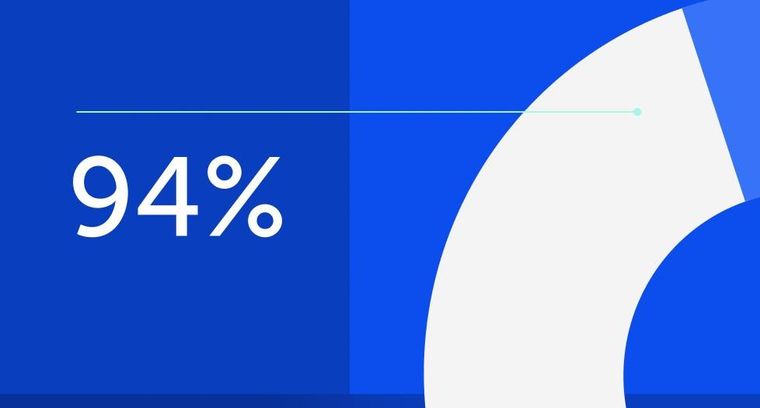
94% of researchers rate our articles as excellent or good
Learn more about the work of our research integrity team to safeguard the quality of each article we publish.
Find out more
REVIEW article
Front. Genet., 19 May 2022
Sec. Evolutionary and Population Genetics
Volume 13 - 2022 | https://doi.org/10.3389/fgene.2022.820442
This article is part of the Research TopicAquatic Genomics and Transcriptomics for Evolutionary BiologyView all 9 articles
As an important hormone, melatonin participates in endocrine regulation of diverse functions in vertebrates. Its biosynthesis is catalyzed by four cascaded enzymes, among them, arylalkylamine N-acetyltransferase (AANAT) is the most critical one. Although only single aanat gene has been identified in most groups of vertebrates, researchers including us have determined that fish have the most diverse of aanat genes (aanat1a, aanat1b, and aanat2), playing various potential roles such as seasonal migration, amphibious aerial vision, and cave or deep-sea adaptation. With the rapid development of genome and transcriptome sequencing, more and more putative sequences of fish aanat genes are going to be available. Related phylogeny and functional investigations will enrich our understanding of AANAT functions in various fish species.
Arylalkylamine N-acetyltransferase (AANAT), also known as serotonin N-acetyltransferase (SNAT or NAT), belongs to the GCN5-related N-acetyltransferase (GNAT) superfamily. It is an acetyl-CoA-dependent enzyme (Wolf et al., 2002) and catalyzes the transfer of the acetyl group in acetyl-CoA to an arylalkylamine. The common AANAT arylalkylamine substrates are serotonin and dopamine (Figure 1A). AANATs play differential functions in various groups of organisms. In insects, multiple AANATs have evolved, particularly in mosquitoes (Han et al., 2012). Insect AANATs are mainly involved in cuticle formation, pigmentation, and some bioamine neurotransmitters and fatty acid amide metabolism, as well as circadian rhythms (O’Flynn et al., 2018; Zhang et al., 2019; Kamruzzaman et al., 2021; Zhang K. et al., 2021; Zhang et al., 2022). In vertebrates, AANATs play differential functions mainly through a small molecule, melatonin (N-acetyl-5-methoxytryptamine; Figure 1).
FIGURE 1. The substrates of AANAT and one of its products (melatonin; Mel) synthesized in fish. (A) AANAT can catalyze arylalkylamines, including phenylethylamines (dopamine, octopamine, tyramine, and phenylethylamine) and indolethylamines (serotonin and tryptamine). (B) The fish pineal organ. Upper left: the depigmented area around the pineal window (circle) of a polar cod (Boreogadus saida) head (adopted from Falcón et al., 2011). Upper right: zebrafish pineal gland with enhanced green fluorescent protein (EGFP) expressed under the control of aanat2 promoter (Ben-Moshe et al., 2014). Down: melatonin synthesis in the fish pineal gland through regulation of light. (C) Melatonin biosynthesis pathway and related enzymes including AANAT. (D) Multiple important functions of melatonin in various fishes.
As one of the important products of the AANAT enzyme (Figure 1C), melatonin participates in the regulation of animal behaviors and physiology such as reproduction, growth, and immunity (Shi, 2005; Coon and Klein, 2006; Li et al., 2015; Kuz’mina, 2020). Initially isolated from bovine pineal gland (Lerner et al., 1958), melatonin was subsequently identified in humans (Brzezinski, 1997) and other vertebrates including fish (Esteban et al., 2013). It has also been detected in various taxa of invertebrates (Vivien-Roels and Pévet, 1993) and even in plants, fungi (Rodriguez-Naranjo et al., 2012), and bacteria (Tilden et al., 1997), although it may perform differential functions in various groups of organisms (Zhao et al., 2019).
Melatonin works as the “gear” of the biological clocks in animals, which are influenced by light/dark or temperature changes in a timescale of day (circadian rhythms) or year (circannual rhythms) (Zhao et al., 2019). In fish, the regulation of melatonin is usually through the eye and depigmented pineal window in response to light (Figure 1B). Therefore, melatonin was previously reported to be mainly synthesized in pinealocytes and then released into the bloodstream to reach central and peripheral organs for diverse roles. However, recent reports indicate that a majority of melatonin is synthesized in other tissues such as gut and skin rather than the pineal gland (Yasmin et al., 2021; Sevilla et al., 2022), suggesting that it can also regulate biological activities by binding to its specific receptors in target tissues (Shi et al., 2004; Isorna et al., 2009; Kuz’mina, 2020).
In fish and other animals, melatonin is synthesized from the precursor tryptophan (Trp) through four enzyme-catalyzed reactions (Figure 1C). The first step is catalyzed by tryptophan hydroxylase (TPH), converting Trp to 5-hydroxytryptophan (HTrp) (Xu et al., 2019), followed by the action of aromatic L-amino acid decarboxylase (AAAD), changing HTrp to serotonin (5-HT) (Li et al., 2018). The third step acetylates 5-HT to N-acetylserotonin (NAS) by arylalkylamine N-acetyltransferase (AANAT) (Li et al., 2015), and the final synthesis of melatonin is catalyzed by acetylserotonin-O-methyltransferase (ASMT) (Zhang et al., 2017).
During these four steps, AANAT is the penultimate enzyme in the melatonin biosynthesis pathway (Falcón et al., 2010) that is well conserved through evolution in various vertebrates (Li et al., 2015). It has a structurally conserved fold consisting of an eight-stranded β sheet flanked by five α helices, and also shares four conserved motifs designated A–D (Hickman et al., 1999), among which motif B contributes acidic residues to the serotonin binding slot (Wolf et al., 2002).
It has been previously reported that AANAT catalyzes the rate-limiting step in animal melatonin biosynthesis (Foulkes et al., 1996). However, many other reports strongly suggest that, rather than AANAT, the last enzyme ASMT might also control melatonin production (Liu and Borjigin, 2005; Chattoraj et al., 2009). Instead, AANAT should be regarded as the “melatonin rhythm-generating enzyme”, because its large nocturnal increase in activity drives the daily rhythm in melatonin secretion (Figure 1C) (Chattoraj et al., 2009). In specific, expression of aanat genes is driven directly by the circadian clock when night falls, followed by phosphorylation-dependent activation by cyclic-AMP-dependent activation of protein kinase A (PKA), and protection by binding to 14-3-3 proteins, resulting in increased melatonin production. In contrast, light during the daytime disrupts the AANAT/14-3-3 complex, leading to proteolytic degradation of the enzyme and suppression of melatonin synthesis (Coon et al., 2001; Falcón et al., 2010; Zhang L. et al., 2021).
This review summarizes the evolution and functions of AANAT in various fishes in a genomic perspective. In more detail, we first discuss the origin and copy number variations (CNVs) of aanat genes among various species. Next, AANAT sequence diversity and evolution in fish are presented. We further introduce its transcription regulations and expression patterns in several representative species. At last, we review its important physiological roles (mainly through melatonin), especially potential new roles of newly evolved aanat duplicates due to gene duplication or whole genome duplication (WGD) to see whether they are shared by teleosts or have occurred independently in specific lineages (Zilberman-Peled et al., 2006).
