- 1USDA-ARS Bee Research Laboratory, Beltsville, MD, United States
- 2Agricultural University of Athens, Athens, Greece
- 3Department of Entomology, University of Maryland, College Park, MD, United States
The genetic diversity of the USA honey bee (Apis mellifera L.) populations was examined through a molecular approach using two mitochondrial DNA (mtDNA) markers. A total of 1,063 samples were analyzed for the mtDNA intergenic region located between the cytochrome c oxidase I and II (COI-COII) and 401 samples were investigated for the NADH dehydrogenase 2 (ND2) coding gene. The samples represented 45 states, the District of Colombia and two territories of the USA. Nationwide, three maternal evolutionary lineages were identified: the North Mediterranean lineage C (93.79%), the West Mediterranean lineage M (3.2%) and the African lineage A (3.01%). A total of 27 haplotypes were identified, 13 of them (95.11%) were already reported and 14 others (4.87%) were found to be novel haplotypes exclusive to the USA. The number of haplotypes per state/territory ranged between two and eight and the haplotype diversity H ranged between 0.236–0.763, with a nationwide haplotype diversity of 0.597. Furthermore, the honey bee populations of the USA were shown to rely heavily (76.64%) on two single haplotypes (C1 = 38.76%, C2j = 37.62%) of the same lineage characterizing A. m. ligustica and A. m. carnica subspecies, respectively. Molecular-variance parsimony in COI-COII and ND2 confirmed this finding and underlined the central and ancestral position of C2d within the C lineage. Moreover, major haplotypes of A. m. mellifera (M3a, M7b, M7c) were recorded in six states (AL, AR, HI, MO, NM and WA). Four classic African haplotypes (A1e, A1v, A4, A4p) were also identified in nine states and Puerto Rico, with higher frequencies in southern states like LA, FL and TX. This data suggests the need to evaluate if a restricted mtDNA haplotype diversity in the US honey bee populations could have negative impacts on the beekeeping sustainability of this country.
1 Introduction
The western honey bee species, A. mellifera L. is not native to the United States of America. It was first imported in the early 1600s and importations have continued since (Carpenter and Harpur, 2021; Marcelino et al., 2022). However, many indigenous non-Apis bee species existed in the country long before the importation of the domestic honey bee to this part of the world. From an ecological and agricultural perspective, all bee species, including non-Apis species, solitary bees and honey bees, play a crucial role in pollinating agricultural food crops and wild flowering plants. Nonetheless, the honey bee remains at the forefront of public interest due to its quantifiable economic benefits. Moreover, the flexibility and efficiency when dispatching honey bee colonies to provide pollination services, especially in the USA, are remarkable and unmatched by any other bee species. The direct economic value of the pollination service provided by honey bees is roughly estimated at 17 billion dollars in the USA (Calderone 2012), and some crops and industries, such as almond production, rely entirely on honey bees for pollination. As a non-native species, the honey bee genetic stock in the USA is limited by the import of genetics from the Old World. Healthy genetic diversity within honey bee populations is critical to provide tolerance and resistance to current and future diseases and predators. Honey bees have suffered high losses over the last decade (Kulhanek et al., 2017), emphasizing the need to understand what drives these losses. A lack of genetic diversity has been suggested as one such cause (Panziera et al., 2022). Considering the crucial role honey bees play in agriculture, as many crops depend on them for pollination, it is important to have a current and realistic estimation of the genetic diversity among the honey bee populations of the USA.
Honey bee diversity across the world was initially assessed based on morphometric characteristics like tergite pigmentation, body and wing sizes, pilosity, tomentum width, cubital index and length of the proboscis (Ruttner et al., 1978; Ruttner 1988; Ftayeh et al., 1994; Kauhausen-Keller et al., 1997; Kandemir et al., 2000; Arias et al., 2006; Meixner et al., 2007; Alburaki and Alburaki, 2008). In line with these attributes, four honey bee evolutionary lineages were described; West Mediterranean lineage M), North Mediterranean lineage C), African lineage A) and Oriental lineage O) (Ruttner et al., 1978; Rinderer, 1986; Ruttner, 1988). Despite some discrepancies among recent studies, all four lineages were molecularly confirmed using mitochondrial and microsatellite markers (Smith and Brown, 1988; Smith and Brown, 1990; Cornuet et al., 1991; Smith et al., 1991; Garnery et al., 1992; Garnery et al., 1993; Moritz et al., 1994; Estoup et al., 1995; Garnery et al., 1998a; Garnery et al., 1998b; Meixner et al., 2000; Bouga et al., 2005). Currently, 31 honey bee subspecies have been identified in their natural areas of repartition in Europe, the Middle East, Western Asia and the African continent (Ruttner 1988; Sheppard and Meixner, 2003; Chen et al., 2016).
Mitochondrial DNA (mtDNA) is a widely used tool in phylogeography and genetic studies due to its absence or low recombination, relatively high evolutionary rate, conserved structure and uniparental inheritance (Avise et al., 1987; Harrison 1989; Avise, 2000). This study used mtDNA to examine the haplotype diversity of Apis mellifera L. (the honey bee) in the USA. The DraI mtDNA COI-COII (DmCC) test (Smith and Brown, 1990; Cornuet et al., 1991; Garnery et al., 1993) stands as one of the most comprehensive mitochondrial markers and is widely used in honey bee genetics (Hall and Smith, 1991; Arias and Sheppard, 1996; De la Rua et al., 1998; Franck et al., 2000; Palmer et al., 2000; Franck et al., 2001; Abrahamovich et al., 2007; El-Niweiri and Moritz, 2008; Alburaki et al., 2011; Rortais et al., 2011; Loucif-Ayad et al., 2014). The DmCC test consists of conducting a CAPS assay (cleaved amplified polymorphic sequence), also known as PCR-RFLP (restriction fragment length polymorphism), of the mtDNA region, which is located between the cytochrome c oxidase subunits I and II. The COI-COII locus is a non-coding region where the restriction patterns reflect the genetic evolution and diversity of A. mellifera. In its traditional form, the DmCC test did not require sequencing of the intergenic region as the evolutionary lineages were assigned based on the molecular weight of the amplicons and the haplotypes based on the patterns of the restricted fragments (Garnery et al., 1993). Numerous studies have employed this marker worldwide and hundreds of haplotypes were identified based on the rules mentioned above. Nonetheless, significant discrepancies concerning haplotype naming and identity have arisen among these investigations, further complicating honey bee phylogeographic analyses. In order to reconcile such discrepancies, an in silico DmCC test was proposed and tested in a recent study (Madella et al., 2021).
In this study, we conducted a comprehensive genetic assessment of the mtDNA haplotype diversity present in the honey bee populations of the USA. The most successful and widely circulating haplotypes among beekeepers and novel haplotypes of different lineages unique to the honey bee populations of the USA were identified.
2 Materials and methods
2.1 Honey bee sampling
This study was conducted using samples from the 2019 APHIS National Honey Bee Disease Survey (NHBDS) and three populations (AZ, MS, MD) sampled in 2020 by the USDA-ARS Bee Research Lab in Beltsville. Samples were collected from migratory and stationary apiaries operated by commercial, sideliner and hobbyist beekeepers. One bee was analyzed from each sample. NHBDS samples consisted of ∼150 bees (1/4 cup) from each of 8 colonies in an apiary. Non-NHBDS samples were collected by sampling a single bee per colony from multiple apiaries per state. Both surveys sampled apiaries belonging to different beekeepers and contained eight or more colonies.
2.2 DNA extraction
A single leg was dissected from each bee sample and the DNA was extracted in 96-well PCR plates using the Chelex method (Walsh et al., 2013) with slight modifications (Madella et al., 2021). Each leg was individually placed in a plate well and 100 μL of warm (60°C) and constantly stirred 10% Chelex solution (w/v) was added to each plate well. The Chelex solution consisted of 1 g Chelex powder and 10 mL molecular grade water. Prior to sealing the plates, 6 μL of Proteinase K (10 mg/mL) was added to each well. The plates were sealed and placed on a CFX1000 Touch Bio-Rad Thermocycler with the following cycling parameters: 55°C for 1h, 99°C for 15min, 37°C for 1 min, 99°C for 15 min and a final hold at 4°C. DNA extracts were stored at −20°C for further downstream analyses.
2.3 Amplification of the mtDNA COI-COII region
2.3.1 DmCC test in silico
The intergenic non-coding region of the mtDNA located between the cytochrome oxidase I and II genes was amplified using a new set of validated primers (Madella et al., 2021) and captured the full sequence length of the COI-COII region: COI_Seq-F: ACCACCTCTAGATCATTCACATTT, COII_Seq-R: AGGATGGAACTGTTCATGAATGAA. This PCR amplification was conducted in a 14 μL reaction mixture that included 6 μL of Bio-Rad’s Master Mix for PCR (2X), 0.5 μL of each primer (10 μM), 5 μL nuclease-free water and 2 μL of DNA. PCR cycling, conducted on a Bio-Rad C1000 Touch Thermal Cycler (CFX96 Real-Time System), was as follows: 92°C for 3 min, followed by 35 cycles of (92°C for 30 sec, 47°C for 90 sec, 63°C for 2 min), 63°C for 10 min and a final hold at 4°C. Electrophoresis on 1% agarose gel was occasionally conducted on a random set of samples to verify proper amplification of the region prior to Sanger sequencing of the PCR products.
2.3.2 Traditional DmCC test (PCR-RFLP)
The previously and widely used set of primers to traditionally carry out the mtDNA DraI COI-COII test on gels (Garnery et al., 1993; Meixner et al., 2000; Franck et al., 2001; Alburaki et al., 2011; Rortais et al., 2011; Achou M et al., 2015) was used in our study for validation purpose: E2-F: 5′-GGCAGAATAAGTGCATTG-3′, H2-R: 5′-CAATATCATTGATGACC-3’. The PCR reaction for this set of primers was carried out in a 26 μL reaction composed of 12.5 μL of Bio-rad’s 2X Master Mix for PCR, 1 μL of each primer (10 μM), 9.5 μL nuclease-free water and 2 μL of DNA. The PCR cycling was similar to the in silico test as described above.
