- 1Zhejiang Provincial Key Laboratory of Plant Evolutionary Ecology and Conservation, Taizhou University, Taizhou, China
- 2Yuhuan Municipal Bureau of Natural Resources and Planning, Yuhuan, China
- 3Marine Academy of Zhejiang Province, Hangzhou, China
Background: Kandelia obovata, a dominant mangrove species, is widely distributed in tropical and subtropical areas. Low temperature is the major abiotic stress that seriously limits the survival and growth of mangroves. WRKY transcription factors (TFs) play vital roles in responses to biotic and abiotic stresses. However, genome-wide analysis of WRKY genes in K. obovata and their responses to chilling stress have not been reported.
Methods: Bioinformatic analysis was used to identify and characterize the K. obovata WRKY (KoWRKY) gene family, RNA-seq and qRT–PCR analyses were employed to screen KoWRKYs that respond to chilling stress.
Results: Sixty-four KoWRKYs were identified and they were unevenly distributed across all 18 K. obovata chromosomes. Many orthologous WRKY gene pairs were identified between Arabidopsis thaliana and K. obovata, showing high synteny between the two genomes. Segmental duplication events were found to be the major force driving the expansion for the KoWRKY gene family. Most of the KoWRKY genes contained several kinds of hormone- and stress-responsive cis-elements in their promoter. KoWRKY proteins belonged to three groups (I, II, III) according to their conserved WRKY domains and zinc-finger structure. Expression patterns derived from the RNA-seq and qRT–PCR analyses revealed that 9 KoWRKYs were significantly upregulated during chilling acclimation in the leaves. KEGG pathway enrichment analysis showed that the target genes of KoWRKYs were significantly involved in 11 pathways, and coexpression network analysis showed that 315 coexpressed pairs (KoWRKYs and mRNAs) were positively correlated.
Conclusion: Sixty-four KoWRKYs from the K. obovata genome were identified, 9 of which exhibited chilling stress-induced expression patterns. These genes represent candidates for future functional analysis of KoWRKYs involved in chilling stress related signaling pathways in K. obovata. Our results provide a basis for further analysis of KoWRKY genes to determine their functions and molecular mechanisms in K. obovata in response to chilling stress.
Introduction
Cold stress, including both chilling stress (0–15°C) and freezing stress (<0°C), is a significant abiotic stress to plants (Jiang et al., 2021; Wu et al., 2021). Plants from tropical and subtropical regions usually show sensitivity to cold stress and are even vulnerable to chilling stress due to the inability for cold acclimation (Wu et al., 2019). Mangroves are important components of coastal ecosystems in many tropical and subtropical regions of the world and play key roles in these ecosystems through various ecological, social, and economic functions (Li et al., 2021). However, mangroves are fragile ecosystems that are often threatened by flooding, high salinity, anaerobic soils, and extreme climate events (Giri et al., 2011). Among all of these abiotic stresses, cold stress is considered a vital environmental factor limiting the growth and geographical distribution of mangrove plants (Fei et al., 2015). For example, white mangrove (Laguncularia racemosa) trees in a northern region of Tampa Bay, Florida, were exposed to freezing temperatures (−2°C) for 8 h in January 2003, and the leaves of these trees noticeably withered as a result of this freezing (Ellis et al., 2006). In southern China, a chilling temperatures occurred in early 2008, and a large number of mangrove plants withered and even died (Chen et al., 2017). Research involving remote sensing technology showed that cold spells affected northeastern Asia in January 2021, confirming that the canopies of mangrove stands had been stressed by low temperatures (Peereman et al., 2021).
Kandelia obovata, a species in the mangrove family Rhizophoraceae, has been reclassified as a new species that was previously recognized as Kandelia candel in regions of China and Japan (Sheue et al., 2003). As a dominant species of mangrove forest in Eastern Asia, K. obovata provides critical ecosystem services to the human beings, including coastal protection, habitat provision, biodiversity maintenance, water purification, and carbon sequestration (Wu et al., 2020; Wei et al., 2022). It is well known that the latitudinal distribution of mangroves is mainly limited by temperature. K. obovata has relatively high tolerance against low-temperature stress and is a major mangrove species distributed along the southeastern coastline of China (Chen et al., 2017). In China, K. obovata is naturally distributed in southern Fuding (27° 20′ N), Fujian Province (Wang et al., 2010). However, climate change has led to the expansion of various mangrove species to higher latitudes (Osland et al., 2013), and K. obovata was successfully introduced to Zhoushan (29° 30′ N), Zhejiang Province, in 2016. A successive freezing spell (minimum −3.2°C) occurred in the winter of 2010 on Ximen Island (28° 25′ N), Yueqing, Zhejiang Province, China, and the leaves of K. obovata had become brown and wilted, and a high mortality of 2-year-old seedlings was reported (Zheng et al., 2016).
In recent years, many studies have provided valuable insight into the physiological mechanisms (Zheng et al., 2016; Liu et al., 2018; Wang et al., 2019) and molecular mechanisms (Peng et al., 2015; Fei et al., 2021) underlying the response of K. obovata to low temperature. In our previous study, we cloned the AP2/EREBP transcription factor (TF) KcCBF3 and demonstrated that it might participate in the adaptation of K. obovata to low-temperature stress (Du and Li, 2019). TFs can bind to cis-elements or interact with other regulatory factors to regulate the expression of downstream defense-related genes (Manna et al., 2021). Increasing numbers of reports show that a number of different TFs, including AP2/EREBP, bHLH, MYB, bZIP, NAC, WRKY, and other TFs, play important regulatory roles in plant stress responses (Gahlaut et al., 2016; Meraj et al., 2020).
WRKY proteins (WRKYs), which constitute the largest family of TFs among all TFs, can identify and bind to W-box [(C/T)TGAC(C/T)] cis-elements in the promoter of their target genes; WRKYs are approximately 60 amino acids in length and contain one or two highly conserved heptapeptide WRKYGQK motifs and a typical zinc-finger C2H2 (CX4-5Cx22-23HXH) or C2HC (CX7CX23HXC) domain at their C-terminus (Eulgem et al., 2000). In addition to the conserved sequences of the WRKYGQK motifs, some variants, including WRKYGEK, WRKYGRK, WKKYGQK, and WKRYGQK, can also be found in plants (Jiang et al., 2017). Based on the number of WRKY domains and the features of their zinc finger motifs, WRKYs are usually divided into three groups (I, II and III). The group I proteins have two WRKY domains, while the group II proteins, which have only one WRKY domain and a C2H2 zinc-finger motif, can be further subdivided into five subgroups (i.e., IIa–e) based on their phylogenetic relations. Proteins from group III have one WRKY domain and a C2HC motif (Eulgem et al., 2000).
By functioning synergistically with different genes and other TFs, WRKYs play important roles in plants in the defense against pathogens (bacteria, fungi, and viruses) (Jiang et al., 2017). Studies have also indicated that WRKYs are involved in regulating gene expression under abiotic stresses, such as cold, heat, drought and salinity stresses. In addition to roles in response to abiotic and biotic stresses, WRKYs also participate in various plant processes involving germination, growth, development, senescence and metabolic pathways (Rushton et al., 2010). Many studies have demonstrated that members of the WRKY family play essential regulatory roles in the cold stress response. Ten WRKY genes were shown to be strongly induced in Solanum lycopersicum during cold stress, and 12 WRKYs were significantly downregulated (Chen et al., 2015). Fifty-nine WRKY genes have been identified in the Vitis vinifera genome, and more than ten of them showed stress-induced expression patterns in response to cold (Wang et al., 2014). Kim et al. (2016) reported that the rice WRKY TF OsWRKY71 has a positive function in cold tolerance by regulating downstream target genes. In contrast, the TF WRKY34 negatively mediates the cold sensitivity of mature pollen of Arabidopsis thaliana (Zou et al., 2010).
Despite the important role of WRKYs in different species under abiotic stress, there is no information on these proteins in K. obovata. Chilling stress represents one of the major environmental stresses that severely affects the distribution and survival of K. obovata by negatively affecting the hypocotyl, impairing the photosynthetic apparatus, and stunting growth. Therefore, screening for chilling tolerance genes and improving the cold tolerance of K. obovata is particularly important against the background of the current increase in occasional extreme weather events. The aim of the current research was to identify KoWRKY genes in the K. obovata genome, to classify their expression patterns and to reveal the putative targets and their underlying regulatory biological processes under chilling stress. Our results might provide insight into the molecular importance of KoWRKYs under chilling stress.
Materials and Methods
Plant Material and Treatments
Healthy and mature propagules of K. obovata were collected from Yueqing Bay, Yuhuan city, Zhejiang Province, China (28°13′N, 121°10′ E). The propagules were cultivated in a growth chamber (25°C temperature, 75% humidity, 14 h light/10 h darkness photoperiod) in plastic pots containing sand and watered with 1/2-strength Hoagland’s nutrient solution weekly. At the six-leaf stage, the seedlings were cultivated under chilling stress (4 °C) for 0, 1, 3, and 12 h. All the treatments included three seedlings. The leaves were collected, frozen immediately in liquid nitrogen and then stored at −80 °C.
Identification of KoWRKY Genes From K. obovata Genome
The complete genome sequence files of K. obovata were downloaded from the Genome Sequence Archive (GSA) webpage (https://bigd.big.ac.cn/gsa/browse/CRA002395). Information on the WRKY domain hidden Markov model (HMM) profile numbered PF03106 was retrieved from the Pfam protein family database (http://pfam.sanger.ac.uk/), and the A. thaliana WRKY (AtWRKY) sequence information was obtained from The Arabidopsis Information Resource (TAIR) (www.arabidopsis.org). The candidate WRKY protein sequences were identified by comprehensive research using HMMER (E-value cutoff <1E-5) and BLAST analyses (72 AtWRKYs were used as queries) of the K. obovata whole-genome and protein databases. These KoWRKY sequences were identified by checking the complete WRKY conserved domain with SMART (http://smart.embl-heidelberg.de/) and InterPro (http://www.ebi.ac.uk/interpro/) and were confirmed manually by checking all sequences containing WRKYGQK motifs and a typical C2H2 (CX4-5Cx22-23HXH) or C2HC (CX7CX23HXC) zinc-finger domain. The sequences of the confirmed KoWRKYs were input into the ExPASy online tool website (http://web.expasy.org/protparam/) to calculate the physicochemical properties of the proteins, including the molecular weight (MW) and isoelectric point (pI). The subcellular localization of the KoWRKYs was determined by ProtComp 9.0 (http://linux1.softberry.com/berry.phtml?topic=protcomppl&group=programs&subgroup=proloc).
Analysis of Chromosomal Localization, Gene Structure and Cis-elements in the Promoter Regions of KoWRKYs
The chromosomal positions of all the KoWRKY genes were determined from the genome annotation file. The physical positions of the KoWRKY genes on the chromosome were mapped using MapChart (Voorrips, 2002). The exon/intron structure of the KoWRKY genes was determined by comparing their predicted coding sequence (CDS) with genomic sequences using the gene structure display server (http://gsds.cbi.pku.edu.cn/). The upstream 2 kb sequences from the transcription initiation site of all the KoWRKY genes were extracted, and the cis-elements in these regions were identified using the PlantCARE database (http://bioinformatics.psb.ugent.be/webtools/plantcare/html/).
Analysis of Gene Duplication, Selective Pressure, and Collinearity of KoWRKYs
The criteria used for identifying duplicate genes were as follows: the similarity of the two aligned sequences was greater than 75%, and the length of the shorter aligned sequence covered more than 75% of that of the longer sequence. Two or more adjacent duplicates on the same chromosome within 100 kb were defined as tandem duplications, while duplicates across different chromosomes or at a distance greater than 100 kb on the same chromosome were considered segmental duplications (Kan et al., 2021). The synonymous rate (Ks) and nonsynonymous rate (Ka) of the identified KoWRKY gene pairs were calculated using KaKs Calculator version 2 (Wang Y et al., 2012). The approximate date [million years ago (Mya])] of each duplication event was estimated using the mean Ks values with the formula T = Ks/2λ, in which the mean synonymous substitution rate (λ) was 6.1 × 10–9 (Lynch and Conery, 2000; Zhu et al., 2014). OrthoFinder with default parameters was employed to identify homologous genes between two different genomes (K. obovata and A. thaliana), and a synteny graph was constructed with TBtools according to the identified homologous gene pairs (Chen et al., 2020a).
