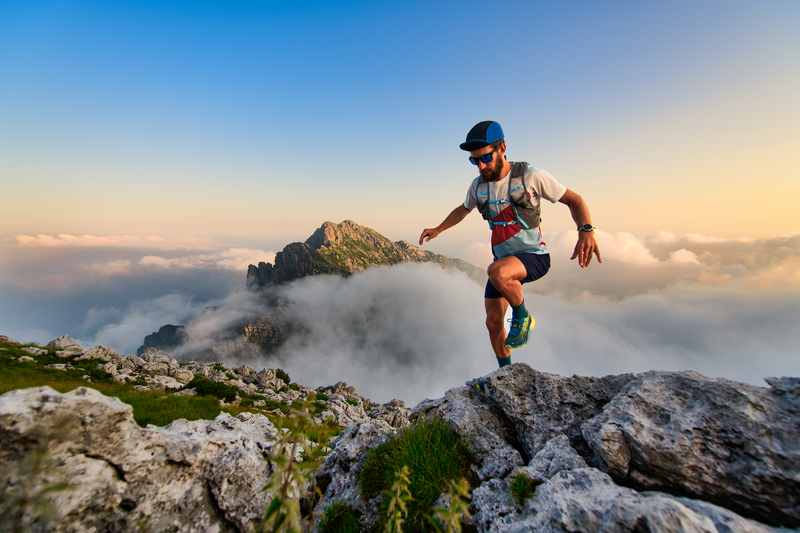
95% of researchers rate our articles as excellent or good
Learn more about the work of our research integrity team to safeguard the quality of each article we publish.
Find out more
ORIGINAL RESEARCH article
Front. Genet. , 17 May 2022
Sec. Livestock Genomics
Volume 13 - 2022 | https://doi.org/10.3389/fgene.2022.820772
This article is part of the Research Topic Sex Determination in Aquatic Animals View all 9 articles
Sex-determining regions have been identified in the Nile tilapia on linkage groups (LG) 1, 20 and 23, depending on the domesticated strains used. Sex determining studies on wild populations of this species are scarce. Previous work on two wild populations, from Lake Volta (Ghana) and from Lake Koka (Ethiopia), found the sex-determining region on LG23. These populations have a Y-specific tandem duplication containing two copies of the Anti-Müllerian Hormone amh gene (named amhY and amhΔY). Here, we performed a whole-genome short-reads analysis using male and female pools on a third wild population from Lake Hora (Ethiopia). We found no association of sex with LG23, and no duplication of the amh gene. Furthermore, we found no evidence of sex linkage on LG1 or on any other LGs. Long read whole genome sequencing of a male from each population confirmed the absence of a duplicated region on LG23 in the Lake Hora male. In contrast, long reads established the structure of the Y haplotype in Koka and Kpandu males and the order of the genes in the duplicated region. Phylogenies constructed on the nuclear and mitochondrial genomes, showed a closer relationship between the two Ethiopian populations compared to the Ghanaian population, implying an absence of the LG23Y sex-determination region in Lake Hora males. Our study supports the hypothesis that the amh region is not the sex-determining region in Hora males. The absence of the Y amh duplication in the Lake Hora population reflects a rapid change in sex determination within Nile tilapia populations. The genetic basis of sex determination in the Lake Hora population remains unknown.
Genetic sex determination (GSD) inherited through sex chromosomes has been highly conserved and relatively stable in mammals and birds for millions of years (Cortez et al., 2014). In the majority of mammals, sex chromosomes are heteromorphic and follow an XX/XY system. The degenerated Y chromosome carries in most mammals the Sry gene, the master gene that triggers the development of male gonads (Sinclair et al., 1990). Birds in contrast have a derived heteromorphic ZW/ZZ system and in most cases ZW embryos become female. Testes develop in the presence of a double dose of the dmrt1 gene on the Z chromosome (Smith et al., 2009; Graves, 2014). The mechanisms of sex determination evolve more rapidly in other vertebrates (Bachtrog et al., 2014). In reptiles, amphibians and fish, sex chromosomes and sex-determination genes often differ among even closely related species (Ezaz et al., 2009; Myosho et al., 2012; Jeffries et al., 2018). Temperature-dependent sex determination (TSD) is also widespread amongst reptiles (Holleley et al., 2016). Most fish species have a GSD dependent on single genes with a large effect carried by sex chromosomes, although some depend on interactions between several genes (Capel, 2017). The environment, particularly temperature, can also determine fish sex (Environmental Sex Determination = ESD) or influence sexual differentiation (Temperature Induced Sex Differentiation = TISD). In the case of temperature-sensitive GSD species, sex reversals can lead to mismatches between phenotypes and sexual genotypes (Baroiller and D'Cotta, 2016).
Sex chromosomes evolve in response to unique patterns of mutation, selection and recombination (Bachtrog et al., 2011). In the classical model of sex chromosome evolution, a new sex chromosome originates when an autosome acquires a sex-determining mutation (e.g., a new Y). If this new sex locus is linked to male-beneficial alleles (sexually antagonistic alleles), it is expected to increase in frequency (Rice, 1987; Charlesworth et al., 2005; van Doorn and Kirkpatrick, 2010). Selection favors suppression of recombination to maintain linkage disequilibrium between the new Y locus and male-beneficial alleles at nearby loci. The non-recombining region may then accumulate a variety of mutations, inversions, and repetitive elements (Kirkpatrick 2010; Bachtrog, 2013; Blaser et al., 2014). Over time the X and Y may become morphologically distinct (Bachtrog, 2013; Abbott et al., 2017). In contrast, 95% of teleost fishes have cytogenetically homomorphic sex chromosomes (Arai, 2011), implying that recombination suppression is probably recent. This is most likely due to the high turnover rates of teleost sex chromosomes, so that the differentiation of the sex chromosomes is reinitiated (Sember et al., 2021). The differences between sexes may be limited to a single missense SNP, such as in pufferfish (Kamiya et al., 2012).
Genomic sequencing has shed light on the diversity of sex systems and sex chromosomes in fish lineages, emphasizing their plasticity with rapid turnover of teleost sex chromosomes and in many cases the existence of multiple sex chromosomes (Sember et al., 2021; Tao et al., 2021). There are frequent transitions in the sex chromosome system, even within the same genus (Cnaani et al., 2008; Takehana et al., 2008; Roberts et al., 2009; Böhne et al., 2019). Some sex-determining genes have been conserved but translocated into different chromosomes (Guiguen et al., 2018). For instance, in the ricefish, the sex-determination gene dmY (also called dmrt1bY) was found on linkage group (LG) 1 in the medaka Oryzias latipes (Matsuda et al., 2002; Nanda et al., 2002). However, in O. luzonensis the sex-determiner is a Y-linked copy of the gsdf gene located on LG12 (Myosho et al., 2012) and in other Oryzias it is sox3 on LG10 (Takehana et al., 2014). A remarkable number of sex chromosome turnovers have occurred in the family Cichlidae, where over 20 sex-determiners have been identified on more than 17 different LGs during an adaptive radiation in East Africa (Gammerdinger & Kocher, 2018; El Taher et al., 2021). Sex determiners have evolved multiple times on the same chromosomes (e.g., on LG5, LG7 and LG19) (Böhne et al., 2019; El Taher et al., 2021). Differentiation of the sex chromosomes can be limited to a small region, or extend over the whole chromosome indicative of extensive recombination suppression (El Taher et al., 2021).
The high species diversity of teleost fish (approximately 27,000 living species) has been proposed to be related to the genomic plasticity of this clade (Helfman et al., 2009). Fishes have a higher rate of evolution and gene duplications than other vertebrates (Mank and Avise, 2009). Indeed, gene duplications are a substrate for the evolution of innovations by giving the duplicated copy (paralog) the possibility to partition the function of the original gene (subfunctionalization), or to acquire a new function (neofunctionalization) (Sandve et al., 2018). Several teleost sex-determining genes have emerged from gene duplication (Mank and Avise, 2009; Ortega-Recalde et al., 2020). Dmy of medaka is a duplicate of the male-differentiating dmrt1 gene found on the autosomal LG9, that was translocated to LG1 (Matsuda et al., 2002; Nanda et al., 2002). In the Patagonian pejerrey (Odontesthes hatcheri) and the Northern pike (Esox lucius) duplicated copies of the amh gene have taken on the role of master sex-determiner (Hattori et al., 2012; Pan et al., 2019). Duplication of genes outside the sex pathway can also result in a major sex-determinant such as the sdy gene in salmonids, a neofunctionalized paralog of an immune-related gene (irf9) (Yano et al., 2012).
Nile tilapia have an XX/XY GSD that can be overridden at high temperatures to generate XX males (Baroiller et al., 2009). This environmental sensitivity, together with extensive hybridization among cultivated strains, has complicated the characterization of the sex chromosomes in tilapias. In some strains sex has been linked to LG1 (Cnaani et al., 2008; Gammerdinger et al., 2014; Palaiokostas et al., 2015) while others show sex linkage on LG23 (Eshel et al., 2014; Li et al., 2015; Wessels et al., 2017; Cáceres et al., 2019) or to both (Taslima et al., 2021). LG20 has also been linked to sex in the domesticated Manzala-Stirling strain (Palaiokostas et al., 2015). The causal gene(s) on LG1 has (ve) not yet been identified. In the Japanese domesticated strain of Nile tilapia, the amh gene on LG23 has been identified as the sex-determination gene, which evolved through a tandem duplication within the same LG. Hence, males have three amh copies: one on the X chromosome and two copies located in tandem on the Y chromosome, one with a missense SNP (amhY) and the other with an insertion that causes a premature stop codon (amhΔY) (Li et al., 2015). We previously found that sex in three wild populations, from Burkina Faso and Ghana (West Africa), and Ethiopia (East Africa) was linked to LG23 (Sissao et al., 2019; Triay et al., 2020). The sex-determining region of the Y chromosome contains an amh duplication estimated to be ∼51 kb using short reads from whole genome sequencing (WGS) and encompassing the adjacent oaz1 and dot1l genes (Triay et al., 2020). However, we were not able to determine the order of the genes on the Y haplotype. We also identified differences in the amhΔY copy in the two wild populations compared with domesticated strains. AmhΔY in the Ethiopian Koka population lacks the 5 bp insertion which causes a premature stop codon (Triay et al., 2020). In addition, some amh markers that were sex-linked in the West African population did not discriminate between XX and XY individuals in the Ethiopian population (Sissao et al., 2019; Triay et al., 2020).