2.4 Amplification of ND2 gene
The coding gene NADH dehydrogenase 2 (ND2) was amplified on a representative set of samples (n = 401) using the same DNA extracts of the DmCC test. This gene was amplified using the primers described in (Arias and Sheppard, 1996): ND2-F: TGATAAAAGAAATATTTTGA, ND2-R: GAATCTAATTAATAAAAAA. The PCR reaction was carried out as described for the in silico DmCC test with the following cycling parameters: 92°C for 3 min, followed by 30 cycles of (92°C for 30 sec, 47°C for 90 sec, 63°C for 2 min), 63°C for 10 min and a final hold at 4°C. Samples were stored at −20°C for further analyses.
2.5 COI-COII & ND2 sequencing
The PCR products for both COI-COII (n = 1,063) and ND2 (n = 401), which each contained a single amplified band, were purified via PCR clean-up procedure and Sanger sequenced from one end (5′end) by Azenta Life Sciences (South Plainfield, NJ, USA). Sequences of novel haplotypes and those exceeding (∼600 bp) were confirmed with a second sequencing conducted from both ends (5′, 3′).
2.6 Constitution of COI-COII databases and blasting
In order to achieve 100% accuracy in haplotype identification with available literature and previously published sequences, the intergenic region’s complete sequence must be captured, including the last 62 bp of the tRNA-Leu (3′end) and the first 317 bp of the cytochrome oxidase II (5′end). Unfortunately, a significant number of available COI-COII sequences on NCBI do not meet this criterion and come short at both 5′ and 3′ ends of this region. Our previous study discussed this challenge (Madella et al., 2021). Therefore, among all available COI-COII sequences found on NCBI, a local database exclusively made of verified sequences with complete length was created. This database consisted of 53 worldwide PopSets with 934 full and verified sequences available in the Supplementary Materials. The COI-COII sequences generated in this study were blasted against this database using Geneious Prime software (Megablast Program) with a scoring value of 1 to 2, a linear gap cost and a maximum E-value of 0.05.
2.7 Evolutionary lineage & haplotype identification
The honey bee evolutionary lineages were determined based on the genetic structure of the intergenic region detailed in previous studies (Cornuet et al., 1991; Smith et al., 1991; Garnery et al., 1992; Garnery et al., 1993; Alburaki et al., 2011). In order to identify the evolutionary lineages and haplotypes, the DmCC test was conducted in silico on all the COI-COII sequences (n = 1,062) following the steps described in (Madella et al., 2021) using Geneious Prime software. Briefly, the sequences were grouped based on the structure and length of the intergenic region, aligned and trimmed at the exact 5′ and 3’ ends of the E2 and H2 primers, annotated and subjected to in silico DraI restriction. The DraI restriction fragments were subsequently revealed on virtual gel with the Lambda DNA/EcoR1 Molecular Marker (ThermoFisher, NY, USA) and other restriction profiles of previously identified haplotypes from prior studies. In order to validate the in silico restriction fragment profiles and analyses, the traditional DmCC test was conducted on a representative set of samples from all lineages, including novel haplotypes, which were identified by the in silico test. The traditional DmCC test, as described in previous studies (Garnery et al., 1993; Alburaki et al., 2011), is performed via a PCR-RFLP on DNA extracts followed by visual identification and comparison of the restricted fragments on polyacrylamide gels.
2.8 Naming of novel haplotypes
Genetic studies on honey bees have generated significant datasets, including many partial sequences and unnamed sequences, sequences with multiple names, and distinct sequences assigned the same name. To improve clarity, newly generated data using the DmCC test requires a systematic approach to naming new sequences, offering uniqueness in the haplotypes’ identities and reconciliation between both traditional (PCR-RFLP) and sequencing methods used for haplotype identification. Since the in silico DmCC test (Madella et al., 2021) can capture the full length of the tRNALeu–COII region, identify variations within the DraI restriction fragments (virtual gels) and any SNP occurring along this region, we used the following universal rule for naming novel haplotypes: (XD
2.9 Data analysis
Sequence alignment, generation of FASTA files and phylogenetic trees were conducted using Geneious Prime 2022.0.1 (https://www.geneious.com). Geographical mapping of populations was conducted using Tableau Public platform (https://www.tableau.com). Generation of haplotype networks and computation of genetic relationships (haplotype and nucleotide diversity, Tajima’s statistic) were conducted in the R environment (R Core Team, 2022) (v. 2022.07.0) using two main Libraries: “ape” and “pegas” (Paradis, 2010; Paradis and Schliep, 2019). Heatmaps were also generated with R using “pheatmap” and “gplots” Libraries. Haplotype diversity (H) is the probability that two randomly sampled alleles are different, while nucleotide diversity (π) represents the average number of nucleotide differences per site in pairwise comparisons among DNA sequences (Nei, 1987). In order to compare our data with previous literature, H was calculated in two ways. 1) Based on endonuclease fragment variations (haplotypes with no DraI restriction pattern differences were considered identical) as proposed in (Nei and Tajima, 1981):
Genetic relationships between haplotypes were computed in R using the molecular-variance parsimony technique. Haplotype networks were estimated under haplotype pairwise differences, which generates the number of mutation steps between haplotypes. ND2 sequences were first aligned using a Cost Matrix of 65% similarity (5.0/4.0) with a “Global alignment with free end gaps” option, then trimmed at both ends to eliminate non-identified nucleotides. The non-coding COI-COII sequences however, were first sorted out according to the genetic structure and length of the intergenic region (Q, P, P0, PQx, P0Qx), where x is a tandem repeat of the Q fragment and analyzed following the steps described in a previous study (Madella et al., 2021). All sequences generated by this study were submitted to NCBI and are publicly available.
Neighbor-joining trees were conducted following the genetic model distance of Tamura-Nei (Tamura and Nei, 1993) with no outgroups for 978 sequences of the C lineage (Q fragment) and 369 corresponding sequences of the ND2 gene. Within the C lineage, COI-COII sequences were run with six reference samples from NCBI (MW677216, HQ287900, JQ977702, MH939332, JQ977700, MH939345), which represent each of the C1, C3, C2e, C2d, C2c, C2j haplotypes respectively. The ND2 sequences of the C lineage were analyzed with a set of available reference samples (n = 22). Inter-lineage and intra-C haplotype phylogenetic analyses were conducted on a representative set of ND2 sequences with Apis cerana as an outgroup as well as reference sequences of A. m. mellifera, A. m. macedonica, A. m. ligustica, A. m. carnica, A. m. sicula, A. m. cecropia, A. m. pomonella, A. m. caucasica, A. m. anatoliaca, A. m. meda, A. m. syricaca, A. m. lamarckii, A. m. intermissa, A. m. sahariensis, A. m. capensis and A. m. scutellata subspecies. These honey bee subspecies represent the four evolutionary lineages known so far (A, M, C, O).
3 Results
3.1 Sample distribution
In this study, 1,063 samples were analyzed from 45 States of the USA, the District of Columbia (D.C.) and two US territories (Guam and Puerto Rico), Figure 1 and Table 1. In most cases, each sample represents the honey bee operation of a single beekeeper. Honey bee samples from New Hampshire, Rhode Island, Wyoming, Ohio and North Carolina were unavailable. The average number of samples analyzed per state was 22 samples except in relation to Alaska (6), Tennessee (13) and Puerto Rico (8), Figure 1.
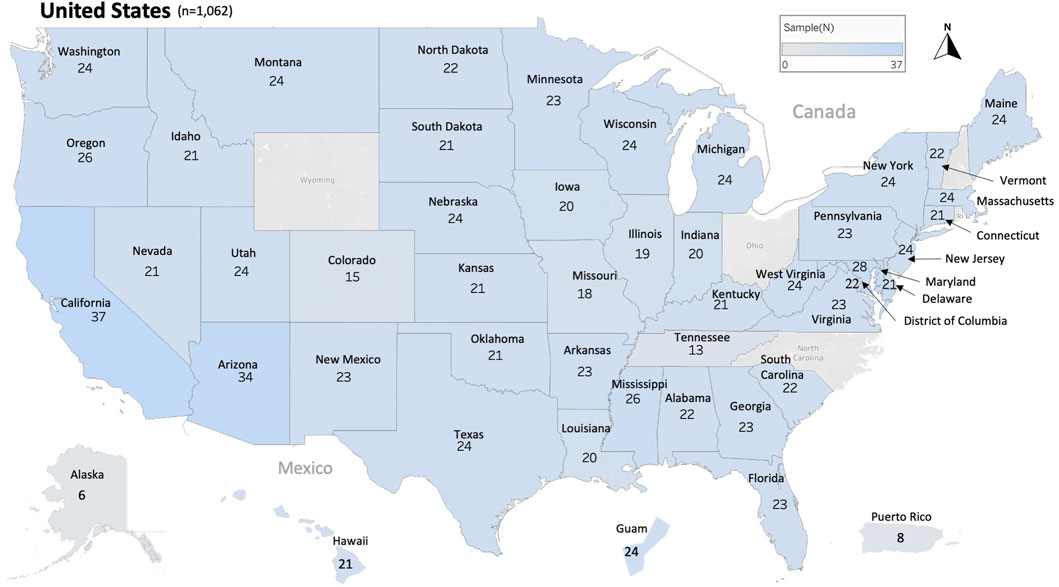
FIGURE 1. Distribution and number of honey bee samples originated from 45 states, the District of Columbia and two territories of the United States of America USA. Total number of samples nationwide is (n = 1,062). Each sample represents a distinct apiary composed of 8 colonies or more.