Analysis of Motif, Conserved Domain and Phylogenesis of KoWRKYs
Multiple EM for Motif Elicitation (MEME) was used to analyze the KoWRKYs and to identify 15 possible conserved motifs (http://meme.nbcr.net/meme/intro.html). The parameters were as follows: the repetitive time was “any”, the maximum motif number was 15, and the motif width was between 5 and 50 residues. The MEME results were subsequently displayed with TBtools software (Chen et al., 2020a). Multiple sequence alignment of the KoWRKY domains was performed using MAFFT version 7 (Katoh and Standley, 2013). Based on the alignment of the WRKY domains of the KoWRKYs and AtWRKYs, a phylogenetic tree was constructed with FastTree version 2 via the generalized time-reversible (GTR) model in conjunction with the Shimodaira–Hasegawa (SH) test (Price et al., 2010). All the identified KoWRKYs were divided into different groups according to the classification of AtWRKY sequences.
KoWRKY Target Gene Prediction and Kyoto Encyclopedia of Genes and Genomes Analysis
The 2-kb DNA sequences upstream of the ATG start codon of all genes assembled from the K. obovata genome were used to identify WRKY TF-binding sites. The potential KoWRKY target genes that were predicted with PlantRegMap were used for further pathway enrichment analysis with the KEGG database (https://www.kegg.jp/kegg/pathway.html) (Tian et al., 2019). Hypergeometric Fisher’s exact test (p < 0.01) and the Benjamini test [false discovery rate (FDR])<0.05] were performed to detect statistically significantly enriched KEGG pathways. The R package ggplot2 was used to visualize the top 11 significantly enriched KEGG pathways.
Expression Profiles of KoWRKY Genes in Different Tissues and in Response to Chilling Stress Based on RNA-Seq
Transcriptomic data of K. obovata were obtained from the National Center for Biotechnology Information (NCBI) publicly accessible database (Accession number: PRJNA678025). The expression levels of the KoWRKY genes were analyzed in various tissues, including root, stem, leaf, flower, pistil, stamen, sepal, and fruit tissues. The expression data were transformed into log2 [transcripts per million (TPM)+1] values for differential expression analysis (Zeng et al., 2020). The resulting gene expression profiles were visualized as a heatmap via TBtools software (Chen et al., 2020a).
The expression profiles of the KoWRKY genes that responded to chilling stress were obtained from the NCBI transcriptomic database (Accession number: PRJNA678025). The expression of the KoWRKY genes was visualized as a heatmap using TBtools software (Chen et al., 2020a). For the transcriptome analysis of KoWRKYs in response to chilling stress, thresholds of p < 0.05 and |log2 (fold-change) ≥ 1| were used to define differentially expressed genes (DEGs).
Experimental Validation of KoWRKY Genes Expression Levels via qRT-PCR
Total RNA was extracted using RNASimple Total RNA Kit (Tiangen, Beijing, China) and cDNA was obtained using TIANScript cDNA kit (Tiangen, Beijing, China) according to the manufacturers’ instructions. Following total RNA extraction and cDNA synthesis, quantitative analysis was performed via real-time PCR in conjunction with SuperReal PreMix Plus (SYBR Green, Tiangen, China) on a CFX96 Touch Real-Time PCR Detection System (Bio–Rad, United States) according to the manufacturers’ instructions. The PCR program included an initial denaturation step at 95°C for 30 s, followed by 40 cycles of 95°C for 10 s, 58°C for 10 s, and 72°C for 30 s. The relative transcript levels of the candidate genes were calculated according to the 2−ΔΔCT method (Livak and Schmittgen, 2001). All the data were generated from averages of three independent replicates, and statistical significance was determined by one-way ANOVA followed by Duncan’s multiple range tests. All the primers used are listed in Supplementary Table S1.
Analysis of Coexpression Networks Between KoWRKY Gene and Potential Regulated Gene
Differentially expressed KoWRKY gene was used as analysis object. Pearson correlation coefficient (PCC) between KoWRKY gene and non-KoWRKY gene was calculated to determine the coexpressed gene pair. Only gene pair with |PCC| value greater than 0.95 (p < 0.05) was used to regard as the potential regulated gene. The network was visualized using Cytoscape software (version 3.6.1).
Results
Identification and Characterization of KoWRKY Genes
To identify the WRKY-encoding genes present in the genome of K. obovata, the hidden Markov model (HMM) profile PF03106 from the Pfam database was used as a query for an HMM search against the genome, and a local BLASTP search was performed for which all 72 A. thaliana WRKY protein sequences were used in the query. In total, 64 potentially WRKY-encoding genes were identified and annotated. The 64 genes were named as KoWRKY1-KoWRKY64 according to the order of their gene ID, and the location on the chromosome, length of CDS, and the MW, pI and subcellular localization of their encoded proteins were shown in Table 1. Each chromosome contained KoWRKY members, of which chromosome 12 had the most, with 9 members; chromosome 18 had the fewest members, with only one. The CDS length ranged from 351 (KoWRKY23) to 2,181 bp (KoWRKY35), with an average length of 1,203 bp. The MW ranged from 12.99 kDa (KoWRKY23) to 78.60 kDa (KoWRKY35), with an average of 43.92 kDa. The theoretical pIs varied from 4.46 (KoWRKY33) to 10.26 (KoWRKY41), and the predicted subcellular localization results indicated that the 64 KoWRKYs were located in the nucleus (Table 1).
Chromosomal Localization, Exon/Intron Structure, and Cis-Elements in the Promoters of KoWRKY Genes
To determine the distribution of the KoWRKY genes across the genome, all 64 identified KoWRKY mRNAs/open reading frames (ORFs) were mapped onto their corresponding chromosome via BLAST searches against the released K. obovata genome sequence. As shown in Figure 1, the KoWRKY genes were unevenly distributed across the 18 chromosomes, and the numbers on each chromosome were not related to chromosome length. Chromosome 12 had 9 WRKY genes (the majority), including one member from each of groups I, IIe and III of the KoWRKY gene family and 2 members from groups IIb, IIc, and IId, followed by 5 members on chromosomes 1, 5, 6, and 8; however, chromosome 18 contained only one WRKY gene (KoWRKY 64, in subgroup IIa).
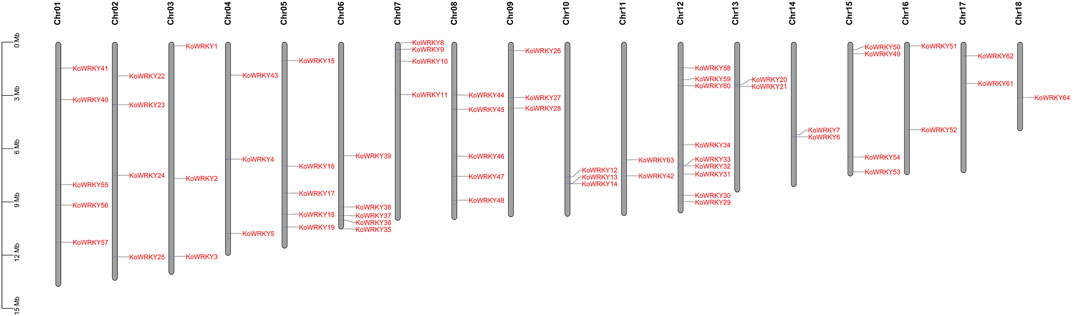
FIGURE 1. Chromosomal distribution of KoWRKY genes. The position of each KoWRKY gene on the chromosomes can be determined by the left scale.
To investigate the structural diversity of the KoWRKY genes, the localization of intron–exon interactions was analyzed. It was found that the number of introns in the KoWRKY genes ranged from 1 (KoWRKY23 and KoWRKY28) to 6 (KoWRKY9) in K. obovata. A total of 34 (53.13%) KoWRKY genes had 2 introns, followed by 8 (12.5%), 11 (17.19%), and 8 (12.5%) genes that contained 3, 4, and 5 introns, respectively (Figure 2). Most KoWRKY genes in group I had 3 to 5 introns, except KoWRKY18 and KoWRKY9, which had 2 and 6 introns, respectively. The number of introns in the KoWRKY genes in group II widely varied, ranging from 1 to 5. However, all 7 KoWRKY genes in group III had 2 introns (Figure 2).
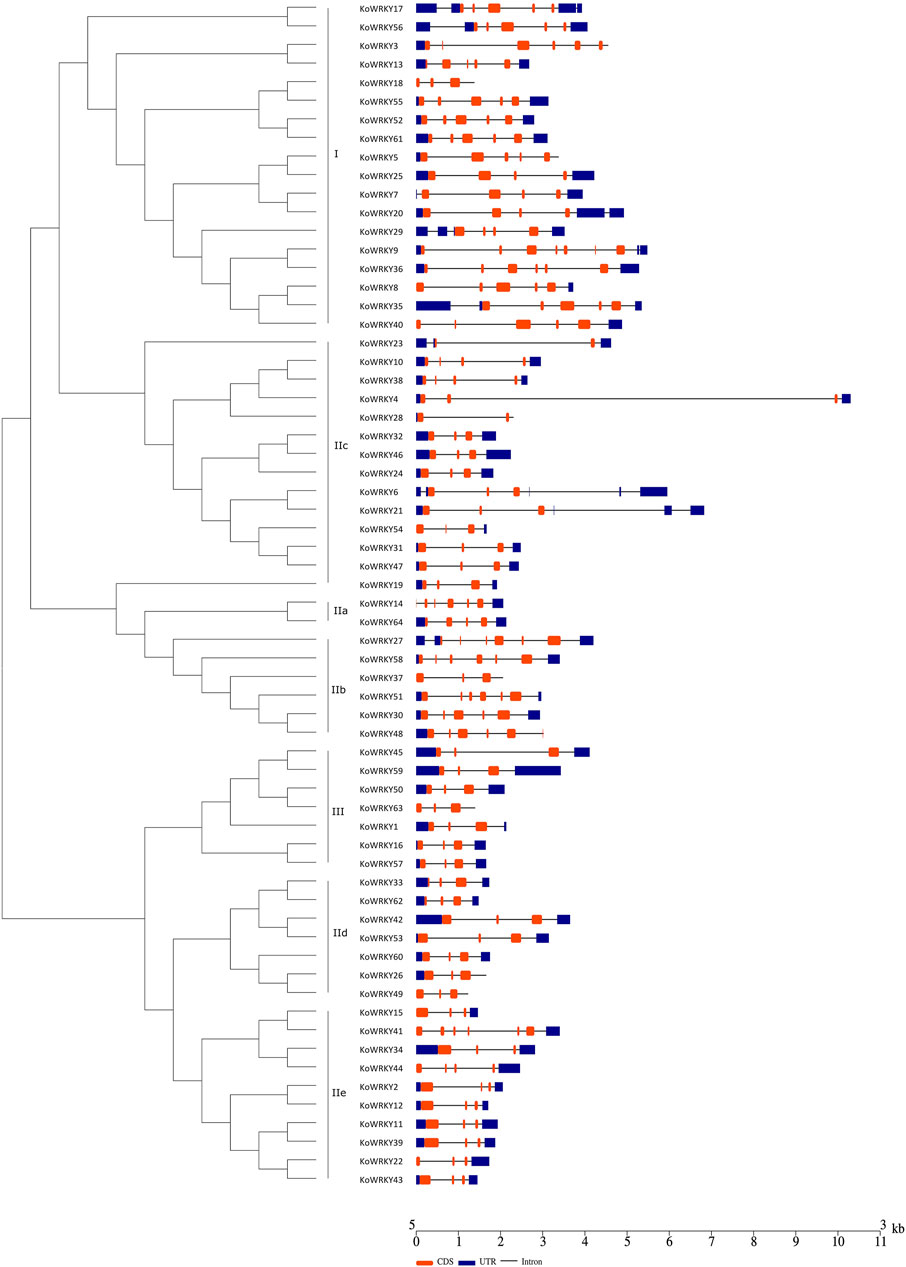
FIGURE 2. Gene structure analysis of KoWRKY genes from Kandelia obovata. Exon–intron structure analyses of the KoWRKY genes were performed via the Gene Structure Display Server (GSDS) online tools. The lengths of the exons and introns of each KoWRKY gene are shown proportional to each other. The introns are represented by black lines, and the exons and UTRs are represented by brown and blue boxes, respectively. A scale of gene length is given at the bottom. CDS, coding DNA sequence; UTR, untranslated region.