Here, we explore the genetic basis of sex determination in another Ethiopian population from Lake Hora, which is geographically and phylogenetically close to the population in Lake Koka. Both populations live in cold lakes (17–26°C) and belong to the subspecies O. niloticus cancellatus. We expected that the sex-linked duplication of the amh region on LG23 would also be present in Hora males. We used WGS to compare pools of Hora males and females, but failed to identify a sex-linked region. We then used long-read Nanopore sequencing to characterize the LG23 Y haplotype of males from the Kpandu (Ghana) and Koka (Ethiopia) populations and compared them to the corresponding LG23 region of a male from Lake Hora (Ethiopia). Lack of sex-associated SNP pattern on LG23 and Y specific structures and sequences of the duplicated amh region in Hora males, support the hypothesis that the amh region is not the sex-determining region in Hora males and suggests that turnover of the sex-determination system can occur extremely rapidly. Finally, we built phylogenetic trees to characterize the evolutionary history of these populations.
Nile tilapia (Oreochromis niloticus) were caught in 2002 and 2003 from the Sudano-Sahelian basin in West Africa and from two populations in the Ethiopian Rift Valley basin of East Africa (Bezault, 2005). The Kpandu population belongs to the subspecies O. niloticus niloticus and was collected in Ghana from a dendritic expansion of the eastern side of Lake Volta where temperatures fluctuate between 27 and 32°C. The Ethiopian populations belong to the subspecies O. niloticus cancellatus and were collected from two cold-temperature (between 17 and 26°C) lakes in the Awash River basin in the Ethiopian highlands. Lake Koka is a large artificial reservoir of the Awash River of 255 km2 with an average depth of 9 m. Lake Hora is an isolated lake of about 1 km2 with a depth of up to 35 m. Fully mature fish were collected using seine nets and the phenotypic sex was then defined by external examination of the genital papilla (males have only one urogenital orifice whereas females have a genital opening and a urinary orifice; see Baroiller and D’Cotta, 2019). Fish were anaesthetized (but not sacrificed) with 2-phenoxyethanol to sample the fin clips which were preserved in 96–100% ethanol.
High-molecular weight genomic DNA (gDNA) was lysed from the fin clips in TNES-Urea buffer (4 M urea; 10 mM Tris-HCl, pH 7.5; 125 mM NaCl; 10 mM EDTA; 1% SDS) and extracted using the phenol-chloroform procedure. The gDNA was stored in Tris-EDTA buffer at -20°C. DNA was quantified first on a NanoDrop spectrophotometer (ThermoFisher, France) to estimate the absorbance ratios and the concentration was then measured on a Qubit 2.0 fluorometer (Invitrogen Carlsbad, United States). gDNA quality was also verified on a 0.8% agarose gel.
Twenty WGS libraries were prepared at Macrogen (Seoul, Korea) from the gDNA of ten males and ten females gDNA that had been sonicated to obtain 550 bp fragments. All libraries were prepared using the TruSeq DNA PCR-Free kit (Illumina) except one male library where TruSeq Nano DNA Kit (Illumina) was used due to limited amounts of gDNA. Libraries were sequenced (150bp paired-end) in an S4 flow cell on a NovaSeq 6000 (Illumina, San Diego, CA).
Raw data quality was checked using FastQC (0.11.7). Illumina TruSeq adapters were trimmed and short reads (<15bp) removed using fastp (version 0.21.0) (Chen et al., 2018). The fastq files were pooled according to the phenotypic sex of the samples, resulting in a pool of females reads (with 10 samples) and a pool of males reads (with 10 samples). The two pools were mapped to the latest O. niloticus reference genome available on NCBI (O_niloticus_UMD_NMBU, Genbank ID: GCA_001858045.3) using BWA mem mapper (version 0.7.15) (Li and Durbin, 2009). Sam files were then converted to Bam files and sorted using SAMtools (version 1.9) (Li et al., 2009) and read groups were added to the two pools using Picard AddOrReplaceReadGroups (2.7.0) (Broad Institute, 2019). The output files were then combined with SAMtools mpileup (version 1.9). Using Popoolation2 mpileup2sync.pl (version 1.2.2) (Kofler et al., 2011), the mpileup file was transformed to a synchronized file (sync). Finally, the sync file was processed with Sex_SNP_finder_GA.pl (Gammerdinger et al., 2014) to calculate the Fst between males and females. This pipeline allowed us to identify sites that are fixed (or nearly fixed) in one sex and at intermediate frequencies in the other sex. The following parameters were used in Sex_SNP_finder: fixed_threshold = 0.9; minimum_polymorphic_frequency = 0.3; maximum_polymorphic_frequency = 0.7; minimum_read_depth = 10; maximum_read_depth = 100; minimum_read_count = 2; sex_SNP_finder_window_size = 10000. Finally, the Fst were plotted on a manhattan plot using R and the qqman package (version 0.1.4) (Turner, 2018).
One Kpandu male, one Koka male and one Hora male were sequenced using the MinION platform (Oxford Nanopore Technology). The male genomic DNA libraries were constructed from 3 to 4 µg of high molecular weight gDNA by MGX (Montpellier, France) using the Ligation Sequencing Kit 1D (ONT). Samples were fragmented, the ends repaired and adapters ligated. The flow cell FLO-MIN106 (flow cell 9.4.1) was charged twice, with a DNase treatment performed on the flow cell to reactivate the pores when half of the library was sequenced.
Using the results of the Illumina short reads (2 × 150bp) whole genome sequencing published in Triay et al. (2020), we built an expected Y haplotype for the LG23 sex region (Figure 1). This expected Y haplotype is based on the sequence of the X haplotype of the reference genome (an XX female), to which the region corresponding to the duplication of the oaz1 to dot1l genes was inserted according to the breakpoints described in Triay et al. (2020). We kept the nucleotides matching the small deletions (<500bp) found using the short read data in this expected haplotype, as we assumed the Nanopore reads to be long enough to cross short deletions. However, we deleted the sequences corresponding to the two large (>5 kb) deletions to obtain the final sequence of the theoretical Y.
FIGURE 1. Alignment of both the X haplotype and the Y predicted haplotype built according to the descriptions of the tandem duplication in Triay et al., 2020. (A) Geneious alignment of both the X haplotype from the reference genome and the Y theoretical haplotype. Grey bars represent homologous sequences between the X and the Y haplotypes whereas the black region in the Y haplotype represents Y specific regions that appeared due to a duplication of the dot1l, oaz1, LOC100707471 and amh genes. A chimera of the dot1l and oaz1 genes is seen as the result of large deletions that occurred after the duplication. The second copy of the amh gene however is well conserved in this inserted region. (B) Y haplotype prediction published in Triay et al. (2020) with the position of the large deleted regions (in red boxes) removed from the tandem duplication to build the Y of the alignment.
Oxford Nanopore MinION raw reads were used to build a BLAST database (Camacho et al., 2009) against which were blasted the sequences of the oaz1, amh and dot1l genes extracted from the latest available O. niloticus XX female reference genome (O_niloticus_UMD_NMBU, Genbank ID: GCA_001858045.3). Since the first intron of the dot1l gene is very similar to the sequence of the unrelated vasa gene, we split the sequence of dot1l in order to remove this intron and limit its influences in the blast results. All raw reads for which at least one of the sequences presented blast hits were kept. This subset of reads was then trimmed for 23bp at the 3′ and 5′ ends to remove regions with low quality bases. This set of trimmed reads was then mapped onto the theoretical Y haplotype in order to identify Y specific reads according to the presence of Y specific indels described in Triay et al. (2020). We mainly focused on the deletion of about 275bp in length in oaz1 shown to be Y specific in both Kpandu and Koka males (Triay et al., 2020). All reads presenting this deletion were thus labelled as “Y-specific” reads. Conversely, all other reads were labelled as “Non Y-specific.”
Reads of each subset (All Reads, Y specific, Non Y-specific) were de novo assembled using Canu (version 2.1.1) (Koren et al., 2017). The resulting contigs were automatically annotated using Geneious (version 9.0.5) (Kearse et al., 2012), based on the annotation of the reference genome for the defined region. Contigs that could not be annotated were checked using BLAST (version 2.11.0) and were removed when not belonging to the LG23 region of interest. This was the case mainly for the “Non Y-specific” subset of reads and when all reads were used to build de novo assemblies. This BLAST approach, used to identify genes belonging to the LG23 sex region, led us to keep some reads of the oaz1b gene (a paralog of oaz1) and vasa (of which part of the sequence is similar to the dot1l gene). However, contigs containing those genes were easily removed from the final set of contigs. Contigs that were confirmed to belong to LG23 were mapped to the O. niloticus XX female reference genome and to the Y theoretical haplotype using the Geneious assembler (version 9.0.5) with high sensitivity settings to check for the haplotype they corresponded to and whether the structure was the expected one.
In order to increase the contiguity of the Koka Y haplotype, we did a pairwise alignment of only the Y-specific reads using MAFFT (Katoh and Standley, 2013) with a scoring matrix of 100PAM/K = 2 and an automatically chosen algorithm. Among the Y-specific reads, some contained both the amh copies or both the dot1l copies. Thus, the pairwise alignment allowed us to compute a consensus sequence with the Y specific reads, increasing the length of the Y haplotype contig for the Koka male, even though the whole region could not be constructed using long reads assembly software. However, this method of consensus sequence with a low coverage of reads results in many ambiguities.
