- 1Department of Diagnostics, Medical Integration and Practice Center, Cheeloo College of Medicine, Shandong University, Jinan, China
- 2Division of Epidemiology, Biostatistics, and Environmental Health, School of Public Health. University of Memphis, Memphis, TN, United States
- 3College of Land Science and Technology, China Agricultural University, Beijing, China
The group of receptor-interacting protein (RIP) kinases has seven members (RIPK1–7), with one homologous kinase domain but distinct non-kinase regions. Although RIPK1–3 have emerged as key modulators of inflammation and cell death, few studies have connected RIPK4–7 to immune responses. The divergence in domain structures and paralogue information in the Ensembl database have raised question about the phylogeny of RIPK1–7. In this study, phylogenetic trees of RIPK1–7 and paralogues constructed using full-length amino acid sequences or Kinase domain demonstrate that RIPK6 and RIPK7 are distinct from RIPK1–5 and paralogues shown in the Ensembl database are inaccurate. Comparative and evolutionary analyses were subsequently performed to gain new clues about the potential functions of RIPK3–7. RIPK3 gene loss in birds and animals that undergo torpor, a common physiological phenomenon in cold environments, implies that RIPK3 may be involved in ischemia-reperfusion injury and/or high metabolic rate. The negligible expression of RIPK4 and RIPK5 in immune cells is likely responsible for the lack of studies on the direct role of these members in immunity; RIPK6 and RIPK7 are conserved among plants, invertebrates and vertebrates, and dominantly expressed in innate immune cells, indicating their roles in innate immunity. Overall, our results provide insights into the multifaceted and conserved biochemical functions of RIP kinases.
Introduction
The innate immune system, as the first line of host defense against infection, is equipped with innate sensors that can effectively clear pathogens by recognizing molecular structures known as pathogen-associated molecular patterns (PAMPs) or danger-associated molecular patterns (DAMPs) (Silke et al., 2015). These innate sensors, including Toll-like receptors, NOD-like receptors and RIG-I-like receptors, are engaged by relevant PAMPs from bacteria, fungi or viruses. This can trigger various intracellular signaling cascades to activate transcription factors, such as nuclear factor-kappa B (NF-κB), activator protein-1 (AP-1), and interferon regulatory factors (IRFs) to induce the production of inflammatory cytokines, chemokines and interferons, as well as activate cell death to eliminate pathogen-infected or damaged cells (Chen et al., 2009; Loo and Gale, 2011; O’Neill et al., 2013; Bhat and Fitzgerald, 2014). Cell death comes in many different forms: apoptosis, which is widely considered silent for inflammation; accidental necrosis and programmed necrosis (such as necroptosis and pyroptosis), which are considered highly inflammatory (Humphries et al., 2015; Li et al., 2021). Therefore, cell death can be closely integrated with inflammation to maintain immune homeostasis (Wallach et al., 2014). Receptor-interacting protein (RIP) kinases have emerged as key molecules in the regulation of inflammation and cell death pathways (He and Wang, 2018).
RIP kinases have seven members (RIPK1–7) that share a conserved serine-threonine kinase domain but have distinct non-kinase functional features (Figure 1A) (He and Wang, 2018). RIPK1, the first identified member of the RIP kinases, contains the following functional features: an N-terminal Kinase domain, which can mediate autophosphorylation to promote its own activation; a C-terminal DEATH domain, which can bind to several death receptors, such as tumor necrosis factor receptor 1 (TNF-R1); and an intermediate RIP homotypic interaction motif (RHIM), which can recruit RIPK3 through activation of IRFs (Ofengeim and Yuan, 2013; Meng et al., 2018; Xu et al., 2018). RIPK1 mutations are associated with arthritis, inflammatory bowel disease (IBD), recurrent fevers and lymphadenopathy (Cuchet-Lourenço et al., 2018; Li et al., 2019). RIPK2, the second characterized member, bears an N-terminal Kinase and a C-terminal caspase-activation and recruitment domain (CARD), through which it can interact with the CARDs of the intracellular peptidoglycan sensors NOD1 and NOD2, resulting in the activation of NF-κB and mitogen-activated protein kinase (MAPK; 15). Recent experimental and clinical studies have provided evidences that RIPK2 is highly associated with leprosy, osteoarthritis and IBD (Zhang et al., 2009; Thiébaut et al., 2011; Jurynec et al., 2018). RIPK3, the third described RIP kinase, is composed of N-terminal Kinase and C-terminal RHIM domain, through which RIPK3 can interact with RIPK1 (Sun et al., 1999).
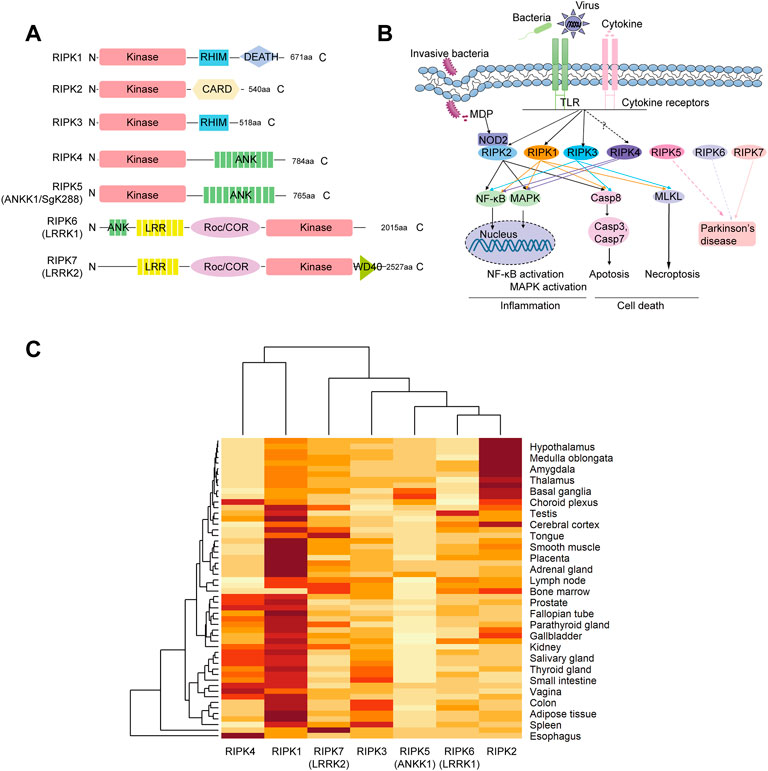
FIGURE 1. Structure organization, signaling pathways and tissue expression of human RIP kinases (A) Domain architecture. RIPK1–7 contains a serine-threonine kinase domain (Kinase). RHIM, RIP homotypic interaction motif; CARD, caspase-activation and recruitment domain; ANK, ankyrin repeats; LRR, leucine-rich repeats; Roc/COR, Ras of complex proteins/C-terminal Roc; WD40, WD40 repeats (B) Diagram of signaling networks shown on the KEGG pathway website and in previous studies. NF-kB, nuclear factor-kappa B; MAPK, mitogen-activated protein kinase; NOD, nucleotide-binding oligomerization domain. (C) Hierarchical clustering of the expression profiles of RIPK1–7 in different tissues derived from the consensus data sets of HPA, GTEx and FANTOM5 in the Human Protein Atlas. Expression values are normalized as transcripts per million levels. Hierarchical clustering was conducted on both the row variable (tissue) and the column variable (gene) by heatmap function in R.4.1.0.
However, few studies have linked immune responses to functions of RIPK4–7. RIPK4 was first identified as protein kinase C (PKC)δ-interacting protein kinase by yeast two-hybrid system (Bähr et al., 2000). RIPK4 has an N-terminal Kinase domain and a C-terminal ankyrin repeats domain (ANK). Overexpression of RIPK4 can induce NF-κB activation and Jun N-terminal kinase (JNK) signaling (Meylan et al., 2002). RIPK5, also known as ankyrin repeat and kinase domain containing 1 (ANKK1) or sugen kinase 288 (SgK288), harbors a Kinase domain in N terminus and ANK in C terminus (He and Wang, 2018). The ANKK1 TaqIA polymorphism is the most studied genetic variant related to neuropsychiatric disorders (Koeneke et al., 2020; Ohira et al., 2022). Furthermore, ANKK1 overexpression can affect phases of the cell cycle, especially G1 and M (España-Serrano et al., 2017). RIPK6 and RIPK7, also known as LRRK1 and LRRK2, are characterized by leucine-rich repeats (LRR), a Roc GTPase and COR dimerization motif (Roc/COR), and a C-terminal Kinase domain. RIPK6 harbors an ANK motif in the N terminus, and RIPK7 has a WD40 in the C terminus. The LRR motif may play a role in the recognition of molecular patterns associated with damage, pathogens, or stress (Festjens et al., 2007; Zhang et al., 2010). The binding of GTP to Roc/COR domain can stimulate RIPK6 kinase activity (Korr et al., 2006) and assist RIPK7 in membrane trafficking via the endo-lysosomal pathway (Shin et al., 2008; Piccoli et al., 2011; Sheehan and Yue, 2019). Although many studies reported that RIPK7 variants and kinase activity is likely central to the pathogenesis of Parkinson’s disease, the basic functions of RIPK7 remain poorly understood (Berwick et al., 2019; Seegobin et al., 2020). A RIPK6 variant has also been proposed as a risk factor for the development of familial Parkinson’s disease (Dachsel et al., 2010; Schulte et al., 2014).