To analyze the functional diversification of the WRKY members, the sequences of the 2-kb upstream promoter regions of the KoWRKY genes were retrieved and analyzed. A number of cis-elements, including 9 elements related to plant development and 17 motifs related to stress responses, were analyzed, and the 26 elements are represented in Supplementary Table S2. The cis-elements related to plant growth and development include light-responsive elements (Box4, G-box, Sp1, ACE, and GT1 motifs), meristem-specific activation elements (CCGTCC-boxes), meristem expression-specific elements (CAT-boxes), endosperm expression-specific elements (GCN4 motif), and circadian control-related elements (circadians). Cis-elements related to stress responses include eight hormone-responsive elements (EREs, ABREs, CGTCA motifs, TGACG motifs, GARE motifs, TATC-boxes, P-boxes, TCA elements, and SAREs), low-temperature–responsive elements (LTRs and DRE cores), wound-responsive elements (WUN motifs), auxin response elements (TGAs and AuxRR cores), anaerobic induction elements (AREs), As shown in Supplementary Table S2, each KoWRKY gene contained more than one cis-element in its promoter region, and most KoWRKY genes contained Box4s, G-boxes, EREs, ABREs, TGACG and ARE motifs. Our analysis suggested that KoWRKY genes play an important role during development and stress responses.
Gene Expansion, Selective Pressure and Synteny Analysis of KoWRKY Genes
To elucidate the mechanism underlying KoWRKY gene family expansion in K. obovata, BLASTP and MCScanX were employed to identify gene duplication patterns. The results showed that among the 64 KoWRKY genes, 49 segmentally duplicated genes formed 34 pairs (Supplementary Table S3); however, no tandem duplications were detected for KoWRKY genes, implying that segmental duplication was the major driving force for the expansion of KoWRKY genes.
To examine the selective pressure of KoWRKY genes, the Ka/Ks ratios of the duplicated gene pairs were calculated. Of the 35 paralogous KoWRKY gene pairs, the Ka values ranged from 0.05 to 0.54, the Ks values varied from 0.27 to 3.02, and all duplicated KoWRKYs had a Ka/Ks < 1, ranging from 0.12 to 0.45 (Supplementary Table S3). Estimated by a universal substitution rate of 6.1 × 10–9 mutations per site per year, duplications of KoWRKYs may have occurred at two time points, approximately 22.3–40.7 MYA and 194.6–247.8 MYA (Supplementary Table S3). Previous studies suggested that a Ka/Ks < 1, a Ka/Ks = 1, and a Ka/Ks > 1 indicate purifying selection, neutral evolution, and positive selection, respectively (Nan and Gao, 2019). In this study, none of the Ka/Ks ratios of repeated KoWRKY gene pairs in K. obovata were greater than 1, which indicates that they underwent purifying selection.
To further explore the synteny relationships of KoWRKY genes with A. thaliana, Orthofinder was used to find the orthologous genes and TBtools was employed to construct the comparative synteny map (Figure 3). It showed that orthologous relationships between 50 KoWRKY genes and 32 AtWRKY genes were detected, and 59 orthologous WRKY gene pairs were identified based on these genes and the syntenic loci in K. obovata and A. thaliana chromosomes, suggesting that WRKY genes in both species had a similar origin and evolutionary process.
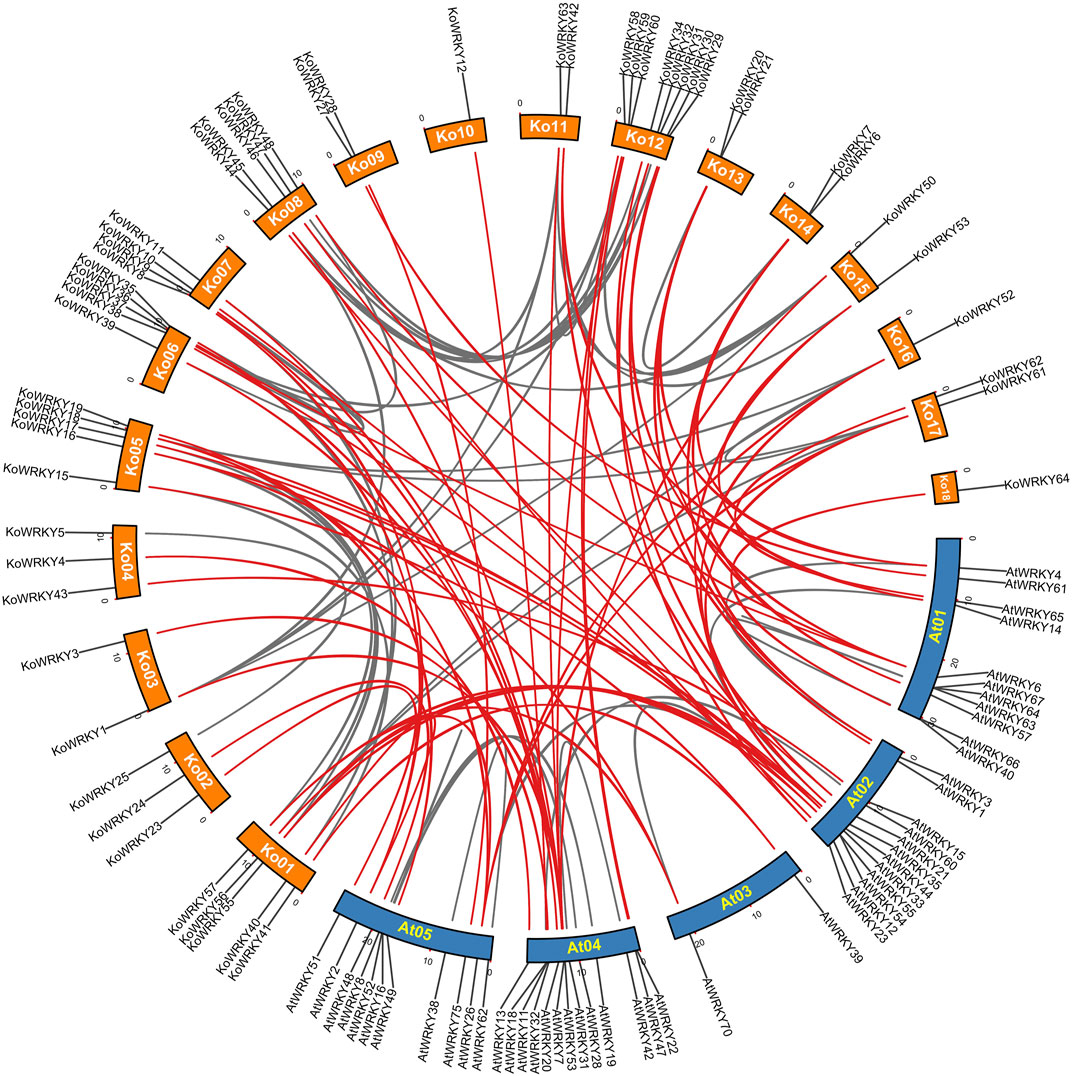
FIGURE 3. Synteny analysis between WRKY genes of Kandelia obovata and Arabidopsis thaliana, as performed by MCScanX. The chromosome numbers of K. obovata are Ko01–18, and the chromosome numbers of A. thaliana are At01–05. The red curves indicate synteny relationships between K. obovata and A. thaliana WRKY genes, and the gray curves indicate synteny between WRKY genes within K. obovata or A. thaliana.
Conserved Motifs, Sequence Alignment, and Phylogenetic Analysis of KoWRKY Proteins
To gain insight into the functional regions of the KoWRKYs, the MEME program was used to identify the conserved motifs among the 64 KoWRKYs. A total of 15 conserved motifs were identified, namely, motifs 1–15 (Figure 4). These 15 conserved motifs are indicated with colored boxes according to their scale, with sizes ranging from 15 to 49 amino acid residues (Supplementary Figure S1). Among these individual motifs, motifs 1 and 3 were found to encode the conserved WRKY domain, and motifs 2 and 4 were found to encode the conserved zinc-finger structure. In addition to these conserved WRKY and zinc-finger motifs, other conserved motifs (motifs 5–15) were also predicted to be present within the KoWRKYs. Each KoWRKY protein had at least two conserved motifs, with the maximum number being seven, which was the case for several KoWRKYs. The distributions of the conserved motifs varied among the different KoWRKY groups. For example, group I KoWRKYs had seven motifs (motifs 1, 2, 3, 4, 6, 10, and 12), each containing one motif 1 and motif 3 (except KoWRKY18) and one zinc finger (motifs 2 and 4). Motifs 5, 6, 9, 11, 13, and 15 were present only in members of groups IIb, I, IIb, III, IIe and IIc, respectively. Motif 8 was present in members of group III and subgroup IIe, and motif 14 was present in subgroup IIa and IIb members. On the whole, the analysis of KoWRKY motifs showed that the KoWRKY members of every group or subgroup had similar motif compositions that corresponded to the clustering results generated by the phylogenetic tree analysis.
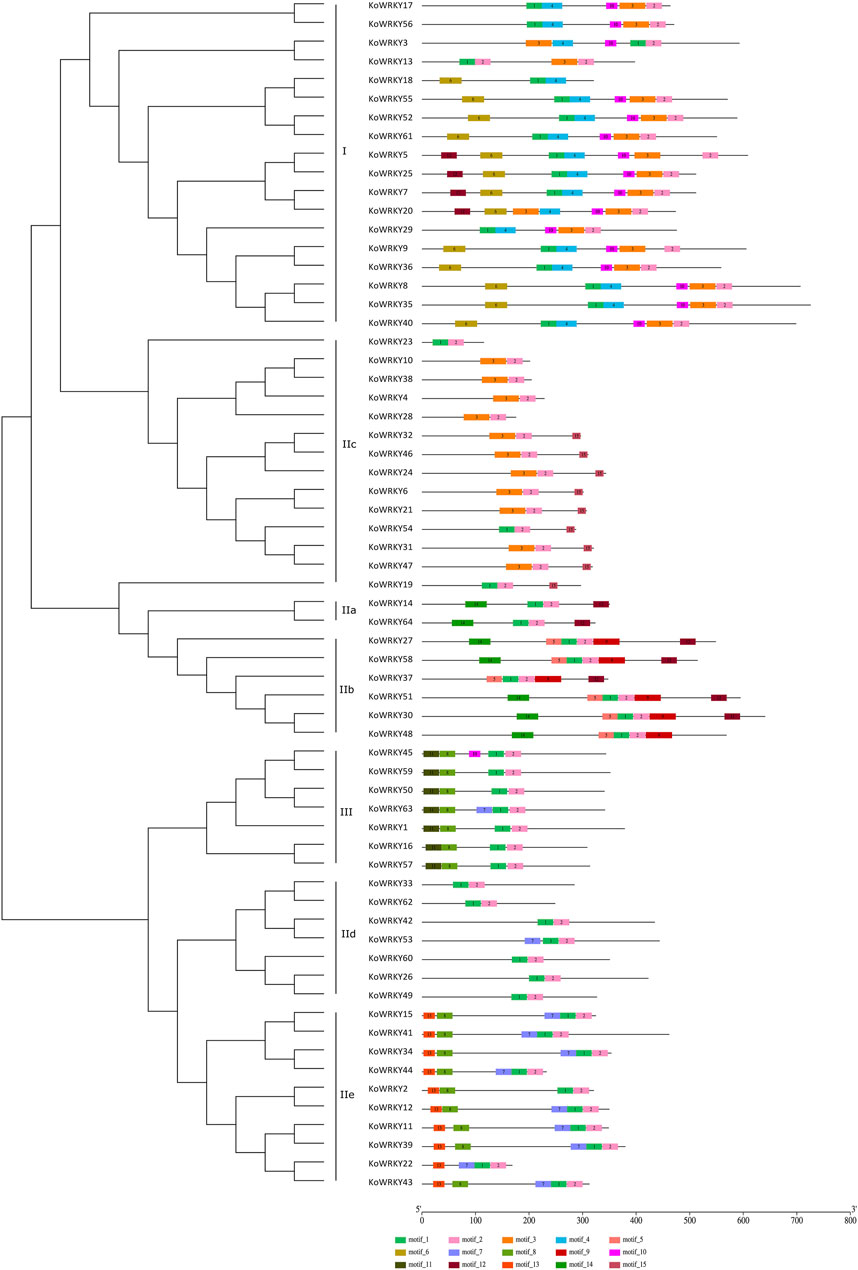
FIGURE 4. Conserved motifs of the KoWRKYs arranged according to their phylogenetic relationships. The ML tree shown was constructed from the amino acid sequences of KoWRKYs via ClustalX and MEGA 5, with 1,000 bootstrap replicates. The conserved motifs in the KoWRKYs were identified using MEME. In total, 15 motifs were identified and are shown in different colors. The motif locations are also indicated.