The 42 loci used by Meyer et al. (2015) to construct their cichlid phylogeny were retrieved in the trimmed data of our wild populations using HMMER (version 3.3.2) (Wheeler and Eddy, 2013) (Supplementary Table S1). In order to maximize the accuracy of nucleotide sequences comparisons, we decided to use only the Illumina short reads data obtained from Hora in the current study, and those for Koka and Kpandu from our previous study (Triay et al., 2020). Because a B chromosome is suspected to be present in the WGS short reads from the female pool of Koka as well as in the male pool of Kpandu individuals, we selected for the phylogeny the Koka male pool, the Kpandu female pool and the Hora female pool. The targeted regions of the mapping alignments were converted from the BAM file to consensus fasta files after SNP calling using bcftools (version 1.9) (Narasimhan et al., 2016). For the two outgroups Etia nguti and Hemichromis elongatus, and for the O. niloticus reference genome, we used the fasta files available on NCBI (Genbank IDs: GCA_015106755.1, GCA_015108515.1 and GCA_001858045.3 respectively). When needed, we produced a reverse complement in order to obtain sequences in identical sense to the sequences of Meyer et al. (2015). The 42 sequences of all samples were then combined into a unique fasta and we added some of the species of Meyer et al. (2015). All sequences were then aligned using MAFFT (v7.475) (Katoh and Standley, 2013) and sites presenting more than 50% missing data among all species/samples, usually corresponding to species-specific insertions, were removed using trimAl (version 1.4. rev22) (Capella-Gutiérrez et al., 2009). Finally, a Maximum-Likelihood phylogeny was produced using IQtree (1.6.12) with 100 bootstraps for node support.
We assembled the mitochondrial genomes from Koka, Hora and Kpandu O. niloticus wild populations obtained from the short reads of our Whole Genome Sequencing trimmed data using MitoFinder (version 1.4) (Allio et al., 2020). We used the reference mitochondrial genome as the basis for annotation (Genbank ID: GU238433.1). In addition, we used the closely related species Haplochromis burtoni as outgroup (NCBI ID: NC_027289.1). In order to use the same basis as the one used for gene annotation, we also ran the reference mitochondrial genome and Haplochromis burtoni through Mitofinder to extract individual genes. The sequences of the 13 mitochondrial protein-coding genes and the two ribosomal subunits (Supplementary Table S2) were then aligned using MAFFT (v7.475) (Katoh and Standley, 2013). A maximum-likelihood phylogeny was inferred from the concatenated sequence alignments using IQtree 1.6.12 (Nguyen et al., 2015) with 100 bootstraps for node support.
Because of the low coverage of long reads data and due to the high error rate of Oxford Nanopore MinION data, we could not use the Y haplotypes built from the de novo analyses. Thus, we focused on the amh gene region and more precisely on the LG23 region from 34,489,620 to 34,532,693 bp according to the XX reference genome and analyzed only female individuals. This corresponds to the region defined as carrying the major sex-determinant in previous studies (Li et al., 2015; Cáceres et al., 2019; Triay et al., 2020; Taslima et al., 2021). This region was extracted from Kpandu, Koka and Hora female mapping files using SAMtools (version 1.9) (Li et al., 2009). These regions were then converted to consensus sequences using Geneious (version 9.0.5) (Kearse et al., 2012). The sequences, along with fasta files of this region from O. niloticus and O. aureus were aligned using MAFFT (v7.475) (Katoh and Standley, 2013). The subsequent alignments were then used to build a phylogeny of Females X haplotypes with 100 bootstraps with IQtree (1.6.12) (Nguyen et al., 2015). The final visualization of all the trees (nuclear, mitochondrion and X haplotypes) were formatted using Geneious (version 9.0.5) (Kearse et al., 2012).
We had previously shown that males had a specific duplication of the amh gene (amhY/amhΔY) on LG23 in the Ethiopian Koka population as well as in the Ghanaian Kpandu population (Triay et al., 2020). We wanted to know if this was also the case in the Ethiopian Hora population. For this, we performed a short read WGS of wild-caught Hora males and females to search for strong sex-biased SNPs between sexes through a genome wide association study (GWAS). We obtained from this sequencing between 108,570,962 and 129,028,644 total reads, representing a mean of 17X per sample. We pooled by sex all the female reads and all the male reads, and then used for our analysis a random subset of 20% of the reads for each pool. The Manhattan plot of Fst (Figure 2) did not reveal any obvious sex pattern on LG23. In the oaz1 to dot1l region we found only one SNP in a non-coding region that differed between males and females. Furthermore, we did not see any reliable signal of sex-specific heterozygosity on other linkage groups for this population.
FIGURE 2. Manhattan plots of Fst for sites fixed or nearly fixed for one sex and at intermediate frequencies for the other sex in the Hora population. (A) Plot for all Linkage Groups. Unanchored contigs were grouped into a single label. (B) Plot of LG23 with the amh region highlighted in yellow. No high Fst was evidenced in the expected amh region.
We also did not find evidence of a B chromosome. We did not observe any of the 16 shared regions of high coverage that potentially belong to a B chromosome, which we had previously found in Kpandu males and Koka females (Triay et al., 2020). Thus, the noisy Fst signal is not from the presence of B chromosomes. Further male/female comparisons will be needed to determine the significance of the high Fst peaks along the genome.
We next performed a long-reads nanopore sequencing to study in Hora males in more depth the region around the amh gene that is duplicated and male-specific in the Koka and Kpandu populations. We sequenced on a MinION a male from each population. We obtained for the Hora male a total of 777,765 long nanopore reads corresponding to 4,034,783,321 bp, 4,474,365 reads corresponding to 22,830,560,109 bp for the Kpandu male and for the Koka male a total of 5,266,388 reads corresponding to 14,776,650,374 bp. The average error rate was 6.44%. This resulted in 228 reads of Kpandu, 287 of Koka and 236 reads of Hora that passed the oaz1, amh and dot1l BLAST filter. The expected Y haplotype structure we constructed is correct since Kpandu and Koka reads mapped to the Y haplotype with high quality, confirming the structure we previously described using WGS short reads data (Triay et al., 2020). However, the coverage was different along this region for the three males. It appeared to be almost constant along the region for the Kpandu and Koka males, but approached 0 in the Hora male (Figure 3). We found no reads in the Hora male that had a good mapping score over the Y specific regions. Moreover, the Hora male presents none of the expected Y specific indels (such as the 275 bp deletion in the truncated copy of the oaz1 gene). This confirms that this Hora male does not carry any tandem duplication in this region and that the amh duplicated region is most likely not the sex-determining region in the Hora population.
FIGURE 3. IGV snapshot of long reads mapping obtained from the Kpandu (Lake Volta, Ghana) male, the Lake Koka (Ethiopia) male and the Lake Hora (Ethiopia) male. Reads were mapped onto a Y expected haplotype. Dashed arrows highlight the coverage level over the beginning of the Y specific region. Reads mapping to the oaz1-dot1l chimera presenting a 275 bp deletion found in the oaz1 truncated copy (within the dashed rectangle) were labelled as Y-specific.
For the Kpandu and Koka males we considered the reads containing a deletion of 275 bp in the truncated oaz1 copy as “Y-specific” reads. The reads not containing this deletion were considered as belonging to the X chromosome. This resulted in 15 long reads that were considered Y-specific for the Kpandu male and 11 for the Koka male. In order to obtain the Y haplotype and study it in more detail, our first approach was to do a de novo assembly of the long reads from the sex-region. The contigs obtained were compared to the O. niloticus XX reference genome (a female from the Stirling-Manzala strain). The automatic annotation of contigs based on the reference genome and the expected Y haplotype allowed us to label the different contigs as X or Y haplotypes.
We observed only one long contig for the Hora male, consistent with an X haplotype (Figure 4). No Y haplotype was assembled, in agreement with the fact that the mapping results revealed no reads presenting Y-specific structures. In contrast, two contigs were assembled for the Kpandu male: one perfectly corresponding to the X reference genome haplotype, and another one presenting the Y-specific structures. The second contig however is much smaller than the X haplotype and is centered around the oaz1-dot1l chimera and a second copy of the amh gene [corresponding to the amhΔY described in the Japanese strain (Li et al., 2015)]. Finally, three contigs were built from the Koka reads. The longest contig carries Y-specific structures, such as the dot1l-oaz1 chimera and the Y-specific indels corresponding to the duplicated region. However, the first copy of the amh gene (which is the amhY in the Japanese strain) is not as fully reconstructed as for the Kpandu male. Two smaller contigs contain respectively the conserved version of the oaz1 gene and the beginning of the amhY gene. No clear X haplotype was built from the de novo using all reads for the Koka male. De novo assemblies of “Non-Y specific” reads did not produce any contig long enough to be associated to the X haplotype either. Moreover, the longest contig assembled using the non Y-specific reads was identified as belonging to the Y haplotype as part of the chimera that could be identified with the automated annotation. Although a Y haplotype is present in the Koka assembly output, we did a de novo assembly with a pairwise alignment method to obtain a longer Koka Y contig.
FIGURE 4. Contigs output of the Canu de novo assembly of the three males belonging to the wild populations of Hora, Koka and Kpandu. Koka and Hora (in blue) are Ethiopian males from lakes of the same names, whereas Kpandu (in red) is from Lake Volta in Ghana. All contigs are visually aligned to the oaz1 gene of the reference genome, based on Geneious automated annotations from the XX reference genome and from the expected Y haplotype built previously. X and Y labels of contigs are deduced according to the structure of contigs, presenting or not the dot1l-oaz1 chimera, specific to the Y haplotype as described in Triay et al. (2020).
The alignment of the Kpandu and Koka Y haplotypes obtained from MinION long reads together with the X haplotypes from the WGS short reads showed that the large structure corresponding to the tandem duplication of oaz1-amh-dot1l genes was well aligned between the two Y haplotypes, and that it was absent in all X haplotypes. This confirmed once again the existence of the Y structure as suggested. The low coverage of the MinION long reads however did not allow us to correct for the sequencing errors or ambiguities, and although it is very informative for the duplicated region it did not allow us to perform SNP or phylogenetic analyses with these Y haplotypes.