In summary, RIPK1–7 exhibit significant divergence in domain structures and functions. Indeed, the common Kinase domain they share is also present in multiple other kinases annotated in the Ensembl database. Some interesting questions remain: What is the evolutionary relationship among RIPK1–7? Are the paralogues shown in the Ensembl database incorrect? Although RIPK1–3 have been adequately reported in human and mice and characterized with similar functions in pig, chicken, frog, zebrafish, black carp and lamprey, they remain poorly investigated in other vertebrate species (Ishizawa et al., 2006; Chang et al., 2010; Park et al., 2018; Hou et al., 2020; Xie et al., 2020; Liu et al., 2021). Meanwhile, little is known about RIPK4–7 roles in immune responses. In this study, phylogenetic trees of RIPK1–7 and paralogues constructed using their full length and their kinase domain indicated that RIPK6 and RIPK7 are clearly distinct from RIPK1–5 and that the paralogues in the Ensembl database are inaccurate. An extensive BLAST survey identified the ancient eukaryotic ortholog of RIP kinases in the protist taxa Cryptophyta (Guillardia theta), which suggests that RIP kinases arose prior to the separation of animal, plant and fungal lineages. The negligible expression of Ripk4 and Ripk5 (Ankk1) in immune cells may explain why there have been no studies of the direct role of Ripk4 and Ripk5 in immune responses. Furthermore, the close evolutionary relationships of gene expression in organs between RIPK1 and RIPK4, and the fact that 10 of 12 critical residues are homologous between RIPK1 and RIPK4, suggests that RIPK4 might play a role on NF-κB or MAPK activation like RIPK1, but mainly in non-immune cell types. RIPK6 and RIPK7 with the domain structure of the ANK-LRR-Roc/COR-Kinase axis are highly conserved among plants, invertebrates and vertebrates, and markedly expressed in innate immune cells, suggesting their potential dominant role in innate immune responses.
Material and methods
Gene extraction
RIP kinases in Homo sapiens were derived from NCBI (http://www.ncbi.nlm.nih.gov/sites/entrez) as follows: amino acid sequences of RIPK1 (GenBank accession number: NP_001341859), RIPK2 (NP_003812), RIPK3 (NP_006862), RIPK4 (NP_065690), RIPK5 (NP_848605), RIPK6 (NP_078928), and RIPK7 (NP_940980). Paralogues of RIP kinases were extracted from “Paralogues” in Comparative Genomics in the Ensembl database (https://asia.ensembl.org/index.html). According to the established taxonomic relationships, representative organisms from Mammal (human H. sapiens and mouse Mus musculus), Ave (chicken Gallus gallus), Reptilia (green anole Anolis carolinensis), Amphibian (clawed frog Xenopus tropicalis), Teleost fish (zebrafish Danio rerio), Cyclostomata (lamprey Lethenteron japonicum), Cephalochordate (amphioxus Branchiostoma floridae), Arthropoda (fruitfly Drosophila grimshawi), Nematomorpha (nematode Caenorhabditis elegans), Cnidaria (fresh-water polyp Hydra vulgaris), Amoebozoa (soil-dwelling amoeba Dictyostelium discoideum), Cryptophyta (cryptomonad algae Guillardia theta), and Plants (silver myrtle Rhodamnia argentea) were selected. RIP kinase homologs of these species were retrieved based on the best hits of an extensive BLASTP against NCBI and JGI database (https://genome.jgi.doe.gov/portal/) with human RIPK1–7 as the queries. All returned sequences were reciprocally searched against other genomes to further verify their identities (identity ≥ 30%, E-value ≤ 1e-3).
Gene expression in organs
Expression patterns of RIPK1–7 mRNA in various human tissues and organs were obtained from the Human Protein Atlas (HPA; https://www.proteinatlas.org/). Expression was normalized as transcripts per million. A heatmap was created using heatmap function in R.4.1.0. With the heatmap function, hierarchical clustering was conducted on both the row variable (tissue) and the column variable (gene). Both tissue and gene names were reordered based on the results of the hierarchical analysis.
Immune features analysis
Values for the expression of mouse Ripk1–7 mRNA normalized by DEseq2 in various immune cells were extracted from Gene Skyline from the Immunological Genome Project (ImmGen; http://rstats.immgen.org/Skyline/skyline.html). Correlated gene of Ripk1–7 in immune cells were obtained with Gene Constellation (http://rstats.immgen.org/GeneConstellation/index.html). An intersection analysis of correlated genes was performed in EVenn (http://www.ehbio.com/test/venn). In addition, correlated genes were uploaded to the Database for Annotation, Visualization and Integrated Discovery (DAVID, https://david.ncifcrf.gov/) with the settings of selected identifier (“OFFICAL_GENE_SYMBOL”), species (“Homo sapiens”), and the functional annotation chart to analyze enriched Kyoto Encyclopedia of Genes and Genomes (KEGG) pathways with p < 0.05.
Sequence and functional motif analysis
Functional domains were identified by SMART (http://smart.emblheidelberg.de), which uses HMMER (biosequence analysis using profile hidden Markov models) together with Pfam (http://pfam.sanger.ac.uk/search) for searching domain homologs by default thresholds. Identify of amino acids of RIP kinases between human and other species were determined by pairwise sequence alignment in EMBL-EBI (https://www.ebi.ac.uk/). Functional motif analyses were calculated by MEME (http://meme-suite.org/tools/meme) with a motif size between 6 and 50 amino acids and a maximum of 25 motifs. Alignment was performed with ClustalW from the UGENE server. Signaling pathways regulated by RIP kinase were analyzed by KEGG database (https://www.genome.jp/kegg/pathway.html).
Evolutionary analysis
Maximum likelihood (ML) phylogenetic trees were constructed using the JTT model by MEGA X software. The reliability of each interior branch was assessed by bootstrapping with 100 replications. The gene gain/loss tree of RIPK3 was derived from Comparative Genomics in the Ensembl database. To indicate whether neighboring genes surrounding RIPK1–7 were evolutionarily conserved, we performed synteny analysis of surrounding genes with transcriptional orientations using genomic data from NCBI Map Viewer assemblies (http://www.ncbi.nlm.nih.gov/mapview/) for H. sapiens, X. tropicalis, B. floridae, C. elegans and D. discoideum.
Results
Structure organization, expression patterns and functions of human RIPK1–7
The immune system protects the host against infection and tissue injury by initiating various cellular signaling pathways (He and Wang, 2018). According to the KEGG database, when innate sensors recognize stimuli (bacteria, viruses, and cytokines), RIPK1 can be recruited to the signaling complex to elicit inflammation (NF-κB and MAPK) pathways as well as cell death (necroptosis and apoptosis). RIPK2 can be recognized by the intracellular receptor NOD2 and subsequently induce NF-κB activation, MAPK activation, and apoptosis (Figure 1B). RIPK3 is involved in apoptosis, necroptosis, and a “non-canonical” NF-κB activation via facilitating RelB-p50 heterodimer nuclear translation in specific cell types, including bone marrow derived dendritic cells and aortic smooth muscle cells (Moriwaki and Chan, 2017). RIPK7 is associated with Parkinson’s disease. Unfortunately, there is no information about RIPK4–6 in the KEGG database. However, Meylan et al. (Meylan et al., 2002) found that RIPK4 overexpression can induce NF-κB activation and JNK signaling (MAPK activation). RIPK5/ANKK1 polymorphisms are associated with neuropsychiatric disorders, including Parkinson’s disease (Ohira et al., 2022). A RIPK6 variant (p. Arg1261Gln) has also been identified as a candidate for a disease-causing genetic variant of Parkinson’s disease (Schulte et al., 2014).
Furthermore, expression patterns of RIP kinases in various tissues were obtained from HPA using a combination of the HPA, GTEx, and FANTOM5 (Functional Annotation of the Mammalian Genome 5) data sets. As shown in Supplementary Figure S1, RIPK1 and RIPK2 are widely detected in all tissues. RIPK3, RIPK4, RIPK5, RIPK6, and RIPK7 are detected in many tissues but enhanced in intestine, esophagus, skin/brain, lymphoid tissue, and lung, respectively. Moreover, we used hierarchical analysis to dissect the correlation between expression data and phylogeny. As shown in Figure 1C, the heatmap analysis showed that the phylogeny of RIPK4 expression is close to that of RIPK1, which are distinct from the other five members. Meanwhile, phylogeny of RIPK6 is close to that of RIPK2.
Associations between human RIPK1–7 and immune cell signatures
Currently, very few studies have connected inflammation and cell death with RIPK4–7. We therefore analyzed expression profiles of mouse Ripk1–7 in various mouse immune cells from the ImmGen server, including bone marrow, B cells, T cells, natural killer cells, innate lymphoid cells, neutrophils, dendritic cells, macrophages, and mast cells. As shown in Figure 2A, Ripk1 is enriched in neutrophils and widely detected in all cells. Ripk2 and Ripk3 are distributed in all immune cells. We were surprised to find that Ripk4 and Ripk5 are negligible in all immune cells except MHCIIhigh thymic medullary epithelial cells, which indicates an insignificant role of Ripk4 and Ripk5 in immune responses. Ripk6 is dominant in dendritic cells and natural killer cells, expressed at a low level in innate lymphoid cells, and missing in some CD4+ and CD8+ T cells. Ripk7 is mainly expressed in neutrophils and B cells and is absent in T cells, natural killer cells, and ILC2 cells, which suggests the predominant role of Ripk7 in innate immune responses.
