013078.2018.1535750
Tricarico, C., Clancy, J., and D’Souza-Schorey, C. (2016). Biology and biogenesis of shed microvesicles. Small GTPases 8, 220–232. doi:10.1080/21541248.2016.1215283
Volz, A.-K., Frei, A., Kretschmer, V., de Jesus Domingues, A. M., Ketting, R. F., Ueffing, M., et al. (2021). Bardet-Biedl syndrome proteins modulate the release of bioactive extracellular vesicles. Nat. Commun. 12, 5671. doi:10.1038/s41467-021-25929-1
Wang, J., and Barr, M. M. (2018). Cell-cell communication via ciliary extracellular vesicles: clues from model systems. Essays Biochem. 62, 205–213. doi:10.1042/EBC20170085
Wang, W.-J., Tay, H. G., Soni, R., Perumal, G. S., Goll, M. G., Macaluso, F. P., et al. (2013). CEP162 is an axoneme-recognition protein promoting ciliary transition zone assembly at the cilia base. Nat. Cell Biol. 15, 591–601. doi:10.1038/ncb2739
Wang, J., Silva, M., Haas, L., Morsci, N., Nguyen, K. C. Q., Hall, D. H., et al. (2014). C. elegans ciliated sensory neurons release extracellular vesicles that function in animal communication. Curr. Biol. 24, 519–525. doi:10.1016/j.cub.2014.01.002
Wang, G., Hu, H.-B., Chang, Y., Huang, Y., Song, Z.-Q., Zhou, S.-B., et al. (2019). Rab7 regulates primary cilia disassembly through cilia excision. J. Cell Biol. 218, 4030–4041. doi:10.1083/jcb.201811136
Wang, J., Nikonorova, I. A., Silva, M., Walsh, J. D., Tilton, P. E., Gu, A., et al. (2021a). Sensory cilia act as a specialized venue for regulated extracellular vesicle biogenesis and signaling. Curr. Biol. 31, 3943–3951.e3. doi:10.1016/j.cub.2021.06.040
Wang, W., Jack, B. M., Wang, H. H., Kavanaugh, M. A., Maser, R. L., Tran, P. V., et al. (2021b). Intraflagellar transport proteins as regulators of primary cilia length. Front. Cell Dev. Biol. 9, 661350. doi:10.3389/fcell.2021.661350
Wheway, G., Nazlamova, L., and Hancock, J. T. (2018). Signaling through the primary cilium. Front. Cell Dev. Biol. 6. Available at: https://www.frontiersin.org/article/10.3389/fcell.2018.00008 (Accessed May 25, 2022).
Wood, C. R., and Rosenbaum, J. L. (2015). Ciliary ectosomes: transmissions from the cell’s antenna. Trends Cell Biol. 25, 276–285. doi:10.1016/j.tcb.2014.12.008
Wood, C., Huang, K., Diener, D., and Rosenbaum, J. (2013). The cilium secretes bioactive ectosomes. Curr. Biol. 23, 906–911. doi:10.1016/j.cub.2013.04.019
Woollard, J. R., Punyashtiti, R., Richardson, S., Masyuk, T. V., Whelan, S., Huang, B. Q., et al. (2007). A mouse model of autosomal recessive polycystic kidney disease with biliary duct and proximal tubule dilatation. Kidney Int. 72, 328–336. doi:10.1038/sj.ki.5002294
Yáñez-Mó, M., Siljander, P. R.-M., Andreu, Z., Bedina Zavec, A., Borràs, F. E., Buzas, E. I., et al. (2015). Biological properties of extracellular vesicles and their physiological functions. J. Extracell. Vesicles 4, 27066. doi:10.3402/jev.v4.27066
Ye, F., Nager, A. R., and Nachury, M. V. (2018). BBSome trains remove activated GPCRs from cilia by enabling passage through the transition zone. J. Cell Biol. 217, 1847–1868. doi:10.1083/jcb.201709041
Yoder, B., Hou, X., and Guay-Woodford, L. (2002). The polycystic kidney disease proteins, polycystin-1, polycystin-2, polaris, and cystin, are co-localized in renal cilia. J. Am. Soc. Nephrol. 13, 2508–2516. doi:10.1097/01.ASN.0000029587.47950.25
Young, R. W., and Bok, D. (1969). Participation of the retinal pigment epithelium in the rod outer segment renewal process. J. Cell Biol. 42, 392–403. doi:10.1083/jcb.42.2.392
Yuan, S., Wang, Z., Peng, H., Ward, S. M., Hennig, G. W., Zheng, H., et al. (2021). Oviductal motile cilia are essential for oocyte pickup but dispensable for sperm and embryo transport. Proc. Natl. Acad. Sci. U. S. A. 118, e2102940118. doi:10.1073/pnas.2102940118
Keywords: ciliary extracellular vesicles, primary cilia, intercellular communication, ectosomes, motile cilia, markers, ectocytosis
Citation: Vinay L and Belleannée C (2022) EV duty vehicles: Features and functions of ciliary extracellular vesicles. Front. Genet. 13:916233. doi: 10.3389/fgene.2022.916233
Received: 08 April 2022; Accepted: 08 July 2022;
Published: 19 August 2022.
Edited by:
Carolina Soekmadji, The University of Queensland, AustraliaReviewed by:
Juan Wang, Rutgers, United StatesCopyright © 2022 Vinay and Belleannée. This is an open-access article distributed under the terms of the Creative Commons Attribution License (CC BY). The use, distribution or reproduction in other forums is permitted, provided the original author(s) and the copyright owner(s) are credited and that the original publication in this journal is cited, in accordance with accepted academic practice. No use, distribution or reproduction is permitted which does not comply with these terms.
*Correspondence: Clémence Belleannée, Y2xlbWVuY2UuYmVsbGVhbm5lZUBjcmNodWRlcXVlYmVjLnVsYXZhbC5jYQ==
Disclaimer: All claims expressed in this article are solely those of the authors and do not necessarily represent those of their affiliated organizations, or those of the publisher, the editors and the reviewers. Any product that may be evaluated in this article or claim that may be made by its manufacturer is not guaranteed or endorsed by the publisher.
Research integrity at Frontiers
Learn more about the work of our research integrity team to safeguard the quality of each article we publish.