To establish the phylogenetic relationships among the three populations studied, we constructed phylogenetic trees based on nuclear genes, on the mitochondrial genome and finally on the X haplotype. Both the nuclear and the mitochondrial phylogenies showed that the two Koka and Hora populations from Ethiopia are more closely related to each other than to the Kpandu population from Ghana (Figure 5). These results are consistent with the geographical location of these three populations as well as with the subspecies taxonomy since both Koka and Hora are populations of O. niloticus cancellatus while the Kpandu population belongs to the subspecies O. niloticus niloticus (Trewavas, 1983; Bezault et al., 2011). The mitochondrial phylogeny also strongly supports a sister clade relationship between the Kpandu O. niloticus population and the O. aureus individual. The X haplotypes of Ethiopian populations also form a monophyletic group, with the Ghanaian Kpandu population as a sister group. In X haplotypes, the divergence between Hora and Koka females is hardly noticeable. Surprisingly, the position of the Kpandu population is different in the three trees. According to the mitochondrial data, Kpandu branches closely to O. aureus, as a sister group to all other O. niloticus sampled here. In the X haplotypes’ phylogeny, the Kpandu population is a sister clade to the reference genome, a tree topology that might be due to the Manzala-Stirling stock (from which was sampled the individual sequenced for the reference genome) originating from the Nile Basin. This basin was isolated from the Sudano-Sahelian region comprising the Kpandu population by several paleo-geographic events (Bezault et al., 2011). However, this result does not stand when studying a wider proportion of the genome, as the analysis of a concatenation of 42 nuclear exons yields a tree where Kpandu is a sister-clade to the Hora and Koka populations although the support for this relation is the weakest in the tree (with a bootstrap support of 71).
FIGURE 5. Phylogenetic analysis of Nile tilapia populations and related cichlids built with the maximum likelihood method. Each node of the tree corresponds to bootstrap values. Koka and Hora (in blue) are Ethiopian populations of O. niloticus cancellatus and Kpandu (in red) is a Lake Volta population of O. niloticus niloticus originating from Ghana. (A) Nuclear DNA phylogeny built using the 42 loci from Meyer et al. (2015). The Oreochromis species are forming a monophyletic clade in which the three wild populations are more closely related to each other than to the individual used from the reference genome (Manzala-Stirling strain). The tree is rooted with Hemichromis elongatus. The species from Meyer et al., 2015 are positioned identically to their phylogeny. These results are also showing that the O. niloticus cancellatus Koka and Hora populations are more closely related to each other than to the O. niltocus niloticus of Kpandu population. (B) Mitochondrial DNA phylogeny built from 13 mitochondrial protein-coding genes and the two ribosomal subunits. The tree is rooted with Haplochromis burtoni. This phylogeny is confirming that Koka and Hora populations are more closely related to each other than to Kpandu population. O. niloticus samples from Kpandu population are forming a monophyletic clade with O. aureus. (C) LG23 X haplotype phylogeny from LG23 oaz1 to dot1l genes. The tree is rooted with O. aureus. Koka and Hora O. niloticus populations are forming a monophyletic clade
Among populations of O. niloticus the sex determining locus has been found on LG1 (Lee et al., 2003; Cnaani et al., 2008; Gammerdinger et al., 2014; Palaiokostas et al., 2015) or on LG23 (Eshel et al., 2014; Li et al., 2015), or ob both (Taslima et al., 2021). The most prevalent and widespread sex chromosome is LG23 since it has been found now in numerous domesticated strains (Eshel et al., 2014; Li et al., 2015; Wessels et al., 2017; Cáceres et al., 2019; Curzon et al., 2020; Taslima et al., 2020) and also in wild populations (Sissao et al., 2019; Triay et al., 2020). We have previously shown that the amhY and amhΔY tandem duplication on the Y haplotype of LG23 is found in males of the two wild populations of Kpandu (from the Volta Lake in Ghana) and Lake Koka (in Ethiopia) (Triay et al., 2020). Because of the distant relationship of Kpandu-Volta in West Africa and Koka populations in East Africa (Bezault et al., 2011), we presume that the male-specific region is most likely ancestral in the O. niloticus species. Hence, we expected to find the same male-specific region on LG23 in another closely related population living in Lake Hora also from Ethiopia. Surprisingly, our short read genomic sequencing of Hora males and females revealed no association of sex with LG23 (Figure 2). Furthermore, among a total of ten wild males studied, no evidence of a duplication was observed in the alignment of the amh region. These results indicate that the LG23 Y is either segregating in very low frequencies and is no longer the major sex determinant in the Hora population or that it could have been lost entirely.
In our previous study, we predicted a Y haplotype based on the analyses of short reads of the duplicated region in males of the Koka and Kpandu populations (Triay et al., 2020). Assemblies of the Nanopore long reads, aided by this expected Y haplotype, allowed us to cross the duplicated region encompassing the two amh genes on LG23. We were able to confirm the presence of this Y-specific duplication, its structure and the order of genes inside this region, in wild populations from Lake Koka in Ethiopia and Lake Volta in Ghana (Figure 3). In both populations, the duplication occurs within the oaz1 and dot1l genes, and contains truncated copies of dot1l and oaz1 upstream of the amhY promoter. Kpandu males from Lake Volta have a similar structure and the same indels as the males from the Japanese strain analyzed by Li et al. (2015). One of the amh genes on the Y of Kpandu males resembles the truncated amhΔY copy found in the males from the Japanese strain (Li et al., 2015) since it also has the ∼233 bp deletion in the last exon 7 as well as the 5 bp insertion of exon 6 that causes a premature stop codon. Therefore, the other Kpandu amh gene is presumably the SD amhY gene but it does not have the missense SNP found in the Japanese strain (Li et al., 2015). In contrast, the two amh genes in Koka males do not have neither the missense SNP of amhY, nor the indels within amhΔY, particularly the 5 bp insertion responsible for a truncated gene. Consequently, both the amhY and amhΔY of Koka males might be functional and may play a role in maleness. In Hora males however, no Y haplotype could be constructed from the long reads (Figures 3, 4). The assemblies of Hora males revealed that they had a single copy of amh similar to the X haplotype, and confirmed that there is no male-specific duplication around the amh region on the LG23. Hora males could therefore have lost the sex determining region and LG23 is likely no longer the sex chromosome.
Regarding the rest of the genome, although we found an overall high Fst throughout the genome, we found no significant association of sex with other LGs. In particular, we did not find an association with LG1in Hora individuals. Sex segregated for LG1 in some domesticated strains from US commercial stocks (Gammerdinger et al., 2014), in a Ghanaian family (Cnaani et al., 2008) and in the Manzala-Stirling strain (Palaiokostas et al., 2015). In this last strain, LG23-Y is still segregating at low frequencies (Taslima et al., 2021), although sex maps to LG23 in both the Manzala-Göttingen stock (Wessels et al., 2017) and the Manzala-Tihange stock (Sissao et al., 2019) which originated from the Manzala-Stirling stock. No wild population studied to date shows sex segregation to LG1. It is plausible that LG1 might have become the pair of sex chromosomes under conditions such as domestication inducing gene loss or fixation by drift. The fact that this individual presents such a long branch in our phylogenies reinforces the idea of a strong molecular divergence of this stock. This might have occurred during gynogenesis to produce the homogygous female clonal line from which the reference genome individual belonged (Sarder et al., 1999). Change in SD system with the loss of the sex chromosome occurred in laboratory stocks of zebrafish (Wilson et al., 2014). Alternatively, this molecular divergence of the Manzala-Stirling strain could result from a hybridization that might have happened during the process of domestication, which could explain the large divergence observed with other O. niloticus populations. This could even be the cause of the emergence of a new sex-determining system on LG1. For instance, the Thai- Chitralada hybrid, a strain originating from crosses of O. niloticus, O. mossambicus and O. aureus, presents sex-associated markers on LG1, LG3 and LG23 (Curzon et al., 2019). An introgression of closely related species of O. niloticus could thus favor the turnover of sex-chromosomes so that LG1 became fixed as a new sex chromosome.
The absence of the Y amh duplication in the Hora Lake population reflects a rapid change in SD within Nile tilapia populations. This is another example of the rapid turnover of SD within the cichlid family. Indeed, more than 20 different major sex-determining genes, on more than 17 LGs, have now been described in cichlids, highlighting the fact that in this family there are a great diversity of sex determining systems that have emerged between closely related species (Gammerdinger and Kocher, 2018; El Taher et al., 2021; Tao et al., 2021). To date, cichlids from Lake Tanganyika, appear to have the highest rates of sex chromosome turnover as well as transitions between male and female heterogamety (El Taher et al., 2021).
Our study emphasizes the need to analyse the evolution of sex chromosomes at the population level. Studies in Nile tilapia have demonstrated that in domesticated strains (Wessels et al., 2014; Taslima et al., 2021) and wild populations (Bezault et al., 2007; Sissao et al., 2019) the sex-determination system can be more complex than just the amh gene on LG23. Sex in the Nile tilapia is genetically inherited through the sex chromosomes but there is also a genetic inheritance of autosomal factors and of temperature sensitivity (Baroiller and D’Cotta, 2001; Bezault et al., 2007; Baroiller et al., 2009; Wessels et al., 2017). Temperature could facilitate the transition to a new sex chromosome/determination system (Grossen et al., 2011; Baroiller and D’Cotta, 2016). This, along with the results of the Hora population lacking the LG23 Y sex-determinant suggest that the turnover in sex chromosomes and sex-determination systems might not be rare events at the intraspecific level. Variance in sexual conflict and recombination patterns, two important factors possibly for the evolution of sex chromosomes, could induce divergence between sex chromosomes of different populations.
