- 1Animal Science Faculty, Universidad de Ciencias Aplicadas y Ambientales (U.D.C.A), Bogotá, Colombia
- 2PhD Program in Tropical Health and Development, Universidad de Salamanca, Salamanca, Spain
- 3Molecular Biology and Immunology Department, Fundación Instituto de Inmunología de Colombia (FIDIC), Bogotá, Colombia
- 4MSc Program in Microbiology, Universidad Nacional de Colombia, Bogotá, Colombia
- 5Health Sciences Division, Main Campus, Universidad Santo Tomás, Bogotá, Colombia
- 6Microbiology Department, Faculty of Medicine, Universidad Nacional de Colombia, Bogotá, Colombia
The major histocompatibility complex (MHC) exerts great influence on responses to infectious diseases and vaccination due to its fundamental role in the adaptive immune system. Knowledge about MHC polymorphism distribution among breeds can provide insights into cattle evolution and diversification as well as population-based immune response variability, thus guiding further studies. Colombian Simmental and Simbrah cattle’s BoLA-DRB3 genetic diversity was compared to that of taurine and zebuine breeds worldwide to estimate functional diversity. High allele richness was observed for Simmental and Simbrah cattle; nevertheless, high homozygosity was associated with individual low sequence variability in both the β1 domain and the peptide binding region (PBR), thereby implying reduced MHC-presented peptide repertoire size. There were strong signals of positive selection acting on BoLA-DRB3 in all populations, some of which were poorly structured and displayed common alleles accounting for their high genetic similarity. PBR sequence correlation analysis suggested that, except for a few populations exhibiting some divergence at PBR, global diversity regarding potential MHC-presented peptide repertoire could be similar for the cattle populations analyzed here, which points to the retention of functional diversity in spite of the selective pressures imposed by breeding.
Introduction
The major histocompatibility complex (MHC) plays an important effector role in the adaptive immune response (Rock et al., 2016). This particular system offers a unique opportunity for addressing functional and evolutionary diversity issues in many species (Trtkova et al., 1995). The MHC is formed by a group of loci encoding specific cell surface glycoproteins which are necessary for T-lymphocyte antigen peptide recognition (Rock et al., 2016). MHC class I proteins are expressed by all nucleated cells and are related to presenting antigens to CD8+ T-lymphocytes processed in the intracellular compartment, thereby eliciting cytotoxic responses (Neefjes et al., 2011; Rock et al., 2016). By contrast, MHC class II proteins are expressed by professional antigen-presenting cells and are associated with the presentation of extracellular antigen peptides to CD4+ T-lymphocytes, triggering cellular or humoral responses against various pathogens (Neefjes et al., 2011; Rock et al., 2016).
The MHC in cattle has been called the bovine leukocyte antigen (BoLA) and the genes encoding the expression of class II antigen presentation (DR and DQ)-related molecules are located in chromosome 23 IIa subregion (Ellis, 2004). BoLA-DR consists of the DRA monomorphic locus and three DRB loci, of which BoLA-DRB3—characterized by a high degree of polymorphism, with 330 different alleles reported to date—is the only one which has been described as functional (Maccari et al., 2017). Such polymorphisms are concentrated in the second exon which encodes the peptide binding region (PBR) β1 domain and has been used for determining BoLA-DRB3 alleles (Sigurdardóttir et al., 1991). Such high variability determines the amino acids (aa) forming PBR binding pockets, influencing the peptides presented on MHC for different alleles and setting different repertoires modulating the immune response (Ellis, 2004; Baxter et al., 2009). BoLA-DRB3 diversity could be used for estimating potential peptide-binding repertoire size, based on the assumption that highly divergent alleles are associated with broader peptide spectra (Klein et al., 2007).
Assessing MHC genetic variability is of interest for animal science researchers, breeders, and evolutionary biologists. Variations regarding susceptibility to infectious diseases (Dietz et al., 1997; Acosta-Rodríguez et al., 2005; Martinez et al., 2006; Nascimento et al., 2006; Kulberg et al., 2007; Juliarena et al., 2008; Nikbakht Brujeni et al., 2016; Carignano et al., 2017), vaccine response (Bacon and Witter, 1995; García-Briones et al., 2000; Rupp et al., 2007; Baxter et al., 2009; Gowane et al., 2013) and production traits (Sharif et al., 1999; Rupp et al., 2007) have been associated with different BoLA-DRB3 alleles, and information regarding their frequency has thus been used for running infectious disease control programs (Maillard et al., 2003). MHC variability patterns reflect evolutionary processes such as adaptation, selection (natural, sexual or artificial) and genetic diversity within and between populations (Trtkova et al., 1995; Edwards and Hedrick, 1998; Uinuk-Ool et al., 2002; Ujvari and Belov, 2011). Some studies have shown that decreased MHC variability (often higher rates than of neutral loci (Sutton et al., 2011)) might be caused by population bottlenecks (Bollmer et al., 2011; Mason et al., 2011; Babik et al., 2012; Taylor et al., 2012; Zhang et al., 2016), while others have shown that a high level of diversity could be maintained by pathogen-driven balancing selection or other mechanisms, despite extreme population decline (Mikko et al., 1997; Edwards and Hedrick, 1998; Garrigan and Hedrick, 2001; Aguilar et al., 2004; Borg et al., 2011; Galaverni et al., 2013; Moutou et al., 2013; Newhouse and Balakrishnan, 2015). Such information can be used for prioritizing other types of study, such as immunopeptidomic or binding affinity assays used for developing peptide-MHC in silico interaction predictive algorithms that could provide feedback regarding immune system response and evolution (Nielsen et al., 2008; Nielsen et al., 2018).
The Simmental is a cattle (Bos taurus) breed that was selected in North America and Europe, mainly for increasing meat production efficiency (Amaya et al., 2020). The introduction of Simmental to Colombia 5 decades ago aimed to increase both milk and beef production by artificial insemination-based genetic improvement schemes using semen from proven bulls in North America and Europe (Amaya et al., 2020). Simbrah is considered a composite breed developed to combine Brahman cattle (Bos indicus) adaptability, maternal instinct, hardiness and disease resistance with Simmental fertility, milk production and beef quality (Goszczynski et al., 2018; Amaya et al., 2020). Most tropical countries where Simbrah cattle occur have chosen a different breeding strategy, producing animals with different percentages of Zebuine genes, ranging from 1/4 (25%) to 5/8 (62.5%) based on the requirement of particular features, such as better adaptation to humid environments (Agung et al., 2016).
Despite recent advances in exploring BoLA-DRB3 genetic diversity in cattle, a significant amount of breeds and crossbreeds still remain uncharacterized (Takeshima et al., 2003; Giovambattista et al., 2013; Takeshima et al., 2014; Takeshima et al., 2015; Takeshima et al., 2018). The aim of this study, therefore, was to describe for the first time BoLA-DRB3 genetic diversity in the Colombian Simmental breed and its common zebuine cross, Simbrah, comparing it with that of worldwide taurine and zebuine breeds to assess the impact on potential peptide-binding repertoire size and divergence. Such new MHC diversity information will assist in introducing appropriate breeding schemes, guiding further MHC studies.
Materials and Methods
Study Population and DNA Extraction
Whole blood was collected from the coccygeal or jugular veins of 130 Simmental (N = 67; 5 farms) and Simbrah cattle (N = 60; 5 farms) (Supplementary Data S1), stored in EDTA-containing vacutainer tubes. Bovines were selected from extensive production systems from Colombia’s main breeding regions characterized by a reduced number of purebred animals per farm. The herds and purebred animals analyzed were randomly sampled avoiding related individuals. Genomic DNA (gDNA) was extracted using the PureLink Genomic DNA Mini Kit (Invitrogen, Carlsbad, CA, United States) and following the manufacturer’s instructions. Previous allelic richness data (Greenbaum et al., 2014) of 14 taurine and zebuine populations from Asia, South America and Europe were included for comparison (Table 1). This study was carried out following the protocol approved by the Universidad de Ciencias Aplicadas y Ambientales’ (U.D.C.A) Animal Research Ethics Committee (minutes No.201901).