AANAT is classified into two subfamilies, termed non-vertebrate (NV-) AANAT and vertebrate (VT-) AANAT (Falcón et al., 2014); that is to say, NV-AANAT is distributed in non-vertebrate species including cephalochordates, some lower plants and bacteria, while VT-AANAT has been identified in vertebrates such as fish and tetrapods. It is estimated that VT-AANAT evolved from the ancestral NV-AANAT (Falcón et al., 2014). However, these two groups of AANAT proteins show dramatic differences in regulatory and catalytic regions, thus playing totally different metabolic roles (Coon and Klein, 2006; Klein, 2006). This is consistent with a previous hypothesis that vertebrate AANAT was acquired by horizontal gene transfer (Iyer et al., 2004). The questions of when and how VT-AANAT evolved in vertebrates were partly addressed by the identification of the gene in representatives of early divergent vertebrates. First, NV-AANAT had been identified in Cephalochordata (Pavlicek et al., 2010). Then, the first appearance of VT-AANAT was in early vertebrate lineages, in both Agnathans (jawless fish) and Chondrichthyes (cartilaginous fish), suggesting a duplication of the ancestral NV-AANAT through vertebrate genome duplication (VGD) (Falcón et al., 2014). Subsequently, Agnatha and Teleostomi (jawed vertebrates) lost NV-AANAT independently, with only VT-AANAT in these groups (Falcón et al., 2014). However, some taxa within Chondrichthyes keep both NV- and VT-AANATs (Falcón et al., 2014). These findings strongly support the origin of VT-AANAT from the ancestral NV-AANAT. Since the most recent common ancestor of Agnathans and Chondrichthyes dated back to 500 million years ago (Mya) (Inoue et al., 2010), it is estimated that VT-AANAT in vertebrates may originate in the early Cambrian period, before or concomitant with the emergence of lateral eyes and the pineal gland (Falcón et al., 2014).
Because of the two rounds of VGDs shared among vertebrates, the ancestral vertebrates evolved into two copies of aanat genes named VT-aanat1 and VT-aanat2 (Falcón et al., 2014). However, only one of the duplicates was retained in tetrapods, while cases in fish are more complicated. Besides the VGDs, fish have an additional teleost-specific genome duplication (TGD, third-round WGD, or 3R) that occurred at the root of the teleost lineage at 320 Mya (Opazo et al., 2012; Glasauer and Neuhauss, 2014). Therefore, while losing the NV-AANAT and retaining both VT-AANATs, teleosts generated another copy of VT-aanat1 but kept only one VT-aanat2, resulting in three VT-aanat genes (namely VT-aanat1a, VT-aanat1b, and VT-aanat2) in most diploid fish (Li et al., 2015).
Moreover, some fish groups such as sturgeons (Cheng et al., 2019; Cheng et al., 2020), carps (Xu et al., 2014), and salmons (Lien et al., 2016), are prone to polyploidization, which means that they have experienced the fourth or more rounds of WGD (Zhou and Gui, 2017). Lineage-specific WGDs in these groups provide new genetic resources to generate new VT-AANAT duplicates. We (Li et al., 2015) found that tetraploid Atlantic salmon (Salmo salar) owns two VT-aanat1b (termed VT-aanat1b1 and VT-aanat1b2) and two VT-aanat2 (termed VT-aanat2a and VT-aanat2b). So does the tetraploid rainbow trout (Oncorhynchus mykiss) (Li et al., 2015). Nevertheless, no comprehensive study has been conducted on such a topic so far. It is predictable that the origins of each of the newly evolved genes could date back to the time of each independent WGD that occurred in the corresponding group or species. Another way for the origin of new fish aanat genes is through tandem gene duplication, which is the case for aanat2 in Amazon molly (Li et al., 2015), but this is the only report to date.
As discussed previously, the copy number of aanat in fish is closely related to the species ploidy. WGDs increase this number, while subsequent gene loss events decrease the total (Li et al., 2015). For quite a long time, only single aanat genes had been identified in birds, reptiles, and mammals except for some lineages in cetartiodacyl clade (Yin D. et al., 2021). Coon et al. (1999) firstly reported two aanat genes (aanat1 and aanat2) in fish, and all the previously known AANATs belong to the AANAT1 subfamily (Coon et al., 1999). In 2006, Coon and others performed genome analysis of four teleost species, revealing the presence of three aanat genes, corresponding to aanat1a, aanat1b, and aanat2 (Coon and Klein, 2006). Since then, along with the big discovery of TGD shared by all teleosts (Taylor et al., 2003), it comes to a consensus that fish have three aanat genes (Figure 2; Cazaméa-Catalan et al., 2014).
FIGURE 2. Phylogenetics of fish aanat genes. (A) The phylogenetic model of aanats. aanat genes from the same species are marked as the same color and shape. Red, purple, and pink dots represent vertebrate, teleost-specific, and lineage-specific genome duplication events, respectively. Red crosses mark the loss of aanat in corresponding clades. (B) Syntenic relationship among aanats in representative species. Twenty genes at the up-stream and down-stream around aanats were chosen to establish the syntenic relationship. Blue (+) and green (-) boxes represent genes with different coding directions, and the brown synteny blocks along various species indicate the aanat genes.
Afterward, with the dramatic increase in the published number of fish genomes, the aanat content in many more species have been studied. In 2015, we conducted a comprehensive analysis of the fish aanat genes at a genome level (Li et al., 2015). In this report, a total of 84 aanat genes were predicted from 37 vertebrate species, including 24 ray-finned fishes. The copy number of aanat genes in all these examined species ranges from two to five (Table 1), but most fishes have the representative number of three (Li et al., 2015).
Species with three aanat genes are usually diploids, but several other diploid fish have lost one of the aanat1 genes randomly, reducing the copy number to two. Atlantic cod and Mexican tetra, for instance, have lost aanat1a and aanat1b, respectively (Li et al., 2015). The loss of aanat2 has not been identified in any fish genomes so far (Lv et al., 2020), suggesting a highly conserved function of this isotype (Zilberman-Peled et al., 2011).
Several tetraploids, such as the Chinese golden-line fish, also have three aanat genes (one aanat1a and two aanat2). They lost aanat1b originated from TGD (Li et al., 2015) and duplicated only aanat2 during a Sinocyclocheilus-specific WGD event (Yang et al., 2016). Another tetraploid, Amazon molly, retains all three aanat copies as in diploids, but has evolved into another aanat2 that was possibly generated by tandem gene duplication instead of WGD, thereby increasing the total number to four. While both rainbow trout and Atlantic salmon have five aanat genes because of the salmonid-specific WGD (Ss4R), with one more aanat1b than Amazon molly, interestingly, no fish has six or more aanat genes to date, but it might be possible in hexaploid and more polyploid fishes such as sturgeons (Ludwig et al., 2001; Cheng et al., 2019) that are under in-depth investigations.
Presumably, CNVs of aanat in fish are a combined result of gene duplication and WGD followed by gene loss (Falcón et al., 2014), while some fish or groups have their own preferences on which aanat duplicate is to be retained and which one is to be lost. In ray-fins, aanat2 seems to be essential and is retained in all genomes sequenced so far (Table 1), although it may be inactivated due to frameshift mutations in certain species like deep-sea snailfish (Lv et al., 2020; Mu et al., 2021). Amphibious giant-fin mudskipper has lost aanat1a (You et al., 2014), whereas Ostariophysi group has lost aanat1b (Li et al., 2015). Such a genetic diversity in various fishes suggests that different isoforms of AANAT may have discrepant functions (Zilberman-Peled et al., 2011).
A phylogenetic tree based on coding sequences divides vertebrate aanat genes into two main groups by using lamprey (Petromyzon marinus) as the outgroup (Figure 2). One group contains tetrapod aanat and teleost aanat1 (aanat1a and aanat1b), and the other is composed of teleost aanat2. Such a consistent topology supports a closer relationship between tetrapod aanat and teleost aanat1, suggesting that the newly evolved aanat2 through VGDs in the ancestral vertebrates (Falcón et al., 2014) was lost immediately in tetrapods. In addition, the fish aanat1 clade was further divided into two groups of aanat1a and aanat1b, possibly as a consequence of TGD (Li et al., 2015).
After being separated from tetrapod aanat, teleost aanat1 is divided into two subgroups as well, namely aanat1a and aanat1b (Figure 2). Within each subgroup, the gene tree is usually consistent with the species tree, except for few conflicting nodes. As members in Ostariophysi, Mexican tetra, zebrafish, and three Chinese golden-line barbels have only one aanat1 closely related to aanat1b in the phylogenetic tree, but synteny analysis strongly support the Ostariophysi aanat1 gene to be aanat1a (Li et al., 2015). Similarly, aanat2 of Ostariophysi, together with the Asian arowana, forms a sister-clade to all other fish aanat2 genes. This phenomenon of such a special clade formed by Ostariophysi aanat genes has been discussed previously (Li et al., 2015), but the detailed reasons and the evolutionary importance behind this phenomenon still needs more investigations.
Fish aanat genes and their corresponding paralogs originating from lineage-specific WGDs after TGD always group together, although some nodes are not well resolved. For example, Ss4R occurred in the common ancestor of salmonids nearly 80 Mya, resulting in the acquisition of an additional aanat1b in both rainbow trout and Atlantic salmon (Berthelot et al., 2014; Macqueen and Johnston, 2014; Lien et al., 2016). The four aanat1b genes form a sister-clade to the pike aanat1b as expected, but within this clade, the newly evolved paralogs and the original copies are not sisters to each other. For another instance, six aanat2 genes (two from each of the three species) diverge to form two clades showing different topologies. One clade consists of the original gene directly descended from a vertebrate ancestor, while the other is duplicated via the recent Sinocyclocheilus-specific WGD (Yang et al., 2016). With the sequencing of more fish genomes and identification of more aanat genes, the evolution of aanat in various fishes and its relationship with WGD can be better resolved and illustrated.