The absence of the Y chromosome on LG23 in Hora raises the question of why the Hora population has experienced a turnover in the system of sex determination. Causes for sex chromosome turnovers can be numerous, including a loss of the sex-determinant by drift, sex ratio bias (e.g. environmental effects inducing sex-reversal), or pleiotropic effects of a sexually antagonistic mutation (Tao et al., 2021). Demographic events might have caused changes in Lake Hora SD. Lake Hora is thought to have been restocked at the beginning of the 20th century with the endemic subspecies O. n. cancellatus (Bezault, 2005). Lake Hora is, nevertheless, a small lake (∼1.15 km2) and the effective population size is supposedly small. The Hora population might have experienced a genetic bottleneck that led to the loss of the major sex-determinant region on LG23-Y by drift. Intraspecific differences in SD was also observed for the haplochromine cichlid Pseudocrenilabrus philander for which WGS detected a strong XY locus in LG7 in a lake population whereas no GSD was found in the outflow creek population which experienced genetic bottleneck (Böhne et al., 2019).
Sex ratio bias can also occur in O. niloticus species because of the effects of environmental temperature. This species can undergo a sex reversal over the threshold temperature of 32°C, causing XX individuals to develop as viable and fertile males. This temperature sensitivity is a variable and heritable trait (Bezault et al., 2007; Wessels and Hörstgen-Schwark, 2007; Baroiller et al., 2009; Wessels and Hörstgen-Schwark, 2011). In the most extreme scenario, the increasing number of XX individuals (males and females) in a population could progressively lead to the loss of the Y chromosome and allow a new master gene to control sex-determination in the population. XX males have been found in many wild populations living in masculinizing temperatures above 32°C such as Lake Metahara in Ethiopia (Bezault et al., 2007; Baroiller et al., 2009). Lake Hora is referred to as a “cold” lake because its temperature varies between 17 and 26°C during the year, which is well below the masculinizing temperature. Nevertheless, Lake Hora might have been restocked with a high proportion of XX males. Hence, the loss of an ancestral LG23 Y system in the Hora fish could have been facilitated by demographic events of this small population, with colonization of XX neo-males and perhaps influenced by founder effects of a small number of females.
What could the current sex determining system(s) of the Hora population be? When a major sex-determinant is lost, it can be replaced by a new “master” gene, possibly already implicated in the sex-determination and differentiation pathway. In the case of the Hora population, we did not identify any obvious sex-determinant loci when using whole genome sequencing of wild male and female pools. This could be due to the new major sex-determinant region being too recent to have accumulated enough SNPs to be detected through genome wide Fst analysis. It is also possible that after the loss of the major determinant LG23 Y, other loci involved in sex determination and differentiation pathway acquire a more important role and coexist during a transition period until the fixation and potential appearance of a new major determinant (Furman et al., 2020; Tao et al., 2021). In this case, more than one locus is segregating on several chromosomes, appearing as a polygenic sex determining system (Mank and Avise, 2009; Roberts et al., 2009). Although WGS of pooled sexes is a very efficient method to quickly detect sex determination locus and the linkage group acting as a sex chromosome when the system is monogenic, it is not as efficient to detect polygenic systems (Gammerdinger and Kocher, 2018). We cannot exclude the scenario of two sex-determining loci (or more) segregating in the Hora population as recently suggested by (Taslima et al., 2021) for the Manzala-Stirling stock, and evidenced in several cichlids (Tao et al., 2021). Another alternative is that the SD in the Hora population has suffered a transition from a genetic to an environmental sex determination system as recently demonstrated in a ZZ/ZW lizard (Holleley et al., 2015). Association of other genomic approaches, other than sex-pooled WGS, with more alignments strategies will be necessary to search further for the sex determination locus or sex chromosome(s) in Hora fish.
The X and Y chromosomes of the Koka and Kpandu wild populations of O. niloticus studied here are visually homomorphic, like all cichlids sex chromosomes karyotyped to date (Gammerdinger and Kocher, 2018). Reduced sex chromosome differentiation is evidenced by the small size of the sex-specific region (<0.5 Mb) of LG23 suggesting that recombination still occurs around the sex-region. The study of genotype-phenotype associations using ddRAD methods on families of Kpandu and Koka populations already suggested that recombination could occur close to the male-specific region (Triay et al., 2020). This reduced recombination further highlights the fact that LG23 has remained a “young” pair of sex chromosomes. The shared structure between males of the two wild populations suggests this XX/XY system predates the divergence of populations of O. niloticus. Thus, even if chronologically old, Nile Tilapia sex chromosomes do not seem to have evolved to a non-recombinant Y that starts to differentiate, but is maintained at a state of low recombination for long evolutionary times.
Our phylogenetic studies confirmed that the Ethiopian Koka and Hora populations, both from the subspecies Oreochromis niloticus cancellatus, belong to a monophyletic clade with the Kpandu population O. niloticus niloticus branching as a sister group. These results appear contradictory with a shared ancestral Y chromosome between Koka and Kpandu males that is not shared between Koka and Hora. Microsatellite analysis showed that the Koka population of the Ethiopian Awash basin is genetically different at the macrogeographical level from the Kpandu population located in the Sudano-Sahelian region (Bezault et al., 2011). The phylogeny we inferred is in accordance with this previous study: the subspecies O. n. cancellatus found in Lake Koka and Lake Hora in the Ethiopian basin are sister populations, reflecting the genetic proximity seen at the micro-geographic level (Bezault et al., 2011), while the Kpandu population is more divergent. It is parsimonious to think that the Y amh duplication on LG23 is ancestral since it is found in Nile tilapia populations from two distant African hydrographic basins. Nevertheless, to better understand the evolutionary history of the LG23 Y chromosome, phylogenies including additional populations are required. It would be important to add populations from the Nile region because fish from Lake Manzala (Egypt) might carry a sex locus on LG1. It would also be important to add other populations from the Ethiopian Rift Valley. Inferring a phylogeny with several of these populations and checking for the presence/absence of the amh duplication on LG23 Y would enable us to date the event that led to the apparent loss of the LG23 Y in the Hora population.
We noted an incongruence for the Kpandu position between the nuclear, mitochondrial and the X haplotypes’ phylogenies (Figure 5). Our mitochondrial DNA analysis demonstrates a clustering of Kpandu O. niloticus population with the sample of O. aureus, also a Nilo-Sudanic species. The formation of a monophyletic group is consistent with the study of Rognon and Guyomard, (2003) in which they hypothesize a complete introgression of the mitochondrial DNA from O. aureus to O. niloticus in Western African populations, but also in Egyptian lakes. Clustering of Kpandu Nile tilapia and O. aureus was also observed when using mtDNA but they were differentiated in phylogenetic trees derived from nuclear DNA markers (SNPs) (Syaifudin et al., 2019). Discordance between mtDNA and nuclear DNA has been shown in several studies within the Oreochromine cichlids (Ford et al., 2019). Mitochondria DNA reflects only maternal inheritance, whereas species topology is probably more reliable when using multi-nuclear markers (Meyer et al., 2015; Ford et al., 2019). Ancient hybridization was suggested as being the most likely explanation for the discordance between mtDNA and nuDNA (Dunz and Schliewen, 2013). If this is the case, our mitochondrial analysis is not suitable to infer the relative position of Kpandu to our other O. niloticus samples. By contrast, our nuclear DNA datasets should not be affected by mitochondrial introgressions. However, we observed two possible branchings of O. niloticus samples. In our phylogeny built with the X haplotypes, Kpandu groups with the reference genome while this population is a sister-clade to the Ethiopian populations Hora and Koka in the nuclear exon tree. These conflicting signals may result from a methodological bias. Indeed, the nuclear exons’ phylogeny was inferred using more data than the X haplotype analysis, sampled from 42 different loci in the genomes. This methodology should alleviate sampling errors due to loci-specific events, such as incomplete lineage sorting or GC-biased gene conversion (Degnan and Rosenberg, 2009), even if it does not completely resolve related difficulties (Philippe et al., 2011). On the other hand, we see that this node is the least supported in the tree, hinting that this incongruence may be due to a phenomenon affecting a large proportion of the genome. Thus, a biological explanation such as hybridization with other strains may also explain this discrepancy. Moreover, the individual of O. niloticus used for the reference genome (a XX female belonging to a gynogenetic homozygous clonal line generated at the University of Stirling (Sarder et al., 1999) shows a longer branch than all other samples in the three trees, which may also be the result of admixture with another more distantly related population. Proper admixture analyses could help untangle this relationship between the Kpandu population and the reference genome.
Our phylogenetic results suggest that at some point in the evolutionary history of O. niloticus introgression with other closely related cichlids species might have occurred. O. niloticus might have hybridized in the past or recently due to aquaculture practices with other closely related species since several species coexist in many African fresh water basins. O. aureus and O. niloticus divergence was estimated to be 2.93 MYA (Xiao et al., 2015). They cohabitate in several river basins, both being Nilo-Sudano species (Trewavas, 1983), but they are not known to hybridize naturally (Payne and Collinson, 1983). In Lake Manzala there is a question on whether hybridization might have occurred between Nile tilapia and S. galilaeus confused perhaps as O. aureus (Ford et al., 2019). This might be the explanation of a SD on LG1 appearing in several O. niloticus strains (Cnaani et al., 2008). Thus, particularly when studying sex chromosomes, it might be essential to consider the other sex-determining loci segregating in the sympatric species of studied populations.
In this work, we sequenced the whole genome of a Nile tilapia from Lake Hora, Ethiopia. The short read analysis revealed the absence of sex-linked sequences on LG23 and we were unable to find a strong association of sex with any region of the genome in this population. Using long reads, we reconstructed a Y haplotype corresponding to a duplicated male-specific region on the LG23 sex chromosome in populations from Lake Volta (Ghana) and Lake Koka (Ethiopia). This male-duplication of LG23 spans ∼51 kb and is likely to be ancestral to the divergence of Eastern and Western African populations. In contrast, the amh male-specific duplication could not be detected in the Hora males using either WGS or nanopore long reads, suggesting that this population has lost the LG23 Y chromosome. This work highlights that turnover of the sex-determination system can occur rapidly even between closely related populations in the O. niloticus species. One hypothesis is that sex determination in the Hora population might be an allelic diversification on another LG, or alternatively sex determiners might be segregating on several LGs in a polygenic SD system. Another alternative is that the Hora population have acquired an ESD system. Further analysis will be required to understand the sex-determination system in the Hora population and potentially find the major sex-determination gene.