DNA Amplification and Sequencing
BoLA-DRB3 exon 2 was amplified with primers DRB3F (5′-TCCCGCATTGGTGGGTGT-3′) and DRB3R (5′-CTCCACACTGGCCGTCCAC-3′) (Ledwidge et al., 2001). The PCR mixture contained 1X Pfx amplification buffer, 300 mM of each dNTP, 0.45 mM of each primer, 1 mM MgSO4, 1 U Platinum Pfx DNA Polymerase (Invitrogen) and 50 ng gDNA, in a 50 ml final volume. Two independent reactions were performed for each sample, following previous recommendations to avoid chimeric product formation (Lenz and Becker, 2008). The thermal profile consisted of a denaturation step at 94°C for 5 min followed by 30 cycles of 94°C for 30 s, 64°C for 30 s and 68°C for 1 min, with no final extension. Wizard SV Gel and PCR Clean-Up System (Promega, Madison, WI, United States) were used for purifying the amplicons according to the manufacturer’s instructions prior to sequencing both directions using the BigDye Terminator Kit.
Sequence Data Analysis
CLC Main Workbench (CLC bio, Aarhus, Denmark) was used for assembling and editing each sequence independently. Polymorphic positions were recognized for producing a final consensus sequence containing IUPAC ambiguity codes. HAPLOFINDER (Miltiadou et al., 2003) was used for assigning each animal’s genotype by comparing the obtained sequences to the BoLA-DRB3 allele sequences reported in the IPD-MHC database (Maccari et al., 2017), as previously indicated (Baxter et al., 2008).
Sequence Diversity, Hardy-Weinberg Equilibrium and Selection Signatures
The number of alleles (Na) and allele frequencies were manually obtained by direct counting using the maximum likelihood method for estimating standard errors for allele frequencies according to Li (Li, 1976). Nei and Chesser’s method (Nei, 1978) was used for calculating the observed heterozygosity (ho) and ARLEQUIN v.3.5 (Excoffier and Lischer, 2010) for estimating the unbiased expected heterozygosity (he). The correlation between sample size and these estimators was used for assessing their dependence. Allele richness (a measure of the average amount of alleles per locus) was also used for comparing the number of alleles found in each population independently from sample size (Greenbaum et al., 2014). The FIS index (Weir and Cockerham, 1984) was estimated for determining potential departures from Hardy-Weinberg equilibrium using the exact test of significance implemented in GENEPOP v.4.5.0 (Rousset, 2008). GENEDOC v.2.7 (Nicholas, 1997) was used for calculating identity and similarity percentages (as assessed by the BLOSUM62 substitution matrix) for all genotypes observed within populations for the whole β1 domain and PBR positions [considering the previously reported 31 putative positions constituting the MHC-DRB PBR (Suárez et al., 2006)]. PBR sequence logos, along with PBR sequence correlation between populations, were analyzed to further evaluate differences in potential MHC-presented peptide repertoire among cattle populations. MEGA X (Kumar et al., 2018) was used for calculating the average amount of synonymous (dS) and nonsynonymous (dN) substitutions per site by Nei-Gojobori’s method with Jukes-Cantor correction. The Z-test was used for assessing dN/dS ratio significance. Codons subject to positive selection were inferred using maximum likelihood (FEL (Kosakovsky Pond and Frost, 2005)) and Bayesian (MEME (Murrell et al., 2012)) methods using the Datamonkey web server (Delport et al., 2010), using a p-value <0.1 as significant threshold.
Population Structure, Genetic Differentiation and PBR Similarity Correlation
Population structure and genetic differentiations between populations were evaluated by calculating pairwise FST statistics (Weir and Cockerham, 1984) using ARLEQUIN. POPTREE v.2 (Takezaki et al., 2010) was used for calculating genetic distances (DA) (Nei, 1978) from allele frequency data and PAST v.3.2 (Hammer et al., 2001) for generating an allele frequency-based principal component analysis (PCA). WebLogo web server (Crooks et al., 2004) was used to construct a logo for PBR positions for each population using BLOSUM62 substitution matrix for each position including all alleles observed within populations. A 620-coordinate vector (31 positions x 20 possible aa genotypes) representing each PBR population was used for calculating Pearson correlation coefficients using R package amap (Ihaka and Gentleman, 1996), while R package ape was used to construct an UPGMA dendrogram (Paradis et al., 2004). Mitochondrial DNA D-loop sequences from individuals of several of the populations analyzed here were recovered from GenBank (Supplementary Data S2) and used for evaluating MHC allele distribution based on genetic affinity between populations. A maximum likelihood phylogeny was built using Tamura-3-parameter model (best fit model) for mitochondrial DNA and JTT model for BoLA-DRB3 aa sequences using MEGA X.
Results
BoLA-DRB3 Allele Distribution in Colombian Simmental and Simbrah Cattle
Sixty BoLA-DRB3 alleles were identified in both focal populations: 37 in Simmental and 43 in Simbrah cattle (GenBank accession numbers OM100952-OM101010). No new alleles were detected. Three alleles (BoLA-DRB3*002:01, 005:03 and 012:01) occurred with >5% frequency in both populations and seven (BoLA-DRB3*005:01, 008:01, 010:01, 013:01, 015:01, 016:01 and 022:01) only in the Simmental breed. Four alleles (BoLA-DRB3*002:01, 005:03, 012:01 and 022:01) occurred with >5% frequency in Simbrah, accounting for 31.67% of cumulated frequency, whereas 10 alleles (BoLA-DRB3*002:01, 005:01, 005:03, 008:01, 010:01, 012:01, 013:01, 015:01, 016:01 and 030:01) with >5% frequency, accounting for 61.94% cumulative frequency, occurred in Simmental. Twenty alleles were shared by both cattle populations, accounting for 64.7% of their mean cumulative allele frequency (summation of weighted mean allele frequency for all shared alleles) (Table2).
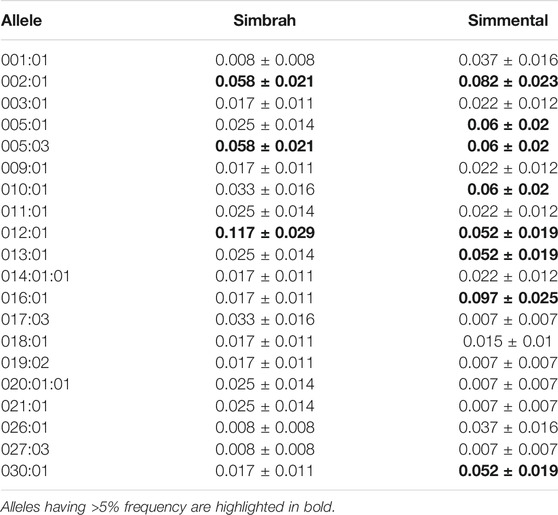
TABLE 2. Allele frequency and standard error for BoLA-DRB3 alleles common to Simmental and Simbrah cattle.
BoLA-DRB3 Genetic Diversity, Hardy-Weinberg Equilibrium and Selection Pattern
The number of alleles corrected for sample size effect (Rs) showed that Simmental and Simbrah had a high genetic diversity, similar to what was observed for Colombian Normande and Philippine populations (Table 3). Sample size appeared to have little effect on both ho (r = 0.36) and he (r = 0.06), highlighting these estimates as being good proxy of BoLA-DRB3 diversity in the populations analyzed here. Colombian Simmental, Colombian Normande, Spanish Morucha and Colombian Simbrah had the lowest ho and the highest he values, in contrast with the results for other breeds (Table 3; Supplementary Data S1). Consequently, the highest departures from Hardy-Weinberg equilibrium were observed in these four breeds, as evidenced by the statistically significant FIS fixation index (Table 3). These results indicated significant heterozygote deficiency regarding the BoLA-DRB3 locus in Colombian Simmental and Colombian Simbrah cattle.
Whole β1 domain identity ranged from 82.45% (Philippine Brahman) to 88.95% (Colombian Normande), with Colombian Simmental (88.79%) and Simbrah (86.33%) displayed some of the highest values (Table 4). Bolivian Holstein and Colombian Normande had the lowest (87.45%) and the highest (92.5%) whole β1 domain similarity, respectively. PBR identity and similarity differences were broader than those for the whole β1 domain. The former ranged from 65.87% (Peruvian Holstein) to 80.81% (Spanish Morucha), whereas the latter ranged from 75.18% (Bolivian Holstein) to 87.59% (Spanish Morucha). Colombian Simmental and Simbrah displayed some of the highest identity and similarity values regarding both whole β1 domain and PBR.