An explosive acceleration of evolution has been detected in the stem of the VT-AANAT subfamily after splitting from NV-AANAT, which was presumably associated with the functional shift to melatonin synthesis (Falcón et al., 2014). However, the calculation of evolutionary rates has not yet been applied to fish aanat genes specifically. It is still unclear that how fast aanat genes have evolved following TGD and multiple rounds of WGD afterward in teleosts.
Encoding regions of fish aanat genes are relatively conserved with high similarities to other vertebrates (Pavlicek et al., 2010; Falcón et al., 2014), consisting of three exons ranging from 150 to 300 bp. The third exon of aanat1b is usually longer, increasing the length to over 400 bp. Therefore, the amino acid (aa) sequence-coded by each exon consists of about 50–100 residues individually, while the last exon of AANAT1b encodes 35 more residues (Figure 3).
FIGURE 3. Sequence alignment of AANAT proteins. Conserved motifs and secondary structures of alpha helix (α) and beta strand (β) are shown. Catalytic residues (red) and substrate binding sites (green) are marked with asterisks. BP (Boleophthalmus pectinirostris) and PM (Periophthalmus magnuspinnatus) are two representative amphibious mudskippers (You et al., 2014).
As a conserved catalytic enzyme, AANAT has a high sequence identity of nearly 60–97% among ten studied vertebrates including sharks, fishes, reptiles, and mammals (Li et al., 2015). Within fish, the identity is even higher. Specifically, fish AANAT1a proteins are the most conserved with a minimum identity of 88%, followed by AANAT2 (81%), while AANAT1b is the most variable (71%) among vertebrate species (Li et al., 2015). Therefore, AANAT proteins have been highly conserved throughout fish evolution, indicating that they have been maintained by natural selection for important biological functions in various fishes (Kuz’mina, 2020).
AANAT is also structurally conserved (Figure 4). Previous reports have shown that VT-AANAT has a conserved fold consisting of about eight β sheets flanked by five α helices, and shares four conserved GNAT family motifs designated A-D (Hickman et al., 1999), among which motif B contributes acidic residues to the serotonin binding slot (Wolf et al., 2002). Take the human AANAT (207 aa in length; UniProt accession Q16613; Figure 4A) as an example, there are a conserved N-acetyltransferase domain (35–194), three acetyl-CoA binding regions (124–126, 132–137, and 168–170), two catalytic histidine residues (His120 and His122), and multiple substrate binding sites (Leu124 via amide nitrogen, and Met159 via carbonyloxygen; Figures 3,4) (Scheibner et al., 2002; Wolf et al., 2002).
FIGURE 4. Structures of human (validated) and zebrafish (predicted by AlphaFold) AANATs. (A) X-ray structure of human AANAT bound to bisubstrate analog, highlighting the four GNAT family motifs (marked as motifs A–D), and catalytic histidine (H120 and H122) residues. It was adopted from Scheibner et al. (2002). (B) Zebrafish AANAT1 (UniProt accession Q6V7J8). (C) Zebrafish AANAT2 (UniProt accession Q9PVD7).
The structures of fish AANATs predicted by AlphaFold (Jumper et al., 2021) are very similar to human AANAT, especially in those conserved motifs and residues (Figures 3, 4B,C). All examined fishes share with humans the same four GNAT family motifs, the three acetyl-CoA binding regions, the two catalytic histidine sites, and most of the substrate binding sites in their AANATs (Figures 3,4). As shown previously, fish AANAT1 is closer to tetraploid AANAT and has fewer variants in comparison to fish AANAT2. Multiple substrate binding sites (T109, M159, and L196) have been mutated to other amino acids in AANAT2 but remain the same in AANAT1.
Mutations of some amino acid sites have been observed, such as F130C and V153L in AANAT2 (Li et al., 2015). Some mutations are found in both fish and humans. The most interesting one is the alternative of Ala129 to Tyr in AANAT1 and to His in AANAT2. This site has been recognized as a single-nucleotide polymorphism (SNP) in humans, showing that individuals with the natural variant A129T have delayed sleep phase syndrome (Hohjoh et al., 2003). Although the impact of such a mutation in fish has not been examined, it is possible that this change might have something to do with the sleep pattern difference between humans and fish.
A newly arisen duplicate of any gene has three possible fates, being lost (including being silenced by degenerative mutations), subfunctionalization or neofunctionalization (Lynch et al., 2001), and the retained duplicate genes may also alter their expression pattern via cooperation (True and Carroll, 2002). Multiple aanat genes in teleosts displaying different expression patterns (Figure 5) is a good example of such a process.
FIGURE 5. Endogenous sources of melatonin and aanat expression in various fishes, including carp (Catla; Maitra and Pal, 2017) and three-spined stickleback (Gasterosteus aculeatus; Kulczykowska et al., 2017). Detection of aanat mRNA in different tissues is according to the references. aanat with a red box marks its existence in the corresponding tissue of the stickleback, aanat with a green box refers to its existence in the catla carp, while those with a yellow box means aanat(s) expressed both in stickleback and catla. “Other species” are the goldlined spinefoot (Siganus guttatus), rainbow trout (Oncorhynchus mykiss), goldfish (Carassius auratus), and sea bass (Dicentrarchus labrax) (Kulczykowska et al., 2017).
The single human aanat is mainly expressed in the pineal gland and retina (Coon et al., 1996), but it has also been identified in other tissues such as testis (Albrecht, 2009) and skin (Slominski et al., 2002). In fish, the two types of aanat display tissue specific distribution. Expression of aanat1 (both aanat1a and aanat1b) have been detected mainly in the retina and brain, while aanat2 is reported to be specifically expressed in the pineal gland, despite that, positive detection of aanat1a and aanat1b has also been observed in dorsal sac (surrounding the pineal organ) (Isorna et al., 2009; Isorna et al., 2011; Paulin et al., 2015). However, the expression of aanat genes has also been detected in other fish tissues, such as gill, kidney, liver, spleen, skin, gonad, and gut (Figure 5) (Fernández-Durán et al., 2007; Velarde et al., 2010; Falcón et al., 2011; Nisembaum et al., 2013; Sanjita Devi et al., 2016; Maitra and Pal, 2017; Kulczykowska et al., 2017; Kuz’mina, 2020).
Although both aanat1a and aanat1b are mainly expressed in retina and brain, their abundance, day-night or lifespan expression patterns have been reported to be distinctly different (Isorna et al., 2011). In flatfish (Solea senegalensis), aanat1a has a lower expression level than aanat1b in the retina of adults. Expression of aanat1a was more abundant during the early than late larval stages, while aanat1b expression was low during early developing stages but rose significantly throughout metamorphosis (Isorna et al., 2011). Furthermore, the expression levels of the two aanat1 genes also differ in outer nuclear (ONL) and inner nuclear (INL) layers of retina, and in the ganglion cell layers (GCL) (Isorna et al., 2011; Paulin et al., 2015) (Figure 6B).
FIGURE 6. Detection of aanat (aanat1a, aanat1b, and aanat2) transcriptions in fish pineal gland (A) and retina (B) (adopted from Paulin et al., 2015). (A) Pinealocytes (arrows) are labeled with the antisense aanat2 probe, but not with either aanat1a or aanat1b probes. Cells of dorsal sac (arrow heads) are stained with aanat1b antisense probes but not with aanat2 probe. ds: dorsal sac; P: pineal gland. (B) Photoreceptor cells of the ONL are stained (double white arrow). Bipolar (arrow heads) and amacrine (upward red arrow) cells in the INL are also labeled. A few cells are labeled in the GCL (downward red arrow) and RPE (downward yellow arrows). GCL: ganglion cell layer; INL: inner nuclear layer; IPL: inner plexiform layer; ONL: outer nuclear layer; OPL: outer plexiform layer; RPE: retinal pigment epithelium.
The notable differences of expression patterns in the tissue distribution, abundance, and the day-night rhythm during development stages throughout their lifespan suggest different functions for the three fish AANAT enzymes (Klein, 2007; Paulin et al., 2015). Further investigations are needed to pay attention to their functional characteristics, and to elucidate their biological roles in regulating the biosynthesis of melatonin, dopamine, and other potential metabolites.
Where and how the three fish AANAT enzymes being expressed strongly suggest that they have various functions. Pineal AANAT2 functions mainly in the melatonin biosynthesis and prefers indolylethylamine over phenylethylamine as its substrate (Paulin et al., 2015). It always follows a day–night rhythm (Zilberman-Peled et al., 2007) and sometimes exhibits a temperature dependency (Cazaméa-Catalan et al., 2012; Paulin et al., 2015). However, the role of retina AANAT1s is less apparent. Having similar affinity for both phenylethylamines and indolylethylamines, AANAT1 enzymes seem to have a broader range of functions in addition to catalyzing the synthesis of melatonin as AANAT2 does. They are also reported to be involved in the catabolism of serotonin and dopamine (Falcón et al., 2010; Paulin et al., 2015; Maitra and Pal, 2017).