The datasets presented in this study can be found in online repositories. The names of the repository/repositories and accession number(s) can be found below: National Center for Biotechnology Information (NCBI) BioProject database under accession number PRJNA802819. The raw Hora WGS Illumina sequencing data are under accession numbers SAMN25598580 to SAMN25598599. The data of the raw MinION nanopore long reads are under accession numbers SAMN25610565 to SAMN25610567.
The animal study was reviewed and approved by Fish captures complied with the veterinary laws of each country as stipulated in 2003. All animal and experimental procedures were performed in accordance with the French protocol N°2016101810463 and the French Government’s authorization for animal experimentation N° 35-15.
Conceptualization CT, HD, TK and J-FB; methodology CT, HD, and TK; software CT and MC; validation CT, HD, TK, and J-FB; formal analysis CT, MC, HD, TK, and J-FB; investigation CT, MC, HD, and PC; resources EB and J-FB; data curation CT, HD, JFB, and TK; writing-original draft preparation CT, MC, HD, J-FB, and TK; writing-review and editing CT, MC, HD, J-FB, TK, and EB; visualization CT, MC, HD, J-FB, and TK; supervision, HD and TK. J-FB and HD contributed to the project administration and funding acquisition J-FB as coordinator of the CLIMSEX project. All authors have read and agreed to the published version of the manuscript.
This work was funded by the CLIMSEX Project awarded by the Agence Nationale de la Recherche grant number ANR-15-CE02-0012.
The authors declare that the research was conducted in the absence of any commercial or financial relationships that could be construed as a potential conflict of interest.
All claims expressed in this article are solely those of the authors and do not necessarily represent those of their affiliated organizations, or those of the publisher, the editors, and the reviewers. Any product that may be evaluated in this article, or claim that may be made by its manufacturer, is not guaranteed or endorsed by the publisher.
The Supplementary Material for this article can be found online at: https://www.frontiersin.org/articles/10.3389/fgene.2022.820772/full#supplementary-material
Abbott, J. K., Nordén, A. K., and Hansson, B. (2017). Sex Chromosome Evolution: Historical Insights and Future Perspectives. Proc. R. Soc. B. 284, 20162806. doi:10.1098/rspb.2016.2806
Allio, R., Schomaker‐Bastos, A., Romiguier, J., Prosdocimi, F., Nabholz, B., and Delsuc, F. (2020). MitoFinder: Efficient Automated Large‐scale Extraction of Mitogenomic Data in Target Enrichment Phylogenomics. Mol. Ecol. Resour. 20, 892–905. doi:10.1111/1755-0998.13160
Arai, R. (2011). Fish Karyotypes: A Check List. 1st edn. Tokyo, Japan: Springer. doi:10.1007/978-4-431-53877-6
Bachtrog, D., Kirkpatrick, M., Mank, J. E., McDaniel, S. F., Pires, J. C., Rice, W., et al. (2011). Are All Sex Chromosomes Created Equal? Trends Genet. 27, 350–357. doi:10.1016/j.tig.2011.05.005
Bachtrog, D., Mank, J. E., Peichel, C. L., Kirkpatrick, M., Otto, S. P., Ashman, T.-L., et al. (2014). Sex Determination: Why So many Ways of Doing it? Plos Biol. 12, e1001899. doi:10.1371/journal.pbio.1001899
Bachtrog, D. (2013). Y-chromosome Evolution: Emerging Insights into Processes of Y-Chromosome Degeneration. Nat. Rev. Genet. 14, 113–124. doi:10.1038/nrg3366
Baroiller, J.-F., and D'Cotta, H. (2016). The Reversible Sex of Gonochoristic Fish: Insights and Consequences. Sex. Dev. 10, 242–266. doi:10.1159/000452362
Baroiller, J. F., D'Cotta, H., Bezault, E., Wessels, S., and Hoerstgen-Schwark, G. (2009). Tilapia Sex Determination: where Temperature and Genetics Meet. Comp. Biochem. Physiol. A: Mol. Integr. Physiol. 153, 30–38. doi:10.1016/j.cbpa.2008.11.018
Baroiller, J. F., and D'Cotta, H. (2001). Environment and Sex Determination in Farmed Fish. Comp. Biochem. Physiol. C: Toxicol. Pharmacol. 130, 399–409. doi:10.1016/s1532-0456(01)00267-8
Baroiller, J. F., and D’Cotta, H. (2019). “Sex Control in Tilapias,” in Sex Control in Fish” Eds Drs. Hanping Wang (US), Francesc Piferrer (Spain) and Songlin Chen (China) (Wiley Blackwell).
Bezault, E., Balaresque, P., Toguyeni, A., Fermon, Y., Araki, H., Baroiller, J.-F., et al. (2011). Spatial and Temporal Variation in Population Genetic Structure of Wild Nile tilapia (Oreochromis niloticus) across Africa. BMC Genet. 12, 102. doi:10.1186/1471-2156-12-102
Bezault, E., Clota, F., Derivaz, M., Chevassus, B., and Baroiller, J.-F. (2007). Sex Determination and Temperature-Induced Sex Differentiation in Three Natural Populations of Nile tilapia (Oreochromis niloticus) Adapted to Extreme Temperature Conditions. Aquaculture 272, S3–S16. doi:10.1016/j.aquaculture.2007.07.227
Bezault, E. (2005). Etude Du Systeme De Determinisme Du Sexe au Sein De Populations Naturelles De Tilapia Du Nil, Oreochromis niloticus (linnaeus, 1758) : importance Des Composantes Genetiques Et Environnementales. PhD thesis. University of Paris-Sud, U.F.R. Scientifique d'Orsay.
Blaser, O., Neuenschwander, S., and Perrin, N. (2014). Sex-Chromosome Turnovers: The Hot-Potato Model. The Am. Naturalist 183, 140–146. doi:10.1086/674026
Böhne, A., Weber, A. A.-T., Rajkov, J., Rechsteiner, M., Riss, A., Egger, B., et al. (2019). Repeated Evolution versus Common Ancestry: Sex Chromosome Evolution in the Haplochromine CichlidPseudocrenilabrus Philander. Genome Biol. Evol. 11, 439–458. doi:10.1093/gbe/evz003
Cáceres, G., López, M. E., Cádiz, M. I., Yoshida, G. M., Jedlicki, A., Palma-Véjares, R., et al. (2019). Fine Mapping Using Whole-Genome Sequencing Confirms Anti-müllerian Hormone as a Major Gene for Sex Determination in Farmed Nile Tilapia (Oreochromis niloticus L.). G3 (Bethesda) 9, 3213–3223. doi:10.1534/g3.119.400297
Camacho, C., Coulouris, G., Avagyan, V., Ma, N., Papadopoulos, J., Bealer, K., et al. (2009). BLAST+: Architecture and Applications. BMC Bioinformatics 10, 421. doi:10.1186/1471-2105-10-421
Capel, B. (2017). Vertebrate Sex Determination: Evolutionary Plasticity of a Fundamental Switch. Nat. Rev. Genet. 18 (11), 675–689. doi:10.1038/nrg.2017.60
Capella-Gutiérrez, S., Silla-Martínez, J. M., and Gabaldón, T. (2009). trimAl: a Tool for Automated Alignment Trimming in Large-Scale Phylogenetic Analyses. Bioinformatics 25, 1972–1973. doi:10.1093/bioinformatics/btp348
Charlesworth, D., Charlesworth, B., and Marais, G. (2005). Steps in the Evolution of Heteromorphic Sex Chromosomes. Heredity 95, 118–128. doi:10.1038/sj.hdy.6800697
Chen, S., Zhou, Y., Chen, Y., and Gu, J. (2018). Fastp: an Ultra-fast All-In-One FASTQ Preprocessor. Bioinformatics 34, i884–i890. doi:10.1093/bioinformatics/bty560
Cnaani, A., Lee, B.-Y., Zilberman, N., Ozouf-Costaz, C., Hulata, G., Ron, M., et al. (2008). Genetics of Sex Determination in Tilapiine Species. Sex. Dev. 2, 43–54. doi:10.1159/000117718
Cortez, D., Marin, R., Toledo-Flores, D., Froidevaux, L., Liechti, A., Waters, P. D., et al. (2014). Origins and Functional Evolution of Y Chromosomes across Mammals. Nature 508, 488–493. doi:10.1038/nature13151
Curzon, A. Y., Seroussi, E., Benet-Perelberg, A., Zak, T., Dor, L., Shirak, A., et al. (2019). Hybrid Origin of the Thai-Chitralada Tilapia Strain Using DNA Barcoding and Microsatellite Analysis. Israeli J. Aquac. - Bamidgeh. doi:10.46989/001c.20988
Curzon, A. Y., Shirak, A., Dor, L., Zak, T., Perelberg, A., Seroussi, E., et al. (2020). A Duplication of the Anti-müllerian Hormone Gene Is Associated with Genetic Sex Determination of Different Oreochromis niloticus Strains. Heredity 125, 317–327. doi:10.1038/s41437-020-0340-x
Degnan, J. H., and Rosenberg, N. A. (2009). Gene Tree Discordance, Phylogenetic Inference and the Multispecies Coalescent. Trends Ecol. Evol. 24, 332–340. doi:10.1016/j.tree.2009.01.009
Dunz, A. R., and Schliewen, U. K. (2013). Molecular Phylogeny and Revised Classification of the Haplotilapiine Cichlid Fishes Formerly Referred to as "Tilapia". Mol. Phylogenet. Evol. 68, 64–80. doi:10.1016/j.ympev.2013.03.015