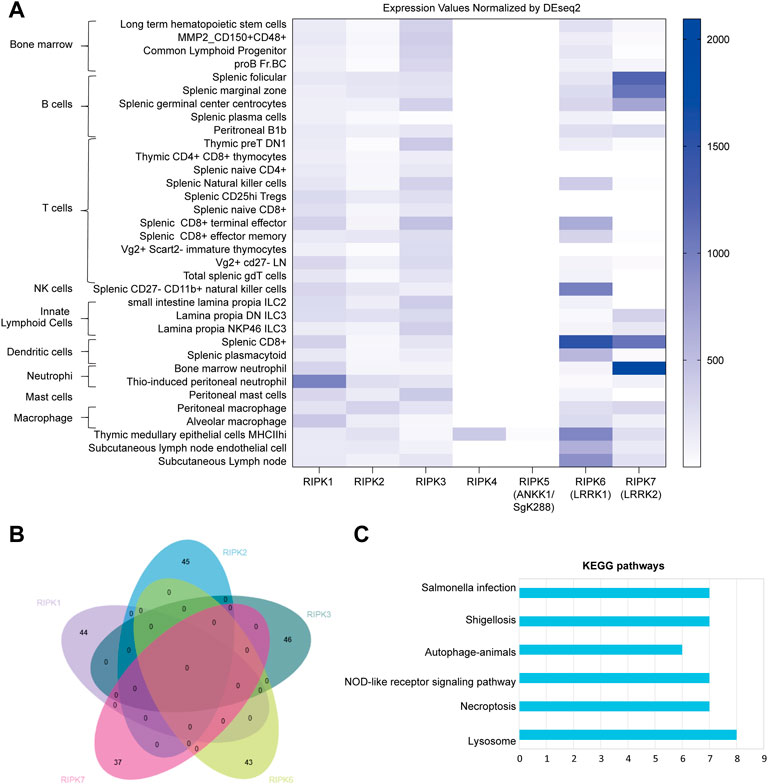
FIGURE 2. Mouse Ripk1–7 in immune cells (A) Expression pattern of Ripk1–7 normalized by DESeq2 in immune cells derived from Gene Skyline in ImmGen, including bone marrow, B cells, T cells, natural killer cells, innate lymphoid cells, neutrophils, dendritic cells, macrophages, and mast cells. (B) Intersection analysis of genes significantly correlated with Ripk1–7 in immune cells extracted from Gene Constellation by EVenn. (C) KEGG pathway enrichment analysis of the correlated gene with Ripk1–7. The x axis shows the number of genes clustered in each category. The y axis shows the names of the enriched signaling pathways. p is set at 0.05.
To further investigate the potential molecular mechanism of RIP kinase in these immune cells, we extracted genes significantly correlated with Ripk1–3 and Ripk6 and Ripk7 using Gene Constellation in ImmGen; Ripk4 and Ripk5 were excluded because of their negligible expression in immune cells. An intersection analysis of the correlated genes in EVenn indicates no common correlated genes between any two RIP kinases (Figure 2B). DAVID showed enriched KEGG pathways for these correlated genes includes pathogen infection (Salmonella infection and shigellosis), inflammation (NOD-like receptor signaling pathway, autophagy-animals and lysosome), and cell death (necroptosis) (Figure 2C).
What is the evolutionary relationship among human RIPK1–7?
It is remarkable that RIPK1–7 exhibit salient divergence in their domain structures, expression patterns, and functions, although they share a similar Kinase domain. In the paralogue family in the Ensembl database (Figure 3A), RIPK1–3 and RIPK7 share the same paralogues, whereas RIPK4–6 have completely different paralogues. Furthermore, the paralogues of RIPK1–5 and RIPK7 contain a Kinase domain and other diverse domains/motifs according to SMART, whereas RIPK6 paralogues do not contain a Kinase domain but have an LRR domain instead (Table 1). Moreover, there is some controversy regarding which gene can be represented as RIPK5. Dusty protein kinase (DSTYK; GenBank accession number: NP_056190) is characterized as RIPK5 by the majority of public databases (e.g., NCBI and Ensembl) and Zha et al., 2004 (Zha et al., 2004). Kinome analysis by Manning et al., 2002 indicated that DSTYK is closer to IRAKs than to the other RIP genes (Manning et al., 2002). This raises the following questions: What is the evolutionary relationship among human RIPK1–7? Are the paralogues shown in the Ensembl database incorrect?
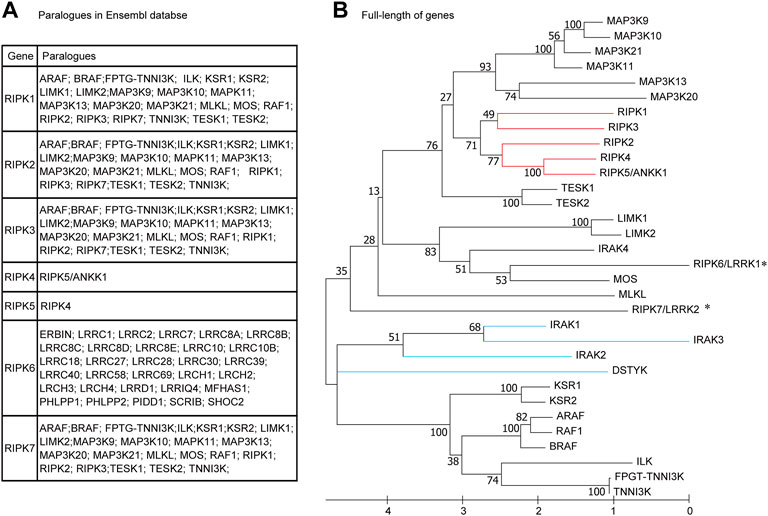
FIGURE 3. Phylogenetic relationships among human RIPK1–7 and their paralogues (A) Paralogues in the Ensembl database. (B) The maximum likelihood (ML) tree using the full-length amino acid sequences. The bootstrap percentage derived from 100 replications is shown on the interior branches. GenBank accession numbers of the full-length genes are as follows: IRAK1 (NP_001560), IRAK2 (NP_001561), IRAK3 (NP_009130), IRAK4 (NP_001107654), DSTYK (NP_056190). Other sequence accession numbers refer to Table 1. * indicates human RIPK6 and RIPK7 genes; the red line indicates RIPK1–5; the blue line indicates DSTYK and IRAK1–3.
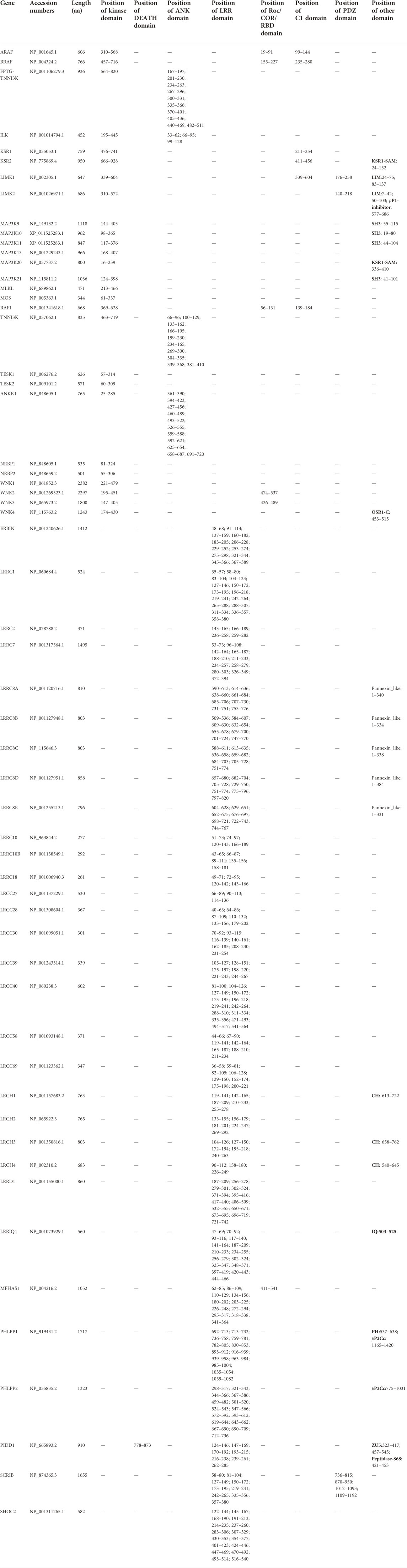
TABLE 1. The overall sequence information of paralogues of human RIPK1–7 in Ensembl genome database.