There are accumulated proofs that fish AANAT enzymes have more functions than just being the “timezyme” for synthesizing the time-measuring hormone melatonin (Klein, 2007). More roles such as detoxification (Besseau et al., 2006; Zilberman-Peled et al., 2011) and neurotransmission (Zilberman-Peled et al., 2006) have already been discussed before, and new functions might be discovered with increasing fish genomic data (Sun et al., 2016; Fan et al., 2020) and verification experiments, especially in fishes with multiple copies of AANATs (Li et al., 2015). More studies are necessary to determine why some fish keep more AANATs while other species lose one or more aanat genes (Table 1). Answers to what roles the additional AANATs play and what impacts the gene loss will be valuable for an in-depth understanding of fish AANATs. Here, we provide a summary of multiple important physiological roles of AANATs and melatonin in various fishes.
Juvenile chum salmons (Oncorhynchus keta) in the rivers of Hokkaido in Northern Japan usually initiate their catadromous migration around March to April each year (at about 100 days after hatching), when the river and shallow-sea surface ices are almost melted. In 2004, we cloned two aanats (aanat1 and aanat2) and two melatonin receptor genes (mel1a and mel1b) in chum salmon, and measured melatonin levels as well as mRNA levels of the four genes in the eye and brain during embryonic and post-embryonic stages (Shi et al., 2004).
The study showed that shortly before the spring season, aanat mRNAs and melatonin levels in the eye and brain of these pre-spawning chum salmons had been elevated to peak values (Figure 7), suggesting that these parameters are important signals for seasonal migration of chum salmon (Shi et al., 2004). The obvious parallelism in developmental changes and circadian rhythms of aanat mRNAs and melatonin levels supports the popular hypothesis that the developmental increases of nocturnal melatonin levels are in part a consequence of the elevated transcription of pineal aanat genes (Shi et al., 2004; Shi, 2005).
FIGURE 7. Transcription of aanat1 and aanat2 mRNAs in the eye (A–C) and brain (B–D) of chum salmon (adopted from Shi et al., 2004). Juveniles begin to migrate downstream from day +100 (boxed). Duncan’s multiple range test (different letters show p < 0.05) and Student’s t test (*, p < 0.05; **, p < 0.01; ***, and p < 0.001) were used to compare the significance between mid-light or mid-dark values at different developmental stages and within the same date, respectively.
Moreover, abundant aanat and mel-R mRNAs in various brain regions and eyes indicates potential roles of melatonin in visual processing and neuroendocrine regulation, through which melatonin might be involved in migratory behaviors of chum salmon (Shi et al., 2004). Interestingly, this report tried to separate aanat into aanat-pineal and aanat-retina, which were renamed formally as aanat1a and aanat1b since 2006 (Coon and Klein, 2006).
Mudskippers are the biggest group of amphibious fishes with a division of four main genera, including Boleophthalmus, Periophthalmodon, Periophthalmus, and Scartelaos. They represent a continuum of adaptations toward terrestrial life, with some species being more terrestrial than the others. In 2014, we published the first genome article (You et al., 2014) of the four representative species of mudskippers, mainly focusing on the blue spotted mudskipper (B. pectinirostris; BP) and giant-fin mudskipper (Periophthalmus magnuspinnatus; PM). Comparative genomics analyses were performed to provide novel insights into the genetic basis of terrestrial adaptation in these mudskippers.
Usually, fully aquatic fishes are likely to somehow have myopic vision in an air condition (You et al., 2014; You et al., 2018). However, mudskippers seem to have good aerial vision due to their strong ability to avoid terrestrial predators. Comparison of vision-related genes in the two representative mudskippers (BP and PM) and several vertebrates highlighted certain adaptive losses or mutations in mudskippers (You et al., 2014).
With the detailed genomic data, we noted that BP contains all the three aanat genes whereas PM possesses only aanat1b and aanat2. The existence of aanat1b was confirmed by abundant reads mapping to the gene locus. In contrast, no PM reads could be mapped to the aanat1a sequence of BP, suggesting that PM may have lost the aanat1a gene. In fact, dopamine acetylation is a novel function of fish AANAT1a in retinae (Zilberman-Peled et al., 2006), which has been proposed to cause low retinal-dopamine levels progressively leading to myopia (Feldkaemper and Schaeffel, 2013). We therefore speculate that the loss of aanat1a in PM may have generated an elevation status of retina dopamine levels in the occurrence of myopia, which would facilitate aerial vision for a selective advantage in PM so as to spend most (over two-third) of its lifetime on an intertidal mudflat surface (You et al., 2014; You et al., 2018).
Cavefishes have often developed degenerated features, such as rudimentary eyes or scales, and loss of pigmentation. As potential compensation, some more sensitive traits have evolved in these fish, such as elongated appendages and non-visual positioning or sensory systems (Yang et al., 2016).
Sinocyclocheilus is endemic to China’s Qinghai Tibetan Plateau. This genus of over 75 species is a good cavefish model due to its high species diversity and phenotypic variations. In 2013, a Science letter reported over 150 naturally caved species in southwestern China, which were unknown before (Shu et al., 2013). Subsequently, genomes of three Sinocyclocheilus species were published (Yang et al., 2016; Yin Y. H. et al., 2021), including surface-dwelling S. grahami (Sg), semi-cave-dwelling S. rhinocerous (Sr), and cave-restricted S. anshuiensis (Sa). They are representatives of three key nodes on the path to a cave life. Interestingly, Sa has sometimes lost its external eyeballs and lens, and its body has become somehow transparent or with albinism. Although abnormality of aanat genes has not been determined in these species, we identified a similar premature stop in the encoding region of aaad gene as we found in the cave-restricted Mexican tetra (Lv et al., 2020), implying a possibility of weakening or disappearing rhythms in cavefishes, possibly caused by low melatonin levels (Yang et al., 2016).
Recently, a draft genome of Pseudoliparis swirei, a deep-sea snailfish (Mariana hadal snailfish, MHS) with a routine residence below 6,000 m, was published (Wang et al., 2019). Meanwhile, the genome of its closed relative Tanaka’s snailfish (Liparis tanakae, TS) from shallow sea was also available in the same article. We performed a detailed comparison of aanat2 gene structures between MHS and TS (Lv et al., 2020), and observed a frameshift insertion in MHS, while its relative TS was normal. As we supposed, the insertion may lead to an inactivity of AANAT2, and hence consequent low levels of blood melatonin in MHS (Lv et al., 2020). These are possibly related to the deep-sea darkness adaption, which is similar to our previously reported cave-restricted Sa (Yang et al., 2016) for cave adaptation.
More recently, we observed the similarly potential inactivation of AANAT2 in a Yap hadal snailfish (YHS) (Mu et al., 2021), which was collected at a 6,903 m depth. These results suggest that the low visual ability of MHS/YHS (Wang et al., 2019; Mu et al., 2021) may be similar to the cavefish-like degenerated eyes with a principal sense of shortwave light (You et al., 2018). Therefore, reducing melatonin synthesis by inactivation of AANAT or other melatonin biosynthesizing enzyme(s) may be a common mechanism for cave or deep-sea adaptation (Lv et al., 2020).
In addition to the aforementioned functions of AANATs in fish, most studies emphasized melatonin effects in the regulation of the following biological processes.
First, the impact of melatonin on the seasonal cycle of fish reproduction (Kumar Bairwa et al., 2013; Maitra and Hasan, 2016) has been largely investigated. The first report was in 1996 focusing on Atlantic croaker (Micropogonias undulates) (Khan and Thomas, 1996), followed by increasing number of reports on other species including stickleback (Kulczykowska et al., 2017), catfishes (Chaube and Joy, 2002; Martinez-Chavez et al., 2008; Aripin et al., 2015; Badruzzaman et al., 2020), and a non-air–breathing subtropical carp (Catla) being extensively used as a model to study fish melatonin (Figure 5) (Bhattacharya et al., 2007; Moniruzzaman and Maitra, 2012; Maitra et al., 2013; Hasan et al., 2014). It is now believed that melatonin not only acts as a hormone in determining the temporal pattern of spawning, but also as an antioxidant in regulation of oocyte maturation at the downstream of hypothalamus-pituitary-gonad (HPG) axis in fish (Shi, 2005; Maitra and Hasan, 2016).
Secondly, melatonin also impacts food intake and growth, which is largely dependent on day-length. Melatonin produced in pineal gland has been proved to control the related behavioral rhythms (Zhdanova et al., 2001; Falcón et al., 2010). However, various experimental results in different species (Spieler, 2001; Taylor et al., 2005; De Pedro et al., 2008) have not drawn a unanimous conclusion of exactly how melatonin impacts growth. There are estimations that melatonin may work by regulating the release of growth hormone (GH), plasma prolactin (PRL), and perhaps other pituitary hormones (Falcón et al., 2010; Falcón et al., 2011).
Additional functions of melatonin in fish include its influences on the water–salt balance, regulation of the antioxidant system, involvement in the immune system, and so on (Kuz’mina, 2020). However, the molecular mechanisms underlying each of these broad functions have not been fully elucidated yet.