Average dN was significantly higher than average dS for Colombian Simmental and Simbrah populations, similar to what was observed across all the other populations (Table 3). Moreover, codons 10, 11, 12, 26, 30, 32, 37, 57, 70, 71, 74, 77 and 78 were identified as sites under diversifying selection, most of which (11, 12, 26, 30, 37, 57, 70, 71, 74 and 78) were PBR-related. Mitochondrial DNA clusters were mostly formed by individuals of one to four breeds. Infrequently occurring allele lineages (i.e., BoLA-DRB3*025:01:02, 025:02, 025:01:01, 037:01 and 039:01 in most populations) or moderately occurring ones (i.e., BoLA-DRB3*030:01, 030:02, 036:01, 023:01 and 044:01) were identified in populations that are not closely related (Supplementary Data S3 and Supplementary Data S4).
Population Structure and Genetic Differentiation Based on BoLA-DRB3 Gene
Pairwise FST values ranged from −0.0009 (Chilean Holstein with Peruvian Holstein) to 0.1185 (Peruvian Holstein with Bolivian Nellore) (Figure 1A: Supplementary Data S5). All comparisons were statistically significant, except for Chilean with Peruvian Holstein and Argentinian with Paraguayan Holstein. Two groups with low FST values were mainly observed (Figure 1A); the first consisted of Holstein populations (0.0088 mean FST value), while the second was formed by Philippine, Colombian Simmental, Colombian Simbrah, Bolivian Yacumeño and Colombian Normande populations (0.0208 mean FST value). Differences between Colombian Simmental and Simbrah were also observed regarding Argentinian, Peruvian, Chilean and Paraguayan Holstein.
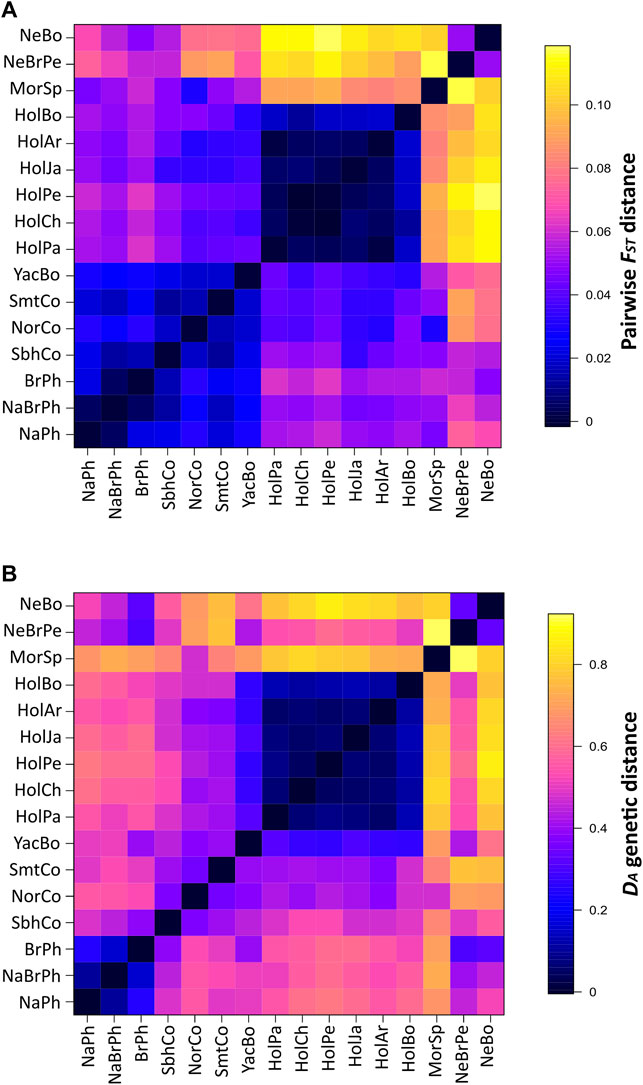
FIGURE 1. Pairwise FST and DA genetic distances between populations. Symmetrical heat maps for pairwise FST values (A) and DA genetic distance (B) between cattle populations based on BoLA-DRB3 alleles. All pairwise FST comparisons (except for Chilean with Peruvian Holstein and Argentinian with Paraguayan Holstein) were significant at 0.05 level.
Colombian Simbrah was differentiated from native Philippine, Colombian Normande, Bolivian Yacumeño and Holstein populations to a greater extent than Colombian Simmental. On the contrary, Colombian Simmental was more differentiated from Philippine Native-Brahman, Philippine Brahman, Spanish Morucha, Peruvian Nellore-Brahman and Bolivian Nellore than Colombian Simbrah (Figure 1A: Supplementary Data S5).
Genetic distance DA clustering was similar to that based on pairwise FST among Holstein and the Philippine populations (Figure 1B). The latter group was well-differentiated from Colombian Simmental, Colombian Simbrah, Bolivian Yacumeño and Colombian Normande populations on the basis of DA but not on pairwise FST. Mean genetic distance values for these breeds indicated that common alleles could explain a large amount of their cumulative allele frequency. Thus, the Holstein population group (0.078 mean DA distance) had 28 alleles in common, accounting for 95.2% of their mean cumulative allele frequency. Ten of these common alleles (BoLA-DRB3*001:01, 002:01, 006:01, 009:02, 010:01, 011:01, 012:01, 14:01:01, 015:01 and 027:03) occurred with >5% frequency in at least one of these populations. The Philippine group (0.169 mean DA distance) had 65 common alleles accounting for 93.1% of their mean cumulative allele frequency, of which 8 (BoLA-DRB3*002:01, 003:01, 012:01, 015:01, 022:01, 030:01, 036:01 and 041:01) were alleles with >5% frequency. The group formed by Colombian and Bolivian Yacumeño populations (0.387 mean DA distance) had 49 alleles in common, accounting for 69.1% of their mean cumulative allele frequency. Seventeen (BoLA-DRB3*001:01, 002:01, 005:01, 005:03, 007:01, 008:01, 009:02, 010:01, 012:01, 013:01, 014:01:01, 015:01, 016:01, 018:01, 022:01, 030:01 and 048:02) of these shared alleles had >5% frequency.
Groups identified in the first two principal component (PC) plots were consistent with those identified in FST and DA distance analysis (Figure 2: Supplementary Data S6). The first PC (42.7% variance) differentiated four main groups. The Holstein population group was characterized by high BoLA-DRB3*015:01, 011:01, 001:01, 027:03, 010:01, 012:01, 014:01:01 and 009:02 allele frequency, while, group formed by Normande, Simmental and Yacumeño by intermediate frequency regarding the same alleles; both groups had intermediate BoLA-DRB3*006:01, 017:01, 016:01, 009:01 and 002:01 allele frequencies. Bolivian Nellore and Peruvian Nellore-Brahman along with a group formed by Philippine Native, Philippine Native-Brahman, Colombian Simbrah, Philippine Brahman and Spanish Morucha were at the other extreme of the first PC. These two groups were characterized by high or intermediate BoLA-DRB3*048:02, 030:01, 028:01 and 022:01 allele frequency. Spanish Morucha was remarkably differentiated in the second PC (20.3% variance) due to high BoLA-DRB3*048:02, 003:01 and 005:01 frequency, whereas Nellore cattle had high BoLA-DRB3*028:01, 009:02 and 022:01 frequencies.
PBR Sequence Similarity and Correlation Between Populations
PBR logo representation showed that positions 70, 71, 74, 11, 13, 30, 67, 37, and 57 were highly variable and tended to accumulate non-conservative changes, while positions 82, 83, 14, 15, 40, 72, 73, 79, 29, 64, 47, 9, and 38 were invariable or only displayed conservative changes (Figure 3A). Some highly variable sites were found under positive selection (11, 30, 37, 57, 70, 71, and 74). PBR logos had very similar substitution patterns for all populations. Likewise, Pearson correlation coefficients (PCC) were remarkably high, having high global correlation (PCC = 0.987), thereby indicating low variation for aa frequency for each PBR position among all populations.