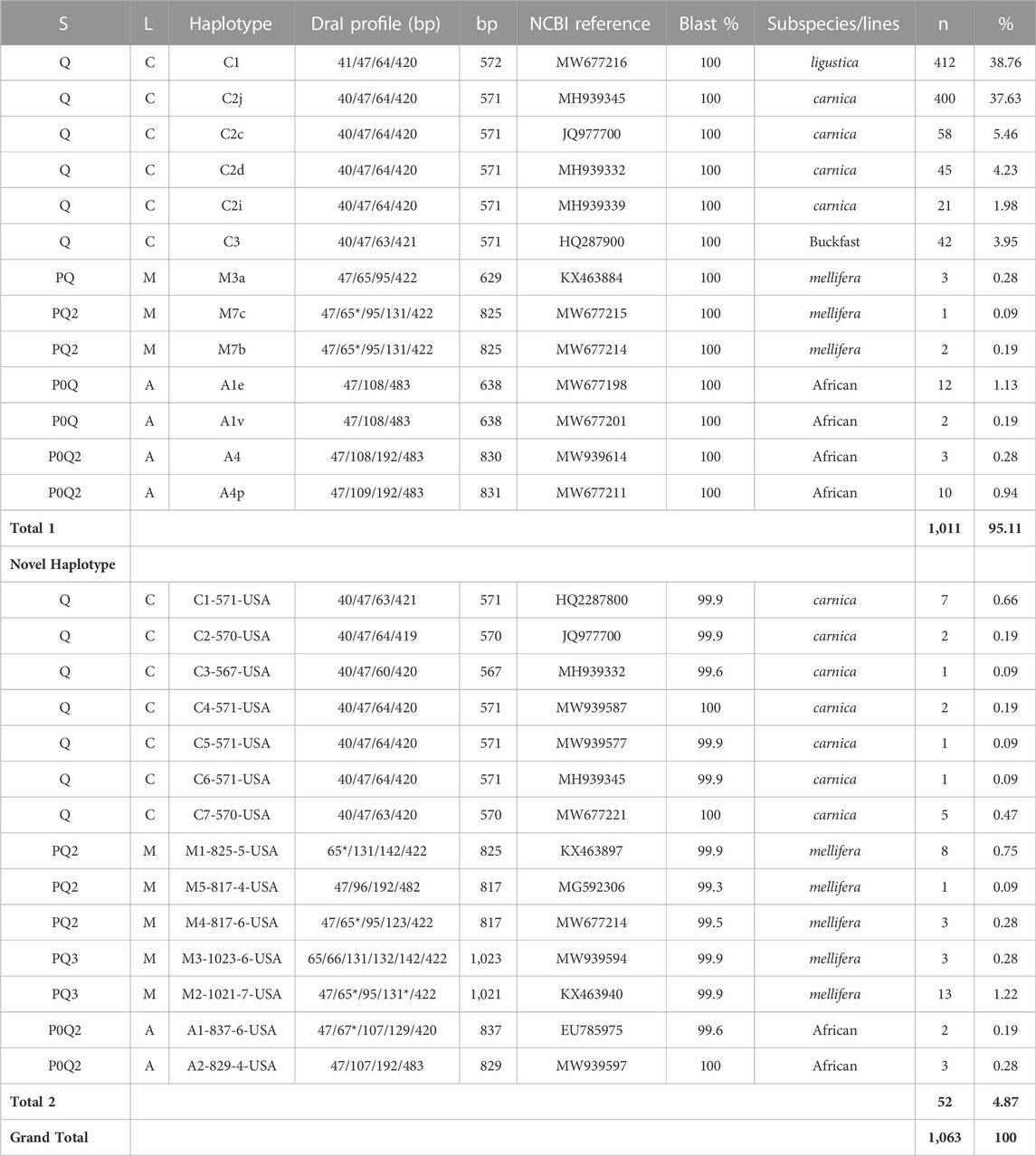
TABLE 1. COI-COII genetic structure (S), evolutionary lineage (L), sequence length (bp), number of samples (n) and overall percentage of each haplotype nationwide (%). (*) indicates a double band of the same size in the DraI profile. Total one is for samples of previously identified haplotypes and Total two is for novel haplotypes identified in the USA populations.
3.2 COI-COII and ND2 sequences
A total of 1,063 sequences for the intergenic COI-COII region and 401 sequences for the ND2 coding gene were obtained, Table 1. All sequences were deposited at the National Center for Biotechnology Information (NCBI) GeneBank with the following accession numbers: 1) ND2 sequences (OM219207-OM219607), (OM219608-OM219613), (OM219614-OM219625), 2) COI-COII sequences (OM219608 - OM219613), (OM219614 - OM219625).
3.3 Honey bee evolutionary lineage
The genetic structure of the mtDNA COI-COII intergenic region of the specified US honey bee populations showed six different polymorphisms: Q, PQ, P0Q, PQ2, PQ3, P0Q2, Table 1 and Figure 2. Furthermore, three evolutionary lineages were identified: 1) North Mediterranean lineage C, 2) West Mediterranean lineage M and 3) the African lineage A, with the C lineage dominating, Table 1 and Figure 3. In total, 93.79% of the samples belonged to the C lineage, 3.2% to the M lineage and 3.01% to the African lineage, Table 1. The M lineage was found in 19 States, while the African lineage was identified in 16 states. Higher African lineage frequencies were found in southern states such as LA (25%), FL (17.4%), TX (12.5%) and the territory of Puerto Rico (87.5%), Figure 3. The last percentage should be carefully considered in light of a restricted number of analyzed samples from this population (n = 8) compared to other states (n = 22), Table 2 and Figure 3.
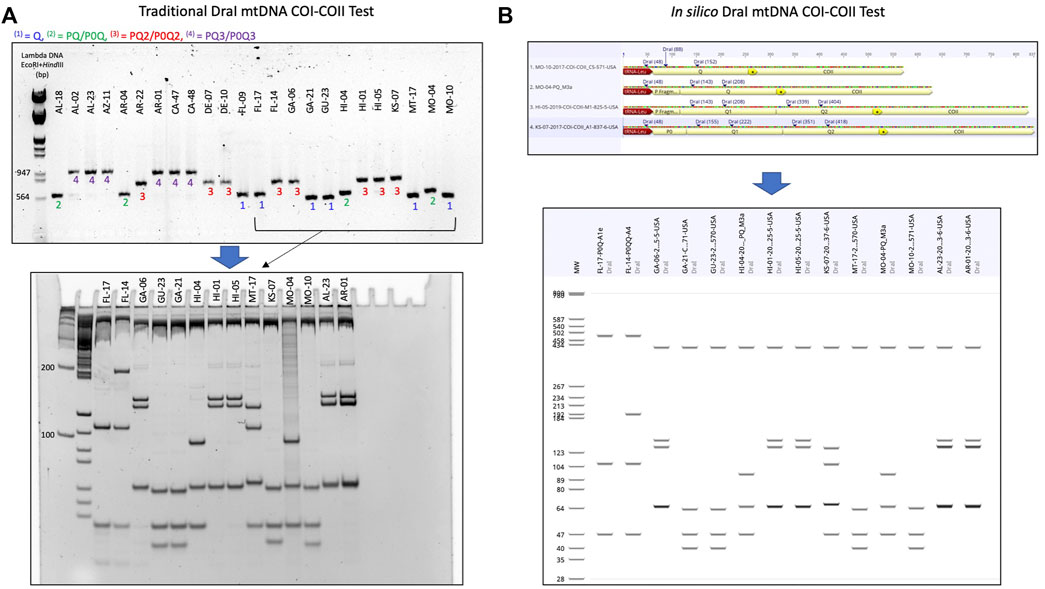
FIGURE 2. Procedure detailing the transition from the traditional DraI mtDNA COI-COII (DmCC) test to the in silico DmCC test. (A) Lineage identification based on the mtDNA COI-COII length revealed on 1.5% agarose gel, DraI enzymatic restriction of the PCR products and visual haplotype identification on 7.5% polyacrylamide gel. (B) Determination of COI-COII genetic structure (Q, PQ, PQ2, P0Q2) by sequence alignment and annotation. In silico DraI restriction of the sequences and haplotype identification on a virtual gel. The same samples shown in (A) were run in (B).
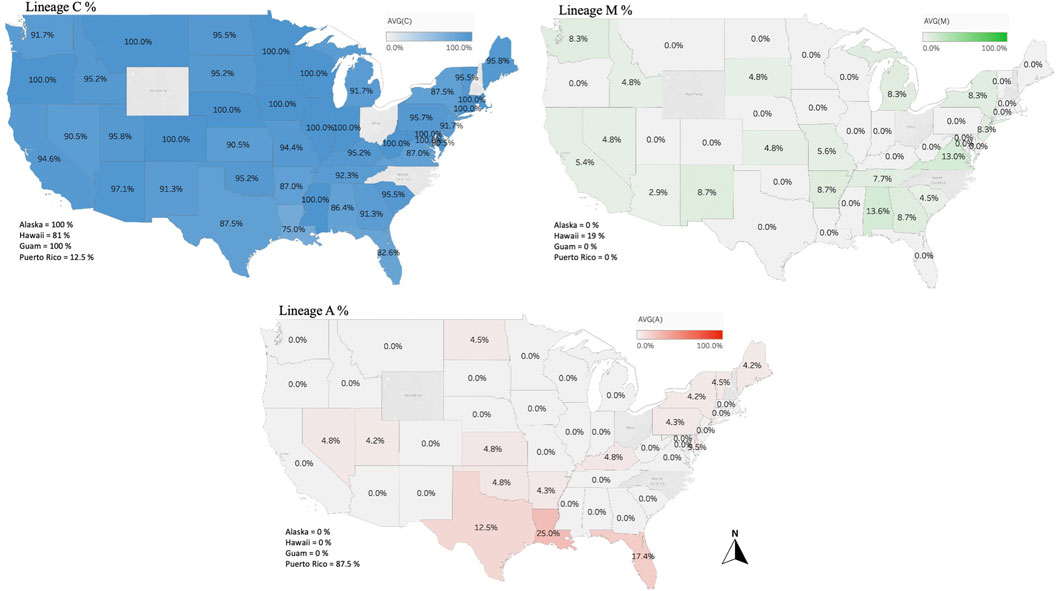
FIGURE 3. Percentages of the three identified maternal lineages (North Mediterranean C, West Mediterranean M and African lineage A) in the US honey bee populations computed and displayed by states and territories. Samples from the States of OH, NC, NH, WY and RI were not available.