El Taher, A., Ronco, F., Matschiner, M., Salzburger, W., and Böhne, A. (2021). Dynamics of Sex Chromosome Evolution in a Rapid Radiation of Cichlid Fishes. Sci. Adv. 7, eabe8215. doi:10.1126/sciadv.abe8215
Eshel, O., Shirak, A., Dor, L., Band, M., Zak, T., Markovich-Gordon, M., et al. (2014). Identification of Male-specific Amh Duplication, Sexually Differentially Expressed Genes and microRNAs at Early Embryonic Development of Nile tilapia (Oreochromis niloticus). BMC Genomics 15, 774. doi:10.1186/1471-2164-15-774
Ezaz, T., Sarre, S. D., O’Meally, D., Marshall Graves, J. A., and Georges, A. (2009). Sex Chromosome Evolution in Lizards: Independent Origins and Rapid Transitions. Cytogenet. Genome Res. 127, 249–260. doi:10.1159/000300507
Ford, A. G. P., Bullen, T. R., Pang, L., Genner, M. J., Bills, R., Flouri, T., et al. (2019). Molecular Phylogeny of Oreochromis (Cichlidae: Oreochromini) Reveals Mito-Nuclear Discordance and Multiple Colonisation of Adverse Aquatic Environments. Mol. Phylogenet. Evol. 136, 215–226. doi:10.1016/j.ympev.2019.04.008
Furman, B. L. S., Metzger, D. C. H., Darolti, I., Wright, A. E., Sandkam, B. A., Almeida, P., et al. (2020). Sex Chromosome Evolution: So many Exceptions to the Rules. Genome Biol. Evol. 12, 750–763. doi:10.1093/gbe/evaa081
Gammerdinger, W. J., Conte, M. A., Acquah, E. A., Roberts, R. B., and Kocher, T. D. (2014). Structure and Decay of a Proto-Y Region in Tilapia, Oreochromis niloticus. BMC Genomics 15. doi:10.1186/1471-2164-15-975
Gammerdinger, W. J., and Kocher, T. D. (2018). Unusual Diversity of Sex Chromosomes in African Cichlid Fishes. Genes 9, 480. doi:10.3390/genes9100480
Graves, J. A. M. (2014). Avian Sex, Sex Chromosomes, and Dosage Compensation in the Age of Genomics. Chromosome Res. 22, 45–57. doi:10.1007/s10577-014-9409-9
Grossen, C., Neuenschwander, S., and Perrin, N. (2011). Temperature-dependent Turnovers in Sex-Determination Mechanisms: a Quantitative Model. Evolution 65, 64–78. doi:10.1111/j.1558-5646.2010.01098.x
Guiguen, Y., Bertho, S., Herpin, A., and Fostier, A. (2018). “Sex Determination and Sex Control in Salmonidae,” in Sex Control in Aquaculture (Chichester, UK: John Wiley & Sons), 251–281. doi:10.1002/9781119127291.ch11
Hattori, R. S., Murai, Y., Oura, M., Masuda, S., Majhi, S. K., Sakamoto, T., et al. (2012). A Y-Linked Anti-mullerian Hormone Duplication Takes over a Critical Role in Sex Determination. Proc. Natl. Acad. Sci. 109, 2955–2959. doi:10.1073/pnas.1018392109
Helfman, G. S., Colette, B. B., Facey, D. E., and Bowen, B. W. (2009). The Diversity of Fishes: Biology, Evolution, and Ecology. Chichester, UK; Hoboken, NJ: Blackwell.
Holleley, C. E., O'Meally, D., Sarre, S. D., Marshall Graves, J. A., Ezaz, T., Matsubara, K., et al. (2015). Sex Reversal Triggers the Rapid Transition from Genetic to Temperature-dependent Sex. Nature 523, 79–82. doi:10.1038/nature14574
Holleley, C. E., Sarre, S. D., O'Meally, D., and Georges, A. (2016). Sex Reversal in Reptiles: Reproductive Oddity or Powerful Driver of Evolutionary Change? Sex. Dev. 10, 279–287. doi:10.1159/000450972
Jeffries, D. L., Lavanchy, G., Sermier, R., Sredl, M. J., Miura, I., Borzée, A., et al. (2018). A Rapid Rate of Sex-Chromosome Turnover and Non-random Transitions in True Frogs. Nat. Commun. 9, 4088. doi:10.1038/s41467-018-06517-2
Kamiya, T., Kai, W., Tasumi, S., Oka, A., Matsunaga, T., Mizuno, N., et al. (2012). A Trans-species Missense SNP in Amhr2 Is Associated with Sex Determination in the Tiger Pufferfish, Takifugu rubripes (Fugu). Plos Genet. 8, e1002798. doi:10.1371/journal.pgen.1002798
Katoh, K., and Standley, D. M. (2013). MAFFT Multiple Sequence Alignment Software Version 7: Improvements in Performance and Usability. Mol. Biol. Evol. 30, 772–780. doi:10.1093/molbev/mst010
Kearse, M., Moir, R., Wilson, A., Stones-Havas, S., Cheung, M., Sturrock, S., et al. (2012). Geneious Basic: An Integrated and Extendable Desktop Software Platform for the Organization and Analysis of Sequence Data. Bioinformatics 28, 1647–1649. doi:10.1093/bioinformatics/bts199
Kirkpatrick, M. (2010). How and Why Chromosome Inversions Evolve. Plos Biol. 8, e1000501. doi:10.1371/journal.pbio.1000501
Kofler, R., Pandey, R. V., and Schlötterer, C. (2011). PoPoolation2: Identifying Differentiation between Populations Using Sequencing of Pooled DNA Samples (Pool-Seq). Bioinformatics 27, 3435–3436. doi:10.1093/bioinformatics/btr589
Koren, S., Walenz, B. P., Berlin, K., Miller, J. R., Bergman, N. H., and Phillippy, A. M. (2017). Canu: Scalable and Accurate Long-Read Assembly via Adaptive K-Mer Weighting and Repeat Separation. Genome Res. 27, 722–736. doi:10.1101/gr.215087.116
Lee, B.-Y., Penman, D. J., and Kocher, T. D. (2003). Identification of a Sex-Determining Region in Nile tilapia (Oreochromis niloticus ) Using Bulked Segregant Analysis. Anim. Genet. 34, 379–383. doi:10.1046/j.1365-2052.2003.01035.x
Li, H., and Durbin, R. (2009). Fast and Accurate Short Read Alignment with Burrows-Wheeler Transform. Bioinformatics 25, 1754–1760. doi:10.1093/bioinformatics/btp324
Li, H., Handsaker, B., Wysoker, A., Fennell, T., Ruan, J., Homer, N., et al. (2009). The Sequence Alignment/Map Format and SAMtools. Bioinformatics 25, 2078–2079. doi:10.1093/bioinformatics/btp352
Li, M., Sun, Y., Zhao, J., Shi, H., Zeng, S., Ye, K., et al. (2015). A Tandem Duplicate of Anti-müllerian Hormone with a Missense SNP on the Y Chromosome Is Essential for Male Sex Determination in Nile Tilapia, Oreochromis niloticus. Plos Genet. 11, e1005678. doi:10.1371/journal.pgen.1005678
Mank, J. E., and Avise, J. C. (2009). Evolutionary Diversity and Turn-Over of Sex Determination in Teleost Fishes. Sex. Dev. 3, 60–67. doi:10.1159/000223071
Matsuda, M., Nagahama, Y., Shinomiya, A., Sato, T., Matsuda, C., Kobayashi, T., et al. (2002). DMY Is a Y-specific DM-Domain Gene Required for Male Development in the Medaka Fish. Nature 417, 559–563. doi:10.1038/nature751
Meyer, B. S., Matschiner, M., and Salzburger, W. (2015). A Tribal Level Phylogeny of Lake Tanganyika Cichlid Fishes Based on a Genomic Multi-Marker Approach. Mol. Phylogenet. Evol. 83, 56–71. doi:10.1016/j.ympev.2014.10.009
Myosho, T., Otake, H., Masuyama, H., Matsuda, M., Kuroki, Y., Fujiyama, A., et al. (2012). Tracing the Emergence of a Novel Sex-Determining Gene in Medaka, Oryzias luzonensis. Genetics 191, 163–170. doi:10.1534/genetics.111.137497
Nanda, I., Kondo, M., Hornung, U., Asakawa, S., Winkler, C., Shimizu, A., et al. (2002). A Duplicated Copy of DMRT1 in the Sex-Determining Region of the Y Chromosome of the Medaka, Oryzias latipes. Proc. Natl. Acad. Sci. 99, 11778–11783. doi:10.1073/pnas.182314699
Narasimhan, V., Danecek, P., Scally, A., Xue, Y., Tyler-Smith, C., and Durbin, R. (2016). BCFtools/RoH: a Hidden Markov Model Approach for Detecting Autozygosity from Next-Generation Sequencing Data. Bioinformatics 32, 1749–1751. doi:10.1093/bioinformatics/btw044
Nguyen, L.-T., Schmidt, H. A., von Haeseler, A., and Minh, B. Q. (2015). IQ-TREE: A Fast and Effective Stochastic Algorithm for Estimating Maximum-Likelihood Phylogenies. Mol. Biol. Evol. 32, 268–274. doi:10.1093/molbev/msu300
Ortega-Recalde, O., Goikoetxea, A., Hore, T. A., Todd, E. V., and Gemmell, N. J. (2020). The Genetics and Epigenetics of Sex Change in Fish. Annu. Rev. Anim. Biosci. 8, 47–69. doi:10.1146/annurev-animal-021419-083634
Palaiokostas, C., Bekaert, M., Khan, M. G., Taggart, J. B., Gharbi, K., McAndrew, B. J., et al. (2015). A Novel Sex-Determining QTL in Nile tilapia (Oreochromis niloticus). BMC Genomics 16, 171. doi:10.1186/s12864-015-1383-x
Pan, Q., Feron, R., Yano, A., Guyomard, R., Jouanno, E., Vigouroux, E., et al. (2019). Identification of the Master Sex Determining Gene in Northern pike (Esox lucius) Reveals Restricted Sex Chromosome Differentiation. Plos Genet. 15, e1008013. doi:10.1371/journal.pgen.1008013