Fish have the most diversity of aanat genes (aanat1a, aanat1b, and aanat2) in vertebrates (Li et al., 2015). AANAT plays critical roles in melatonin biosynthesis, and sometimes for potential dopamine metabolism, which are responsible for various physiological functions, such as seasonal migration, amphibious aerial vision, and cave or deep-sea adaptation. Interestingly, many transparent fish (Liu et al., 2017; Bian et al., 2020) are short of melatonin, possibly due to an inactivation of AANAT or other melatonin biosynthesizing enzyme(s); their shorter life-time (half a year for a transparent roy zebrafish (Bian et al., 2020), instead of 3–5 years for the wildtype) suggests that restoration of AANAT or melatonin may become an effective way to increase the lifetime of transparent fish. Meanwhile, accumulated genome and transcriptome data (Li et al., 2015; Sun et al., 2016; Hughes et al., 2018) provide genetic resources to obtain more and more sequences of aanat genes, which will enrich our understanding of AANAT and melatonin functions in various fishes, and ultimately improve and standardize husbandry practices (such as management of light, feeding, and spawning) in fish aquaculture worldwide.
Conceptualization, QS; writing—original draft preparation, YH, JL, and RL; writing—revision, YH, JL; writing—review and editing, QS, CB, and XY; supervision, QS; project administration and funding acquisition, QS. All authors have read and agreed to the published version of the manuscript.
This research was funded by the Shenzhen Science and Technology Program for International Cooperation (No. GJHZ20190819152407214), Grant Plan for Demonstration City Project for Marine Economic Development in Shenzhen (No. 86), and Shenzhen Dapeng Special Program for Industrial Development (No. KJYF202101-01).
The authors YH, CB, RL, XY, and QS were employed by BGI Academy of Marine Sciences.
The remaining author declares that the research was conducted in the absence of any commercial or financial relationships that could be construed as a potential conflict of interest.
All claims expressed in this article are solely those of the authors and do not necessarily represent those of their affiliated organizations, or those of the publisher, the editors, and the reviewers. Any product that may be evaluated in this article, or claim that may be made by its manufacturer, is not guaranteed or endorsed by the publisher.
We thank Dr. Yunyun Lv, an assistant professor at Neijiang Normal University (Neijiang, China), who has provided technical assistance for manuscript preparation.
AAAD, aromatic L-amino acid decarboxylase; AANAT, SNAT or NAT, arylalkylamine N-acetyltransferase; ASMT, acetylserotonin-O-methyltransferase; CNV, copy number variation; GCL, ganglion cell layer; GCN5, general control non-repressed five protein; GNAT, GCN5-related N-acetyltransferase; 5-HT, serotonin; HTrp, 5-hydroxytryptophan; INL, inner nuclear layer; NAS, N-acetylserotonin; NV-AANAT, nonvertebrate AANAT; ONL, outer nuclear layer; Ss4R, salmonid-specific WGD; TGD, teleost-specific genome duplication; TPH, tryptophan hydroxylase; Trp, tryptophan; VGD, vertebrate genome duplication; VT-AANAT, vertebrate AANAT; WGD, whole genome duplication.
Albrecht, M. (2009). Insights into the Nature of Human Testicular Peritubular Cells. Ann. Anat. - Anatomischer Anzeiger 191, 532–540. doi:10.1016/j.aanat.2009.08.002
Aripin, S. A., Jintasataporn, O., and Yoonpundh, R. (2015). Effects of Exogenous Melatonin in Clarias Macrocephalus Male Broodstock First Puberty Stage. J. Aquac. Res. Develop. 6, 307. doi:10.4172/2155-9546.1000307
Badruzzaman, M., Ikegami, T., Amin, A. K. M. R., and Shahjahan, M. (2020). Melatonin Inhibits Reproductive Activity through Changes of Serotonergic Activity in the Brain of Freshwater Catfish (Mystus Cavasius). Aquaculture 526, 735378. doi:10.1016/j.aquaculture.2020.735378
Ben-Moshe, Z., Foulkes, N. S., and Gothilf, Y. (2014). Functional Development of the Circadian Clock in the Zebrafish Pineal Gland. Biomed. Res. Int. 2014, 1–8. doi:10.1155/2014/235781
Berthelot, C., Brunet, F., Chalopin, D., Juanchich, A., Bernard, M., Noël, B., Bento, P., Da Silva, C., Labadie, K., Alberti, A., Aury, J.-M., and Louis, (2014). The Rainbow trout Genome Provides Novel Insights into Evolution after Whole-Genome Duplication in Vertebrates. Nat. Commun. 5, 3657. doi:10.1038/ncomms4657
Besseau, L., Benyassi, A., Møller, M., Coon, S. L., Weller, J. L., Boeuf, G., et al. (2006). Melatonin Pathway: Breaking the 'high-At-Night' Rule in trout Retina. Exp. Eye Res. 82, 620–627. doi:10.1016/j.exer.2005.08.025
Bhattacharya, S., Chattoraj, A., and Maitra, S. K. (2007). Melatonin in the Regulation of Annual Testicular Events in CarpCatla Catla: Evidence from the Studies on the Effects of Exogenous Melatonin, Continuous Light, and Continuous Darkness. Chronobiology Int. 24, 629–650. doi:10.1080/07420520701534665
Bian, C., Chen, W., Ruan, Z., Hu, Z., Huang, Y., Lv, Y., et al. (2020). Genome and Transcriptome Sequencing of casper and roy Zebrafish Mutants Provides Novel Genetic Clues for Iridophore Loss. Int. J. Mol. Sci. 21, 2385. doi:10.3390/ijms21072385
Brzezinski, A. (1997). Melatonin in Humans. N. Engl. J. Med. 336, 186–195. doi:10.1056/NEJM199701163360306
Cazaméa-Catalan, D., Besseau, L., Falcón, J., and Magnanou, E. (2014). The Timing of Timezyme Diversification in Vertebrates. PLoS One 9, e112380–22. doi:10.1371/journal.pone.0112380
Cazaméa-Catalan, D., Magnanou, E., Helland, R., Vanegas, G., Besseau, L., Boeuf, G., et al. (2012). Functional Diversity of Teleost arylalkylamineN-Acetyltransferase-2: Is Thetimezymeevolution Driven by Habitat Temperature? Mol. Ecol. 21, 5027–5041. doi:10.1111/j.1365-294X.2012.05725.x
Chattoraj, A., Liu, T., Zhang, L. S., Huang, Z., and Borjigin, J. (2009). Melatonin Formation in Mammals: In Vivo Perspectives. Rev. Endocr. Metab. Disord. 10, 237–243. doi:10.1007/s11154-009-9125-5
Chaube, R., and Joy, K. P. (2002). Effects of Altered Photoperiod and Temperature, Serotonin-Affecting Drugs, and Melatonin on Brain Tyrosine Hydroxylase Activity in Female catfish,Heteropneustes Fossilis: A Study Correlating Ovarian Activity Changes. J. Exp. Zool. 293, 585–593. doi:10.1002/jez.10185
Cheng, P., Huang, Y., Du, H., Li, C., Lv, Y., Ruan, R., et al. (2019). Draft Genome and Complete Hox-Cluster Characterization of the Sterlet (Acipenser ruthenus). Front. Genet. 10, 776. doi:10.3389/FGENE.2019.00776
Cheng, P., Huang, Y., Lv, Y., Du, H., Ruan, Z., Li, C., et al. (2020). The American Paddlefish Genome Provides Novel Insights into Chromosomal Evolution and Bone Mineralization in Early Vertebrates. Mol. Biol. Evol. 38, 1595–1607. doi:10.1093/molbev/msaa326
Coon, S. L., Bégay, V., Deurloo, D., Falcón, J., and Klein, D. C. (1999). Two Arylalkylamine N-Acetyltransferase Genes Mediate Melatonin Synthesis in Fish. J. Biol. Chem. 274, 9076–9082. doi:10.1074/jbc.274.13.9076