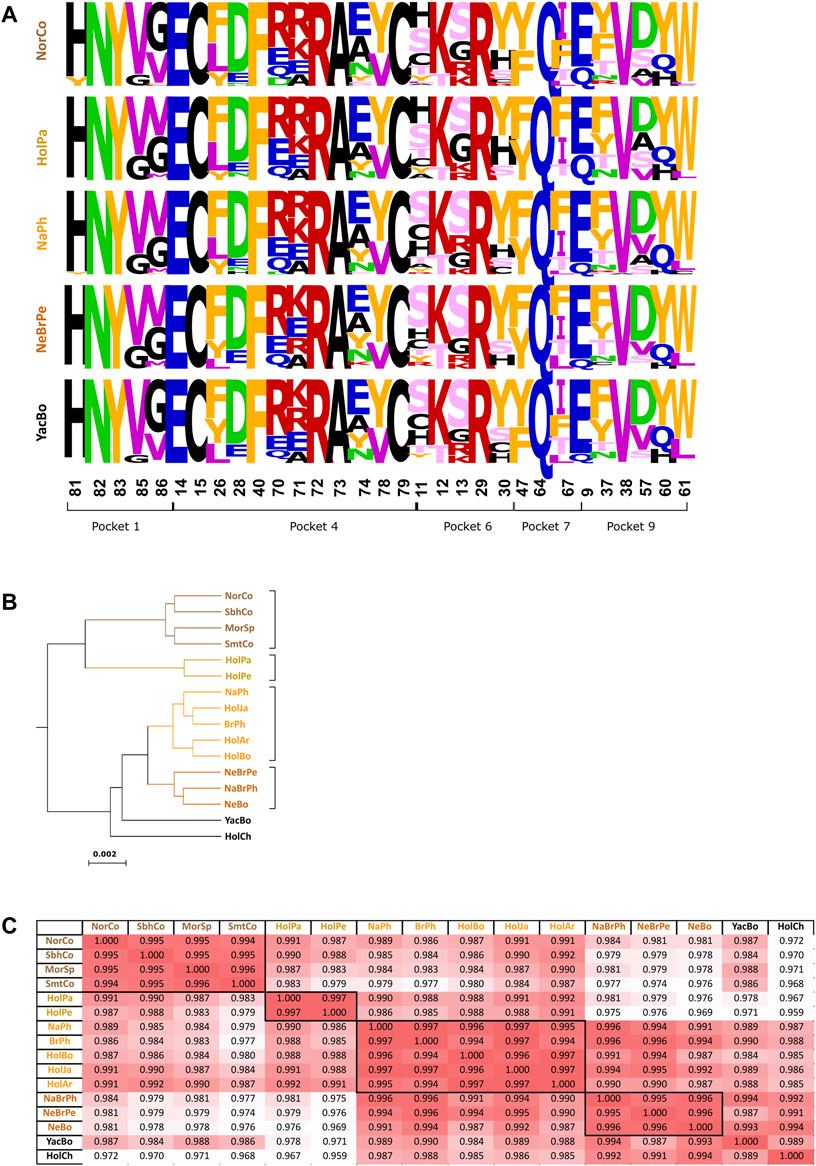
FIGURE 3. Peptide binding region similarity and correlation. (A) BoLA-DRB3 allele peptide binding region (PBR) logos representative of each cattle population group. Conservative aa changes according to the BLOSUM 62 substitution matrix are shown in the same color; different colors indicate non-conservative changes: FYW (yellow), MILV (purple), RK (red), EQ (blue), DN (green), ST (pink), HCPAG (black). PBR Pockets and their aa forming positions are indicated below the logos. (B) Clustering analysis based on correlation analysis. (C) Pearson correlation coefficient matrix for populations based on PBR sequence variability. Population group colors are the same for panels (A,B,C).
PBR correlation analysis identified four major groups (Figures 3B,C). The first one consisted of Colombian Normande, Colombian Simbrah, Spanish Morucha and Colombian Simmental (0.994 mean PCC). It is worth mentioning that the most notable differences between Colombian Simmental and Simbrah occurred regarding aa frequency for positions 70, 30 and 57. Paraguayan Holstein and Peruvian Holstein clustered together (PCC = 0.996). The third group was formed by native Philippine, Philippine Brahman, Bolivian Holstein, Japanese Holstein and Argentinian Holstein (0.996 mean PCC). The fourth group included native Philippine-Brahman, Peruvian Nellore-Brahman and Bolivian Nellore (0.995 mean PCC). Divergence between population groups was mainly due to PBR Pockets 6 (PCC = 0.981), 7 (PCC = 0.979) and 9 (PCC = 0.978), while Pockets 1 (PCC = 0.992) and 4 (PCC = 0.992) were less variable.
Discussion
The MHC influences susceptibility and resistance to infectious diseases, vaccine responses and productions traits. MHC allele distribution information can be used to guide resource-consumption studies (such as immunopeptidomic or binding affinity assays) aimed at identifying MHC-associated peptides and developing in silico binding predictive algorithms that can be used for understanding and predicting immune response patterns (Nielsen et al., 2018; Rappazzo et al., 2020). MHC high polymorphism can also provide insights into populations evolutionary history. Nevertheless, MHC diversity has only been explored in a few cattle breeds to date (Takeshima et al., 2003; Giovambattista et al., 2013; Takeshima et al., 2014; Takeshima et al., 2015; Takeshima et al., 2018). In this study, we have first characterized BoLA-DRB3 genetic diversity in the taurine Simmental breed and in its most common cross with zebuine cattle in tropical regions, the Simbrah in Colombia. Considering the recent origin of Colombian Simmental and Simbrah cattle, a large percentage of highly related animals is expected since small herds are derived from few parents, thus reflecting a potentially reduced genetic diversity, a condition previously found for other pure cattle breeds in the country (Amaya et al., 2020; Bohórquez et al., 2020).
Cattle populations varied considerably in terms of allele richness and Hardy-Weinberg equilibrium. It is worth noting that unequal sample size have no significant impact on genetic diversity estimates such as ho (unbiased parametric value estimator, mainly determined by the sampling method used (Nei and Chesser, 1983; Bohórquez et al., 2020)), he or Rs, also when the pertinent corrections were applied (Nei, 1978; Leberg, 2002). Simmental and Simbrah Rs and he were among the highest values, similar to those of Normande and Philippine populations. However, differences in Rs (or Na) associated with similar he values across populations (as observed for Simmental and Simbrah) indicated a marked allele frequency distribution variation, while ho values were the lowest for Colombian cattle, with FIS indices being thereby the highest for these populations. Population sub-structuring may have reduced ho [by means of the Wahlund effect, i.e., reduced observed heterozygosity in a population caused by subpopulation structure (De Meeûs, 2017)], thus magnifying allele frequency differences compared to those found in other populations.
Natural selection and random genetic drift (notoriously exerting higher effects in populations with smaller effective population size) are factors affecting allele frequency distribution (Nei and Tajima, 1981; Tajima and Nei, 1984; Akashi et al., 2012; Husemann et al., 2016), which in the case of the MHC is expected to reflect balancing selection with heterozygote excess (Hughes and Nei, 1988; Hughes and Yeager, 1998; Takeshima et al., 2008). Even though FIS indicated heterozygote deficiency for BoLA-DRB3, the persistence of identical or similar alleles (allelic lineages) in spite of the overall genetic differentiation points to the action of balancing selection. Moreover, the highly significant dN/dS values and codons identified under positive selection suggest that aa variability preference has not been eroded in these cattle. Although drift and non-random mating are well-known factors leading to increased homozygosity (Crow, 2010) as well as small effective population sizes and inbreeding, the use of only one locus hampered from singling out the underlying cause of such pattern. Likewise, the high FIS values for some of these populations, indicating higher homozygote percentages than those expected for Hardy-Weinberg equilibrium, points to the occurrence of evolutionary forces acting on these populations. Therefore, further studies based on genome-wide data including both neutral and non-neutral loci, are necessary to get a comprehensive picture of the evolutionary forces acting on this system, and of their relative contributions [in Simmental, selection based on production and its small effective population size should be taken into account (Amaya et al., 2020; de Araujo Neto et al., 2020)].