Payne, A. I., and Collinson, R. I. (1983). A Comparison of the Biological Characteristics of Sarotherodon Niloticus (L.) with Those of S. aureus (Steindachner) and Other tilapia of the delta and Lower Nile. Aquaculture 30, 335–351. doi:10.1016/0044-8486(83)90174-6
Philippe, H., Brinkmann, H., Lavrov, D. V., Littlewood, D. T. J., Manuel, M., Wörheide, G., et al. (2011). Resolving Difficult Phylogenetic Questions: Why More Sequences Are Not Enough. Plos Biol. 9, e1000602. doi:10.1371/journal.pbio.1000602
Rice, W. R. (1987). The Accumulation of Sexually Antagonistic Genes as a Selective Agent Promoting the Evolution of Reduced Recombination between Primitive Sex Chromosomes. Evolution 41, 911–914. doi:10.1111/j.1558-5646.1987.tb05864.x
Roberts, R. B., Ser, J. R., and Kocher, T. D. (2009). Sexual Conflict Resolved by Invasion of a Novel Sex Determiner in Lake Malawi Cichlid Fishes. Science 326, 998–1001. doi:10.1126/science.1174705
Rognon, X., and Guyomard, R. (2003). Large Extent of Mitochondrial DNA Transfer from Oreochromis aureus to O. niloticus in West Africa. Mol. Ecol. 12, 435–445. doi:10.1046/j.1365-294x.2003.01739.x
Sandve, S. R., Rohlfs, R. V., and Hvidsten, T. R. (2018). Subfunctionalization versus Neofunctionalization after Whole-Genome Duplication. Nat. Genet. 50, 908–909. doi:10.1038/s41588-018-0163-310.1038/s41588-018-0162-4
Sarder, M. R. I., Penman, D. J., Myers, J. M., and McAndrew, B. J. (1999). Production and Propagation of Fully Inbred Clonal Lines in the Nile tilapia (Oreochromis niloticus L.). J. Exp. Zool. 284 (6), 675–685. doi:10.1002/(SICI)1097-010X(19991101)284:6<675::AID-JEZ9>3.0.CO;2-D
Sember, A., Nguyen, P., Perez, M. F., Altmanová, M., Ráb, P., and Cioffi, M. d. B. (2021). Multiple Sex Chromosomes in Teleost Fishes from a Cytogenetic Perspective: State of the Art and Future Challenges. Phil. Trans. R. Soc. B 376 (1833), 20200098. doi:10.1098/rstb.2020.0098
Sinclair, A. H., Berta, P., Palmer, M. S., Hawkins, J. R., Griffiths, B. L., Smith, M. J., et al. (1990). A Gene from the Human Sex-Determining Region Encodes a Protein with Homology to a Conserved DNA-Binding Motif. Nature 346, 240–244. doi:10.1038/346240a0
Sissao, R., D’Cotta, H., Baroiller, J.-F., and Toguyeni, A. (2019). Mismatches between the Genetic and Phenotypic Sex in the Wild Kou Population of Nile tilapia Oreochromis niloticus. PeerJ 7, e7709. doi:10.7717/peerj.7709
Smith, C. A., Roeszler, K. N., Ohnesorg, T., Cummins, D. M., Farlie, P. G., Doran, T. J., et al. (2009). The Avian Z-Linked Gene DMRT1 Is Required for Male Sex Determination in the Chicken. Nature 461, 267–271. doi:10.1038/nature08298
Syaifudin, M., Bekaert, M., Taggart, J. B., Bartie, K. L., Wehner, S., Palaiokostas, C., et al. (2019). Species-Specific Marker Discovery in Tilapia. Sci. Rep. 9, 13001. doi:10.1038/s41598-019-48339-2
Takehana, Y., Hamaguchi, S., and Sakaizumi, M. (2008). Different Origins of ZZ/ZW Sex Chromosomes in Closely Related Medaka Fishes, Oryzias javanicus and O. Hubbsi. Chromosome Res. 16, 801–811. doi:10.1007/s10577-008-1227-5
Takehana, Y., Matsuda, M., Myosho, T., Suster, M. L., Kawakami, K., Shin-I, T., et al. (2014). Co-option of Sox3 as the Male-Determining Factor on the Y Chromosome in the Fish Oryzias Dancena. Nat. Commun. 5, 4157. doi:10.1038/ncomms5157
Tao, W., Conte, M. A., Wang, D., and Kocher, T. D. (2021). Network Architecture and Sex Chromosome Turnovers. BioEssays 43, 2000161. doi:10.1002/bies.202000161
Taslima, K., Khan, M. G. Q., McAndrew, B. J., and Penman, D. J. (2021). Evidence of Two XX/XY Sex-Determining Loci in the Stirling Stock of Nile tilapia (Oreochromis niloticus). Aquaculture 532, 735995. doi:10.1016/j.aquaculture.2020.735995
Taslima, K., Wehner, S., Taggart, J. B., de Verdal, H., Benzie, J. A. H., Bekaert, M., et al. (2020). Sex Determination in the GIFT Strain of tilapia Is Controlled by a Locus in Linkage Group 23. BMC Genet. 21, 49. doi:10.1186/s12863-020-00853-3
Trewavas, E. (1983). Tilapiine Fishes of the Genera Sarotherodon, Oreochromis and Danakilia. London, UK: British Museum Natural History.
Triay, C., Conte, M. A., Baroiller, J.-F., Bezault, E., Clark, F. E., Penman, D. J., et al. (2020). Structure and Sequence of the Sex Determining Locus in Two Wild Populations of Nile Tilapia. Genes 11, 1017. doi:10.3390/genes11091017
Turner, S. D. (2018). Qqman: An R Package for Visualizing GWAS Results Using Q-Q and manhattan Plots. JOSS 3, 731. doi:10.21105/joss.00731
van Doorn, G. S., and Kirkpatrick, M. (2010). Transitions between Male and Female Heterogamety Caused by Sex-Antagonistic Selection. Genetics 186, 629–645. doi:10.1534/genetics.110.118596
Wessels, S., and Hörstgen-Schwark, G. (2007). Selection Experiments to Increase the Proportion of Males in Nile tilapia (Oreochromis niloticus) by Means of Temperature Treatment. Aquaculture 272, S80–S87. doi:10.1016/j.aquaculture.2007.08.009
Wessels, S., and Hörstgen-Schwark, G. (2011). Temperature Dependent Sex Ratios in Selected Lines and Crosses with a YY-Male in Nile tilapia (Oreochromis niloticus). Aquaculture 318, 79–84. doi:10.1016/j.aquaculture.2011.04.039
Wessels, S., Krause, I., Floren, C., Schütz, E., Beck, J., and Knorr, C. (2017). ddRADseq Reveals Determinants for Temperature-dependent Sex Reversal in Nile tilapia on LG23. BMC Genomics 18, 531. doi:10.1186/s12864-017-3930-0
Wessels, S., Sharifi, R. A., Luehmann, L. M., Rueangsri, S., Krause, I., Pach, S., et al. (2014). Allelic Variant in the Anti-müllerian Hormone Gene Leads to Autosomal and Temperature-dependent Sex Reversal in a Selected Nile Tilapia Line. PLoS One 9, e104795. doi:10.1371/journal.pone.0104795
Wheeler, T. J., and Eddy, S. R. (2013). Nhmmer: DNA Homology Search with Profile HMMs. Bioinformatics 29, 2487–2489. doi:10.1093/bioinformatics/btt403
Wilson, C. A., High, S. K., McCluskey, B. M., Amores, A., Yan, Y.-l., Titus, T. A., et al. (2014). Wild Sex in Zebrafish: Loss of the Natural Sex Determinant in Domesticated Strains. Genetics 198, 1291–1308. doi:10.1534/genetics.114.169284
Xiao, J., Zhong, H., Liu, Z., Yu, F., Luo, Y., Gan, X., et al. (2015). Transcriptome Analysis Revealed Positive Selection of Immune-Related Genes in tilapia. Fish Shellfish Immunol. 44, 60–65. doi:10.1016/j.fsi.2015.01.022
Keywords: sex chromosome, sex determination, Y-haplotype, AMH, duplication, populations
Citation: Triay C, Courcelle M, Caminade P, Bezault E, Baroiller J-F, Kocher TD and D’Cotta H (2022) Polymorphism of Sex Determination Amongst Wild Populations Suggests its Rapid Turnover Within the Nile Tilapia Species. Front. Genet. 13:820772. doi: 10.3389/fgene.2022.820772
Received: 23 November 2021; Accepted: 07 February 2022;
Published: 17 May 2022.
Edited by:
Mateus Contar Adolfi, Julius Maximilian University of Würzburg, GermanyReviewed by:
Marcelo De Bello Cioffi, Federal University of São Carlos, BrazilCopyright © 2022 Triay, Courcelle, Caminade, Bezault, Baroiller, Kocher and D’Cotta. This is an open-access article distributed under the terms of the Creative Commons Attribution License (CC BY). The use, distribution or reproduction in other forums is permitted, provided the original author(s) and the copyright owner(s) are credited and that the original publication in this journal is cited, in accordance with accepted academic practice. No use, distribution or reproduction is permitted which does not comply with these terms.
*Correspondence: Helena D’Cotta, ZGNvdHRhQGNpcmFkLmZy
Disclaimer: All claims expressed in this article are solely those of the authors and do not necessarily represent those of their affiliated organizations, or those of the publisher, the editors and the reviewers. Any product that may be evaluated in this article or claim that may be made by its manufacturer is not guaranteed or endorsed by the publisher.
Research integrity at Frontiers
Learn more about the work of our research integrity team to safeguard the quality of each article we publish.