To address these questions, we used MEGA X to construct an ML phylogenetic tree using the full-length amino acid sequences from RIPK1–7, twenty paralogues with a Kinase domain in the Ensembl database, DSTYK, and four IRAK genes (IRAK1–4). As shown in Figure 3B, RIPK1–4 and RIPK5/ANKK1 cluster together, whereas RIPK6 and RIPK7 branch with other genes (e.g., MOS and MLKL), implying RIPK6 and RIPK7 are distantly related to RIPK1–5. Meanwhile, DSTYK branches with IRAK1–3 and other “paralogues” of RIPK1–3 (e.g., KSR1–2, ARAF, and BRAF), suggesting DSTYK is clearly distinct from RIPK1–7 and the paralogue information in the Ensembl database is inaccurate.
Furthermore, we dissected the evolutionary relationship of homologous regions, Kinase domain across RIPK1–7 and “paralogues”, using a phylogenetic tree and sequence alignment (Figure 4). Similarly, the ML tree of the Kinase domain exhibits RIPK1–5 clusters together and RIPK6 and RIPK7 with DSTYK in a separate clade (Figure 4A). Moreover, Cuny et al., 2021 identified a total of 12 amino acids in RIPK1 to be critical for the function of Kinase domain, such as canonical catalytic elements including P-loops, catalytic Lys, αC helix, Gatekeeper, HRD motif and DLG motif, and activation loop (Cuny and Degterev, 2021). The sequence alignment of Kinase domain by Clustal-W reveals that the number of conserved critical residues is as follows: RIPK4 and MOS have 10; RIPK5/ANKK1, DSTYK and IRAK4 exhibit 9; RIPK2, RIPK3, RIPK6, RIPK7, MAP3K12 and LIMK1 possess 8; MLKL consists 4 (Figure 4B). Because of possible mismatches, Lys (K) and Glu (E) next to the aligned residues in the alignment were also counted. Importantly, both Lys (K) in the catalytic Lys motif and Glu (E) in the αC helix, as the key catalytic site in the Kinase domain, are detected in RIPK1–7 except RIPK3.
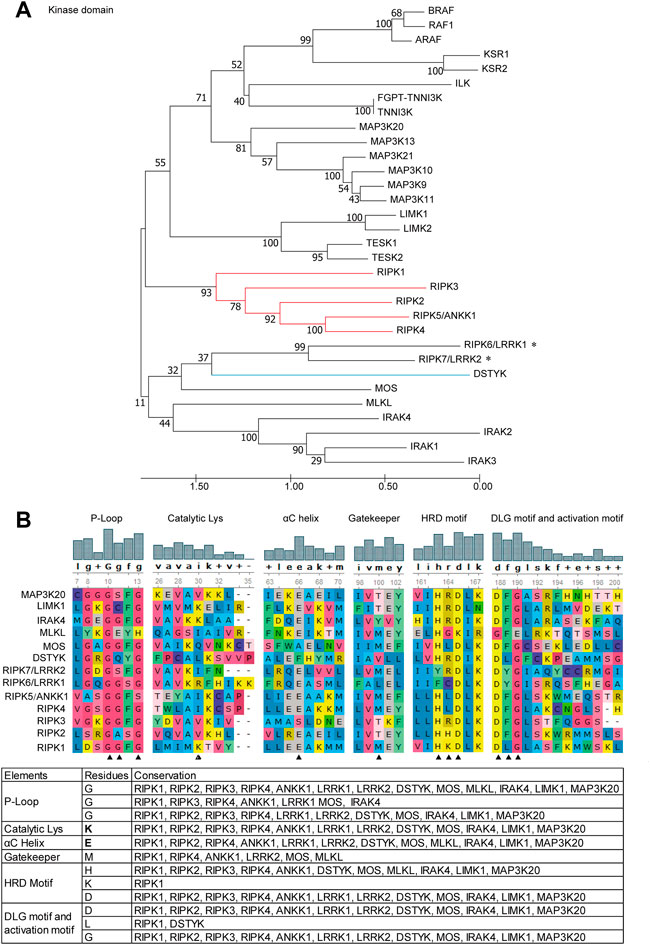
FIGURE 4. Phylogenetic tree and critical residues of Kinase domains from RIPK1–7 and their paralogues (A) ML tree. Branch support values represent a percentage of 100 bootstrap replicates. (B) Alignments of the critical catalytic elements by UGENE using ClustalW analysis. Sequence accession numbers used in this analysis refer to Table 1. * indicates human RIPK6 and RIPK7 genes; the red line indicates RIPK1–5; the blue line indicates DSTYK and IRAK1–3; ▲ indicates critical residues.
Evolutionary analysis of RIPK1–7 across different species
In general, an evolutionary framework can provide more insights into multifaceted and conserved functions of genes. In particular, differences in RIPK1–7 between vertebrates have been poorly characterized, and their evolutionary origins have not been investigated in detail. To define the origin of RIPK1–7, we performed an extensive BLASTP search in NCBI and the JGI database using amino acids of human RIPK1–7 to identify homologs in representative organisms (Tables 2, 3). The homologs of RIPK1–7 are not identified in the bacterial genomes examined, which rules out a prokaryotic origin. It is also possible that the current phylogenetic signal is inadequate to identify ancient orthologs. The ancient eukaryotic homologs of RIPK1–7 can be traced back to protist taxa Cryptophyta (G. theta) that arose almost two billion years ago (Macqueen and Johnston, 2009; Califice et al., 2012). This places the occurrence of RIPK1–7 ahead of the split of animal, plant, and fungal lineages. Although there are no homologs in fungal genomes, the presence of a homolog of RIP kinases is identified in plants (R. argentea). The number of RIPK1–7 homologs varies in different eukaryotic species: seven in human, mouse, and frog (RIPK1–7); 6 in chicken with RIPK3 loss; 6 in sea lamprey (RIPK1, RIPK3, RIPK6, RIPK7, two RIPK2); 8 in green anole and zebrafish (RIPK1–7 with two RIPK2); 19 in amphioxus; one in fruitfly; one in nematode; four in fresh-water polyp; one in soil-dwelling amoeba, two in cryptomonad algae; and one in plant silver myrtle.
To examine the potential evolutionary relationships among RIPK1–7 across different species, we used MEGA X to generate an ML tree using amino acid sequences from representative animals (Figure 5). Chicken Ripk6 and Ripk7 were not included in this tree because they decreased the overall bootstrap values. In total, the tree contains eight respective clades including each of the RIPK genes and amphioxus homologs. The content of each clade is consistent with established taxonomic relationships. The RIPK6 and RIPK7 clades are outgroups of the RIPK1–5 clades, which suggests that RIPK6 and RIPK7 may be more ancient than RIPK1–5. This is also supported by the lack of sequences from plants and invertebrates in the RIPK1–5 clades, except in amphioxus, which is representative of the transition between invertebrates and vertebrates. This phenomenon indicates RIPK1–5 may have arisen from one or more common ancestor and then have experienced two rounds of whole-genome duplication in amphioxus. The common ancestor of early-diverging vertebrates might be closer to the orthologues from cryptomonad algae (G. theta) and soil-dwelling amoeba, according to their location at the base of the RIPK1–5 clades. Meanwhile, amphioxus has 16 homologs in RIPK1–5 clades and three homologs in RIPK6 and RIPK7 clades, revealing amphioxus has undergone extensive lineage-specific duplication during two rounds of whole-genome duplication to produce the species with the most RIP kinase homologous present. In addition, the tree demonstrates that green anole ANKK1 (XP_008123542.1) in the NCBI database should be classified into RIPK3.
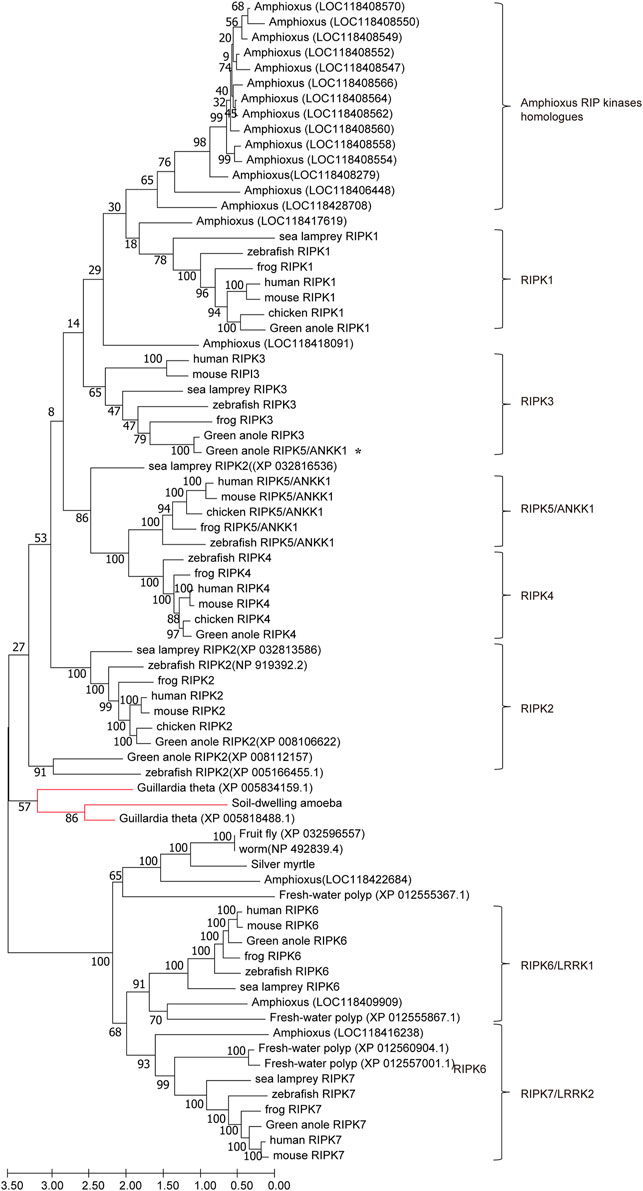
FIGURE 5. ML tree of RIPK1–7 across different species. The bootstrap percentage is shown on the interior branches. Entirely, the tree is divided into eight respective branches according to RIPK members and amphioxus, which is labeled next to the tree. Some vertebrate and invertebrate RIP kinase homologs are shown as species common names followed by GenBank accession numbers to distinguish genes with repeating or unclear names. The remaining homologs/orthologs are shown as species names followed by gene names. All sequence accession numbers used in this tree refer to Tables 2, 3. * indicates green anole ANKK1; the red line indicates sequences from Guillardia theta and soil-dwelling amoeba.