Alleles fell into different categories based on their distribution throughout the populations tested. The first category consisted of alleles widely distributed in populations from different continents and often displaying relatively high frequencies. These alleles, such as BoLA-DRB3*011:01, are possibly present in all populations, or absent in a few of them, such as BoLA-DRB3*010:01, 012:01 and 014:01:01. Considering that taurine and zebuine cattle were domesticated in more than two independent events (Beja-Pereira et al., 2006; Decker et al., 2014), these alleles probably predate Bos primigenius divergence which gave rise to these cattle types. Such alleles might have been either present in just a subset of the founder populations or ubiquitous before undergoing secondary loss due to random genetic drift and/or natural selection (alternatively, their very low frequencies impaired their sampling) (Barton, 1996; Sutton et al., 2011). Another category consisted of alleles, such as BoLA-DRB3*017:01, 006:01, 009:01 and 027:10, found predominantly in taurine or zebuine cattle with their presence in the other type of cattle populations being possibly indicative of admixture. The last category includes the alleles found exclusively in some populations and displaying low frequencies, possibly representing the most recently arisen ones (Uinuk-Ool et al., 2002). Despite forming just a moderate proportion of known BoLA-DRB3 alleles found in and Simbrah cattle (67 and 60 out of 330, respectively) (Maccari et al., 2017), they occur with significant frequency in other cattle populations, representing the major allele variants (Bohórquez et al., 2020) (Supplementary Data S3, Supplementary Data S4 and Supplementary Data S6). Nevertheless, some alleles contributing towards the distinction of Colombian Simmental from Simbrah were also significant in differentiating zebuine from taurine cattle, such as BoLA-DRB3*015:01 and 022:01 frequently occurring in taurine and zebuine cattle, respectively (Takeshima et al., 2018). Alleles private to Simmental or Simbrah further contributed to their differentiation.
Although most of the target bovine populations analyzed here were genetically well-differentiated based on BoLA-DRB3, others had a shallow structure due to the sharing of several alleles occurring with high or intermediate frequency. These populations clustered into five groups according to the measures of differentiation used. Low mean distance values indicated high genetic affinity for these populations and more detailed analysis showed that commonly occurring alleles accounted for a large percentage of their mean cumulative allele frequency. Several factors may result in weak structure. For instance, the limited genetic distance between Colombian Simmental and Normande might be due to sample origin, as geographical dispersal patterns in cattle reflects those of exportation and co-migration in humans (Decker et al., 2014), as well as similar selection pressure (Bohórquez et al., 2020). Furthermore, as zebuine introgression occurred independently in American and Indian cattle (Decker et al., 2014), crossbreeding with Brahman may have led to low genetic differentiation between Simbrah and Philippine populations. These results contrasted with the weak differentiation in Holstein cattle, such breed forming a very compact group in spite of multiple sample origins (Takeshima et al., 2015), possibly as the result of intense selective pressure regarding milk production traits (Bohórquez et al., 2020) and a high level of gene flow via genetic improvement strategies, thereby leading to a high degree of homogenization (Spieth, 1974; Leroy et al., 2013).
MHC PBR positions hosted the highest β1 domain variability associated with the peptides to which an allele could bind (Hughes and Nei, 1989; Stern et al., 1994). The similarity/identity matrix of the MHC-DRB PBR position across cattle populations suggested potential differences in MHC-presented peptide repertoire size (Table 4). Colombian populations had the highest identity and similarity values in both β1 domain and PBR. This could be due to high overall homozygosity but also to a limited variability at these specific loci and suggested that these animals had smaller individual MHC-presented peptide repertoires. Nevertheless, the good correlation regarding PBR aa sequence and logo analysis may suggest that potential population-related MHC-presented peptide repertoire diversity could be equivalent among all cattle populations analyzed here, with just a few groups displaying a much higher variability. This implies that breeding is unlikely to have decreased functional MHC variability, which bears important implications for peptide-based vaccine design, so that different cattle populations could be targeted using similar peptide combinations. Although it has been shown that decreased MHC variability might be caused by population bottlenecks (Bollmer et al., 2011; Zhang et al., 2016), balancing selection driven by pathogens can still maintain a high degree of diversity (Aguilar et al., 2004; Newhouse and Balakrishnan, 2015). Moutou et al., have shown that functional polymorphism may be lower than genetic polymorphism in pigs (Moutou et al., 2013). The highest similarities within and between porcine populations were mainly due to high correlation in PBR Pocket 1 and 4, whereas a higher divergence was observed for Pocket 6, 7 and 9. This could have arisen from Pocket 1, 4 and 6 aa preferences as anchor positions (Rappazzo et al., 2020). This work has shown that, in spite of high values for some genetic diversity measures regarding BoLA-DRB3 (such as allele richness and expected heterozygosity), Colombian Simmental, Simbrah and other cattle populations may have a limited potential MHC-presented peptide repertoire diversity could be similar among all cattle populations analyzed and that breeding did not decrease functional diversity. Additional analyses directly addressing peptide repertoire diversity are needed to confirm these results.
Data Availability Statement
The original contributions presented in the study are included in the article/Supplementary Material, further inquiries can be directed to the corresponding author.
Ethics Statement
The animal study was reviewed and approved by the Universidad de Ciencias Aplicadas y Ambientales’ (U.D.C.A) Animal Research Ethics Committee (minute No. 201901). Written informed consent was obtained from the owners for the participation of their animals in this study.
Author Contributions
DO, MB, CA, and MP: conceptualisation. DO and MB: data curation. MB, DO and CA: formal analysis. MP: funding acquisition. MB, DO and CA: investigation. DO: resources. MP: supervision. MB: visualisation. MB and DO: writing – original draft preparation. MB, DO, CA, and MP: writing—review and editing.
Funding
This study was funded by the Fundación Instituto de Inmunología de Colombia (FIDIC).
Conflict of Interest
The authors declare that the research was conducted in the absence of any commercial or financial relationships that could be construed as a potential conflict of interest.
Publisher’s Note
All claims expressed in this article are solely those of the authors and do not necessarily represent those of their affiliated organizations, or those of the publisher, the editors and the reviewers. Any product that may be evaluated in this article, or claim that may be made by its manufacturer, is not guaranteed or endorsed by the publisher.
Acknowledgments
We would like to thank Doctor Ricardo Buitrago for his support in obtaining Simmental and Simbrah samples and thank Jason Garry for reviewing and editing this manuscript. We would like to thank the reviewers for their kind suggestions to improve this manuscript.