On the basis of the multiple alignment results for K. obovata WRKY protein sequences, the 64 WRKYs could be categorized into three groups (I, II and III) (Supplementary Figure S2). Most members of group I contained two conserved domains, WRKYGQK and a CX4CX22-23HXH zinc-finger motif in the N-terminal or a CX4CX23HXH motif in the C-terminal region. However, in KoWRKY18, the WRKYGQK sequence was replaced by WRKYGEK in the C-terminal region, and KoWRKY18 lacked a C-terminal WRKY domain. The 39 KoWRKYs in group II had a conserved heptapeptide WRKYGQK sequence (only KoWRKY23 contained a WRKYGEK sequence) and a CX4–5CX23HX1H zinc-finger domain, while 7 KoWRKYs in group III had a conserved WRKYGQK sequence and a CX7CX23HX1C zinc-finger domain.
To investigate the evolutionary relationships between KoWRKYs and WRKYs from A. thaliana, an unrooted maximum-likelihood phylogenetic tree based on multiple alignments of the predicted amino acid sequences of the WRKY domains from K. obovata and A. thaliana was constructed. According to the tree (Figure 5), the KoWRKYs could be classified into three primary groups (groups I, II and III), with 18 KoWRKYs in group I, 39 KoWRKYs in group II, and 7 KoWRKYs in group III. Moreover, the KoWRKYs in group II could be further classified into five subgroups (groups IIa, IIb, IIc, IId and IIe) containing 2, 6, 14, 10, and 7 members, respectively. Notably, among all these groups and subgroups, most KoWRKYs were the members of subgroup IIc, the case of which is similar to AtWRKYs.
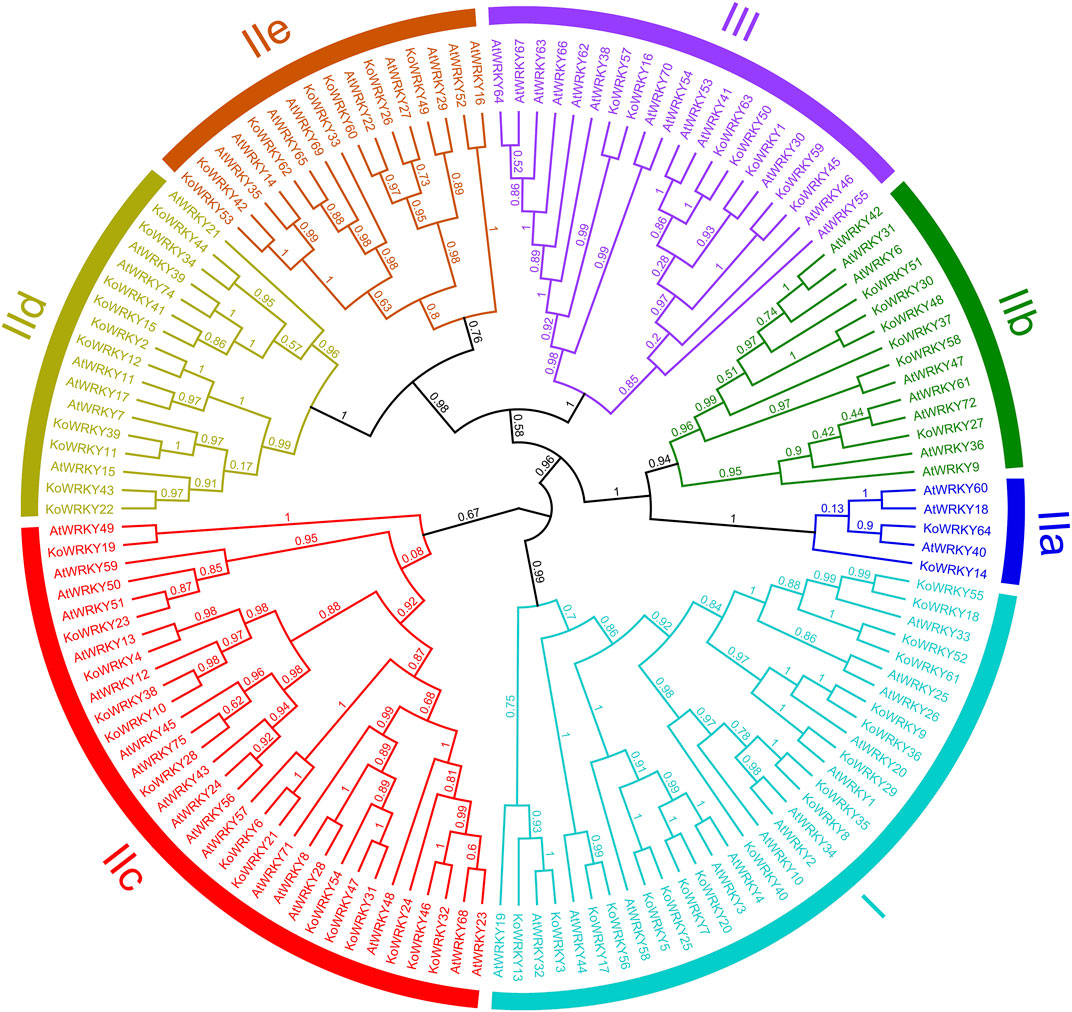
FIGURE 5. Phylogenetic relationships among WRKYs identified in Kandelia obovata and Arabidopsis thaliana. In total, 64 KoWRKY and 72 AtWRKY protein sequences were used to construct the phylogenetic tree using MEGA 7 and maximum likelihood (ML) method analysis (1,000 replicates). The K. obovata and A. thaliana genes are indicated at the ends of the branches. Subgroups I, IIa, IIb, IIc, IId, IIe, and III were named according to the results for A. thaliana. The colored regions indicate different subfamilies.
Potential Target Genes of KoWRKY Proteins Were Enriched Into 11 Significant Pathways by KEGG Analysis
In order to lay the foundation for the functional analysis of KoWRKY proteins, their potential target genes were subjected to KEGG enrichment analysis. KEGG pathway enrichment analysis showed that the potential target genes of KoWRKY proteins were significantly (p < 0.05) involved in 11 pathways (Figure 6). The three pathways of metabolism, lipid metabolism, and glycosyltransferases had the most enriched genes, which were 637, 113, and 86, respectively. In particular, 73 target genes were involved in environmental adaptation pathways (Supplementary Table S4), which may play an important role in how KoWRKY genes regulate the adaptation process of K. obovata to the environment.
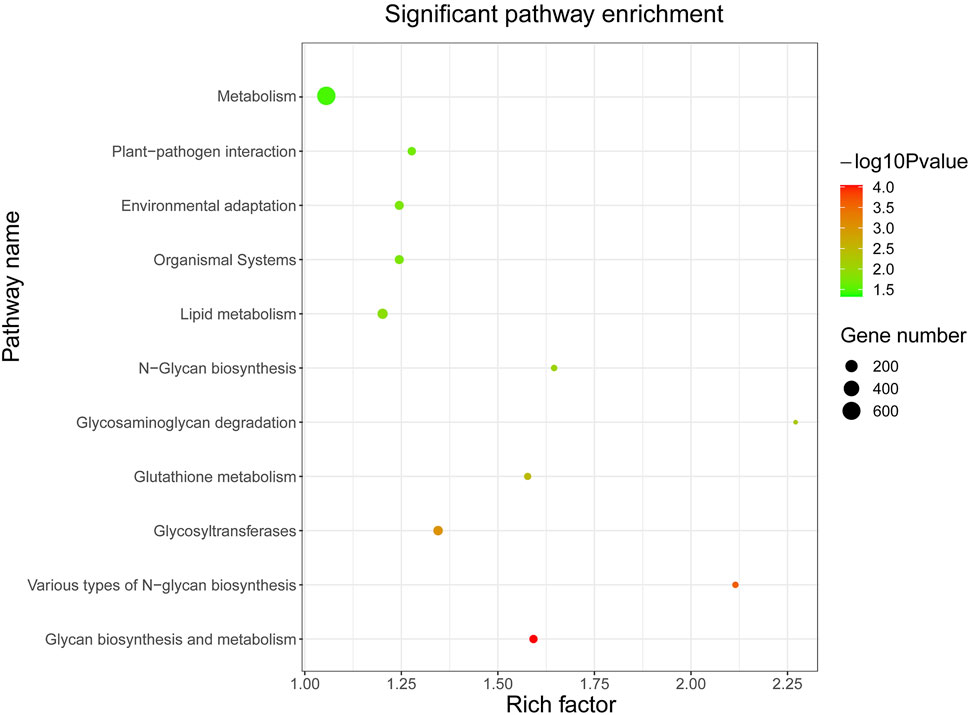
FIGURE 6. KEGG pathway enrichment analysis of predicted putative target genes of KoWRKYs in K. obovata. The coloring of the q-values represents the significance of the matched gene ratio; the circle size represents the target gene number.
Expression Profiles of KoWRKY Genes in Different Tissues and in Response to Chilling Stress
The expression profiles of all 64 KoWRKY genes were investigated using a standard transcriptome analysis procedure based on publicly available transcriptomic data of different tissues of K. obovata, including root, stem, leaf, flower, pistil, stamen, sepal, and fruit tissues. Among the 64 KoWRKYs, 50 were expressed in samples above (TPM > 0). Some KoWRKY genes showed preferential expression across all the tissues tested. KoWRKY genes, especially KoWRKY33, KoWRKY39, KoWRKY45, KoWRKY50, KoWRKY52, KoWRKY56, KoWRKY57, KoWRKY59 and KoWRKY63, were most highly expressed in the roots. Several members, such as KoWRKY2, KoWRKY6, KoWRKY9, KoWRKY21, KoWRKY39, KoWRKY50, KoWRKY54, KoWRKY60, and KoWRKY62 presented higher expression levels in the fruits than in the other tissues. Among all the tissues, the fewest KoWRKY members were expressed the most in the sepals. In addition, KoWRKY19 and KoWRKY37 were barely expressed in any of the tissues tested (Figure 7A).