Genomic organization
A comparison of the genomic organization of RIPK1–7 homologs in vertebrates and invertebrates can provide clues to their evolutionary heritage. If different gene members originated historically from the duplication of a region in a common ancestor, other surrounding genes should have been duplicated at the same time (Hedges et al., 2004). Accordingly, we examined the genomic neighborhood surrounding RIPK1–7 in mammals, amphibians, cephalochordates, nematomorpha, and amoebozoa (Figure 6).
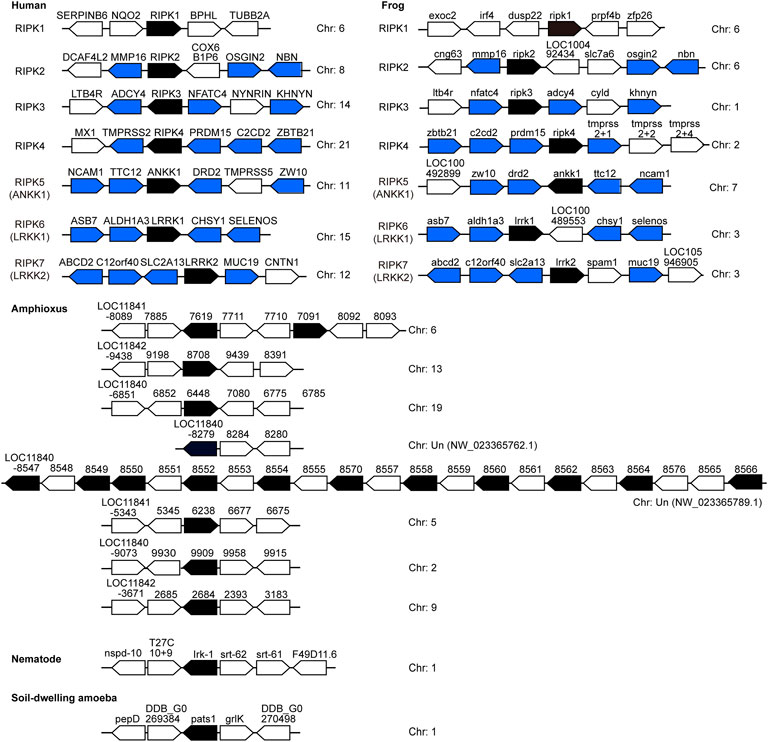
FIGURE 6. Chromosomal disposition of RIP kinase genes and their neighboring genes in the examined animals. Black blocks indicate putatively functional RIP kinases, whereas blue/white blocks represent other genes. Blue blocks indicate genes in common between human and frog. The distance between genes is not scaled to the locations of the chromosomal region. Arrows denote transcription orientation. Sequences refer to Tables 2, 3.
Significantly, no similar surrounding genes were identified between vertebrates and invertebrates (amphioxus) as well as among RIPK1–5 members from both human and frog. This phenomenon suggests the clear proto-orthologs of vertebrate RIPK1–5 in amphioxus are difficult to be established. In human and frog, upstream and downstream genes of RIPK2–7 positioned on different chromosomes demonstrate a strong conserved synteny, indicating a few intra-chromosomal and inter-chromosomal rearrangements for vertebrate RIPK1–7 and RIP kinase evolution prior to the speciation events. However, amphioxus RIP kinase homologs are located in different chromosomes/regions: a single, two or eleven genes in a separate chromosome/region. This suggests an intra-chromosomal duplication, which is supported by the appearance of 14 copies clustered into one clade in Figure 5.
Structural organization of RIPK1–7 from vertebrates and invertebrates
Furthermore, the functional features of RIPK1–7 in vertebrates and invertebrates were identified by SMART as follows: (1) A Kinase domain is present in all RIPK homologs. (2) RHIM and CARD domain are only identified in vertebrate RIPK1, RIPK3, and RIPK2, which indicates that functions regulated by CARD and RHIM domain arose in vertebrates. (3) The combination of ANK and a Kinase domain is observed in vertebrate RIPK4 and RIPK5, while the combination of ANK-LRR-Roc/COR-Kinase is detected in both vertebrate RIPK6 and RIPK7 and invertebrates. (4) Many unique compositions of domain organization occur in amphioxus, such as LRR-Kinase, LRR-DEATH-Kinase, and DEATH-Kinase, as well as new small motif SH3, ZU5, and Zalpha, implying RIP kinase gene family in amphioxus has undergone gene conversion to produce the proto-ortholog of vertebrate RIPK1–5 (Figure 7A).
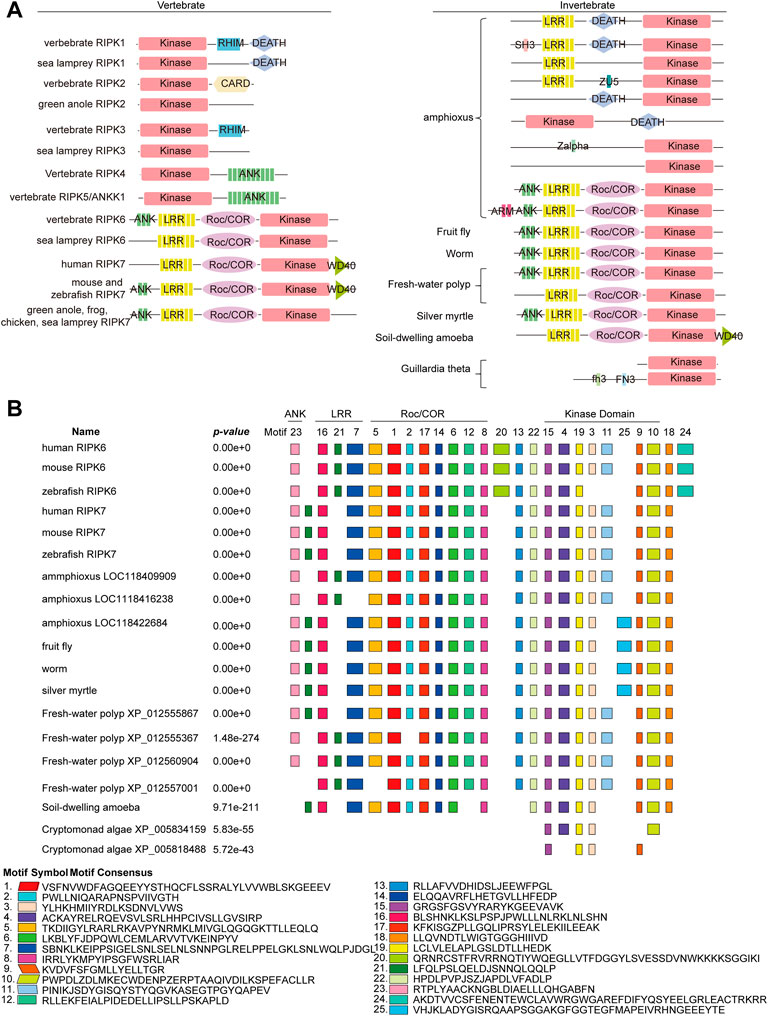
FIGURE 7. Conservation of the domain structure of RIPK1–7 from vertebrates and invertebrates (A) Diagram of the domain structure. (B) Motif composition of RIPK6 and RIPK7 with invertebrate RIP kinase by MEME analysis. Boxes of different colors indicate separate motifs. The best possible matched sequences for motifs are listed below the diagram.
Taking into account the fact that the combination of ANK-LRR-Roc/COR-Kinase is the ancestral architecture of RIPK6 and RIPK7, but its functions are still unclear, we attempted to explore new smaller functional motifs in homologs in RIPK6 and RIPK7 clades using the MEME server. As shown in Figure 7B, eight conserved motifs (motif 3, 4, 9, 10, 11, 15, 19, 25) are detected in the Kinase domain, eight conserved motifs (motif 1, 2, 5, 6, 8, 12, 14, 17) in the Roc/COR domain, three motifs (motif 7, 16, 21) in the LRR domain, and one motif (Koeneke et al., 2020) in ANK. In particular, motif 15 and 19 are highly conserved in Kinase, as well as motif 1, 6, 8, 14 and 17 in Roc/COR domain, and motif 16 and 21 in LRR. Interestingly, motif 13, 22 and 18 are highly conserved, although they are located outside of functional domains. Motif 20 and 24 are only present in vertebrate RIPK6, which suggests they might be specifically involved in distinct vertebrate immune responses. Motif 25 is only observed in invertebrates, indicating it might be unique to invertebrate immune responses.