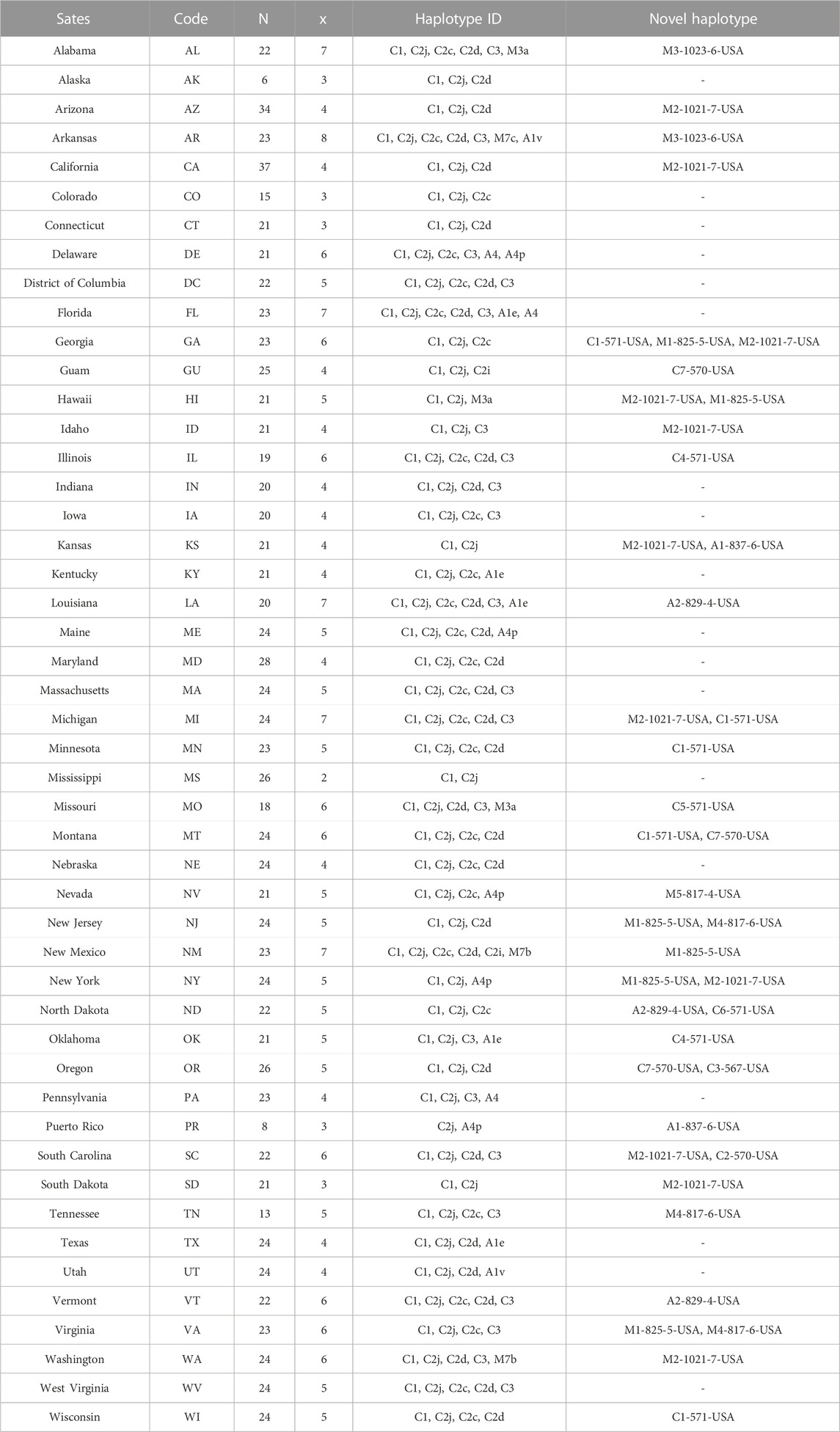
TABLE 2. Haplotype identity and occurrence on a state-by-state basis. Sample size (n), total number of haplotypes found in each population (x). Novel haplotypes found in the USA were named according to the nomenclature detailed in this study.
3.4 COI-COII haplotypes
Overall, 27 haplotypes were identified; 13 (95.11%) had already been reported and 14 (4.87%) others were deemed to be novel haplotypes, Table 1. By far, the two predominant haplotypes found in the USA are C1 and C2j, representing (38.76%) and (37.62%) of the total sample size, respectively (Table 1). The C1 and C2j haplotypes characterize the A. m. ligustica and A. m. carnica subspecies, respectively Figure 4 (Alburaki et al., 2011; Bouga et al., 2011). Haplotype C2i was exclusively found in the isolated island of Guam at a very high rate (87.5%), excluding a single occurrence identified in NM, Table 2. Haplotype C3 which characterizes the Buckfast line (Alburaki et al., 2011; Bouga et al., 2011) was recorded 42 times in an overall percentage of 3.95%, Table 1 and Figure 4. Three typical M haplotypes of A.m. mellifera subspecies (M3a, M7b, M7c) (Rortais et al., 2011; Bertrand et al., 2015) were found in solitary instances in AL, AR, HI, MO, NM and WA. Meanwhile, commonly known haplotypes of the African lineage (A1e, A1v, A4, A4p) were recorded in 11 states and a territory (Puerto Rico), with higher frequencies in Southeastern states (LA, FL, TX), Figure 3 and Table 2.
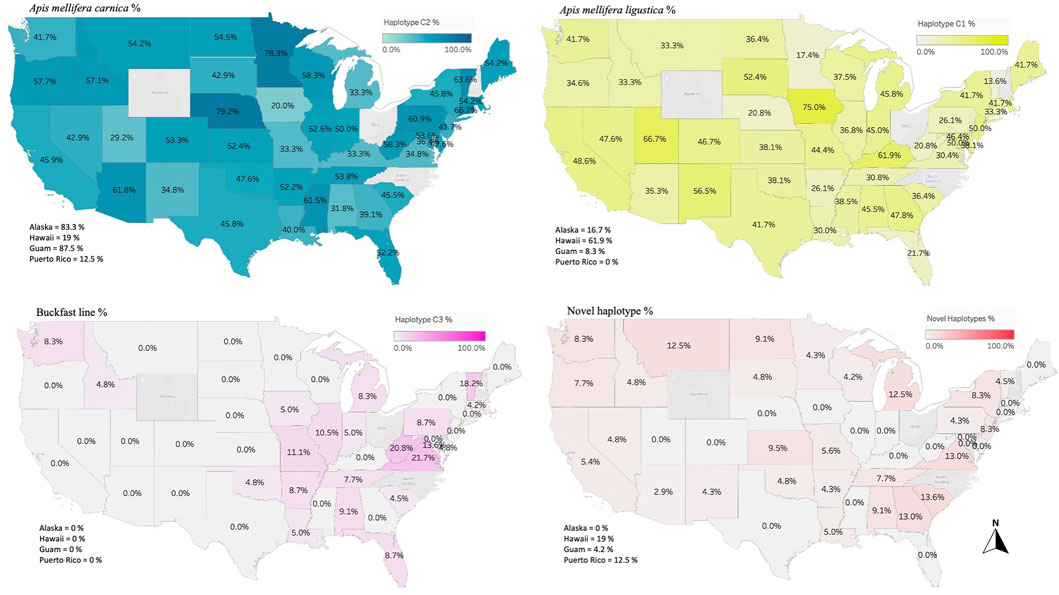
FIGURE 4. Major three haplotypes found in the honey bee populations of the USA. Haplotype C1 characterizes the honey bee subspecies A. m. ligustica (Italian honey bee), C2 haplotype for A. m. carnica (Carniolan honey bee) and C3 represents the Buckfast line. Novel haplotypes found in this study are also provided in percentage of the total analyzed samples per state/territory.
3.5 Novel haplotypes
Seven of the 14 novel haplotypes documented in this study belong to the C lineage, five to the M lineage and two to the A lineage, Table 1. Additionally, three novel haplotypes (C4-571-USA, C7-570-USA, A2-829-4-USA) blasted at 100% with orphan sequences deposited at NCBI with no track records or haplotype identity to reference, Table 1. The five novel haplotypes of the M lineage were identified at low frequencies (1–13) and percentages (0.09%–1.22%) in AL, AZ, AR, CA, HI, ID, KY, MI, NV, NJ, NM, NY, SC, SD, TN, VA and WA, (Table 1 & 2). The most frequently identified novel haplotype (M2-1021-7-USA) was found in 13 apiaries in 11 different States (AZ, CA, GA, HI, ID, KS, MI, NY, SC, SD, WA), signaling significant circulation of this haplotype across the USA, Table 1 & 2. The two novel African haplotypes (A1-837-6-USA, A2-829-4-USA) were shown to exhibit the (P0) fragment of 68bp directly after the tRNA-Leu region with a single replication of the (Q) sequence. Both haplotypes were identified in LA, ND, PR, and VT, Tables 1 & 2.
3.6 Haplotype genetic relationship
The number of haplotypes per state ranged between two to eight, with a national average of (4.9 ± 0.2) haplotypes, Table 2. The national average for haplotype diversity (H) was (0.597) and it ranged between (0.236–0.763) per state (Supplementary Table S1), while the lowest H value was found in GU (0.236) and the highest H was recorded in VA (0.763). No corrected H was calculated as the studied species is not native to the USA. The nucleotide diversity (π) within samples of the C lineage was (0.002) with (H = 0.661) and there was an overwhelming frequency of both C1 and C2j haplotypes (Figures 5, 6), confirming the in silico DmCC results shown in Table 1. Tajima’s statistic (D = −2.49, p < 0.01) indicates a deviation from neutrality and highlights evidence of strong selection activity, Figure 6.
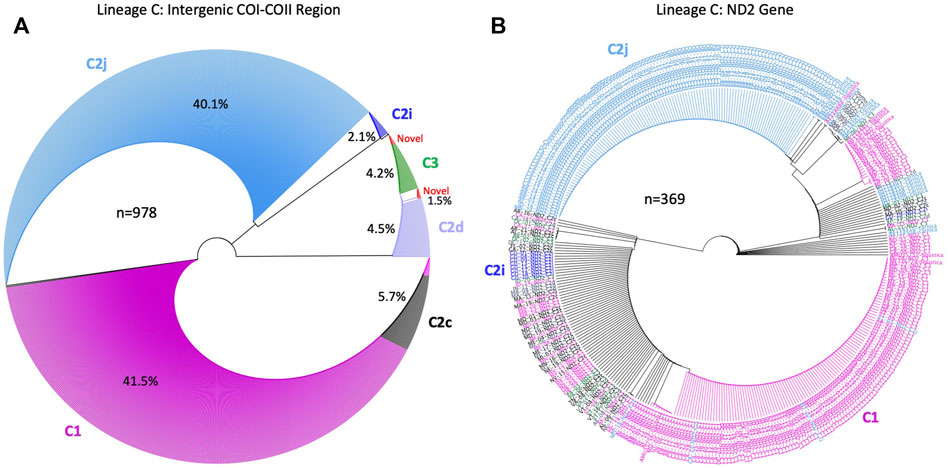
FIGURE 5. Phylogenetic analysis conducted on sequences of the C lineage in two mtDNA genetic regions: (A): COI–COII intergenic region and (B): NADH dehydrogenase 2 (ND2) coding gene (n) is the number of sequences run in each phylogenetic tree, contribution of each COI-COII haplotypes is given in (%). ND2 sequences in (B) were labeled with their respective COI-COII haplotypes identified in (A). Analyses were computed with available NCBI sequences of the C lineage for both genes.