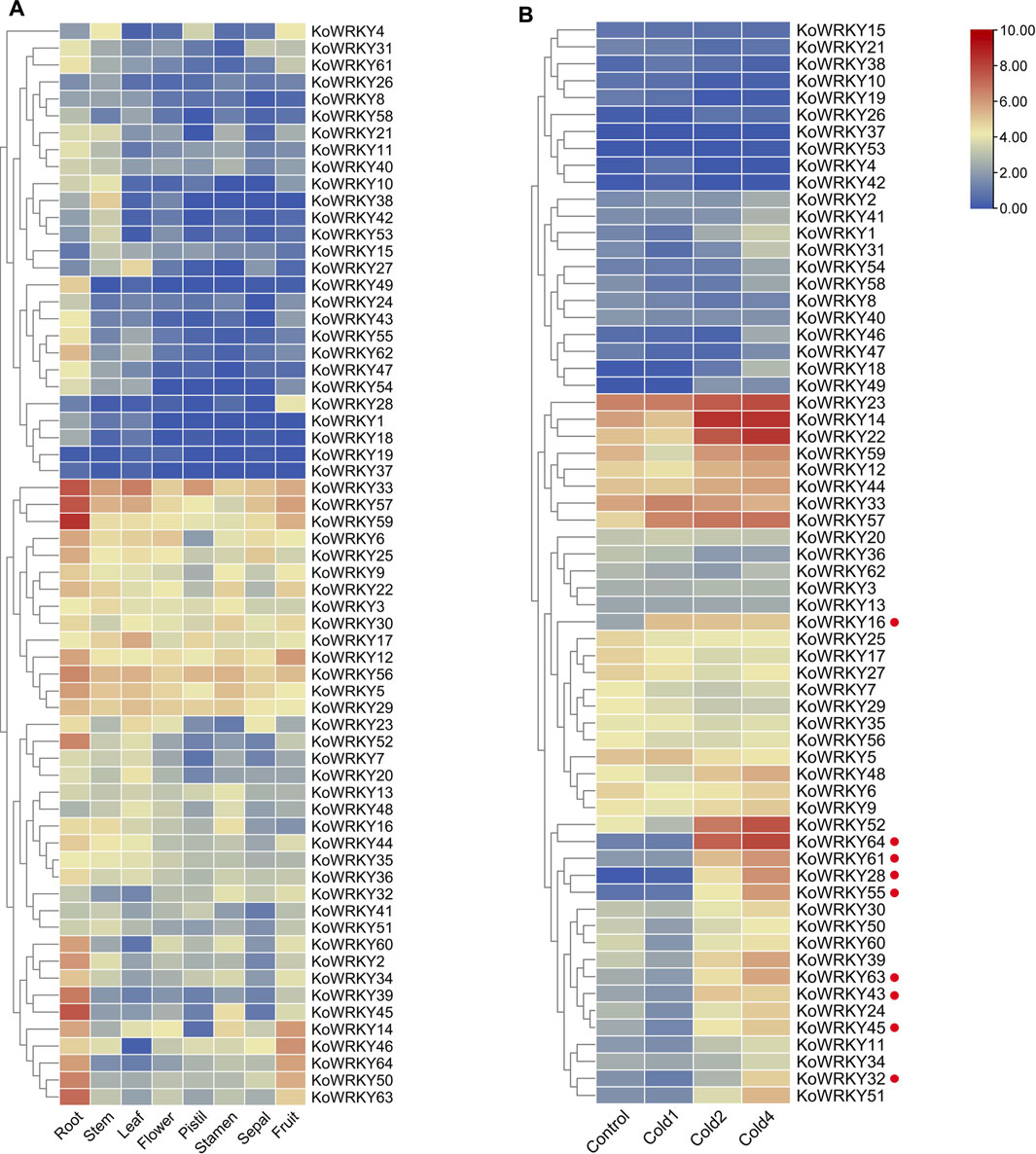
FIGURE 7. Expression of KoWRKY genes in Kandelia obovata. (A) The transcript levels of the KoWRKY genes in 8 tissues of K. obovata were investigated based on publicly available transcriptomic data. The color scale shows increasing expression levels from blue to red. (B) The transcript levels of KoWRKY genes in response to cold stress were investigated based on publicly available transcriptomic data. Cold1, first cold treatment; Cold2, second cold treatment; Cold4, fourth cold treatment. The genes whose expression increased more than two-fold after cold treatment are labeled with red dots.
To investigate the potential functions of KoWRKYs in response to chilling stress, the expression of KoWRKYs after chilling treatment based on publicly available transcriptomic data was analyzed. The results showed that the expression levels of most KoWRKY genes, especially KoWRKY16, KoWRKY28, KoWRKY32, KoWRKY43, KoWRKY45, KoWRKY55, KoWRKY61, KoWRKY63, and KoWRKY64, were upregulated after chilling treatment, the expression of which increased more than two-fold. However, the expression levels of KoWRKY37, KoWRKY42 and KoWRKY53 were not different from those of the control after the first, second and fourth cold treatments (Figure 7B).
To confirm the candidate KoWRKY genes that are important for cold tolerance, 9 DEGs were selected, and their expression levels were quantified via qRT–PCR. As shown in Figure 8, the expression of all the selected KoWRKY genes was upregulated under 4°C. Under low-temperature stress, the expression of KoWRKY16 increased sharply but then decreased, peaking after 1 h, the level of which was significantly higher than that of the control. The expression of KoWRKY43, KoWRKY63 and KoWRKY64 peaked at 3 h after 4°C treatment; however, the expression of KoWRKY28, KoWRKY32, KoWRKY45, KoWRKY55 and KoWRKY61 peaked at 12 h. The largest increase in the expression level (approximately 90-fold) was detected for KoWRKY28 after 12 h of chilling treatment.
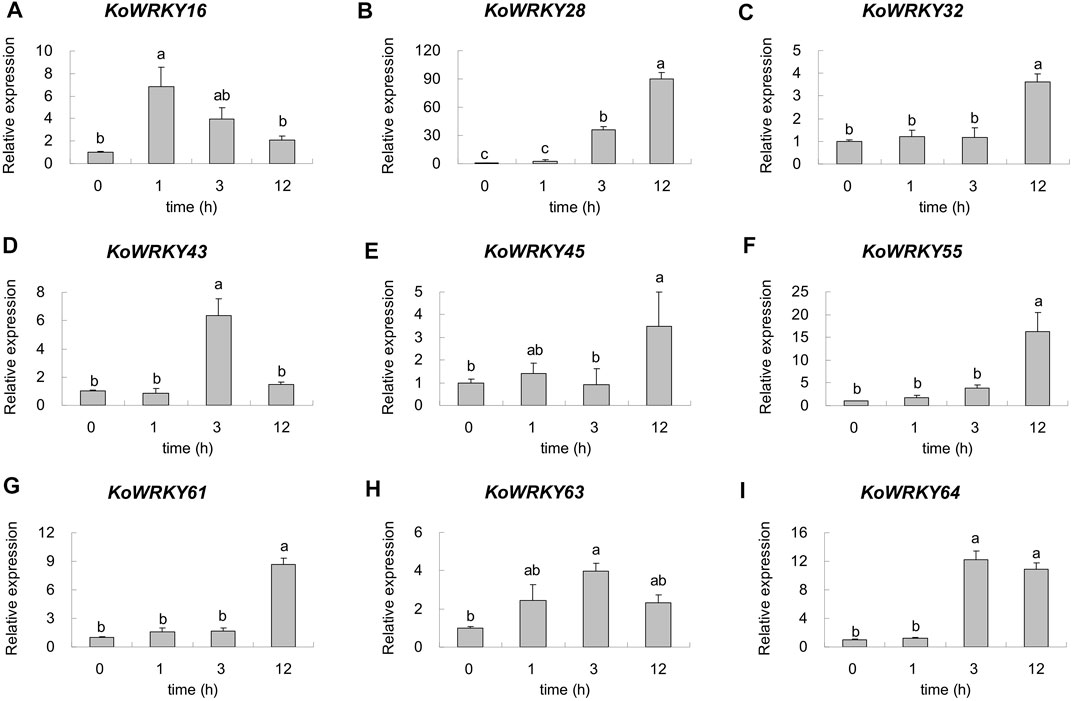
FIGURE 8. Expression profiles of nine selected KoWRKY genes in response to chilling stress. The relative expression levels of nine KoWRKY genes were measured in plants subjected to 4°C for 0, 1, 3, and 12 h. The transcript levels of the selected genes were assessed via qRT–PCR and normalized to 18S rRNA levels. The error bars represent the standard errors. The values with the same letter are not significantly different according to Duncan’s multiple range test (p < 0.05, n = 3). Panels (A–I) represented the expression level of gene KoWRKY16, KoWRKY28, KoWRKY32, KoWRKY43, KoWRKY45, KoWRKY55, KoWRKY61, KoWRKY63, and KoWRKY64, respectively.
315 Significantly Correlated Coexpressed Pairs of KoWRKYs and mRNAs Were Identified
Genome-wide gene expression profiling of KoWRKY16, KoWRKY28, KoWRKY32, KoWRKY43, KoWRKY45, KoWRKY55, KoWRKY61, KoWRKY63, and KoWRKY64 and mRNAs from leaves of plants subjected to chilling stress was conducted to identify genes coexpressed with KoWRKYs. The potential target mRNAs were predicted via Pearson correlation test for the 9 above mentioned KoWRKY genes whose expression increased more than two-fold after chilling treatment. The results showed that 263 significantly expressed mRNAs were correlated (PCC >0.95, p < 0.001) with 9 KoWRKYs, and for all 315 coexpressed pairs, each KoWRKY and mRNA was positively correlated (Figure 9, Supplementary Table S5). Taken together, the results indicated that the KoWRKYs might positively regulate the response of these putative genes in chilling stress.
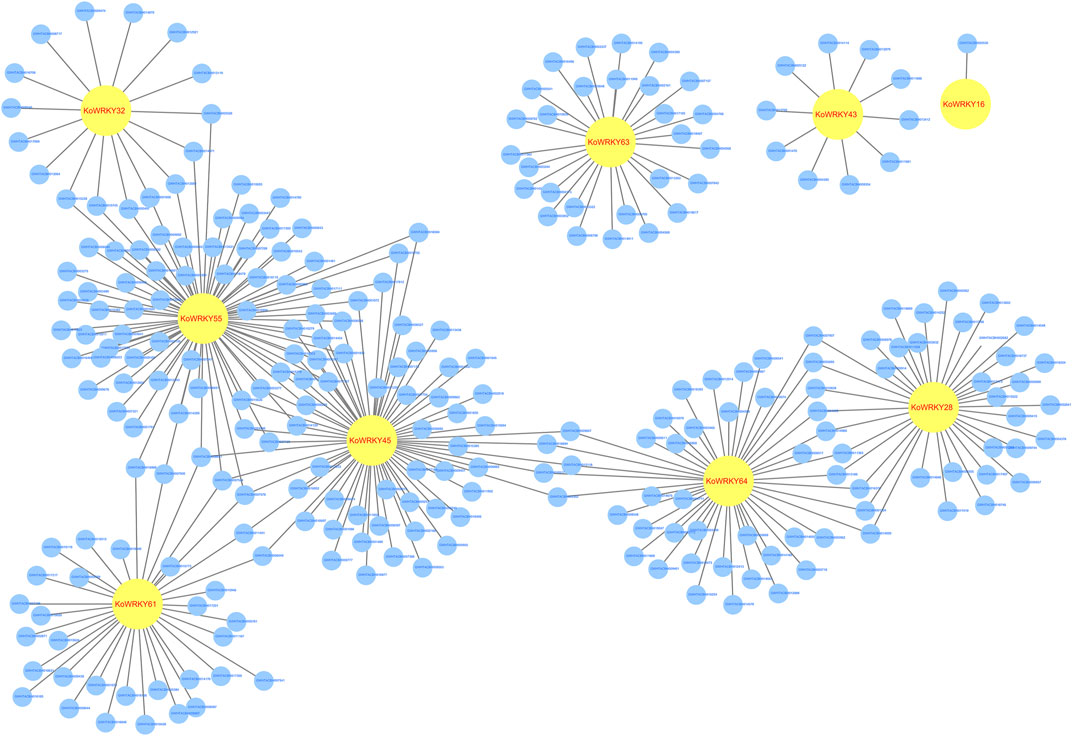
FIGURE 9. Coexpression network of KoWRKY genes with other genes in Kandelia obovata under cold stress. The coexpression network was established between the 9 KoWRKYs and 263 significantly expressed mRNAs whose Spearman correlation coefficients were equal to or greater than 0.95. Within this coexpression network, all 315 pairs were positive. The yellow circles represent KoWRKY genes, while the blue circles represent the coexpressed genes.