RIPK3 loss in birds, snakes and early-diverging mammals
In the RIPK3 clade, RIPK3 loss was observed in chicken. Interestingly, we also found RIPK3 loss in many other species, including the complete class of Aves (all 13 birds), the infraclass of Reptilia (4 kinds of snake, tortoise, crocodile), some early-diverging mammals (platypus, koala, common wombat, wallaby, opossum, Tasmanian devil) (Figure 8). A common characteristic of these animals with RIPK3 loss is that they undergo torpor, a physiological phenomenon during cold environmental conditions, when they slow their body metabolism and lower body temperature overnight or the whole season (Dondelinger et al., 2016). Torpor can be considered as a cold ischemia condition followed by reperfusion when body metabolism and temperature are restored (Bogren et al., 2014). Meanwhile, accumulating evidences indicate gene loss in birds is generally associated with physiological features, such as hyperglycemia, high metabolic rate, non-shivering thermogenesis loss, and low glomerular filtration rate (Xiong and Lei, 2021). RIPK3 may be involved in ischemia-reperfusion injury or high metabolic rate through glucose homeostasis, and therefore RIPK3 loss can represent an evolutionary adaptation to the physiological characteristics of torpor (Dondelinger et al., 2016).
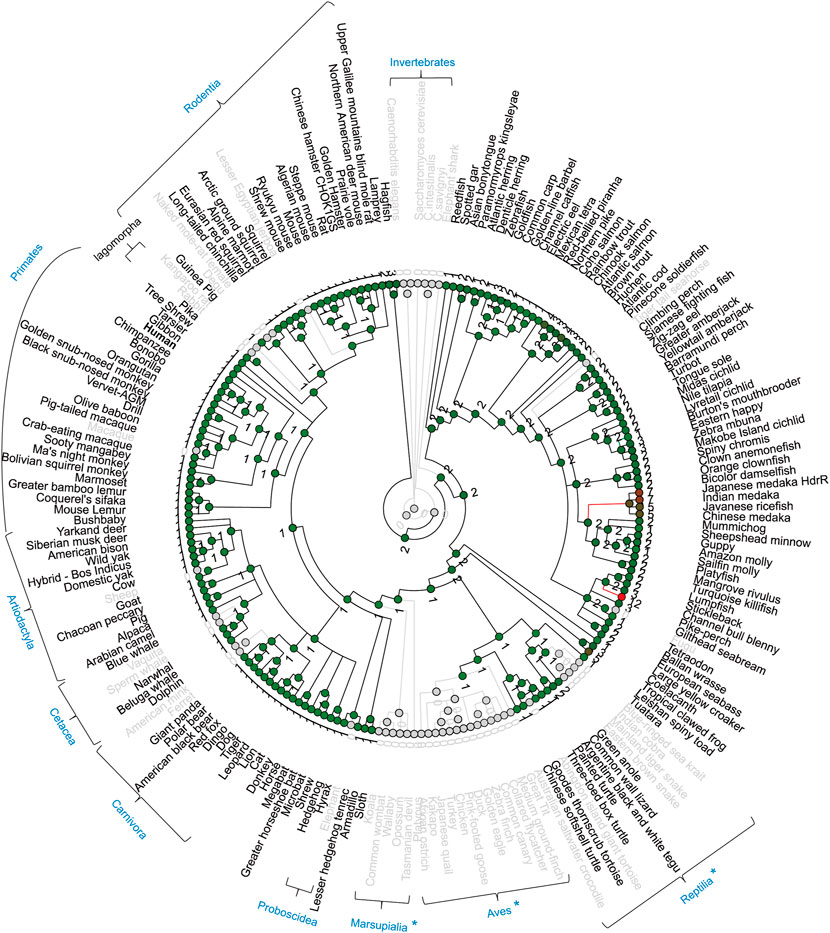
FIGURE 8. Gene gain/loss tree of RIPK3 homologs of different species. The tree is retrieved from Comparative Genomics in the Ensembl database. The black line indicates no significant change. The red line indicates significant expansion. The green line indicates significant contraction. Species in gray are species with no gene. The number of homologs is labeled in the nodes. Gray circles indicate nodes with 0 number. { indicates species taxonomy.
Moreover, possibly because of the incompleteness of the genome sequencing or lineage-specific gene loss/evolution in mammals, RIPK3 loss was also observed in macaque Macaca mulatta (Primates), kangaroo rat Dipodomys ordii (Rodentia), rabbit Oryctolagus cuniculus (Lagomorpha), ferret Mustela putorius furo (Carnivora), sperm whale Physeter catodon (Cetacea), sheep Ovis aries (Artiodactyla), and elephant Loxodonta africana (Proboscidea).
Discussion
Perplexing information on RIPK4–7 in reported studies
Currently, there is some inconsistent information regarding which gene can be considered as RIPK5. Zha et al., 2004 found that DSTYK overexpression can induce caspase-dependent and -independent cell death and DNA fragmentation in 293 cell line and thereby proposed DSTYK as RIPK5 (Zha et al., 2004). Cuny and Degterev 2021 identified that DSTYK/SgK496 had homology to RIPK4, and ANKK1 was most similar to RIPK1 based on the phylogenic tree of the Kinase domain. Thus, both DSTYK and ANKK1 could be considered as RIPK members (Cuny and Degterev, 2021). Yet the majority of public databases describe DSTYK as RIPK5. However, since the analysis of the human kinome by Manning et al. in 2002, RIP kinase researchers have referred to ANKK1/SgK288 as RIPK5 (Manning et al., 2002). In this study, our phylogenetic trees of full-length amino acid sequences (Figure 3B) and Kinase domain (Figure 4A) provide evidence that DSTYK is clearly distinct from RIPK1–7, in contrast to ANKK1.
Meanwhile, it is worth noting that the domain organization of human RIPK6 and RIPK7 differs in different papers. That is, RIPK6 is shown with a WD40 motif by Humphries et al., 2015 and He and Wang, 2018, but without WD40 in Zhang et al., 2009 and Meylan and Tschopp, 2005. RIPK7 is shown with an N-terminal ANK motif by He and Wang, 2018, whereas an N-terminal ANK and ARM (Armadillo) motif is attributed by Humphries et al., 2015, and no ANK is shown by Zhang et al., 2009 and Meylan and Tschopp, 2005. Our Figure 1A with domains predicted by SMART, supports Zhang et al., 2009 and Meylan and Tschopp, 2005 (Meylan and Tschopp, 2005; Zhang et al., 2010; Humphries et al., 2015; He and Wang, 2018).
Overall, future studies would benefit from a clear description of RIPK4–7 to aid in the dissemination of results between different research groups.
Potential function of RIPK4 and RIPK5 (ANKK1) in immune responses
Given the negligible expression of Ripk4 in immune cells (Figure 2A), it is not surprising that the direct role of Ripk4 in immune responses has not been studied. Nevertheless, the close evolutionary relationships of the expression patterns of RIPK4 and RIPK1 in human organs (Figure 1C), and the fact that 10 of 12 critical residues are homologous between RIPK1 and RIPK4 (Figure 4B), demonstrates that RIPK4 may be involved in NF-κB and MAPK activation like RIPK1 in non-immune cell types. This is supported by the observation that RIPK4 overexpression can activate NF-κB and MAPK in 293 T cells and can induce pro-inflammatory cytokine interleukin-8 and chemokine CCL5 and CXCL11 in human oral keratinocytes (Meylan et al., 2002; Kwa et al., 2016).
Nevertheless, the domain structure of ANKK1 is highly similar to RIPK4 with an overall identity of 35% (Meylan and Tschopp, 2005), which suggests that ANKK1 might have a function similar to RIPK4 in the induction of pro-inflammatory cytokines (Meylan et al., 2002; Shin et al., 2008). ANKK1 is dominantly expressed in brain (Supplementary Figure S1), which is consistent with the polymorphism Taq1A that leads to ANKK1 reduced stability being associated with schizophrenia (Habibzadeh et al., 2021). However, immune cell expression by ImmGen (Figure 2A) does not include immune cells from the brain, such as microglia. Therefore, the potential role of ANKK1 in inflammation could not be excluded, especially given the significant association between inflammation and neuropsychiatric disorders (Williams et al., 2022).
Potential function of RIPK6 and RIPK7 in immune responses
RIPK6 and RIPK7 homologs have been identified in early-diverging invertebrate worm, fruitfly and fresh-water polyp, and even plants (Table 3; Figure 5 and Figure 7A). This distinguishes them from RIPK1–3, revealing RIPK6 and RIPK7 might be involved in some evolutionarily conserved signaling pathways, such as cadmium signal transduction pathways in cell homeostasis and the MAPK pathway in innate immune responses (Ausubel, 2005; Chmielowska-Bąk and Deckert, 2012). At the same time, the dominant expression of Ripk7 in innate immune cells and its absence in T cells reveals that Ripk7 may participate preferentially in innate immune responses rather than adaptive immune responses (Figure 2A). RIPK7 mutations and kinase activity play very important roles in the pathogenesis of PD (Berwick et al., 2019; Seegobin et al., 2020), whereas RIPK7 is expressed at low levels in neurons but at high levels in immune cells. Significantly, a recent genome-wide association study demonstrated that genetic polymorphisms in RIPK7 are associated with autoimmune disease multibacillary leprosy and inflammatory bowel disease (Zhang et al., 2009; Hui et al., 2018). Meanwhile, RIPK7 contributes to cytokine production in response to a limited group of bacterial pathogens, but the outcomes and mechanisms mediated by RIPK7 for viral or fungal pathogens are poorly characterized (Eng et al., 2021). Future research on RIPK6 and RIPK7 may shift from neuronal toxicity to inflammation and cell death.