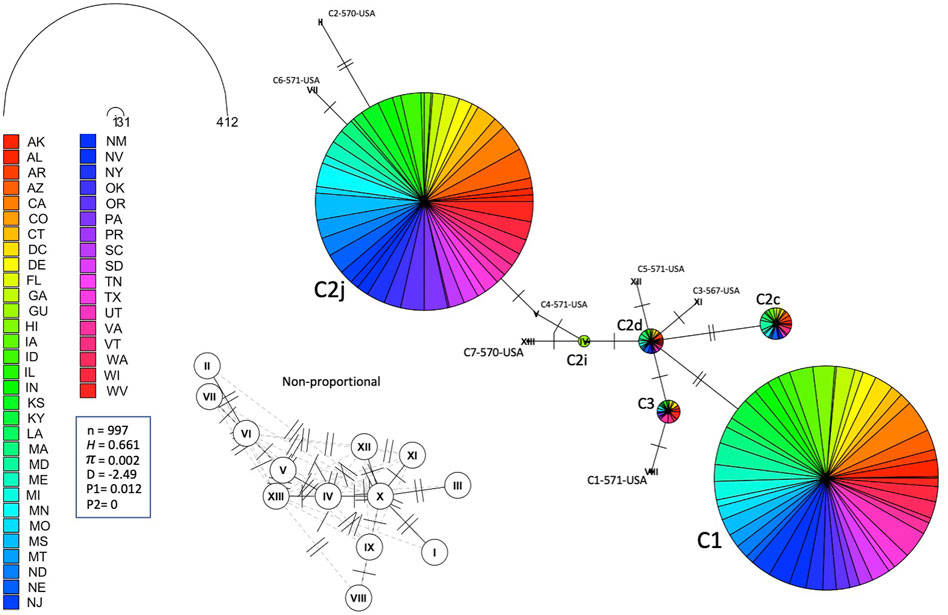
FIGURE 6. Haplotype network of the mtDNA COI-COII region for samples of Lineage C represented by states. Number of samples (n), haplotype diversity (H), nucleotide diversity (π), Tajima’s statistic (D), Tajima’s p-values under normal (P1) and beta (P2) distributions. Circle size is relative to the number of haplotype copies in the dataset. Perpendicular lines on branches represent SNP events. Non-proportional subfigure shows haplotype secondary network (dotted line) without haplotype copy representation. C2j and C1 are the two major haplotypes (76.64%) identified in the USA populations.
Leading on from this, the COI-COII haplotype network suggests that C2d is the ancestral haplotype from which other haplotypes of the C lineage have diverged through (≥1) SNPs, Figure 6. Meanwhile, the M haplotype network shows higher frequencies of novel haplotypes in US populations compared to previously identified M haplotypes found in the native range of the M lineage, Figure 7A. The novel M haplotype (M5-817-4-USA) showed significant deviation from other M haplotypes (Figure 7A), similar to the novel A haplotype (A1-837-6-USA), Figure 7B. In contrast to the M lineage, previously identified haplotypes of the A lineage were pervasive in US populations as compared to their A lineage novel counterparts, Figure 7B.
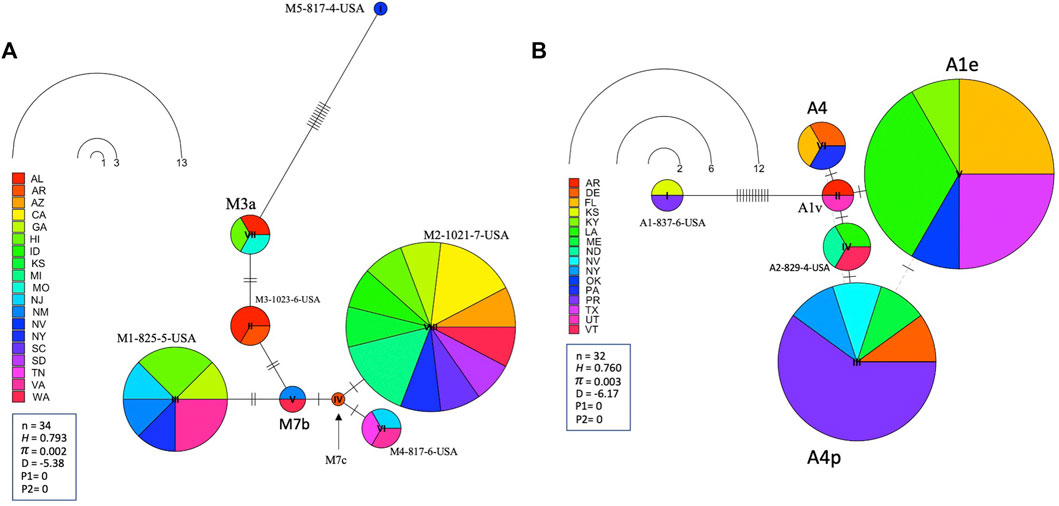
FIGURE 7. State-by-State representation of the COI-COII haplotype network of both M and A lineages; (A) and (B) respectively. Number of samples (n), haplotype diversity (H), nucleotide diversity (π), Tajima’s statistic (D), Tajima’s p-values under normal (P1) and beta (P2) distributions. Circle size is relative to the number of haplotype copies present in the dataset. Perpendicular lines on branches represent nucleotide variations.
The ND2 sequences followed the trend of the COI-COII network but they exhibited slightly higher haplotype (H = 0.773) and nucleotide diversities (π = 0.004), which was due to the difference in the nature and rate of evolution between the two genetic regions, Figure 8. This network classified the sequences into three lineages and confirmed the overwhelming findings of the COI-COII region, particularly the significant predominance of both C1 and C2j haplotypes. Figure 8. Major ND2 haplotype groups were labeled with their respective COI-COII identities. Both haplotype diversities (H1, H2) followed similar trends, with H1 being more conservative, Figure 9. The populations with the highest (H) were AR, LA, VA, IL and VT. In contrast the population with the lowest H value was GU. Tajima’s D indicates that most US populations do not adhere to neutrality and that bottlenecking or high levels of selection pressure are likely occurring among these populations.
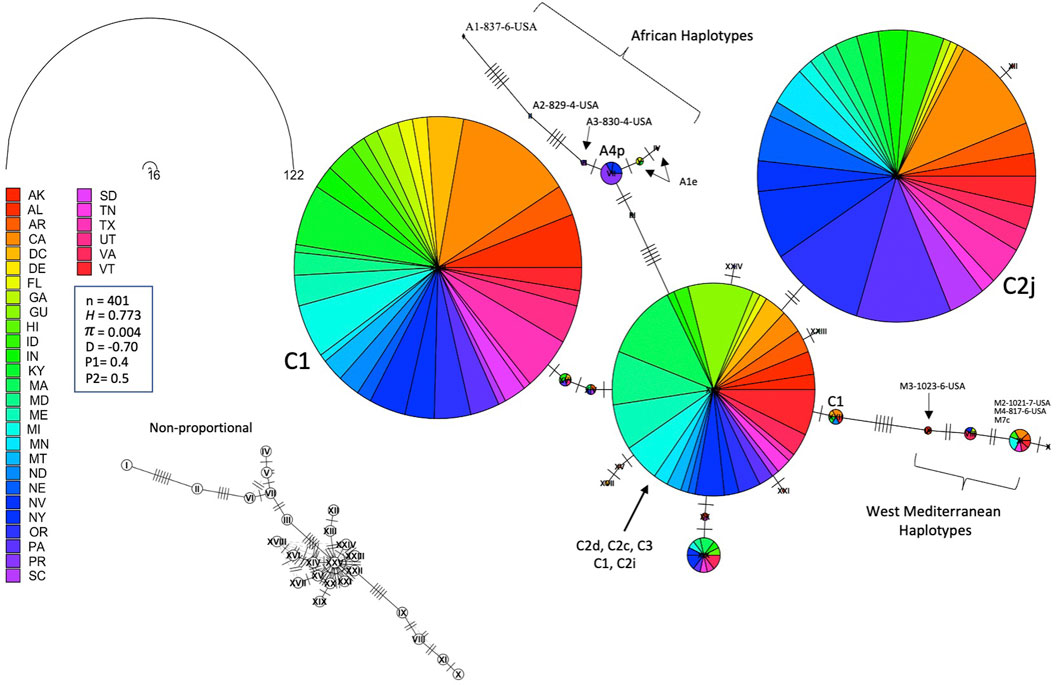
FIGURE 8. Haplotype network of the ND2 gene for samples of all COI-COII lineages displayed by states. Number of samples (n), haplotype diversity (H), nucleotide diversity (π), Tajima’s statistic (D), Tajima’s p-values under normal (P1) and beta (P2) distributions. Circle size is relative to the number of haplotype copies present in the dataset. Nucleic changes are represented by perpendicular lines on branches. Non-proportional subfigure shows the haplotype secondary network (dotted line) without haplotype copy representation. Central ND2 haplotype is an admixture of multiple COI-COII haplotypes.