Coon, S. L., and Klein, D. C. (2006). Evolution of Arylalkylamine N-Acetyltransferase: Emergence and Divergence. Mol. Cell Endocrinol. 252, 2–10. doi:10.1016/j.mce.2006.03.039
Coon, S. L., Mazuruk, K., Bernard, M., Roseboom, P. H., Klein, D. C., and Rodriguez, I. R. (1996). The Human Serotonin N-Acetyltransferase (EC 2.3.1.87) Gene (AANAT): Structure, Chromosomal Localization, and Tissue Expression. Genomics 34, 76–84. doi:10.1006/geno.1996.0243
Coon, S. L., Weller, J. L., Korf, H.-W., Namboodiri, M. A. A., Rollag, M., and Klein, D. C. (2001). cAMP Regulation of Arylalkylamine N-Acetyltransferase (AANAT, EC 2.3.1.87). J. Biol. Chem. 276, 24097–24107. doi:10.1074/jbc.M011298200
De Pedro, N., Martnez-lvarez, R. M., and Delgado, M. J. (2008). Melatonin Reduces Body Weight in Goldfish (Carassius auratus): Effects on Metabolic Resources and Some Feeding Regulators. J. Pineal Res. 45, 32–39. doi:10.1111/j.1600-079X.2007.00553.x
Esteban, M. Á., Cuesta, A., Chaves-Pozo, E., and Meseguer, J. (2013). Influence of Melatonin on the Immune System of Fish: A Review. Int. J. Mol. Sci. 14, 7979–7999. doi:10.3390/ijms14047979
Falcón, J., Besseau, L., Magnanou, E., Herrero, M. J., Nagai, M., and Boeuf, G. (2011). Melatonin, the Time Keeper: Biosynthesis and Effects in Fish. Cybium 35, 3–18. doi:10.26028/cybium/2011-351-001
Falcón, J., Coon, S. L., Besseau, L., Cazaméa-Catalan, D., Fuentès, M., Magnanou, E., et al. (2014). Drastic Neofunctionalization Associated with Evolution of the Timezyme AANAT 500 Mya. Proc. Natl. Acad. Sci. U.S.A. 111, 314–319. doi:10.1073/pnas.1312634110
Falcón, J., Migaud, H., Muñoz-Cueto, J. A., and Carrillo, M. (2010). Current Knowledge on the Melatonin System in Teleost Fish. Gen. Comp. Endocrinol. 165, 469–482. doi:10.1016/j.ygcen.2009.04.026
Fan, G., Song, Y., Yang, L., Huang, X., Zhang, S., Zhang, M., et al. (2020). Initial Data Release and Announcement of the 10,000 Fish Genomes Project (Fish10K). Gigascience 9, 1–7. doi:10.1093/gigascience/giaa080
Feldkaemper, M., and Schaeffel, F. (2013). An Updated View on the Role of Dopamine in Myopia. Exp. Eye Res. 114, 106–119. doi:10.1016/j.exer.2013.02.007
Fernández-Durán, B., Ruibal, C., Polakof, S., Ceinos, R. M., Soengas, J. L., and Míguez, J. M. (2007). Evidence for Arylalkylamine N-Acetyltransferase (AANAT2) Expression in Rainbow trout Peripheral Tissues with Emphasis in the Gastrointestinal Tract. Gen. Comp. Endocrinol. 152, 289–294. doi:10.1016/j.ygcen.2006.12.008
Foulkes, N. S., Borjigin, J., Snyder, S. H., and Sassone-Corsi, P. (1996). Transcriptional Control of Circadian Hormone Synthesis via the CREM Feedback Loop. Proc. Natl. Acad. Sci. U.S.A. 93, 14140–14145. doi:10.1073/pnas.93.24.14140
Glasauer, S. M. K., and Neuhauss, S. C. F. (2014). Whole-genome Duplication in Teleost Fishes and its Evolutionary Consequences. Mol. Genet. Genomics 289, 1045–1060. doi:10.1007/s00438-014-0889-2
Han, Q., Robinson, H., Ding, H., Christensen, B. M., and Li, J. (2012). Evolution of Insect Arylalkylamine N -acetyltransferases: Structural Evidence from the Yellow Fever Mosquito, Aedes aegypti. Proc. Natl. Acad. Sci. U.S.A. 109, 11669–11674. doi:10.1073/pnas.1206828109
Hasan, K. N., Moniruzzaman, M., and Maitra, S. K. (2014). Melatonin Concentrations in Relation to Oxidative Status and Oocyte Dynamics in the Ovary during Different Reproductive Phases of an Annual Cycle in Carp Catla Catla. Theriogenology 82, 1173–1185. doi:10.1016/j.theriogenology.2014.08.001
Hickman, A. B., Klein, D. C., and Dyda, F. (1999). Melatonin Biosynthesis. Mol. Cel 3, 23–32. doi:10.1016/S1097-2765(00)80171-9
Hohjoh, H., Takasu, M., Shishikura, K., Takahashi, Y., Honda, Y., and Tokunaga, K. (2003). Significant Association of the Arylalkylamine N-Acetyltransferase (AA-NAT) Gene with Delayed Sleep Phase Syndrome. Neurogenetics 4, 151–153. doi:10.1007/s10048-002-0141-9
Hughes, L. C., Ortí, G., Huang, Y., Sun, Y., Baldwin, C. C., Thompson, A. W., et al. (2018). Comprehensive Phylogeny of ray-finned Fishes (Actinopterygii) Based on Transcriptomic and Genomic Data. Proc. Natl. Acad. Sci. U.S.A. 115, 6249–6254. doi:10.1073/pnas.1719358115
Inoue, J. G., Miya, M., Lam, K., Tay, B.-H., Danks, J. A., Bell, J., et al. (2010). Evolutionary Origin and Phylogeny of the Modern Holocephalans (Chondrichthyes: Chimaeriformes): A Mitogenomic Perspective. Mol. Biol. Evol. 27, 2576–2586. doi:10.1093/molbev/msq147
Isorna, E., Aliaga-Guerrero, M., M’Rabet, A. E., Servili, A., Falcón, J., and Muñoz-Cueto, J. A. (2011). Identification of Two Arylalkylamine N-Acetyltranferase 1 Genes with Different Developmental Expression Profiles in the Flatfish Solea Senegalensis. J. Pineal Res. 51, 434–444. doi:10.1111/j.1600-079X.2011.00907.x
Isorna, E., M’Rabet, A. E., Confente, F., Falcón, J., and Muñoz-Cueto, J. A. (2009). Cloning and Expression of Arylalkylamine N-Acetyltranferase-2 during Early Development and Metamorphosis in the Sole Solea Senegalensis. Gen. Comp. Endocrinol. 161, 97–102. doi:10.1016/j.ygcen.2008.10.007
Iyer, L. M., Aravind, L., Coon, S. L., Klein, D. C., and Koonin, E. V. (2004). Evolution of Cell-Cell Signaling in Animals: Did Late Horizontal Gene Transfer from Bacteria Have a Role? Trends Genet. 20, 292–299. doi:10.1016/j.tig.2004.05.007
Jumper, J., Evans, R., Pritzel, A., Green, T., Figurnov, M., Ronneberger, O., et al. (2021). Highly Accurate Protein Structure Prediction with AlphaFold. Nature 596, 583–589. doi:10.1038/s41586-021-03819-2
Kamruzzaman, A. S. M., Hiragaki, S., Watari, Y., Natsukawa, T., Yasuhara, A., Ichihara, N., et al. (2021). Clock‐controlled Arylalkylamine N‐acetyltransferase ( aaNAT ) Regulates Circadian Rhythms of Locomotor Activity in the American Cockroach, Periplaneta americana, via melatonin/MT2‐like Receptor. J. Pineal Res. 71, e12751. doi:10.1111/jpi.12751
Khan, I. A., and Thomas, P. (1996). Melatonin Influences Gonadotropin II Secretion in the Atlantic Croaker (Micropogonias Undulatus). Gen. Comp. Endocrinol. 104, 231–242. doi:10.1006/gcen.1996.0166
Klein, D. C. (2007). Arylalkylamine N-Acetyltransferase: "the Timezyme". J. Biol. Chem. 282, 4233–4237. doi:10.1074/jbc.R600036200
Klein, D. C. (2006). Evolution of the Vertebrate Pineal Gland: The Aanat Hypothesis. Chronobiology Int. 23, 5–20. doi:10.1080/07420520500545839
Kulczykowska, E., Kleszczyńska, A., Gozdowska, M., and Sokołowska, E. (2017). The Time Enzyme in Melatonin Biosynthesis in Fish: Day/night Expressions of Three Aralkylamine N-acetyltransferase Genes in Three-Spined Stickleback. Comp. Biochem. Physiol. A: Mol. Integr. Physiol. 208, 46–53. doi:10.1016/j.cbpa.2017.03.005
Kumar Bairwa, M., Saharan, N., Dube Rawat, K., Kumar Jakhar, J., and Bera, A. (2013). Photoperiod, Melatonin and its Importance in Fish Reproduction. Cent. Eur. J. Exp. Biol. 2, 7–15. Available at: http://scholarsresearchlibrary.com/archive.html.