Discussion
WRKY gene family members are types of TFs that have long been indicated to regulate multiple physiological processes in plants. Since Ishiguro and Nakamura (1994) cloned the first WRKY gene (SPF1) from sweet potato, it has been cloned from the roots, leaves, inflorescences, seeds, and microstructures of various plant species, such as A. thaliana, Oryza sativa, Zea mays, and Medicago truncatula. With the continuous completion of genome sequencing of different species, an increasing number of WRKY genes have been identified, and their biological functions have also been extensively explored. Specifically, WRKY TFs have been shown to regulate a variety of processes in response to biotic and abiotic stress in plants (Eulgem et al., 2000). However, studies of the WRKY gene family of K. obovata have not yet been reported. In the present study, a total of 64 WRKY gene members in K. obovata were identified via bioinformatics methods to further analyze their structure, function and expression, aiming to provide some reference for follow-up studies on KoWRKY gene function and regulatory mechanisms.
According to the number of conserved WRKY regions and the patterns of zinc-finger motifs, all of the WRKY genes can be classified into three groups: groups I, II, and III (Eulgem et al., 2000). Group I members have two conserved WRKY domains and a C2H2 zinc-finger motif; group II members have only one conserved WRKY domain and the same zinc-finger motif as group I members have; and group III members have one conserved WRKY domain and a C2HC zinc-finger motif (Rushton et al., 2010). In the present study, the KoWRKYs were categorized into three groups based on the conserved domains of the proteins, and the results were consistent with previous findings. Almost all of the KoWRKYs shared the highly conserved WRKYGQK domain; however, variants of the WRKYGEK and WRKYGKK domains could still be found in KoWRKY13 and KoWRKY23, respectively. Such variations in the WRKYGQK conserved motif have also been reported in many other species. For example, six OnWRKYs (OnWRKY18, OnWRKY46, OnWRKY52, OnWRKY55, OnWRKY84, and OnWRKY114) in O. nivara had a WRKYGEK domain instead of the WRKYGQK conserved domain (Xu et al., 2016), and four VvWRKYs (VvWRKY8, VvWRKY13, VvWRKY14, and VvWRKY24) from V. vinifera contained the variant WRKYGKK rather than the WRKYGQK motif (Guo et al., 2014). Previous studies demonstrated that the WRKYGQK domain can bind to the core W-box cis-element motif (C/T)TGAC(C/T) to activate the expression of downstream genes (Eulgem et al., 2000). The variation in or loss of the conserved WRKYGQK domain might affect the specificity of binding to cis-elements (Ciolkowski et al., 2008). For example, the AtWRKY59 protein in A. thaliana could not bind to TTGAC (a W-box) because its WRKYGKK motif was replaced with WRKYGQK (Dong et al., 2003). Furthermore, in Nicotiana tabacum NtWRKY12 with a WRKYGKK domain could bind to WK-box elements rather than W-box motifs (van Verk et al., 2008). Thus, further efforts are needed to experimentally prove the binding specificities of the KoWRKY13 and KoWRKY23 proteins with variants of the WRKYGQK motif.
In addition to having a variant of the highly conserved WRKYGQK domain, some WRKYs may lack conserved motifs. For instance, both AtWRKY10 (group I) in A. thaliana and CsWRKY42 in Cucumis sativus have only one WRKY domain (Wei et al., 2012; Chen et al., 2020b). The same result occurred in this paper, for KoWRKY18 (group I) lost its C-terminal WRKYGQK domain. It was reported that the C-terminal domain of WRKYs (group I) was sufficient for W-box element recognition, whereas the N-terminal WRKY domain alone failed to bind W-box element, but it may increase the overall binding affinity of the protein to DNA by making additional contacts with DNA or by interacting with other proteins (Eulgem et al., 1999; Maeo et al., 2001). Therefore, loss of the C-terminal WRKY domain might influence the recognition and binding of KoWRKY18 to target genes, and further study of the binding specificities and functions of the KoWRKY18 protein might be worthwhile.
It was found that the number of WRKY genes in different species is not positively related to the size of their genome. For instance, A. thaliana genome is only 125 Mb and contains 72 WRKY genes. C. melo and O. sativa ssp. japonica have similar genome sizes (450 and 466 Mb, respectively); however, the former contains 65 WRKY genes, and the latter contains 128 WRKY genes (http://planttfdb.gao-lab.org/family.php?fam=WRKY, 2022.2.14). In the present study, the K. obovata genome size was 180 Mb, but the genome was found to contain 64 members of the WRKY family. Although the number of WRKY genes is nearly the same, the genome size of C. melo is more than 2 times that of K. obovata. Recent studies have proposed that gene duplication is considered to be one of the primary driving forces in the expansion of gene families and genome evolution, and the major duplication patterns are tandem duplication and segmental duplication (Cannon et al., 2004). Tandem duplications have been reported to play major roles in the expansion of the WRKY family in Solanum tuberosum (Shi et al., 2017) and Citrus sinensis (Silva et al., 2017); however, segmental duplications seem to be more common than tandem duplications are in the expansion of the WRKY family, such as in O. rufipogon (Nan et al., 2020), Cicer arietinum (Waqas et al., 2019), and Ananas comosus (Xie et al., 2018). These findings are consistent with research in Camelina sativa (Song et al., 2020). Our results showed that 49 segmental duplication events were present in 64 KoWRKY genes; however, no tandem duplication was detected for any KoWRKY gene. These events revealed that segmental duplication was the major driving force for the expansion of KoWRKY genes. The Ks value is widely used to estimate the evolutionary history of segmental duplication events. It was reported that the Ks distributions for paralogous K. obovata genes exhibited two peaks, one at Ks = 0.38 and the other at Ks = 1.5–1.9 (Hu et al., 2020). These results are consistent with the results of the present study, in which the mean Ks value of the KoWRKY genes in the present paper exhibited two peak values, 0.39 and 2.56, which implied that K. obovata underwent two segmental duplications in recent years.
As important types of TFs, by acting as positive or negative regulators WRKYs, regulate the responses to biotic and abiotic stresses of plant species such as A. thaliana, O. sativa and Glycine max (Rushton et al., 2010). However, there is still no relevant research on WRKY genes in K. obovata. Studies have shown that the tissue-specific expression of WRKY genes exerts strong effects during plant growth and development by regulating the expression of gene involved in growth and differentiation (Li et al., 2014). In the present study, a large number of KoWRKY genes were found to be constitutively expressed in the roots of K. obovata, with many of them, such as KoWRKY27, KoWRKY28, KoWRKY38, KoWRKY39, and KoWRKY49, showing a tissue-specific expression pattern (Figure 7A), implying that KoWRKYs have vital functions in plant development and function differently in different tissues. K. obovata, a dominant mangrove species distributed along the southern coast of China, survives in harsh environments and experiences environmental stresses such as submergence, hypoxia, salinity, and even extremely low temperatures in winter (Fei et al., 2021; Nizam et al., 2022). Hypoxia (which can be caused by submergence or waterlogging) and salinity stresses affect the survival and growth of many plants, some of which have developed multiple strategies to cope with these stressful conditions during their evolution, including morphological changes and scavenging of reactive oxygen species (Tang et al., 2020). However, the most critical mechanism is based on gene regulation involving signaling cascades, in which responses to hypoxia or salinity signals are triggered. Recent studies have also demonstrated that WRKY33- and WRKY12-overexpressing A. thaliana showed enhanced resistance to hypoxia (Tang et al., 2020), and overexpression of VvWRKY30 in A. thaliana increased resistance to salt stress at different stages of growth (Zhu et al., 2019). As such, many KoWRKY genes showed a high expression pattern in the roots, implying that these TF genes play vital roles in the adaptation of those plants to mudflat environments. Further research on KoWRKY function and the regulatory mechanisms should be conducted.
In the present study, RNA-seq revealed that the expression of some KoWRKYs in the leaves of K. obovata changed under chilling stress. Among these KoWRKYs, most were found to be upregulated in response to chilling stress, but some were downregulated. Similar results were also found in Coffea canephora (Dong et al., 2019) and Prunus mume (Bao et al., 2019), indicating that TF KoWRKYs might act as positive or negative regulators. In addition, nine putative candidate KoWRKY genes (KoWRKY16, KoWRKY28, KoWRKY32, KoWRKY43, KoWRKY45, KoWRKY55, KoWRKY61, KoWRKY63, and KoWRKY64) were upregulated more than twofold after low-temperature treatment. The expression profile generated by qRT–PCR in this work showed that the nine candidate KoWRKY genes were upregulated after treatment with 4°C (Figure 8), which in most cases coincides with the expression patterns obtained via RNA-seq. It has been reported that over-expression of OsWRKY71 in rice enhanced the tolerance to chilling stress (Kim et al., 2016); moreover, over-expression of BcWRKY46 has been shown to increase the chilling and freezing tolerance of tobacco (Wang F et al., 2012). Similar functions were also found in the study of genes CsWRKY46 (Zhang et al., 2016) and GmWRKY21 (Zhou et al., 2008). From the phylogenetic tree, it can be seen that KoWRKY64 and OsWRKY71, KoWRKY45 and BcWRKY46 are very closely related (Supplementary Figure S3), which means that KoWRKY64 and KoWRKY45 may play an important role in coping with cold stress. The results suggest that these genes potentially are involved in the chilling resistance of K. obovata. Nonetheless, further experimental analyses should be carried out to elucidate the precise regulatory mechanism through which KoWRKY genes respond to chilling stress.
Conclusion
In conclusion, 64 KoWRKYs were identified in the genome of K. obovata, and they were unevenly distributed across all 18 chromosomes. The evolution, gene structure and cis-elements in the promoter regions of the KoWRKYs were also analyzed. Some KoWRKYs were highly expressed in specific tissues, and 9 KoWRKYs in the leaves were significantly induced in response to chilling stress. These genes represent candidates for future functional analysis of K. obovata in response to low temperature. Our results provide a basis for further analysis of KoWRKY genes to determine their function and elucidate the molecular mechanisms underlying the response of K. obovata to chilling stress.
Data Availability Statement
The original contributions presented in the study are included in the article/Supplementary Materials, further inquiries can be directed to the corresponding author.
Author Contributions
ZD and JL designed the research study. ZD, SY, and XZ performed the experiments. ZD and LX analyzed the data. ZD and JL wrote the manuscript. All authors contributed to editorial changes in the manuscript. All authors read and approved the final manuscript.
Funding
This study was supported by the Public Welfare Project of Science Technology Department of Zhejiang Province (LGN21C160007), the Science and Technology Project of Department of Natural Resources of Zhejiang Province (2021-43), and the Project of Yuhuan Municipal Bureau of Natural Resources and Planning (BY-YHGQCG-2021-04).
Conflict of Interest
The authors declare that the research was conducted in the absence of any commercial or financial relationships that could be construed as a potential conflict of interest.
Publisher’s Note
All claims expressed in this article are solely those of the authors and do not necessarily represent those of their affiliated organizations, or those of the publisher, the editors and the reviewers. Any product that may be evaluated in this article, or claim that may be made by its manufacturer, is not guaranteed or endorsed by the publisher.