Data availability statement
Publicly available datasets were analyzed in this study. Further inquiries can be requested from the corresponding author.
Author contributions
JY, XL, NL conceived and designed the research. SL, YJ, YL, RH, LP, ZM and JY analyzed and expounded of data for this work. JY wrote the manuscript.
Funding
This research received support from Qilu Young Scholarship (Shandong University) and Shandong Provincial Natural Science Foundation (ZR2021QH198 and ZR2020MH260).
Conflict of interest
The authors declare that the research was conducted in the absence of any commercial or financial relationships that could be construed as a potential conflict of interest.
Publisher’s note
All claims expressed in this article are solely those of the authors and do not necessarily represent those of their affiliated organizations, or those of the publisher, the editors and the reviewers. Any product that may be evaluated in this article, or claim that may be made by its manufacturer, is not guaranteed or endorsed by the publisher.
Supplementary material
The Supplementary Material for this article can be found online at: https://www.frontiersin.org/articles/10.3389/fgene.2022.796291/full#supplementary-material
References
Ausubel, F. M. (2005). Are innate immune signaling pathways in plants and animals conserved? Nat. Immunol. 6 (10), 973–979. doi:10.1038/ni1253
Bähr, C., Rohwer, A., Stempka, L., Rincke, G., Marks, F., and Gschwendt, M. (2000). DIK, a novel protein kinase that interacts with protein kinase Cdelta. cloning, characterization, and gene analysis. J. Biol. Chem. 275 (46), 36350–36357. doi:10.1074/jbc.M004771200
Berwick, D. C., Heaton, G. R., Azeggagh, S., and Harvey, K. (2019). LRRK2 biology from structure to dysfunction: research progresses, but the themes remain the same. Mol. Neurodegener. 14 (1), 49. doi:10.1186/s13024-019-0344-2
Bhat, N., and Fitzgerald, K. A. (2014). Recognition of cytosolic DNA by cGAS and other STING dependent sensors. Eur. J. Immunol. 44, 634–640. doi:10.1002/eji.201344127
Bogren, L. K., Olson, J. M., Carpluk, J., Moore, J. M., and Drew, K. L. (2014). Resistance to systemic inflammation and multi organ damage after global ischemia/reperfusion in the arctic ground squirrel. PLoS One 9 (4), e94225. doi:10.1371/journal.pone.0094225
Califice, S., Baurain, D., Hanikenne, M., and Motte, P. (2012). A single ancient origin for prototypical serine/arginine-rich splicing factors. Plant Physiol. 158 (2), 546–560. doi:10.1104/pp.111.189019
Chang, M. X., Chen, W. Q., and Nie, P. (2010). Structure and expression pattern of teleost caspase recruitment domain (CARD) containing proteins that are potentially involved in NF-kappaB signalling. Dev. Comp. Immunol. 34 (1), 1–13. doi:10.1016/j.dci.2009.08.002
Chen, G., Shaw, M. H., Kim, Y. G., and Nunez, G. (2009). NOD-Like receptors: Role in innate immunity and inflammatory disease. Annu. Rev. Pathol. 4, 365–398. doi:10.1146/annurev.pathol.4.110807.092239
Chmielowska-Bąk, J., and Deckert, J. (2012). A common response to common danger? comparison of animal and plant signaling pathways involved in cadmium sensing. J. Cell Commun. Signal. 6 (4), 191–204. doi:10.1007/s12079-012-0173-3
Cuchet-Lourenço, D., Eletto, D., Wu, C., Plagnol, V., Papapietro, O., Curtis, J., et al. (2018). Biallelic RIPK1 mutations in humans cause severe immunodeficiency, arthritis, and intestinal inflammation. Science 361, 810–813. doi:10.1126/science.aar2641
Cuny, G. D., and Degterev, A. (2021). RIPK protein kinase family: Atypical lives of typical kinases. Semin. Cell Dev. Biol. 109, 96–105. doi:10.1016/j.semcdb.2020.06.014
Dachsel, J. C., Nishioka, K., Vilariño-Güell, C., Lincoln, S. J., Soto-Ortolaza, A. I., Kachergus, J., et al. (2010). Heterodimerization of lrrk1-lrrk2: Implications for LRRK2-associated Parkinson disease. Mech. Ageing Dev. 131 (3), 210–214. doi:10.1016/j.mad.2010.01.009
Dondelinger, Y., Hulpiau, P., Saeys, Y., Bertrand, M. J. M., and Vandenabeele, P. (2016). An evolutionary perspective on the necroptotic pathway. Trends Cell Biol. 26 (10), 721–732. doi:10.1016/j.tcb.2016.06.004
Eng, V. V., Wemyss, M. A., and Pearson, J. S. (2021). The diverse roles of RIP kinases in host-pathogen interactions. Semin. Cell Dev. Biol. 109, 125–143. doi:10.1016/j.semcdb.2020.08.005
España-Serrano, L., Guerra Martín-Palanco, N., Montero-Pedrazuela, A., Pérez-Santamarina, E., Vidal, R., García-Consuegra, I., et al. (2017). The addiction-related protein ANKK1 is differentially expressed during the cell cycle in neural Precursors. Cereb. Cortex 27 (5), 2809–2819. doi:10.1093/cercor/bhw129
Festjens, N., Berghe, V. T., Cornelis, S., and Vandenabeele, P. (2007). RIP1, a kinase on the crossroads of a cell’s decision to live or die. Cell Death Differ. 14, 400–410. doi:10.1038/sj.cdd.4402085
Habibzadeh, P., Nemati, A., Dastsooz, H., Taghipour-Sheshdeh, A., Mariam Paul, P., Sahraian, A., et al. (2021). Investigating the association between common DRD2/ANKK1 genetic polymorphisms and schizophrenia: a meta-analysis. J. Genet. 100, 59. doi:10.1007/s12041-021-01306-1
He, S., and Wang, X. (2018). RIP kinases as modulators of inflammation and immunity. Nat. Immunol. 19 (9), 912–922. doi:10.1038/s41590-018-0188-x
Hedges, S. B., Blair, J. E., Venturi, M. L., and Shoe, J. L. (2004). A molecular timescale of eukaryote evolution and the rise of complex multicellular life. BMC Evol. Biol. 4, 2. doi:10.1186/1471-2148-4-2
Hou, J., Pang, Y., and Li, Q. (2020). Comprehensive evolutionary analysis of lamprey TNFR-associated factors (TRAFs) and receptor-interacting protein kinase (RIPKs) and insights into the functional characterization of TRAF3/6 and RIPK1. Front. Immunol. 11, 663. doi:10.3389/fimmu.2020.00663
Hui, K. Y., Fernandez-Hernandez, H., Hu, J., Schaffner, A., Pankratz, N., Hsu, N. Y., et al. (2018). Functional variants in the LRRK2 gene confer shared effects on risk for Crohn's disease and Parkinson's disease. Sci. Transl. Med. 10 (423), eaai7795. doi:10.1126/scitranslmed.aai7795
Humphries, F., Yang, S., Wang, B., and Moynagh, P. N. (2015). RIP kinases: key decision makers in cell death and innate immunity. Cell Death Differ. 22 (2), 225–236. doi:10.1038/cdd.2014.126
Ishizawa, Y. H., Tamura, K., Yamaguchi, T., Matsumoto, K., Komiyama, M., Takamatsu, N., et al. (2006). Xenopus death-domain-containing proteins FADD and RIP1 synergistically activate JNK and NF-kappaB. Biol. Cell 98 (8), 465–478. doi:10.1042/BC20050091
Jurynec, M. J., Sawitzke, A. D., Beals, T. C., Redd, M. J., Stevens, J., Otterud, B., et al. (2018). A hyperactivating proinflammatory RIPK2 allele associated with early-onset osteoarthritis. Hum. Mol. Genet. 27 (13), 2383–2391. doi:10.1093/hmg/ddy132
Koeneke, A., Ponce, G., Troya-Balseca, J., Palomo, T., and Hoenicka, J. (2020). Ankyrin repeat and kinase domain containing 1 gene, and addiction vulnerability. Int. J. Mol. Sci. 21 (7), 2516. doi:10.3390/ijms21072516
Korr, D., Toschi, L., Donner, P., Pohlenz, H. D., Kreft, B., and Weiss, B. (2006). LRRK1 protein kinase activity is stimulated upon binding of GTP to its Roc domain. Cell. Signal. 18 (6), 910–920. doi:10.1016/j.cellsig.2005.08.015
Kwa, M. Q., Scholz, G. M., and Reynolds, E. C. (2016). RIPK4 activates an IRF6-mediated proinflammatory cytokine response in keratinocytes. Cytokine 83, 19–26. doi:10.1016/j.cyto.2016.03.005