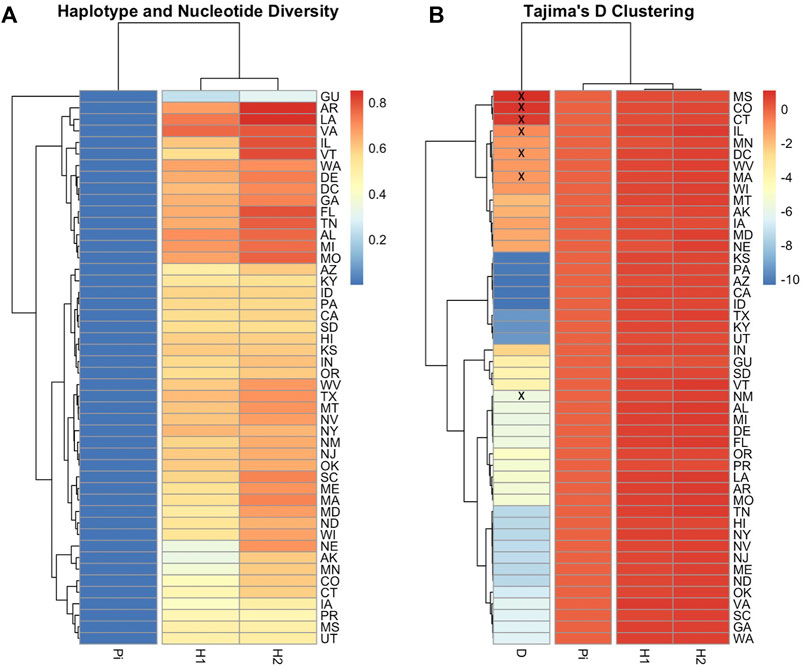
FIGURE 9. Heatmaps illustrating COI-COII gene diversity per population. Nucleotide diversity (Pi), Haplotype diversity calculated based on endonuclease fragment variations (H1) (Nei and Tajima 1981), Haplotype diversity computed on DNA sequences (H2), Tajima’s statistic (D). (A): Genetic relationship among populations based on gene diversity parameters (H1, H2, Pi). (B): Population clusters based on Tajima’s D values. Population with non-significant D values (p > 0.05) were crossed by (x). Full range of data is available in Supplementary Table S1.
3.7 Phylogenetic analyses
The unrooted genetic tree of the COI-COII sequences of the C lineage showed four major clusters representing haplotypes C1, C2j, C2d, and C2i, Figure 5A. The novel haplotypes uncovered in this study clustered within the C2d branch along with haplotype C3. Nevertheless, haplotype C2c clustered within the C1 group showing more genetic proximity with A. m. ligustica than A. m. carnica, Figure 5A. The ND2 coding region demonstrated relatively similar evolution patterns found in the COI-COII region, particularly for C1 and C2j haplotypes, while C2d and C3 did not follow a clear pattern, Figure 5B. In subfigure 5b, ND2 sequences were labeled with the names of their respective COI-COII haplotype. Meanwhile, the alignment of the COI-COII and ND2 sequences provides visual evidence of the actual polymorphisms present among these haplotypes, Supplementary Figure S1. The uniqueness of the C1 haplotype is reflected by a single insertion in its early Q fragment, which cannot be found anywhere else in the C lineage, as well as a substitution in its COII coding region that is shared with C2c and the novel haplotype (C2-570-USA), Supplementary Figure S1.
Multiple deletions and SNPs are observed in the novel haplotypes’ sequences when compared to Old World haplotypes Supplementary Figure S1. Both haplotypes C1 and C2j retained a clear contrast in their ND2 sequences compared to other C haplotypes with a substitution at 40 and 250 bp, respectively, Supplementary Figure S1. This divergence led to their clear distinction (Figure 5) compared to other C haplotypes. The phylogenetic tree rooted with A. cerana revealed three clusters representing the three evolutionary lineages (C, M, A) with a bootstrap value of 53, Figure 10A. Moreover, the analysis of intra-C haplotypes (excluding C1) confirmed the presence of a clear distinction with regards to C2j, differentiating it from other C2 sub-haplotypes in which C2j clustered with reference samples of caucasica subspecies (morphometrical lineage O) (Ruttner, 1988) (Figure 10B). Samples of M and A lineages in the USA clustered in two independent groups with reference samples of A. m. mellifera and other African subspecies (A. m. sahariensis, A. m. lamarckii, A. m. syriaca, A. m. scutellata) with a bootstrap value of 75, Figure 10C.
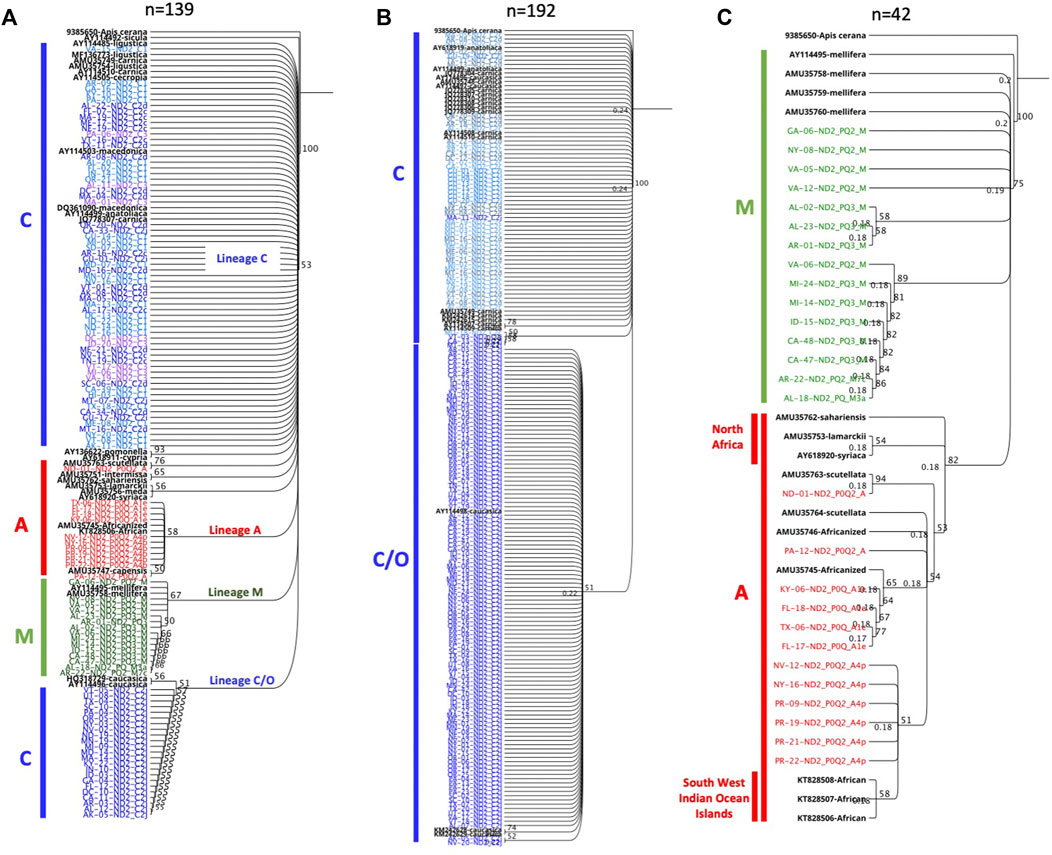
FIGURE 10. Phylogenetic analyses of ND2 coding gene conducted on representative set of samples from three mtDNA COI-COII lineages (C, M, A). Sequences in black font are NCBI reference samples of major honey bee subspecies. Trees are rooted with Apis cerana as an outgroup species and numbers beside the node show bootstrap support values. (A) represents inter-lineage analysis (bootstrap = 53). (B) intra-C haplotype analysis. (C) Samples of lineages M (green) and A (red) discriminated by a bootstrap value of 75.
4 Discussion
Our data shows that the managed honey bee populations of the USA (n = 1,063) belong to three known honey bee evolutionary lineages (C, M, A) with an overwhelming reliance (93.79%) on the North Mediterranean C lineage. The remaining samples belonged to the West Mediterranean M lineage (3.20%) and the African A lineage (3.01%), Table 1. A previous study conducted on feral honey bee colonies (n = 422) sampled from nine states in the USA found that 77% of the samples belonged to the C lineage, 22% to the M lineage and 1% to the A lineage (Schiff and Sheppard, 1993). In a more recent study conducted on unmanaged colonies (n = 247) from 12 states, 83% of the samples belonged to the C lineage, 7% to the M lineage, while 9% were attributed to the Oriental lineage O) (Magnus et al., 2014). As the Oriental lineage cannot be discerned with the DmCC test, we verified the aforementioned study’s sequences, which revealed that the O lineage should actually be characterized as the African lineage A) (Z haplotypes of A. m. syriaca). This issue was discussed in a previous study (Kandemir et al., 2006) and previous COI-COII mtDNA O haplotypes were reclassified accordingly as Z haplotypes of the African lineage A (Alburaki et al., 2011). Another recent study indicated that 100% of the examined managed colonies (n = 11) in PA were of the C lineage (Rangel et al., 2020). Unmanaged colonies, as well as feral swarms, initially originated from the managed stock. Although it is expected for wild populations to follow a more neutral selection path compared to managed counterparts, their diversity often mirrors the managed populations. Taking into consideration our data and previous studies conducted on feral and managed colonies (Schiff et al., 1994; Delaney et al., 2009), it is clear that lineage C is by far the most dominant lineage found in the honey bee populations of the USA, with only traces of M and A lineages.
4.1 The North Mediterranean lineage C
This lineage originates from the North Mediterranean region of Southeast Europe. It includes several well-defined subspecies, such as A. m. ligustica, A. m. carnica, A. m. macedonica, A. m. cecropia, and A. m. cypria. The first two examples are this lineage’s most historically imported and common subspecies (Ruttner 1988; Ilyasov et al., 2020). In our samples, the C lineage is represented by two major haplotypes (C1, C2x), which characterize A. m. ligustica and A. m. carnica subspecies, respectively (Pinto et al., 2014). The Carniolan bee, A. m. carnica, was the most abundant subspecies among the USA populations, indicating significant circulation of Carniolan queen stocks, represented by four haplotypes (C2j, C2c, C2d, C2i), with low frequencies for the last three, Table 1. Haplotype diversity among European populations differs starkly from the US populations. In their native range of repartition (Central and Southeastern Europe), a recent phylogeographic study (n = 564) that used the same COI-COII marker identified a total of 13 C2 native haplotypes (C2d, C2c, C2b, C2e, C2i, C2j, C2q, C2u, C2v, C2w, C2aa, C2ab, C2ag) as well as highly common C2d (38%) and C2c (23.8%) haplotypes, with a haplotype diversity H) of 0.756 (Munoz and de la Rua, 2021). In US populations, the overwhelming reliance appeared to be on the C2j haplotype (37.63%), while C2d (5.46%) and C2c (4.23%) were much less frequent and only displayed a haplotype diversity (H) of 0.542 within the C lineage. As a predominant lineage of the USA, to a great extent, the low H within the C lineage mirrors the national H (0.597), which should be given careful attention. Interestingly, our study confirms the ancestral position of C2d, which was uncovered in Munoz and De La Rua, 2021, from which C2i deviated by a single mutation, Figure 6. Moreover, Three out of seven novel C haplotypes found in the USA’s populations were derived from the ancestral C2d haplotype by just one or two mutations. Despite its overall low percentage in the USA, C2d is widely distributed and was identified in 28 states, suggesting that a genetic shift towards C2j occurred over time in accordance with natural or human selection. Human selection pressure is more probable in this context as most US populations rejected Tajima’s assumption of “neutral evolution” except in MS, CO, CT, IL, DC, MA and NM populations, Figure 9 and Supplementary Table S1. Further genetic investigation is needed of the C2j haplotype within the USA, which constantly clustered with reference samples of A. m. caucasica (Russian bees) in the ND2 phylogenetic analyses, Figure 10.