Acknowledgments
We are grateful to Mingzhi Li (Bio&Data Biotechnologies Co. Ltd., Guangzhou) for providing technical assistance.
Supplementary Material
The Supplementary Material for this article can be found online at: https://www.frontiersin.org/articles/10.3389/fgene.2022.875316/full#supplementary-material
Supplementary Figure S1 | Details of 15 motifs of KoWRKYs.
Supplementary Figure S2 | Multiple sequence alignment of the WRKY domain from KoWRKYs. Alignment was performed using DNAMAN. The conserved heptapeptide is WRKYGQK and the zinc-finger motif are C2H2 or C2HC. The color shade of the amino acid residues highlighted the homology level: dark blue = 100%, pink ≥75%, and cambridge blue ≥50%.
Supplementary Figure S3 | Phylogenetic relationships among WRKYs identified with other species. In total, 64 KoWRKY, 72 AtWRKY, 1 BcWRKY46, 1 CsWRKY46. 1 GmWRKY21, and 1 OsWRKY71 protein sequences were used to construct the phylogenetic tree using MEGA 7 and maximum likelihood (ML) method analysis (1000 replicates). Subgroups I, IIa, IIb, IIc, IId, IIe, and III were named according to the results for A. thaliana. The colored regions indicate different subfamilies. The Genbank accession no. of BcWRKY46, CsWRKY46. GmWRKY21, and OsWRKY71 were ADM32893, ADU52524, ABC26913 and AAT84158, respectively. The two purple and blue and dots represented the closely related sequences.
References
Bao, F., Ding, A., Cheng, T., Wang, J., and Zhang, Q. (2019). Genome-Wide Analysis of Members of the WRKY Gene Family and Their Cold Stress Response in Prunus Mume. Genes 10, 911. doi:10.3390/genes10110911
Cannon, S. B., Mitra, A., Baumgarten, A., Young, N. D., and May, G. (2004). The Roles of Segmental and Tandem Gene Duplication in the Evolution of Large Gene Families in Arabidopsis thaliana. BMC Plant Biol. 4, 10. doi:10.1186/1471-2229-4-10
Chen, C., Chen, H., Zhang, Y., Thomas, H. R., Frank, M. H., He, Y., et al. (2020a). TBtools: an Integrative Toolkit Developed for Interactive Analyses of Big Biological Data. Mol. Plant 13, 1194–1202. doi:10.1016/j.molp.2020.06.009
Chen, C., Chen, X., Han, J., Lu, W., and Ren, Z. (2020b). Genome-wide Analysis of the WRKY Gene Family in the Cucumber Genome and Transcriptome-wide Identification of WRKY Transcription Factors that Respond to Biotic and Abiotic Stresses. BMC Plant Biol. 20, 443. doi:10.1186/s12870-020-02625-8
Chen, L., Wang, W., Li, Q. Q., Zhang, Y., Yang, S., Osland, M. J., et al. (2017). Mangrove Species' Responses to winter Air Temperature Extremes in China. Ecosphere 8, e01865. doi:10.1002/ecs2.1865
Chen, L., Yang, Y., Liu, C., Zheng, Y., Xu, M., Wu, N., et al. (2015). Characterization of WRKY Transcription Factors in Solanum lycopersicum Reveals Collinearity and Their Expression Patterns under Cold Treatment. Biochem. Biophysical Res. Commun. 464, 962–968. doi:10.1016/j.bbrc.2015.07.085
Ciolkowski, I., Wanke, D., Birkenbihl, R. P., and Somssich, I. E. (2008). Studies on DNA-Binding Selectivity of WRKY Transcription Factors Lend Structural Clues into WRKY-Domain Function. Plant Mol. Biol. 68, 81–92. doi:10.1007/s11103-008-9353-1
Dong, J., Chen, C., and Chen, Z. (2003). Expression Profiles of the Arabidopsis WRKY Gene Superfamily during Plant Defense Response. Plant Mol. Biol. 51, 21–37. doi:10.1023/A:1020780022549
Dong, X., Yang, Y., Zhang, Z., Xiao, Z., Bai, X., Gao, J., et al. (2019). Genome-Wide Identification of WRKY Genes and Their Response to Cold Stress in Coffea Canephora. Forests 10, 335. doi:10.3390/f10040335
Du, Z., and Li, J. (2019). Expression, Purification and Molecular Characterization of a Novel Transcription Factor KcCBF3 from Kandelia candel. Protein Expr. Purif. 153, 26–34. doi:10.1016/j.pep.2018.08.006
Ellis, W. L., Bowles, J. W., Erickson, A. A., Stafford, N., Bell, S. S., and Thomas, M. (2006). Alteration of the Chemical Composition of Mangrove (Laguncularia Racemosa) Leaf Litter Fall by Freeze Damage. Estuarine, Coastal Shelf Sci. 68, 363–371. doi:10.1016/j.ecss.2006.02.017
Eulgem, T., Rushton, P. J., Robatzek, S., and Somssich, I. E. (2000). The WRKY Superfamily of Plant Transcription Factors. Trends Plant Sci. 5, 199–206. doi:10.1016/S1360-1385(00)01600-9
Eulgem, T., Rushton, P. J., Schmelzer, E., Hahlbrock, K., and Somssich, I. E. (1999). Early Nuclear Events in Plant Defence Signalling: Rapid Gene Activation by WRKY Transcription Factors. EMBO J. 18, 4689–4699. doi:10.1093/emboj/18.17.4689
Fei, J., Wang, Y.-s., Cheng, H., Su, Y.-b., Zhong, Y., and Zheng, L. (2021). Cloning and Characterization of KoOsmotin from Mangrove Plant Kandelia Obovata under Cold Stress. BMC Plant Biol. 21, 10. doi:10.1186/s12870-020-02746-0
Fei, J., Wang, Y.-S., Jiang, Z.-Y., Cheng, H., and Zhang, J.-D. (2015). Identification of Cold Tolerance Genes from Leaves of Mangrove Plant Kandelia Obovata by Suppression Subtractive Hybridization. Ecotoxicology 24, 1686–1696. doi:10.1007/s10646-015-1486-9
Gahlaut, V., Jaiswal, V., Kumar, A., and Gupta, P. K. (2016). Transcription Factors Involved in Drought Tolerance and Their Possible Role in Developing Drought Tolerant Cultivars with Emphasis on Wheat (Triticum aestivum L.). Theor. Appl. Genet. 129, 2019–2042. doi:10.1007/s00122-016-2794-z
Giri, C., Ochieng, E., Tieszen, L. L., Zhu, Z., Singh, A., Loveland, T., et al. (2011). Status and Distribution of Mangrove Forests of the World Using Earth Observation Satellite Data. Glob. Ecol. Biogeogr. 20, 154–159. doi:10.1111/j.1466-8238.2010.00584.x
Guo, C., Guo, R., Xu, X., Gao, M., Li, X., Song, J., et al. (2014). Evolution and Expression Analysis of the Grape (Vitis vinifera L.) WRKY Gene Family. J. Exp. Bot. 65, 1513–1528. doi:10.1093/jxb/eru007
Hu, M.-J., Sun, W.-H., Tsai, W.-C., Xiang, S., Lai, X.-K., Chen, D.-Q., et al. (2020). Chromosome-scale Assembly of the Kandelia Obovata Genome. Hortic. Res. 7, 75. doi:10.1038/s41438-020-0300-x
Ishiguro, S., and Nakamura, K. (1994). Characterization of a cDNA Encoding a Novel DNA-Binding Protein, SPF1, that Recognizes SP8 Sequences in the 5′ Upstream Regions of Genes Coding for Sporamin and β-amylase from Sweet Potato. Mol. Gen. Genet. 244, 563–571. doi:10.1007/BF00282746
Jiang, J., Hou, R., Yang, N., Li, L., Deng, J., Qin, G., et al. (2021). Physiological and TMT-Labeled Proteomic Analyses Reveal Important Roles of Sugar and Secondary Metabolism in Citrus Junos under Cold Stress. J. Proteomics 237, 104145. doi:10.1016/j.jprot.2021.104145
Jiang, J., Ma, S., Ye, N., Jiang, M., Cao, J., and Zhang, J. (2017). WRKY Transcription Factors in Plant Responses to Stresses. J. Integr. Plant Biol. 59, 86–101. doi:10.1111/jipb.12513
Kan, J., Gao, G., He, Q., Gao, Q., Jiang, C., Ahmar, S., et al. (2021). Genome-Wide Characterization of WRKY Transcription Factors Revealed Gene Duplication and Diversification in Populations of Wild to Domesticated Barley. Ijms 22, 5354. doi:10.3390/ijms22105354
Katoh, K., and Standley, D. M. (2013). MAFFT Multiple Sequence Alignment Software Version 7: Improvements in Performance and Usability. Mol. Biol. Evol. 30, 772–780. doi:10.1093/molbev/mst010
Kim, C.-Y., Vo, K. T. X., Nguyen, C. D., Jeong, D.-H., Lee, S.-K., Kumar, M., et al. (2016). Functional Analysis of a Cold-Responsive rice WRKY Gene, OsWRKY71. Plant Biotechnol. Rep. 10, 13–23. doi:10.1007/s11816-015-0383-2
Li, H.-L., Guo, D., Yang, Z.-P., Tang, X., and Peng, S.-Q. (2014). Genome-wide Identification and Characterization of WRKY Gene Family in Hevea Brasiliensis. Genomics 104, 14–23. doi:10.1016/j.ygeno.2014.04.004
Li, H., Ghoto, K., Wei, M.-Y., Gao, C.-H., Liu, Y.-L., Ma, D.-N., et al. (2021). Unraveling Hydrogen Sulfide-Promoted Lateral Root Development and Growth in Mangrove Plant Kandelia Obovata: Insight into Regulatory Mechanism by TMT-Based Quantitative Proteomic Approaches. Tree Physiol. 41, 1749–1766. doi:10.1093/treephys/tpab025
Liu, W., Zheng, C., Chen, J., Qiu, J., Huang, Z., Wang, Q., et al. (2018). Cold Acclimation Improves Photosynthesis by Regulating the Ascorbate-Glutathione Cycle in Chloroplasts of Kandelia Obovata. J. For. Res. 30, 755–765. doi:10.1007/s11676-018-0791-6
Livak, K. J., and Schmittgen, T. D. (2001). Analysis of Relative Gene Expression Data Using Real-Time Quantitative PCR and the 2−ΔΔCT Method. Methods 25, 402–408. doi:10.1006/meth.2001.1262
Lynch, M., and Conery, J. S. (2000). The Evolutionary Fate and Consequences of Duplicate Genes. Science 290, 1151–1155. doi:10.1126/science.290.5494.1151
Maeo, K., Hayashi, S., Kojima-Suzuki, H., Morikami, A., and Nakamura, K. (2001). Role of Conserved Residues of the WRKY Domain in the DNA-Binding of Tobacco WRKY Family Proteins. Biosci. Biotechnol. Biochem. 65, 2428–2436. doi:10.1271/bbb.65.2428
Manna, M., Thakur, T., Chirom, O., Mandlik, R., Deshmukh, R., and Salvi, P. (2021). Transcription Factors as Key Molecular Target to Strengthen the Drought Stress Tolerance in Plants. Physiologia Plantarum 172, 847–868. doi:10.1111/ppl.13268
Meraj, T. A., Fu, J., Raza, M. A., Zhu, C., Shen, Q., Xu, D., et al. (2020). Transcriptional Factors Regulate Plant Stress Responses through Mediating Secondary Metabolism. Genes 11, 346. doi:10.3390/genes11040346
Nan, H., and Gao, L.-z. (2019). Genome-wide Analysis of WRKY Genes and Their Response to Hormone and Mechanic Stresses in Carrot. Front. Genet. 10, 363. doi:10.3389/fgene.2019.00363