Supplementary Material
The Supplementary Material for this article can be found online at: https://www.frontiersin.org/articles/10.3389/fgene.2022.772885/full#supplementary-material
References
Acosta-Rodríguez, R., Alonso-Morales, R., Balladares, S., Flores-Aguilar, H., García-Vazquez, Z., and Gorodezky, C. (2005). Analysis of BoLA Class II Microsatellites in Cattle Infested with Boophilus Microplus Ticks: Class II Is Probably Associated with Susceptibility. Vet. Parasitol. 127 (3-4), 313–321. doi:10.1016/j.vetpar.2004.10.007
Aguilar, A., Roemer, G., Debenham, S., Binns, M., Garcelon, D., and Wayne, R. K. (2004). High MHC Diversity Maintained by Balancing Selection in an Otherwise Genetically Monomorphic Mammal. Proc. Natl. Acad. Sci. 101 (10), 3490–3494. doi:10.1073/pnas.0306582101
Agung, P. P., Saputra, F., Septian, W. A., Zein, M. S., Sulandari, S., Anwar, S., et al. (2016). Study of Genetic Diversity Among Simmental Cross Cattle in West Sumatra Based on Microsatellite Markers. Asian-Australas J. Anim. Sci. 29 (2), 176–183. doi:10.5713/ajas.15.0155
Akashi, H., Osada, N., and Ohta, T. (2012). Weak Selection and Protein Evolution. Genetics. 192 (1), 15–31. doi:10.1534/genetics.112.140178
Amaya, A., Martínez, R., and Cerón-Muñoz, M. (2020). Population Structure and Genetic Diversity in Colombian Simmental Cattle. Trop. Anim. Health Prod. 52 (3), 1133–1139. doi:10.1007/s11250-019-02111-w
Babik, W., Kawałko, A., Wójcik, J. M., and Radwan, J. (2012). Low Major Histocompatibility Complex Class I (MHC I) Variation in the European Bison (Bison Bonasus). J. Hered. 103 (3), 349–359. doi:10.1093/jhered/ess005
Bacon, L. D., and Witter, R. L. (1995). Efficacy of Marek's Disease Vaccines in Mhc Heterozygous Chickens: Mhc Congenic × Inbred Line F1 Matings. J. Hered. 86 (4), 269–273. doi:10.1093/oxfordjournals.jhered.a111580
Barton, N. H. (1996). Natural Selection and Random Genetic Drift as Causes of Evolution on Islands. Philos. Trans. R. Soc. Lond. B Biol. Sci. 351 (1341), 785–795. doi:10.1098/rstb.1996.0073
Baxter, R., Craigmile, S. C., Haley, C., Douglas, A. J., Williams, J. L., and Glass, E. J. (2009). BoLA-DR Peptide Binding Pockets Are Fundamental for Foot-And-Mouth Disease Virus Vaccine Design in Cattle. Vaccine. 28 (1), 28–37. doi:10.1016/j.vaccine.2009.09.131
Baxter, R., Hastings, N., Law, A., and Glass, E. J. (2008). A Rapid and Robust Sequence-Based Genotyping Method forBoLA-DRB3alleles in Large Numbers of Heterozygous Cattle. Anim. Genet. 39 (5), 561–563. doi:10.1111/j.1365-2052.2008.01757.x
Beja-Pereira, A., Caramelli, D., Lalueza-Fox, C., Vernesi, C., Ferrand, N., Casoli, A., et al. (2006). The Origin of European Cattle: Evidence from Modern and Ancient DNA. Proc. Natl. Acad. Sci. 103 (21), 8113–8118. doi:10.1073/pnas.0509210103
Bohórquez, M. D., Ordoñez, D., Suárez, C. F., Vicente, B., Vieira, C., López-Abán, J., et al. (2020). Major Histocompatibility Complex Class II (DRB3) Genetic Diversity in Spanish Morucha and Colombian Normande Cattle Compared to Taurine and Zebu Populations. Front. Genet. 10 (1293), 1293. doi:10.3389/fgene.2019.01293
Bollmer, J. L., Hull, J. M., Ernest, H. B., Sarasola, J. H., and Parker, P. G. (2011). Reduced MHC and Neutral Variation in the Galápagos Hawk, an Island Endemic. BMC Evol. Biol. 11 (143), 143–2148. doi:10.1186/1471-2148-11-143
Borg, Å. A., Pedersen, S. A., Jensen, H., and Westerdahl, H. (2011). Variation in MHC Genotypes in Two Populations of House Sparrow (Passer domesticus) with Different Population Histories. Ecol. Evol. 1 (2), 145–159. doi:10.1002/ece3.13
Carignano, H. A., Beribe, M. J., Caffaro, M. E., Amadio, A., Nani, J. P., Gutierrez, G., et al. (2017). BOLA-DRB3gene Polymorphisms Influence Bovine Leukaemia Virus Infection Levels in Holstein and Holstein × Jersey Crossbreed Dairy Cattle. Anim. Genet. 48 (4), 420–430. doi:10.1111/age.12566
Crooks, G. E., Hon, G., Chandonia, J.-M., and Brenner, S. E. (2004). WebLogo: A Sequence Logo Generator: Figure 1. Genome Res. 14 (6), 1188–1190. doi:10.1101/gr.849004
Crow, J. F. (2010). Wright and Fisher on Inbreeding and Random Drift. Genetics. 184 (3), 609–611. doi:10.1534/genetics.109.110023
de Araujo Neto, F. R., Vieira, D. A., Santos, D. J. d. A., Pessoa, M. C., Borquis, R. R. A., de Oliveira, H. N., et al. (2020). Population Structure of Simmental Beef Cattle Using Pedigree Analysis. Trop. Anim. Health Prod. 52 (3), 1513–1517. doi:10.1007/s11250-019-02102-x
De Meeûs, T. (2017). Revisiting FIS, FST, Wahlund Effects, and Null Alleles. J. Hered. 109 (4), 446–456. doi:10.1093/jhered/esx106
Decker, J. E., McKay, S. D., Rolf, M. M., Kim, J., Molina Alcalá, A., Sonstegard, T. S., et al. (2014). Worldwide Patterns of Ancestry, Divergence, and Admixture in Domesticated Cattle. Plos Genet. 10 (3), e1004254. doi:10.1371/journal.pgen.1004254
Delport, W., Poon, A. F. Y., Frost, S. D. W., and Kosakovsky Pond, S. L. (2010). Datamonkey 2010: a Suite of Phylogenetic Analysis Tools for Evolutionary Biology. Bioinformatics. 26 (19), 2455–2457. doi:10.1093/bioinformatics/btq429
Dietz, A. B., Detilleux, J. C., Freeman, A. E., Kelley, D. H., Stabel, J. R., and Kehrli, M. E. (1997). Genetic Association of Bovine Lymphocyte Antigen DRB3 Alleles with Immunological Traits of Holstein Cattle. J. Dairy Sci. 80 (2), 400–405. doi:10.3168/jds.s0022-0302(97)75950-2
Edwards, S. V., and Hedrick, P. W. (1998). Evolution and Ecology of MHC Molecules: from Genomics to Sexual Selection. Trends Ecol. Evol. 13 (8), 305–311. doi:10.1016/s0169-5347(98)01416-5
Ellis, S. (2004). The Cattle Major Histocompatibility Complex: Is it Unique? Vet. Immunol. Immunopathol. 102 (1-2), 1–8. doi:10.1016/j.vetimm.2004.06.007
Excoffier, L., and Lischer, H. E. L. (2010). Arlequin Suite Ver 3.5: a New Series of Programs to Perform Population Genetics Analyses Under Linux and Windows. Mol. Ecol. Resour. 10 (3), 564–567. doi:10.1111/j.1755-0998.2010.02847.x
Galaverni, M., Caniglia, R., Fabbri, E., Lapalombella, S., and Randi, E. (2013). MHC Variability in an Isolated Wolf Population in Italy. J. Hered. 104 (5), 601–612. doi:10.1093/jhered/est045
García-Briones, M. M., Russell, G. C., Oliver, R. A., Tami, C., Taboga, O., Carrillo, E., et al. (2000). Association of Bovine DRB3 Alleles with Immune Response to FMDV Peptides and protection against Viral challenge. Vaccine. 19 (9-10), 1167–1171. doi:10.1016/s0264-410x(00)00313-3
Garrigan, D., and Hedrick, P. (2001). Class I MHC Polymorphism and Evolution in Endangered California Chinook and Other Pacific salmon. Immunogenetics. 53 (6), 483–489. doi:10.1007/s002510100352
Giovambattista, G., Takeshima, S.-n., Ripoli, M. V., Matsumoto, Y., Franco, L. A. A., Saito, H., et al. (2013). Characterization of Bovine MHC DRB3 Diversity in Latin American Creole Cattle Breeds. Gene. 519 (1), 150–158. doi:10.1016/j.gene.2013.01.002
Goszczynski, D. E., Corbi-Botto, C. M., Durand, H. M., Rogberg-Muñoz, A., Munilla, S., Peral-Garcia, P., et al. (2018). Evidence of Positive Selection Towards Zebuine Haplotypes in the BoLA Region of Brangus Cattle. Animal. 12 (2), 215–223. doi:10.1017/s1751731117001380
Gowane, G. R., Sharma, A. K., Sankar, M., Narayanan, K., Das, B., Subramaniam, S., et al. (2013). Association of BoLA DRB3 Alleles with Variability in Immune Response Among the Crossbred Cattle Vaccinated for Foot-And-Mouth Disease (FMD). Res. Vet. Sci. 95 (1), 156–163. doi:10.1016/j.rvsc.2013.03.001
Greenbaum, G., Templeton, A. R., Zarmi, Y., and Bar-David, S. (2014). Allelic Richness Following Population Founding Events-Aa Stochastic Modeling Framework Incorporating Gene Flow and Genetic Drift. PLoS ONE. 9, e115203–23. doi:10.1371/journal.pone.0115203
Hammer, Ø., Harper, D., and Ryan, P. D. (2001). PAST: Paleontological Statistics Software Package for Education and Data Analysis. Palaeontol. Electronica. 4, 1–9.