Italian queens (A. m. ligustica), characterized by the C1 haplotype, were the second most widely circulated queens among beekeepers in the USA (38.76%). Within a sample size of 412 C1 haplotypes spread across extensive geographical and climatic ranges, no COI-COII polymorphisms were found in this subspecies. Regarding the C2i haplotype, the absence of queen importation from Guam to the mainland US states could explain its exclusiveness on the isolated Island. All C1 and C2x haplotypes were identified in the Old World (Europe) in their native geographical ranges (Bouga et al., 2011).
Tracking the geographical location and occurrence rate of the rare and novel mtDNA haplotypes offers a powerful tool that could shed even more light on beekeeping and queen circulations nationwide. For instance, our data shows that honey bee queens carrying the most common novel haplotype (C1-571-USA) were found in sampled operations from four states (GA, MN, MT, WI), followed by the second most common novel haplotype (C7-570-USA), which was found in two States (MT, OR) and the isolated island of Guam. All the other novel haplotypes (C2-570-USA, C3-567-USA, C4-571-USA, C5-571-USA, and C6-571-USA) that were found in SC, OR, OK, MO, and ND respectively, have so far remained state-specific. None of these particular novel haplotypes identified in our study were reported elsewhere, including in the native geographical range of the C lineage, which makes them unique and singles them out as valuable material to further our understanding of the honey bee evolution and beekeeping practices in the USA. The most common and circulating C haplotypes in the USA were those haplotypes that are well documented and broadly known in Europe, while novel ones remained rare and state-restricted for some.
4.2 The West Mediterranean lineage M
This lineage has been widely studied and comprises numerous documented haplotypes that characterize two subspecies; A. m. mellifera and A. m. iberiensis (Rortais et al., 2011; Chávez-Galarza et al., 2017; Henriques et al., 2018). Within the US populations, only 3.2% (n = 34) of the queen bees originated from this lineage which carries three of the most predominant and well-known M haplotypes (M3a, M7c, M7b). In its natural areas of repartition (West Europe), at least 91 haplotypes were described in this lineage (Franck et al., 1998; Rortais et al., 2011), while only three of them (M haplotypes) were identified in the USA. This distinction suggests that queens/bees of this lineage were not imported or incorporated into US breeding programs at the same rate as the C lineage. This low M haplotype integration in US beekeeping practices is surprising considering the lineage’s high genetic diversity and multiple identified ecotypes (Louveaux, 1973). In addition to the three haplotypes mentioned above, five novel M lineage haplotypes were identified, Table 1. These novel haplotypes were identified 28 times within 22 states, with the most abundant (M2-1021-7-USA) found in (AZ, CA, GA, HI, ID, KS, MI, NY, SC, SD and WA). The low number of M haplotypes samples (n = 34) found in the USA undermines the possibility of a comprehensive phylogenetic analysis. Nonetheless, it is safe to conclude that novel M haplotypes, which may have developed local adaptations, are used more widely by beekeepers than the traditional M haplotypes originating in Europe, Figure 7A. Among the lineages in the USA, the M lineage exhibited the highest H (0.720) Supplementary Table S1, as evidenced by eight identified haplotypes in the USA populations from a relatively small sample size (n = 32). This H level is clearly healthier than what was found among USA’s C lineage and it is closer to the higher end (0.324–0.771) of the M (H) that was documented in a previous study (n = 219), which was conducted within the lineage’s native range in France and Spain (Franck et al., 1998).
4.3 The African lineage A
This lineage is native to the African continent and is considered the most genetically diverse lineage comprising over 13 subspecies, such as A. m. lamarckii, A. m. intermissa, A. m. syriaca, A. m. scutellata, A. m. capen, A. m. siciliana and A. m. unicolor (Franck et al., 2001; Alburaki et al., 2011; Henriques et al., 2022). Unfortunately, only a few subspecies of this lineage were characterized by specific haplotypes, which renders the exact determination of a subspecies by molecular means challenging. Africanized honey bees are the results of crossbreeding between A. m. scutellata with various European honey bee subspecies (Smith and Brown, 1988). However, a clear distinction should be drawn between the identification of African mtDNA haplotypes and Africanized bees, particularly knowing that A. m. scutellata has been sporadically introduced into the United States before the arrival of the Africanized honey bees from South America in 1990 (Pinto et al., 2007). Africanization can occur either by gene flow from Africanized drones mating with European queens or by dispersal of Africanized queens in swarms (Smith and Brown, 1988). Thus, both European and A. m. scutellata haplotypes could carry Africanized traits, which renders the mtDNA a non-specific marker for this purpose. In our study, the African lineage represented 3.01% (n = 32) of the analyzed samples with four previously reported haplotypes (A1e, A1v, A4, A4p) and two novel haplotypes (A1-837-6-USA, A2-829-4-USA). The three highest percentages were found in three southern States: (LA: 25%, FL: 17.4%, TX: 12.5%), Figure 3. Both A1 and A4 are widely spread and common haplotypes of the African continent. They were identified at high frequencies in West and Central Africa among populations of A. m. adansoni and A. m. jemenitica (Dukku et al., 2022), in the Northeast of Africa (El-Niweiri and Moritz, 2008), among A. m. intermissa in North Africa (Achou M et al., 2015) as well as in the Middle East in the native range of A. m. syriaca (Alburaki et al., 2011). Meanwhile, Magnus et al.‘s study provided evidence of A. m. syriaca in the USA, which we did not identify in this current study (Magnus et al., 2014). According to the available records, this subspecies was imported to the USA in 1880 (Marcelino et al., 2022). A. m. syriaca is a subspecies of the African lineage A, which carries a distinct COI-COII profile compared to other subspecies of the A lineage (Alburaki et al., 2011). This unique mtDNA profile of A. m. syriaca (Z haplotypes), which was confirmed via genomic analyses (Alburaki et al., 2013), allows reliable attribution of Z haplotypes to this specific subspecies (A. m. syriaca).
5 Conclusion
This study provides a comprehensive and unprecedented analysis of the mtDNA genetic diversity of the honey bee populations in the USA. It details and validates the methodology used to transit a widely used mtDNA genetic marker in honey bees from its traditional way to a more precise and systemic in silico test, eliminating discrepancies and reconciling haplotype identities between various investigations. This work identified and named fourteen novel haplotypes of three honey bee evolutionary lineages that have never been reported before, providing new insights into the evolutionary history of the USA’s honey bees since their importation to North America in the 1600s. Finally, from the perspective of national importance, the overwhelming reliance on two mtDNA haplotypes and a single lineage are all alarming signals of restrictions in the mtDNA haplotype diversity within the honey bee populations of the USA.
Data availability statement
The datasets presented in this study can be found in online repositories. The names of the repository/repositories and accession number(s) can be found in the article/Supplementary Material.
Author contributions
Conceptualization and Design: MA, DV. Sampling: DV, MA. Fund acquisition: MA, YC, Methodology and Data Collection: MA, SM, JL. Data Analysis: MA. Data Curation: MA, MB, YC, DV. Wrote First Draft: MA. Revised Manuscript: MB, YC, DV, SM. All authors have read and approved the content of this manuscript.
Acknowledgments
We thank the beekeepers who volunteered their apiaries for sampling through the National Honey Bee Disease Survey (NHBDS). The survey and samples made available for this project would not have been possible without the dedicated NHBDS staff Rachel Fahey, Ashrafun Nessa, Heather Eversole, Nathan Swan, Mikalya Wilson and UMD undergraduate students. We appreciate the help of the Apiary Inspectors of America, and the Bee Informed Partnership for sample collection. This work of the NHBDS was made possible with the help of UMD Bee Lab project manager Karen Rennich. USDA-APHIS Farm Bill funded the collection of the 2019 NHBDS samples under agreement number AP18PPQHQ000C014.
Conflict of interest
The authors declare that the research was conducted in the absence of any commercial or financial relationships that could be construed as a potential conflict of interest.
Publisher’s note
All claims expressed in this article are solely those of the authors and do not necessarily represent those of their affiliated organizations, or those of the publisher, the editors and the reviewers. Any product that may be evaluated in this article, or claim that may be made by its manufacturer, is not guaranteed or endorsed by the publisher.