Li, Y., Führer, M., Bahrami, E., Socha, P., Klaudel-Dreszler, M., Bouzidi, A., et al. (2019). Human RIPK1 deficiency causes combined immunodeficiency and inflammatory bowel diseases. Proc. Natl. Acad. Sci. U. S. A. 116, 970–975. doi:10.1073/pnas.1813582116
Li, J., Yao, Y., and Tian, Y. P. (2021). Ferroptosis: A trigger of proinflammatory state Progression to immunogenicity in necroinflammatory disease. Front. Immunol. 12, 701163. doi:10.3389/fimmu.2021.701163
Liu, L., Liu, Y., Cheng, X., and Qiao, X. (2021). The alleviative effects of quercetin on cadmium-induced necroptosis via inhibition ROS/iNOS/NF-κB pathway in the chicken brain. Biol. Trace Elem. Res. 199 (4), 1584–1594. doi:10.1007/s12011-020-02563-4
Loo, Y. M., and Gale, M. (2011). Immune signaling by RIG-I-like receptors. Immunity 34, 680–692. doi:10.1016/j.immuni.2011.05.003
Macqueen, D. J., and Johnston, I. A. (2009). Evolution of the multifaceted eukaryotic akirin gene family. BMC Evol. Biol. 69, 34. doi:10.1186/1471-2148-9-34
Manning, G., Whyte, D. B., Martinez, R., Hunter, T., and Sudarsanam, S. (2002). The protein kinase complement of the human genome. Science 298 (5600), 1912–1934. doi:10.1126/science.1075762
Meng, H., Liu, Z., Li, X., Wang, H., Jin, T., Wu, G., et al. (2018). Death-domain dimerization-mediated activation of RIPK1 controls necroptosis and RIPK1-dependent apoptosis. Proc. Natl. Acad. Sci. U. S. A. 115 (9), E2001–E2009. doi:10.1073/pnas.1722013115
Meylan, E., and Tschopp, J. (2005). The RIP kinases: crucial integrators of cellular stress. Trends biochem. Sci. 30 (3), 151–159. doi:10.1016/j.tibs.2005.01.003
Meylan, E., Martinon, F., Thome, M., Gschwendt, M., and Tschopp, J. (2002). RIP4 (DIK/PKK), a novel member of the RIP kinase family, activates NF-kappa B and is processed during apoptosis. EMBO Rep. 3 (12), 1201–1208. doi:10.1093/embo-reports/kvf236
Moriwaki, K., and Chan, F. K. (2017). The inflammatory signal adaptor RIPK3: Functions beyond necroptosis. Int. Rev. Cell Mol. Biol. 328, 253–275. doi:10.1016/bs.ircmb.2016.08.007
O’Neill, L. A., Golenbock, D., and Bowie, A. G. (2013). The history of Toll-like receptors - redefining innate immunity. Nat. Rev. Immunol. 13, 453–460. doi:10.1038/nri3446
Ofengeim, D., and Yuan, J. (2013). Regulation of RIP1 kinase signalling at the crossroads of inflammation and cell death. Nat. Rev. Mol. Cell Biol. 14 (11), 727–736. doi:10.1038/nrm3683
Ohira, K., Yokota, H., Hirano, S., Nishimura, M., Mukai, H., Horikoshi, T., et al. (2022). DRD2 Taq1A polymorphism-related brain volume changes in Parkinson's disease: Voxel-based morphometry. Park. Dis. 2022, 8649195. doi:10.1155/2022/8649195
Park, H. S., Liu, G., Liu, Q., and Zhou, Y. (2018). Swine influenza virus induces RIPK1/DRP1-mediated interleukin-1 beta production. Viruses 10 (8), 419. doi:10.3390/v10080419
Piccoli, G., Condliffe, S. B., Bauer, M., Giesert, F., Boldt, K., Astis, S. D., et al. (2011). LRRK2 controls synaptic vesicle storage and mobilization within the recycling pool. J. Neurosci. 31 (6), 2225–2237. doi:10.1523/JNEUROSCI.3730-10.2011
Schulte, E. C., Ellwanger, D. C., Dihanich, S., Manzoni, C., Stangl, K., Schormair, B., et al. (2014). Rare variants in LRRK1 and Parkinson's disease. Neurogenetics 15 (1), 49–57. doi:10.1007/s10048-013-0383-8
Seegobin, S. P., Heaton, G. R., Liang, D., Choi, I., Ramirez, M. B., Tang, B., et al. (2020). Progress in LRRK2-associated Parkinson’s disease animal models. Front. Neurosci. 14, 674. doi:10.3389/fnins.2020.00674
Sheehan, P., and Yue, Z. (2019). Deregulation of autophagy and vesicle trafficking in Parkinson's disease. Neurosci. Lett. 697, 59–65. doi:10.1016/j.neulet.2018.04.013
Shin, N., Jeong, H., Kwon, J., Heo, H. Y., Kwon, J. J., Yun, H. J., et al. (2008). LRRK2 regulates synaptic vesicle endocytosis. Exp. Cell Res. 314 (10), 2055–2065. doi:10.1016/j.yexcr.2008.02.015
Silke, J., Rickard, J. A., and Gerlic, M. (2015). The diverse role of RIP kinases in necroptosis and inflammation. Nat. Immunol. 16 (7), 689–697. doi:10.1038/ni.3206
Sun, X., Lee, J., Navas, T., Baldwin, D. T., Stewart, T. A., and Dixit, V. M. (1999). RIP3, a novel apoptosis-inducing kinase. J. Biol. Chem. 274 (24), 16871–16875. doi:10.1074/jbc.274.24.16871
Thiébaut, R., Douchin, V., Jung, C., Merlin, F., Colombel, J. F., Lemann, M., et al. (2011). RIP2 polymorphisms in inflammatory bowel diseases. Inflamm. Bowel Dis. 17 (4), 1055. doi:10.1002/ibd.21406
Wallach, D., Kang, T. B., and Kovalenko, A. (2014). Concepts of tissue injury and cell death in inflammation: a historical perspective. Nat. Rev. Immunol. 14 (1), 51–59. doi:10.1038/nri3561
Williams, J. A., Burgess, S., Suckling, J., Lalousis, P. A., Batool, F., Griffiths, S. L., et al. (2022). Inflammation and brain structure in schizophrenia and other neuropsychiatric disorders: A mendelian randomization study. JAMA Psychiatry 79 (5), 498–507. doi:10.1001/jamapsychiatry.2022.0407
Xie, X., Cao, Y., Dai, Y., Chen, Z., Wei, J., Tan, Y., et al. (2020). Black carp RIPK1 negatively regulates MAVS-mediated antiviral signaling during the innate immune activation. Dev. Comp. Immunol. 109, 103726. doi:10.1016/j.dci.2020.103726
Xiong, Y., and Lei, F. (2021). SLC2A12 of SLC2 gene family in bird provides functional compensation for the loss of SLC2A4 gene in other vertebrates. Mol. Biol. Evol. 38 (4), 1276–1291. doi:10.1093/molbev/msaa286
Xu, D., Jin, T., Zhu, H., Chen, H., Ofengeim, D., Zou, C., et al. (2018). TBK1 suppresses RIPK1-driven apoptosis and inflammation during development and in aging. Cell 174 (6), 1477–1491.e19. doi:10.1016/j.cell.2018.07.041
Zha, J., Zhou, Q., Xu, L. G., Chen, D., Li, L., Zhai, Z., et al. (2004). RIP5 is a RIP-homologous inducer of cell death. Biochem. Biophys. Res. Commun. 319, 298–303. doi:10.1016/j.bbrc.2004.04.194
Zhang, F. R., Huang, W., Chen, S. M., Sun, L. D., Liu, H., Li, Y., et al. (2009). Genome wide association study of leprosy. N. Engl. J. Med. 361 (27), 2609–2618. doi:10.1056/NEJMoa0903753
Keywords: RIP kinases, evolution, comparative analysis, immune responses, ripk3
Citation: Lv S, Jiang Y, Li Y, Huang R, Peng L, Ma Z, Lu N, Lin X and Yan J (2022) Comparative and evolutionary analysis of RIP kinases in immune responses. Front. Genet. 13:796291. doi: 10.3389/fgene.2022.796291
Received: 19 November 2021; Accepted: 01 August 2022;
Published: 03 October 2022.
Edited by:
Vangelis Kondylis, University Hospital of Cologne, GermanyReviewed by:
Roger Holmes, Griffith University, AustraliaYuanyue Li, College of Biological Sciences, University of California, Davis, United States
Copyright © 2022 Lv, Jiang, Li, Huang, Peng, Ma, Lu, Lin and Yan. This is an open-access article distributed under the terms of the Creative Commons Attribution License (CC BY). The use, distribution or reproduction in other forums is permitted, provided the original author(s) and the copyright owner(s) are credited and that the original publication in this journal is cited, in accordance with accepted academic practice. No use, distribution or reproduction is permitted which does not comply with these terms.
*Correspondence: Nan Lu, bHVuYW5Ac2R1LmVkdS5jbg==; Xiaoying Lin, eGlhb3lpbmdsaW5Ac2R1LmVkdS5jbg==; Jie Yan, anlhbkBzZHUuZWR1LmNu