Hughes, A. L., and Yeager, M. (1998). Natural Selection and the Evolutionary History of Major Histocompatibility Complex Loci. Front. Biosci. 3 (3), d509–16. doi:10.2741/a298
Hughes, A. L., and Nei, M. (1988). Pattern of Nucleotide Substitution at Major Histocompatibility Complex Class I Loci Reveals Overdominant Selection. Nature. 335 (6186), 167–170. doi:10.1038/335167a0
Hughes, A. L., and Nei, M. (1989). Nucleotide Substitution at Major Histocompatibility Complex Class II Loci: Evidence for Overdominant Selection. Proc. Natl. Acad. Sci. 86 (3), 958–962. doi:10.1073/pnas.86.3.958
Husemann, M., Zachos, F. E., Paxton, R. J., and Habel, J. C. (2016). Effective Population Size in Ecology and Evolution. Heredity. 117 (4), 191–192. doi:10.1038/hdy.2016.75
Ihaka, R., and Gentleman, R. (1996). R: A Language for Data Analysis and Graphics. J. Comput. Graphical Stat. 5 (3), 299–314. doi:10.1080/10618600.1996.10474713
Juliarena, M. A., Poli, M., Sala, L., Ceriani, C., Gutierrez, S., Dolcini, G., et al. (2008). Association of BLV Infection Profiles with Alleles of theBoLA-DRB3.2gene. Anim. Genet. 39 (4), 432–438. doi:10.1111/j.1365-2052.2008.01750.x
Klein, J., Sato, A., and Nikolaidis, N. (2007). MHC, TSP, and the Origin of Species: from Immunogenetics to Evolutionary Genetics. Annu. Rev. Genet. 41, 281–304. doi:10.1146/annurev.genet.41.110306.130137
Kosakovsky Pond, S. L., and Frost, S. D. W. (2005). Not so Different after All: a Comparison of Methods for Detecting Amino Acid Sites under Selection. Mol. Biol. Evol. 22 (5), 1208–1222. doi:10.1093/molbev/msi105
Kulberg, S., Heringstad, B., Guttersrud, O. A., and Olsaker, I. (2007). Study on the Association of BoLA-DRB3.2 Alleles with Clinical Mastitis in Norwegian Red Cows. J. Anim. Breed. Genet. 124 (4), 201–207. doi:10.1111/j.1439-0388.2007.00662.x
Kumar, S., Stecher, G., Li, M., Knyaz, C., and Tamura, K. (2018). MEGA X: Molecular Evolutionary Genetics Analysis across Computing Platforms. Mol. Biol. Evol. 35 (6), 547–1549. doi:10.1093/molbev/msy096
Leberg, P. L. (2002). Estimating Allelic Richness: Effects of Sample Size and Bottlenecks. Mol. Ecol. 11 (11), 2445–2449. doi:10.1046/j.1365-294x.2002.01612.x
Ledwidge, S. A., Mallard, B. A., Gibson, J. P., Jansen, G. B., and Jiang, Z. H. (2001). Multi-primer Target PCR for Rapid Identification of Bovine DRB3 Alleles. Anim. Genet. 32 (4), 219–221. doi:10.1046/j.1365-2052.2001.00761.x
Lenz, T. L., and Becker, S. (2008). Simple Approach to Reduce PCR Artefact Formation Leads to Reliable Genotyping of MHC and Other Highly Polymorphic Loci-Iimplications for Evolutionary Analysis. Gene. 427 (1-2), 117–123. doi:10.1016/j.gene.2008.09.013
Leroy, G., Mary-Huard, T., Verrier, E., Danvy, S., Charvolin, E., and Danchin-Burge, C. (2013). Methods to Estimate Effective Population Size Using Pedigree Data: Examples in Dog, Sheep, Cattle and Horse. Genet. Sel Evol. 45 (1), 1. doi:10.1186/1297-9686-45-1
Li, C. C. First Course in Population Genetics1976 Pacific Grove. California, USA: Boxwood Press, 631.
Maccari, G., Robinson, J., Ballingall, K., Guethlein, L. A., Grimholt, U., Kaufman, J., et al. (2017). IPD-MHC 2.0: an Improved Inter-species Database for the Study of the Major Histocompatibility Complex. Nucleic Acids Res. 45 (D1), D860–D864. doi:10.1093/nar/gkw1050
Maillard, J. C., Berthier, D., Chantal, I., Thevenon, S., Sidibé, I., Stachurski, F., et al. (2003). Selection Assisted by a BoLA-DR/DQ Haplotype against Susceptibility to Bovine Dermatophilosis. Genet. Sel Evol. 35 (Suppl. 1), S193–S200. doi:10.1186/1297-9686-35-S1-S193
Martinez, M. L., Machado, M. A., Nascimento, C. S., Silva, M. V., Teodoro, R. L., Furlong, J., et al. (2006). Association of BoLA-DRB3.2 Alleles with Tick (Boophilus Microplus) Resistance in Cattle. Genet. Mol. Res. 5 (3), 513–524.
Mason, R. A. B., Browning, T. L., and Eldridge, M. D. B. (2011). Reduced MHC Class II Diversity in Island Compared to mainland Populations of the Black-Footed Rock-Wallaby (Petrogale Lateralis Lateralis). Conserv Genet. 12 (1), 91–103. doi:10.1007/s10592-009-9993-y
Mikko, S., Spencer, M., Morris, B., Stabile, S., Basu, T., Stormont, C., et al. (1997). A Comparative Analysis of Mhc DRB3 Polymorphism in the American Bison (Bison bison). J. Hered. 88 (6), 499–503. doi:10.1093/oxfordjournals.jhered.a023144
Miltiadou, D., Law, A. S., and Russell, G. C. (2003). Establishment of a Sequence-Based Typing System for BoLA-DRB3 Exon 2. Tissue Antigens. 62 (1), 55–65. doi:10.1034/j.1399-0039.2003.00080.x
Moutou, K. A., Koutsogiannouli, E. A., Stamatis, C., Billinis, C., Kalbe, C., Scandura, M., et al. (2013). Domestication Does Not Narrow MHC Diversity in Sus scrofa. Immunogenetics. 65 (3), 195–209. doi:10.1007/s00251-012-0671-8
Murrell, B., Wertheim, J. O., Moola, S., Weighill, T., Scheffler, K., and Kosakovsky Pond, S. L. (2012). Detecting Individual Sites Subject to Episodic Diversifying Selection. Plos Genet. 8 (7), e1002764. doi:10.1371/journal.pgen.1002764
Nascimento, C. S. d., Machado, M. A., Martinez, M. L., Silva, M. V. G. B. d., Guimarães, M. F. M., Campos, A. L., et al. (2006). Association of the Bovine Major Histocompatibility Complex (BoLA) BoLA-DRB3 Gene with Fat and Protein Production and Somatic Cell Score in Brazilian Gyr Dairy Cattle (Bos indicus). Genet. Mol. Biol. 29, 641–647. doi:10.1590/s1415-47572006000400011
Neefjes, J., Jongsma, M. L. M., Paul, P., and Bakke, O. (2011). Towards a Systems Understanding of MHC Class I and MHC Class II Antigen Presentation. Nat. Rev. Immunol. 11 (12), 823–836. doi:10.1038/nri3084
Nei, M., and Chesser, R. K. (1983). Estimation of Fixation Indices and Gene Diversities. Ann. Hum. Genet. 47 (3), 253–259. doi:10.1111/j.1469-1809.1983.tb00993.x
Nei, M. (1978). Estimation of Average Heterozygosity and Genetic Distance from a Small Number of Individuals. Genetics. 89 (3), 583–590. doi:10.1093/genetics/89.3.583
Nei, M., and Tajima, F. (1981). Genetic Drift and Estimation of Effective Population Size. Genetics. 98 (3), 625–640. doi:10.1093/genetics/98.3.625
Newhouse, D. J., and Balakrishnan, C. N. (2015). High Major Histocompatibility Complex Class I Polymorphism Despite Bottlenecks in Wild and Domesticated Populations of the Zebra Finch (Taeniopygia guttata). BMC Evol. Biol. 15 (265), 265–0546. doi:10.1186/s12862-015-0546-3
Nicholas, K. B. (1997). GeneDoc : Analysis and Visualization of Genetic Variation. Embnew. News. 4, 14.