Nan, H., Li, W., Lin, Y.-l., and Gao, L.-z. (2020). Genome-Wide Analysis of WRKY Genes and Their Response to Salt Stress in the Wild Progenitor of Asian Cultivated Rice, Oryza Rufipogon. Front. Genet. 11, 359. doi:10.3389/fgene.2020.00359
Nizam, A., Meera, S. P., and Kumar, A. (2022). Genetic and Molecular Mechanisms Underlying Mangrove Adaptations to Intertidal Environments. iScience 25, 103547. doi:10.1016/j.isci.2021.103547
Osland, M. J., Enwright, N., Day, R. H., and Doyle, T. W. (2013). Winter Climate Change and Coastal Wetland Foundation Species: Salt Marshes vs. Mangrove Forests in the southeastern United States. Glob. Change Biol. 19, 1482–1494. doi:10.1111/gcb.12126
Peereman, J., Hogan, J. A., and Lin, T.-C. (2021). Cold Wave-Induced Reductions in NDII and ChlRE for north-western pacific Mangroves Varies with Latitude and Climate History. Remote Sensing 13, 2732. doi:10.3390/rs13142732
Peng, Y.-L., Wang, Y.-S., Fei, J., Sun, C.-C., and Cheng, H. (2015). Ecophysiological Differences between Three Mangrove Seedlings (Kandelia Obovata, Aegiceras corniculatum, and Avicennia marina) Exposed to Chilling Stress. Ecotoxicology 24, 1722–1732. doi:10.1007/s10646-015-1488-7
Price, M. N., Dehal, P. S., and Arkin, A. P. (2010). FastTree 2 - Approximately Maximum-Likelihood Trees for Large Alignments. Plos One 5, e9490. doi:10.1371/journal.pone.0009490
Rushton, P. J., Somssich, I. E., Ringler, P., and Shen, Q. J. (2010). WRKY Transcription Factors. Trends Plant Sci. 15, 247–258. doi:10.1016/j.tplants.2010.02.006
Sheue, C.-R., Liu, H.-Y., and Yong, J. W. H. (2003). Kandelia Obovata (Rhizophoraceae), a New Mangrove Species from Eastern Asia. Taxon 52, 287–294. doi:10.2307/3647398
Silva, E. G. d., Ito, T. M., Ito, T. M., and Souza, S. G. H. d. (2017). In Silico genome-wide Identification and Phylogenetic Analysis of the WRKY Transcription Factor Family in Sweet orange (Citrus Sinensis). Aust. J. Crop Sci. 11, 716–726. doi:10.21475/ajcs.17.11.06.p471
Song, Y., Cui, H., Shi, Y., Xue, J., Ji, C., Zhang, C., et al. (2020). Genome-wide Identification and Functional Characterization of the Camelina Sativa WRKY Gene Family in Response to Abiotic Stress. BMC Genomics 21, 786. doi:10.1186/s12864-020-07189-3
Tang, H., Bi, H., Liu, B., Lou, S., Song, Y., Tong, S., et al. (2020). WRKY33 Interacts with WRKY12 Protein to Up‐regulate RAP2 . 2 during Submergence Induced Hypoxia Response in Arabidopsis thaliana. New Phytol. 229, 106–125. doi:10.1111/nph.17020
Tian, F., Yang, D.-C., Meng, Y.-Q., Jin, J., and Gao, G. (2019). PlantRegMap: Charting Functional Regulatory Maps in Plants. Nucleic Acids Res. 48, 1104–1113. doi:10.1093/nar/gkz1020
van Verk, M. C., Pappaioannou, D., Neeleman, L., Bol, J. F., and Linthorst, H. J. M. (2008). A Novel WRKY Transcription Factor Is Required for Induction of PR-1a Gene Expression by Salicylic Acid and Bacterial Elicitors. Plant Physiol. 146, 1983–1995. doi:10.1104/pp.107.112789
Voorrips, R. E. (2002). MapChart: Software for the Graphical Presentation of Linkage Maps and QTLs. J. Hered. 93, 77–78. doi:10.1093/jhered/93.1.77
Wang, F., Hou, X., Tang, J., Wang, Z., Wang, S., Jiang, F., et al. (2012). A Novel Cold-Inducible Gene from Pak-Choi (Brassica Campestris Ssp. Chinensis), BcWRKY46, Enhances the Cold, Salt and Dehydration Stress Tolerance in Transgenic Tobacco. Mol. Biol. Rep. 39, 4553–4564. doi:10.1007/s11033-011-1245-9
Wang, M., Vannozzi, A., Wang, G., Liang, Y.-H., Tornielli, G. B., Zenoni, S., et al. (2014). Genome and Transcriptome Analysis of the grapevine (Vitis vinifera L.) WRKY Gene Family. Hortic. Res. 1, 1–16. doi:10.1038/hortres.2014.16
Wang, W., You, S., Wang, Y., Huang, L., and Wang, M. (2010). Influence of Frost on Nutrient Resorption during Leaf Senescence in a Mangrove at its Latitudinal Limit of Distribution. Plant Soil 342, 105–115. doi:10.1007/s11104-010-0672-z
Wang, Y., Tang, H., DeBarry, J. D., Tan, X., Li, J., Wang, X., et al. (2012). MCScanX: a Toolkit for Detection and Evolutionary Analysis of Gene Synteny and Collinearity. Nucleic Acids Res. 40, e49. doi:10.1093/nar/gkr1293
Wang, Z., Yu, D., Zheng, C., Wang, Y., Cai, L., Guo, J., et al. (2019). Ecophysiological Analysis of Mangrove Seedlings Kandelia Obovata Exposed to Natural Low Temperature at Near 30°N. Jmse 7, 292. doi:10.3390/jmse7090292
Waqas, M., Azhar, M. T., Rana, I. A., Azeem, F., Ali, M. A., Nawaz, M. A., et al. (2019). Genome-wide Identification and Expression Analyses of WRKY Transcription Factor Family Members from Chickpea (Cicer Arietinum L.) Reveal Their Role in Abiotic Stress-Responses. Genes Genom 41, 467–481. doi:10.1007/s13258-018-00780-9
Wei, K.-F., Chen, J., Chen, Y.-F., Wu, L.-J., and Xie, D.-X. (2012). Molecular Phylogenetic and Expression Analysis of the Complete WRKY Transcription Factor Family in maize. DNA Res. 19, 153–164. doi:10.1093/dnares/dsr048
Wei, M.-Y., Li, H., Zhong, Y.-H., Shen, Z.-J., Ma, D.-N., Gao, C.-H., et al. (2022). Transcriptomic Analyses Reveal the Effect of Nitric Oxide on the Lateral Root Development and Growth of Mangrove Plant Kandelia Obovata. Plant Soil 472, 543–564. doi:10.1007/s11104-021-05271-7
Wu, M., He, Z., Fung, S., Cao, Y., Guan, D., Peng, Y., et al. (2020). Species Choice in Mangrove Reforestation May Influence the Quantity and Quality of Long-Term Carbon Sequestration and Storage. Sci. Total Environ. 714, 136742. doi:10.1016/j.scitotenv.2020.136742
Wu, Y., Huang, W., Tian, Q., Liu, J., Xia, X., Yang, X., et al. (2021). Comparative Transcriptomic Analysis Reveals the Cold Acclimation during Chilling Stress in Sensitive and Resistant Passion Fruit (Passiflora edulis) Cultivars. PeerJ 9, e10977. doi:10.7717/peerj.10977
Wu, Y., Müller, M., Bai, T., Yao, S., Gailing, O., and Liu, Z. (2019). Transcriptome Profiling in Camellia Japonica Var. Decumbens for the Discovery of Genes Involved in Chilling Tolerance under Cold Stress. Ann. For. Res. 62, 51–68. doi:10.15287/afr.2018.1311
Xie, T., Chen, C., Li, C., Liu, J., Liu, C., and He, Y. (2018). Genome-wide Investigation of WRKY Gene Family in Pineapple: Evolution and Expression Profiles during Development and Stress. BMC Genomics 19, 490. doi:10.1186/s12864-018-4880-x
Xu, H., Watanabe, K. A., Zhang, L., and Shen, Q. J. (2016). WRKY Transcription Factor Genes in Wild riceOryza Nivara. DNA Res. 23, 311–323. doi:10.1093/dnares/dsw025
Zeng, Z., Lu, J., Wu, D., Zuo, R., Li, Y., Huang, H., et al. (2020). Poly( ADP ‐ribose) Glycohydrolase Silencing‐mediated H2B Expression Inhibits Benzo(a)pyrene‐induced Carcinogenesis. Environ. Toxicol. 36, 291–297. doi:10.1002/tox.23034
Zhang, C., Wang, D., Yang, C., Kong, N., Shi, Z., Zhao, P., et al. (2017). Genome-wide Identification of the Potato WRKY Transcription Factor Family. Plos One 12, e0181573. doi:10.1371/journal.pone.0181573
Zhang, Y., Yu, H., Yang, X., Li, Q., Ling, J., Wang, H., et al. (2016). CsWRKY46 , a WRKY Transcription Factor from Cucumber, Confers Cold Resistance in Transgenic-Plant by Regulating a Set of Cold-Stress Responsive Genes in an ABA-dependent Manner. Plant Physiol. Biochem. 108, 478–487. doi:10.1016/j.plaphy.2016.08.013
Zheng, C., Ye, Y., Liu, W., Tang, J., Zhang, C., Qiu, J., et al. (2016). Recovery of Photosynthesis, Sucrose Metabolism, and Proteolytic Enzymes in Kandelia Obovata from Rare Cold Events in the Northernmost Mangrove, China. Ecol. Process. 5, 1–12. doi:10.1186/s13717-016-0047-3
Zhou, Q.-Y., Tian, A.-G., Zou, H.-F., Xie, Z.-M., Lei, G., Huang, J., et al. (2008). Soybean WRKY-type Transcription Factor Genes, GmWRKY13, GmWRKY21, and GmWRKY54, Confer Differential Tolerance to Abiotic Stresses in Transgenic Arabidopsis Plants. Plant Biotechnol. J. 6, 486–503. doi:10.1111/j.1467-7652.2008.00336.x
Zhu, D., Hou, L., Xiao, P., Guo, Y., Deyholos, M. K., and Liu, X. (2019). VvWRKY30, a Grape WRKY Transcription Factor, Plays a Positive Regulatory Role under Salinity Stress. Plant Sci. 280, 132–142. doi:10.1016/j.plantsci.2018.03.018
Zhu, Y., Wu, N., Song, W., Yin, G., Qin, Y., Yan, Y., et al. (2014). Soybean (Glycine max) Expansin Gene Superfamily Origins: Segmental and Tandem Duplication Events Followed by Divergent Selection Among Subfamilies. BMC Plant Biol. 14, 93. doi:10.1186/1471-2229-14-93
Keywords: expression profiles, Kandelia obovata, low temperature, phylogenetic analysis, WRKY transcriptional factor
Citation: Du Z, You S, Zhao X, Xiong L and Li J (2022) Genome-Wide Identification of WRKY Genes and Their Responses to Chilling Stress in Kandelia obovata. Front. Genet. 13:875316. doi: 10.3389/fgene.2022.875316
Received: 14 February 2022; Accepted: 11 March 2022;
Published: 31 March 2022.
Edited by:
Zefeng Yang, Yangzhou University, ChinaReviewed by:
Yan Cheng, Fujian Agriculture and Forestry University, ChinaVívian Ebeling Viana, Federal University of Pelotas, Brazil
Hongmin Hou, Qingdao Agricultural University, China
Copyright © 2022 Du, You, Zhao, Xiong and Li. This is an open-access article distributed under the terms of the Creative Commons Attribution License (CC BY). The use, distribution or reproduction in other forums is permitted, provided the original author(s) and the copyright owner(s) are credited and that the original publication in this journal is cited, in accordance with accepted academic practice. No use, distribution or reproduction is permitted which does not comply with these terms.
*Correspondence: Junmin Li, bGlqbXR6Y0AxMjYuY29t