Kuz’mina, V. V. (2020). Melatonin. Multifunctionality. Fish. J. Evol. Biochem. Phys. 56, 89–101. doi:10.1134/s0022093020020015
Lerner, A. B., Case, J. D., Takahashi, Y., Lee, T. H., and Mori, W. (1958). Isolation of Melatonin, the Pineal Gland Factor that Lightens Melanocytes1. J. Am. Chem. Soc. 80, 2587. doi:10.1021/ja01543a060
Li, J., You, X., Bian, C., Yu, H., Coon, S., and Shi, Q. (2015). Molecular Evolution of Aralkylamine N-Acetyltransferase in Fish: A Genomic Survey. Int. J. Mol. Sci. 17, 51. doi:10.3390/ijms17010051
Li, Y., Lv, Y., Bian, C., You, X., Deng, L., and Shi, Q. (2018). A Comparative Genomic Survey Provides Novel Insights into Molecular Evolution of L-Aromatic Amino Acid Decarboxylase in Vertebrates. Molecules 23, 917. doi:10.3390/molecules23040917
Lien, S., Koop, B. F., Sandve, S. R., Miller, J. R., Kent, M. P., Nome, T., et al. (2016). The Atlantic salmon Genome Provides Insights into Rediploidization. Nature 533, 200–205. doi:10.1038/nature17164
Liu, K., Xu, D., Li, J., Bian, C., Duan, J., Zhou, Y., et al. (2017). Whole Genome Sequencing of Chinese Clearhead Icefish, Protosalanx Hyalocranius. Protosalanx Hyalocranius. Gigascience 6, 1–6. doi:10.1093/gigascience/giw012
Liu, T., and Borjigin, J. (2005). N-acetyltransferase Is Not the Rate-Limiting Enzyme of Melatonin Synthesis at Night. J. Pineal Res. 39, 91–96. doi:10.1111/j.1600-079X.2005.00223.x
Ludwig, A., Belfiore, N. M., Pitra, C., Svirsky, V., and Jenneckens, I. (2001). Genome Duplication Events and Functional Reduction of Ploidy Levels in sturgeon (Acipenser, Huso and Scaphirhynchus). Genetics 158, 1203–1215. doi:10.1093/genetics/158.3.1203
Lv, Y., Li, Y., Li, J., Bian, C., Qin, C., and Shi, Q. (2020). A Comparative Genomics Study on the Molecular Evolution of Serotonin/melatonin Biosynthesizing Enzymes in Vertebrates. Front. Mol. Biosci. 7, 1–11. doi:10.3389/fmolb.2020.00011
Lynch, M., O'Hely, M., Walsh, B., and Force, A. (2001). The Probability of Preservation of a Newly Arisen Gene Duplicate. Genetics 159, 1789–1804. doi:10.1093/genetics/159.4.1789
Macqueen, D. J., and Johnston, I. A. (2014). A Well-Constrained Estimate for the Timing of the Salmonid Whole Genome Duplication Reveals Major Decoupling from Species Diversification. Proc. R. Soc. B. 281, 20132881. doi:10.1098/rspb.2013.2881
Maitra, S. K., Chattoraj, A., Mukherjee, S., and Moniruzzaman, M. (2013). Melatonin: a Potent Candidate in the Regulation of Fish Oocyte Growth and Maturation. Gen. Comp. Endocrinol. 181, 215–222. doi:10.1016/j.ygcen.2012.09.015
Maitra, S. K., and Hasan, K. N. (2016). The Role of Melatonin as a Hormone and an Antioxidant in the Control of Fish Reproduction. Front. Endocrinol. 7, 1–11. doi:10.3389/fendo.2016.00038
Maitra, S. K., and Pal, P. K. (2017). Melatonin Rhythms in the Pineal and Non-pineal Tissues and Their Physiological Implications in Subtropical Fish. Biol. Rhythm Res. 48, 757–776. doi:10.1080/09291016.2017.1345453
Martinez‐Chavez, C. C., Al‐Khamees, S., Campos‐Mendoza, A., Penman, D. J., and Migaud, H. (2008). Clock‐Controlled Endogenous Melatonin Rhythms in Nile Tilapia (Oreochromis niloticus Niloticus) and African Catfish (Clarias gariepinus). Chronobiology Int. 25, 31–49. doi:10.1080/07420520801917547
Moniruzzaman, M., and Maitra, S. K. (2012). Influence of Altered Photoperiods on Serum Melatonin and its Receptors (MT1 and MT2) in the Brain, Retina, and Ovary in CarpCatla Catla. Chronobiology Int. 29, 175–188. doi:10.3109/07420528.2011.645753
Mu, Y., Bian, C., Liu, R., Wang, Y., Shao, G., Li, J., et al. (2021). Whole Genome Sequencing of a Snailfish from the Yap Trench (∼7,000 M) Clarifies the Molecular Mechanisms Underlying Adaptation to the Deep Sea. PLOS Genet. 17, e1009530. doi:10.1371/journal.pgen.1009530
Nisembaum, L. G., Tinoco, A. B., Moure, A. L., Alonso Gómez, A. L., Delgado, M. J., and Valenciano, A. I. (2013). The Arylalkylamine-N-Acetyltransferase (AANAT) Acetylates Dopamine in the Digestive Tract of Goldfish: A Role in Intestinal Motility. Neurochem. Int. 62, 873–880. doi:10.1016/j.neuint.2013.02.023
O'Flynn, B. G., Suarez, G., Hawley, A. J., and Merkler, D. J. (2018). Insect Arylalkylamine N-Acyltransferases: Mechanism and Role in Fatty Acid Amide Biosynthesis. Front. Mol. Biosci. 5, 66. doi:10.3389/fmolb.2018.00066
Opazo, J. C., Butts, G. T., Nery, M. F., Storz, J. F., and Hoffmann, F. G. (2012). Whole-genome Duplication and the Functional Diversification of Teleost Fish Hemoglobins. Mol. Biol. Evol. 30, 140–153. doi:10.1093/molbev/mss212
Paulin, C. H., Cazaméa‐Catalan, D., Zilberman‐Peled, B., Herrera‐Perez, P., Sauzet, S., Magnanou, E., et al. (2015). Subfunctionalization of Arylalkylamine N‐acetyltransferases in the Sea Bass Dicentrarchus labrax : Two‐ones for One Two. J. Pineal Res. 59, 354–364. doi:10.1111/jpi.12266
Pavlicek, J., Sauzet, S., Besseau, L., Coon, S. L., Weller, J. L., Boeuf, G., et al. (2010). Evolution of AANAT: Expansion of the Gene Family in the Cephalochordate Amphioxus. BMC Evol. Biol. 10, 154. doi:10.1186/1471-2148-10-154
Rodriguez-Naranjo, M. I., Torija, M. J., Mas, A., Cantos-Villar, E., and Garcia-Parrilla, M. D. C. (2012). Production of Melatonin by Saccharomyces Strains under Growth and Fermentation Conditions. J. Pineal Res. 53, 219–224. doi:10.1111/j.1600-079X.2012.00990.x
Sanjita Devi, H., Rajiv, C., Mondal, G., Khan, Z. A., Dharmajyoti Devi, S., Yumnamcha, T., et al. (2016). Melatonin Bio-Synthesizing Enzyme Genes (Tph1, Aanat1, Aanat2, and Hiomt) and Their Temporal Pattern of Expression in Brain and Gut of a Tropical Carp in Natural Environmental Conditions. Cogent Biol. 2, 1230337. doi:10.1080/23312025.2016.1230337
Scheibner, K. A., De Angelis, J., Burley, S. K., and Cole, P. A. (2002). Investigation of the Roles of Catalytic Residues in Serotonin N-Acetyltransferase. J. Biol. Chem. 277, 18118–18126. doi:10.1074/jbc.M200595200
Sevilla, A., Chéret, J., Slominski, R. M., Slominski, A. T., and Paus, R. (2022). Revisiting the Role of Melatonin in Human Melanocyte Physiology: A Skin Context Perspective. J. Pineal Res. 72, e12790. doi:10.1111/jpi.12790
Shi, Q., Ando, H., Coon, S. L., Sato, S., Ban, M., and Urano, A. (2004). Embryonic and post-embryonic Expression of Arylalkylamine N-Acetyltransferase and Melatonin Receptor Genes in the Eye and Brain of Chum salmon (Oncorhynchus keta). Gen. Comp. Endocrinol. 136, 311–321. doi:10.1016/j.ygcen.2004.01.004
Shi, Q. (2005). Melatonin Is Involved in Sex Change of the Ricefield Eel, Monopterus albus Zuiew. Rev. Fish. Biol. Fish. 15, 23–36. doi:10.1007/s11160-005-7848-2
Shu, S.-S., Jiang, W.-S., Whitten, T., Yang, J.-X., and Chen, X.-Y. (2013). Drought and China's Cave Species. Science 340, 272. doi:10.1126/science.340.6130.272-a
Slominski, A., Pisarchik, A., Semak, I., Sweatman, T., Wortsman, J., Szczesniewski, A., et al. (2002). Serotoninergic and Melatoninergic Systems Are Fully Expressed in Human Skin. FASEB j. 16, 896–898. doi:10.1096/fj.01-0952fje
Spieler, R. E. (2001). Circadian Timing of Meal Feeding and Growth in Fishes. Rev. Fish. Sci. 9, 115–131. doi:10.1080/20016491101726
Sun, Y., Huang, Y., Li, X., Baldwin, C. C., Zhou, Z., Yan, Z., et al. (2016). Fish-T1K (Transcriptomes of 1,000 Fishes) Project: Large-Scale Transcriptome Data for Fish Evolution Studies. GigaSci 5, 18. doi:10.1186/s13742-016-0124-7