Nielsen, M., Lundegaard, C., Blicher, T., Peters, B., Sette, A., Justesen, S., et al. (2008). Quantitative Predictions of Peptide Binding to Any HLA-DR Molecule of Known Sequence: NetMHCIIpan. Plos Comput. Biol. 4 (7), e1000107. doi:10.1371/journal.pcbi.1000107
Nielsen, M., Connelley, T., and Ternette, N. (2018). Improved Prediction of Bovine Leucocyte Antigens (BoLA) Presented Ligands by Use of Mass-Spectrometry-Determined Ligand and In Vitro Binding Data. J. Proteome Res. 17 (1), 559–567. doi:10.1021/acs.jproteome.7b00675
Nikbakht Brujeni, G., Ghorbanpour, R., Esmailnejad, A., and Association of BoLA-Drb3, (2016). Association of BoLA-DRB3.2 Alleles with BLV Infection Profiles (Persistent Lymphocytosis/Lymphosarcoma) and Lymphocyte Subsets in Iranian Holstein Cattle. Biochem. Genet. 54 (2), 194–207. doi:10.1007/s10528-016-9712-6
Paradis, E., Claude, J., and Strimmer, K. (2004). APE: Analyses of Phylogenetics and Evolution in R Language. Bioinformatics. 20 (2), 289–290. doi:10.1093/bioinformatics/btg412
Rappazzo, C. G., Huisman, B. D., and Birnbaum, M. E. (2020). Repertoire-scale Determination of Class II MHC Peptide Binding via Yeast Display Improves Antigen Prediction. Nat. Commun. 11 (1), 4414. doi:10.1038/s41467-020-18204-2
Rock, K. L., Reits, E., and Neefjes, J. (2016). Present Yourself! by MHC Class I and MHC Class II Molecules. Trends Immunol. 37 (11), 724–737. doi:10.1016/j.it.2016.08.010
Rousset, F. (2008). genepop'007: a Complete Re-implementation of the Genepop Software for Windows and Linux. Mol. Ecol. Resour. 8 (1), 103–106. doi:10.1111/j.1471-8286.2007.01931.x
Rupp, R., Hernandez, A., and Mallard, B. A. (2007). Association of Bovine Leukocyte Antigen (BoLA) DRB3.2 with Immune Response, Mastitis, and Production and Type Traits in Canadian Holsteins. J. Dairy Sci. 90 (2), 1029–1038. doi:10.3168/jds.s0022-0302(07)71589-8
Sharif, S., Mallard, B. A., Wilkie, B. N., Sargeant, J. M., Scott, H. M., Dekkers, J. C. M., et al. (1999). Associations of the Bovine Major Histocompatibility Complex DRB3 (BoLA-DRB3 ) with Production Traits in Canadian Dairy Cattle. Anim. Genet. 30 (2), 157–160. doi:10.1046/j.1365-2052.1999.00459.x
Sigurdardóttir, S., Borsch, C., Gustafsson, K., and Andersson, L. (1991). Cloning and Sequence Analysis of 14 DRB Alleles of the Bovine Major Histocompatibility Complex by Using the Polymerase Chain Reaction. Anim. Genet. 22 (3), 199–209. doi:10.1111/j.1365-2052.1991.tb00670.x
Spieth, P. T. (1974). Gene Flow and Genetic Differentiation. Genetics. 78 (3), 961–965. doi:10.1093/genetics/78.3.961
Stern, L. J., Brown, J. H., Jardetzky, T. S., Gorga, J. C., Urban, R. G., Strominger, J. L., et al. (1994). Crystal Structure of the Human Class II MHC Protein HLA-DR1 Complexed with an Influenza Virus Peptide. Nature. 368 (6468), 215–221. doi:10.1038/368215a0
Suárez, C. F., Patarroyo, M. E., Trujillo, E., Estupiñán, M., Baquero, J. E., Parra, C., et al. (2006). Owl Monkey MHC-DRB Exon 2 Reveals High Similarity with Several HLA-DRB Lineages. Immunogenetics. 58 (7), 542–558. doi:10.1007/s00251-006-0127-0
Sutton, J. T., Nakagawa, S., Robertson, B. C., and Jamieson, I. G. (2011). Disentangling the Roles of Natural Selection and Genetic Drift in Shaping Variation at MHC Immunity Genes. Mol. Ecol. 20 (21), 4408–4420. doi:10.1111/j.1365-294x.2011.05292.x
Tajima, F., and Nei, M. (1984). Note on Genetic Drift and Estimation of Effective Population Size. Genetics. 106 (3), 569–574. doi:10.1093/genetics/106.3.569
Takeshima, S.-N., Giovambattista, G., Okimoto, N., Matsumoto, Y., Rogberg-Muñoz, A., Acosta, T. J., et al. (2015). Characterization of Bovine MHC Class II DRB3 Diversity in South American Holstein Cattle Populations. Tissue Antigens. 86 (6), 419–430. doi:10.1111/tan.12692
Takeshima, S., Matsumoto, Y., Chen, J., Yoshida, T., Mukoyama, H., and Aida, Y. (2008). Evidence for Cattle Major Histocompatibility Complex (BoLA) Class IIDQA1gene Heterozygote Advantage against Clinical Mastitis Caused byStreptococciandEscherichiaspecies. Tissue Antigens. 72 (6), 525–531. doi:10.1111/j.1399-0039.2008.01140.x
Takeshima, S. N., Corbi-Botto, C., Giovambattista, G., and Aida, Y. (2018). Genetic Diversity of BoLA-DRB3 in South American Zebu Cattle Populations. BMC Genet. 19 (1), 33–0618. doi:10.1186/s12863-018-0618-7
Takeshima, S. N., Miyasaka, T., Polat, M., Kikuya, M., Matsumoto, Y., Mingala, C. N., et al. (2014). The Great Diversity of Major Histocompatibility Complex Class II Genes in Philippine Native Cattle. Meta Gene 2, 176–190. doi:10.1016/j.mgene.2013.12.005
Takeshima, S., Saitou, N., Morita, M., Inoko, H., and Aida, Y. (2003). The Diversity of Bovine MHC Class II DRB3 Genes in Japanese Black, Japanese Shorthorn, Jersey and Holstein Cattle in Japan. Gene. 316, 111–118. doi:10.1016/s0378-1119(03)00744-3
Takezaki, N., Nei, M., and Tamura, K. (2010). POPTREE2: Software for Constructing Population Trees from Allele Frequency Data and Computing Other Population Statistics with Windows Interface. Mol. Biol. Evol. 27 (4), 747–752. doi:10.1093/molbev/msp312
Taylor, S. S., Jenkins, D. A., and Arcese, P. (2012). Loss of Mhc and Neutral Variation in Peary Caribou: Genetic Drift Is Not Mitigated by Balancing Selection or Exacerbated by Mhc Allele Distributions. Plos One. 7 (5), e36748. doi:10.1371/journal.pone.0036748
Trtkova, K., Mayer, W. E., O’hUign, C., and Klein, J. (1995). Mhc-DRB Genes and the Origin of New World Monkeys. Mol. Phylogenet. Evol. 4 (4), 408–419. doi:10.1006/mpev.1995.1038
Uinuk-Ool, T. S., Takezaki, N., Sukernik, R. I., Nagl, S., and Klein, J. (2002). Origin and Affinities of Indigenous Siberian Populations as Revealed by HLA Class II Gene Frequencies. Hum. Genet. 110 (3), 209–226. doi:10.1007/s00439-001-0668-0
Ujvari, B., and Belov, K. (2011). Major Histocompatibility Complex (MHC) Markers in Conservation Biology. Int. J. Mol. Sci. 12 (8), 5168–5186. doi:10.3390/ijms12085168
Weir, B. S., and Cockerham, C. C. (1984). Estimating F -Statistics for the Analysis of Population Structure. Evolution. 38 (6), 1358–1370. doi:10.1111/j.1558-5646.1984.tb05657.x
Keywords: MHC, BoLA-DRB3, genetic diversity, peptide-binding region, genetic resistance, cattle
Citation: Ordoñez D, Bohórquez MD, Avendaño C and Patarroyo MA (2022) Comparing Class II MHC DRB3 Diversity in Colombian Simmental and Simbrah Cattle Across Worldwide Bovine Populations. Front. Genet. 13:772885. doi: 10.3389/fgene.2022.772885
Received: 08 September 2021; Accepted: 17 January 2022;
Published: 04 February 2022.
Edited by:
Thales Renato Ochotorena De Freitas, Federal University of Rio Grande do Sul, BrazilReviewed by:
Giovanni Forcina, Centro de Investigacao em Biodiversidade e Recursos Geneticos (CIBIO-InBIO), PortugalAna Cutrera, CONICET Mar del Plata, Argentina
Copyright © 2022 Ordoñez, Bohórquez, Avendaño and Patarroyo. This is an open-access article distributed under the terms of the Creative Commons Attribution License (CC BY). The use, distribution or reproduction in other forums is permitted, provided the original author(s) and the copyright owner(s) are credited and that the original publication in this journal is cited, in accordance with accepted academic practice. No use, distribution or reproduction is permitted which does not comply with these terms.
*Correspondence: Manuel Alfonso Patarroyo, bWFwYXRhcnIuZmlkaWNAZ21haWwuY29t
†Both authors contributed equally to this study.