Taylor, J. F., Migaud, H., Porter, M. J. R., and Bromage, N. R. (2005). Photoperiod Influences Growth Rate and Plasma Insulin-like Growth Factor-I Levels in Juvenile Rainbow trout, Oncorhynchus mykiss. Gen. Comp. Endocrinol. 142, 169–185. doi:10.1016/j.ygcen.2005.02.006
Taylor, J. S., Braasch, I., Frickey, T., Meyer, A., and Van de Peer, Y. (2003). Genome Duplication, a Trait Shared by 22,000 Species of ray-finned Fish. Genome Res. 13, 382–390. doi:10.1101/gr.640303
Tilden, A. R., Becker, M. A., Amma, L. L., Arciniega, J., and McGaw, A. K. (1997). Melatonin Production in an Aerobic Photosynthetic Bacterium: An Evolutionarily Early Association with Darkness. J. Pineal Res. 22, 102–106. doi:10.1111/j.1600-079X.1997.tb00310.x
True, J. R., and Carroll, S. B. (2002). Gene Co-option in Physiological and Morphological Evolution. Annu. Rev. Cel Dev. Biol. 18, 53–80. doi:10.1146/annurev.cellbio.18.020402.140619
Velarde, E., Cerdá-Reverter, J. M., Alonso-Gómez, A. L., Sánchez, E., Isorna, E., and Delgado, M. J. (2010). Melatonin-synthesizing Enzymes in Pineal, Retina, Liver, and Gut of the Goldfish (Carassius): MRNA Expression Pattern and Regulation of Daily Rhythms by Lighting Conditions. Chronobiology Int. 27, 1178–1201. doi:10.3109/07420528.2010.496911
Vivien-Roels, B., and Pévet, P. (1993). Melatonin: Presence and Formation in Invertebrates. Experientia 49, 642–647. doi:10.1007/BF01923945
Wang, K., Shen, Y., Yang, Y., Gan, X., Liu, G., Hu, K., et al. (2019). Morphology and Genome of a Snailfish from the Mariana Trench Provide Insights into Deep-Sea Adaptation. Nat. Ecol. Evol. 3, 823–833. doi:10.1038/s41559-019-0864-8
Wolf, E., De Angelis, J., Khalil, E. M., Cole, P. A., and Burley, S. K. (2002). X-ray Crystallographic Studies of Serotonin N-Acetyltransferase Catalysis and Inhibition. J. Mol. Biol. 317, 215–224. doi:10.1006/jmbi.2001.5371
Xu, J., Li, Y., Lv, Y., Bian, C., You, X., Endoh, D., et al. (2019). Molecular Evolution of Tryptophan Hydroxylases in Vertebrates: A Comparative Genomic Survey. Genes 10, 203. doi:10.3390/genes10030203
Xu, P., Zhang, X., Wang, X., Li, J., Liu, G., Kuang, Y., et al. (2014). Genome Sequence and Genetic Diversity of the Common Carp, Cyprinus carpio. Nat. Genet. 46, 1212–1219. doi:10.1038/ng.3098
Yang, J., Chen, X., Bai, J., Fang, D., Qiu, Y., Jiang, W., et al. (2016). The Sinocyclocheilus Cavefish Genome Provides Insights into Cave Adaptation. BMC Biol. 14, 1. doi:10.1186/s12915-015-0223-4
Yasmin, F., Sutradhar, S., Das, P., and Mukherjee, S. (2021). Gut Melatonin: A Potent Candidate in the Diversified Journey of Melatonin Research. Gen. Comp. Endocrinol. 303, 113693. doi:10.1016/j.ygcen.2020.113693
Yin, D., Zhou, R., Yin, M., Chen, Y., Xu, S., and Yang, G. (2021). Gene Duplication and Loss of AANAT in Mammals Driven by Rhythmic Adaptations. Mol. Biol. Evol. 38, 3925–3937. doi:10.1093/molbev/msab125
Yin, Y. H., Zhang, X. H., Wang, X. A., Li, R. H., Zhang, Y. W., Shan, X. X., et al. (2021). Construction of a Chromosome-Level Genome Assembly for Genome-wide Identification of Growth-Related Quantitative Trait Loci in Sinocyclocheilus Grahami (Cypriniformes, Cyprinidae). Zool. Res. 42, 262–266. doi:10.24272/J.ISSN.2095-8137.2020.321
You, X., Bian, C., Zan, Q., Xu, X., Liu, X., Chen, J., et al. (2014). Mudskipper Genomes Provide Insights into the Terrestrial Adaptation of Amphibious Fishes. Nat. Commun. 5, 1–8. doi:10.1038/ncomms6594
You, X., Sun, M., Li, J., Bian, C., Chen, J., Yi, Y., et al. (2018). Mudskippers and Their Genetic Adaptations to an Amphibious Lifestyle. Animals 8, 24–12. doi:10.3390/ani8020024
Zhang, K., Huang, Y., and Shi, Q. (2021). Genome-wide Identification and Characterization of 14-3-3 Genes in Fishes. Gene 791, 145721. doi:10.1016/j.gene.2021.145721
Zhang, K., Ruan, Z., Li, J., Bian, C., You, X., Coon, S., et al. (2017). A Comparative Genomic and Transcriptomic Survey Provides Novel Insights into N-Acetylserotonin Methyltransferase (ASMT) in Fish. Molecules 22, 1653. doi:10.3390/molecules22101653
Zhang, L., Li, M. Z., Chen, Z. H., Tang, Y., Liao, C. H., and Han, Q. (2021). Arylalkalamine N‐acetyltransferase‐1 Functions on Cuticle Pigmentation in the Yellow Fever Mosquito, Aedes aegypti. Insect Sci. 28, 1591–1600. doi:10.1111/1744-7917.12895
Zhang, L., Tang, Y., Chen, H., Zhu, X., Gong, X., Wang, S., et al. (2022). Arylalkalamine N-acetyltransferase-1 Acts on a Secondary Amine in the Yellow Fever Mosquito, Aedes aegypti. FEBS Lett 596, 1081–1091. doi:10.1002/1873-3468.14316
Zhang, Y., Li, H., Du, J., Zhang, J., Shen, J., and Cai, W. (2019). Three Melanin Pathway Genes, TH, Yellow, and aaNAT, Regulate Pigmentation in the Twin-Spotted Assassin Bug, Platymeris Biguttatus (Linnaeus). Int. J. Mol. Sci. 20, 2728. doi:10.3390/ijms20112728
Zhao, D., Yu, Y., Shen, Y., Liu, Q., Zhao, Z., Sharma, R., et al. (2019). Melatonin Synthesis and Function: Evolutionary History in Animals and Plants. Front. Endocrinol. 10, 1–16. doi:10.3389/fendo.2019.00249
Zhdanova, I. V., Wang, S. Y., Leclair, O. U., and Danilova, N. P. (2001). Melatonin Promotes Sleep-like State in Zebrafish. Brain Res. 903, 263–268. doi:10.1016/s0006-8993(01)02444-1
Zhou, L., and Gui, J. (2017). Natural and Artificial Polyploids in Aquaculture. Aquac. Fish. 2, 103–111. doi:10.1016/j.aaf.2017.04.003
Zilberman-Peled, B., Appelbaum, L., Vallone, D., Foulkes, N. S., Anava, S., Anzulovich, A., et al. (2007). Transcriptional Regulation of Arylalkylamine-N-Acetyltransferase-2 Gene in the Pineal Gland of the Gilthead Seabream. J. Neuroendocrinol. 19, 46–53. doi:10.1111/j.1365-2826.2006.01501.x
Zilberman-Peled, B., Bransburg-Zabary, S., Klein, D. C., and Gothilf, Y. (2011). Molecular Evolution of Multiple Arylalkylamine N-Acetyltransferase (AANAT) in Fish. Mar. Drugs 9, 906–921. doi:10.3390/md9050906
Keywords: melatonin biosynthesis, arylalkylamine N-acetyltransferase (AANAT), phylogeny, physiological function, fish
Citation: Huang Y, Li J, Bian C, Li R, You X and Shi Q (2022) Evolutionary Genomics Reveals Multiple Functions of Arylalkylamine N-Acetyltransferase in Fish. Front. Genet. 13:820442. doi: 10.3389/fgene.2022.820442
Received: 23 November 2021; Accepted: 15 April 2022;
Published: 19 May 2022.
Edited by:
Robert A. Haney, Ball State University, United StatesReviewed by:
Frederic Guy Brunet, UMR5242 Institut de Génomique Fonctionnelle de Lyon (IGFL), FranceCopyright © 2022 Huang, Li, Bian, Li, You and Shi. This is an open-access article distributed under the terms of the Creative Commons Attribution License (CC BY). The use, distribution or reproduction in other forums is permitted, provided the original author(s) and the copyright owner(s) are credited and that the original publication in this journal is cited, in accordance with accepted academic practice. No use, distribution or reproduction is permitted which does not comply with these terms.
*Correspondence: Yu Huang, aHVhbmd5dUBnZW5vbWljcy5jbg==; Qiong Shi, c2hpcWlvbmdAZ2Vub21pY3MuY24=
†These authors have contributed equally to this work
Disclaimer: All claims expressed in this article are solely those of the authors and do not necessarily represent those of their affiliated organizations, or those of the publisher, the editors and the reviewers. Any product that may be evaluated in this article or claim that may be made by its manufacturer is not guaranteed or endorsed by the publisher.
Research integrity at Frontiers
Learn more about the work of our research integrity team to safeguard the quality of each article we publish.