Supplementary material
The Supplementary Material for this article can be found online at: https://www.frontiersin.org/articles/10.3389/fgene.2022.1092121/full#supplementary-material
References
Abrahamovich, A. H., Atela, O., De La Rúa, P., and Galián, J. (2007). Assessment of the mitochondrial origin of honey bees from Argentina. J. Api. Res. 46, 191–194. doi:10.3896/ibra.1.46.3.10
Achou, M., Loucif-Ayad, L., Legout, H., Hmidan, H., Alburaki, M., et al. (2015). An insightful molecular analysis reveals foreign honeybees among Algerian honeybee populations (Apis mellifera L.). J. Data Min. Genomics Proteomics 6, 1. doi:10.4172/2153-0602.1000166
Alburaki, M., and Alburaki, A. (2008). Morphometrical study on Syrian honey bee Apis mellifera syriaca. Emir. J. Food Agric. 20, 89. doi:10.9755/ejfa.v20i1.5184
Alburaki, M., Bertrand, B., Legout, H., Moulin, S., Alburaki, A., Sheppard, W. S., et al. (2013). A fifth major genetic group among honeybees revealed in Syria. BMC Genet. 14, 117. doi:10.1186/1471-2156-14-117
Alburaki, M., Moulin, S., Legout, H., Alburaki, A., and Garnery, L. (2011). Mitochondrial structure of eastern honeybee populations from Syria, Lebanon and Iraq. Apidologie 42, 628–641. doi:10.1007/s13592-011-0062-4
Arias, M. C., Rinderer, T. E., and Sheppard, W. S. (2006). Further characterization of honey bees from the Iberian Peninsula by allozyme, morphometric and mtDNA haplotype analyses. J. Api. Res. 45, 188–196. doi:10.3896/ibra.1.45.4.04
Arias, M. C., and Sheppard, W. S. (1996). Molecular phylogenetics of honey bee subspecies (Apis mellifera L.) inferred from mitochondrial DNA sequence. Mol. Phylogenet. Evol. 5, 557–566. doi:10.1006/mpev.1996.0050
Avise, J. C., Arnold, J., Ball, R. M., Bermingham, E., Lamb, T., Neigel, J. E., et al. (1987). Intraspecific phylogeography: The mitochondrial DNA bridge between population genetics and systematics. Annu. Rev. Ecol. Syst. 18, 489–522. doi:10.1146/annurev.es.18.110187.002421
Avise, J. C. (2000). Phylogeography: The history and formation of species. Cambridge: Harvard University Press, 447.
Bertrand, B., Alburaki, M., Legout, H., Moulin, S., Mougel, F., and Garnery, L. (2015). MtDNA COI-COII marker and drone congregation area: An efficient method to establish and monitor honeybee (Apis mellifera L.) conservation centres. Mol. Ecol. Resour. 15, 673–683. doi:10.1111/1755-0998.12339
Bouga, M., Alaux, C., Bienkowska, M., Büchler, R., Carreck, N. L., et al. (2011). A review of methods for discrimination of honey bee populations as applied to European beekeeping. J. Apic. Res. 50, 51. doi:10.3896/IBRA.1.50.1.06
Bouga, M., Harizanis, P. C., Kilias, G., and Alahiotis, S. (2005). Genetic divergence and phylogenetic relationships of honey bee Apis mellifera (Hymenoptera: Apidae) populations from Greece and Cyprus using PCR-RFLP analysis of three mtDNA segments. Apidologie 36, 335–344. doi:10.1051/apido:2005021
Calderone, N. W. (2012). Insect pollinated crops, insect pollinators and US agriculture: Trend analysis of aggregate data for the period 1992-2009. PLoS One 7, e37235. doi:10.1371/journal.pone.0037235
Carpenter, M. H., and Harpur, B. A. (2021). Genetic past, present, and future of the honey bee (Apis mellifera) in the United States of America. Apidologie 52, 63–79. doi:10.1007/s13592-020-00836-4
Chávez-Galarza, J., Garnery, L., Henriques, D., Neves, C. J., Loucif-Ayad, W., Jonhston, J. S., et al. (2017). Mitochondrial DNA variation of Apis mellifera iberiensis: Further insights from a large-scale study using sequence data of the tRNAleu-cox2 intergenic region. Apidologie 48, 533–544. doi:10.1007/s13592-017-0498-2
Chen, C., Liu, Z., Pan, Q., Chen, X., Wang, H., Guo, H., et al. (2016). Genomic analyses reveal demographic history and temperate adaptation of the newly discovered honey bee subspecies Apis mellifera sinisxinyuan n. ssp. Mol. Biol. Evol. 33, 1337–1348. doi:10.1093/molbev/msw017
Cornuet, J. M., Garnery, L., and Solignac, M. (1991). Origin and function of the intergenic region between COI and COII of Apis mellifera L. mitochondrial DNA. Genetics 128, 393–403. doi:10.1093/genetics/128.2.393
De la Rua, P., Serrano, J., and Galian, J. (1998). Mitochondrial DNA variability in the canary islands honeybees (Apis mellifera L.). Mol. Ecol. 7, 1543–1547. doi:10.1046/j.1365-294x.1998.00468.x
Delaney, D. A., Meixner, M. D., Schiff, N. M., and Sheppard, W. S. (2009). Genetic characterization of commercial honey bee (Hymenoptera: Apidae) populations in the United States by using mitochondrial and microsatellite markers. Ann. Entomological Soc. Am. 102, 666–673. doi:10.1603/008.102.0411
Dukku, U. H., Fuchs, S., Danailu, G., Grünewald, B., Tofilski, A., Kryger, P., et al. (2022). Morphometric and mitochondrial variation of Apis mellifera L. and its relationship with geographical variables in parts of West and Central Africa. J. Apic. Res. 61, 296–304. doi:10.1080/00218839.2022.2030000
El-Niweiri, M. A. A., and Moritz, R. F. A. (2008). Mitochondrial discrimination of honeybees (Apis mellifera) of Sudan. Apidologie 39, 566–573. doi:10.1051/apido:2008039
Estoup, A., Garnery, L., Solignac, M., and Cornuet, J. M. (1995). Microsatellite variation in honey bee (Apis mellifera L.) populations: Hierarchical genetic structure and test of the infinite allele and stepwise mutation models. Genetics 140, 679–695. doi:10.1093/genetics/140.2.679
Franck, P., Garnery, L., Celebrano, G., Solignac, M., and Cornuet, J. M. (2000). Hybrid origins of honeybees from Italy (Apis mellifera ligustica) and sicily (A. m. sicula). Mol. Ecol. 9, 907–921. doi:10.1046/j.1365-294x.2000.00945.x
Franck, P., Garnery, L., Loiseau, A., Oldroyd, B. P., Hepburn, H. R., et al. (2001). Genetic diversity of the honeybee in Africa: Microsatellite and mitochondrial data. Heredity 86, 420–430. doi:10.1046/j.1365-2540.2001.00842.x
Franck, P., Garnery, L., Solignac, M., and Cornuet, J. M. (1998). The origin of west European subspecies of honeybees (Apis mellifera): New insights from microsatellite and mitochondrial data. Evolution 52, 1119–1134. doi:10.1111/j.1558-5646.1998.tb01839.x
Ftayeh, A., Meixner, M., and Fuchs, S. (1994). Morphometrical investigation in Syrian honeybees. Apidologie 25, 396–401. doi:10.1051/apido:19940406
Garnery, L., Cornuet, J. M., and Solignac, M. (1992). Evolutionary history of the honey bee Apis mellifera inferred from mitochondrial DNA analysis. Mol. Ecol. 1, 145–154. doi:10.1111/j.1365-294x.1992.tb00170.x
Garnery, L., Franck, P., Baudry, E., Vautrin, D., Cornuet, J. M., and Solignac, M. (1998b). Genetic diversity of the west European honey bee (Apis mellifera mellifera and A. m. iberica). II. Microsatellite loci. Genet. Sel. Evol. 30, S49–S74. doi:10.1186/1297-9686-30-s1-s49
Garnery, L., Franck, P., Baudry, E., Vautrin, D., Cornuet, J. M., and Solignac, M. (1998a). Genetic diversity of the west European honey bee (Apis mellifera mellifera and A. m. iberica). I. Mitochondrial DNA. Genet. Sel. Evol. 30, S31–S47. doi:10.1186/1297-9686-30-s1-s31
Garnery, L., Solignac, M., Celebrano, G., and Cornuet, J. M. (1993). A simple test using restricted pcr-amplified mitochondrial-DNA to study the genetic-structure of apis-mellifera L. Experientia 49, 1016–1021. doi:10.1007/bf02125651
Hall, H. G., and Smith, D. R. (1991). Distinguishing African and European honeybee matrilines using amplified mitochondrial DNA. Proc. Natl. Acad. Sci. U. S. A. 88, 4548–4552. doi:10.1073/pnas.88.10.4548
Harrison, R. G. (1989). Animal mitochondrial DNA as a genetic marker in population and evolutionary biology. Trends Ecol. Evol. 4, 6–11. doi:10.1016/0169-5347(89)90006-2
Henriques, D., Browne, K. A., Barnett, M. W., Parejo, M., Kryger, P., Freeman, T. C., et al. (2018). High sample throughput genotyping for estimating C-lineage introgression in the dark honeybee: An accurate and cost-effective SNP-based tool. Sci. Rep. 8, 8552. doi:10.1038/s41598-018-26932-1
Henriques, D., Costa, C., Rufino, J., and Pinto, M. A. (2022). The mitochondrial genome of Apis mellifera siciliana. Mitochondrial DNA. B Resour. 7, 828–830. doi:10.1080/23802359.2022.2073844
Ilyasov, R. A., Lee, M. L., Takahashi, J. I., Kwon, H. W., and Nikolenko, A. G. (2020). A revision of subspecies structure of Western honey bee Apis mellifera. Saudi J. Biol. Sci. 27, 3615–3621. doi:10.1016/j.sjbs.2020.08.001
Kandemir, I., Kence, M., and Kence, A. (2000). Genetic and morphometric variation in honeybee (Apis mellifera L.) populations of Turkey. Apidologie 31, 343–356. doi:10.1051/apido:2000126
Kandemir, I., Pinto, M. A., Meixner, M. D., and Sheppard, W. S. (2006). Hinf-I digestion of cytochrome oxidase I region is not a diagnostic test for A. m. lamarckii. Genet. Mol. Biol. 29, 747–749. doi:10.1590/s1415-47572006000400027
Kauhausen-Keller, D., Ruttner, F., and Keller, R. (1997). Morphometric studies on the microtaxonomy of the species Apis mellifera L. Apidologie 28, 295–307. doi:10.1051/apido:19970506
Kulhanek, K., Steinhauer, N., Rennich, K., Caron, D. M., Sagili, R. R., Pettis, J. S., et al. (2017). A national survey of managed honey bee 2015–2016 annual colony losses in the USA. J. Apic. Res. 56, 328–340. doi:10.1080/00218839.2017.1344496
Loucif-Ayad, W., Achou, M., Legout, H., Alburaki, M., and Garnery, L. (2014). Genetic assessment of Algerian honeybee populations by microsatellite markers. Apidologie 46, 392–402. doi:10.1007/s13592-014-0331-0
Louveaux, J. (1973). The acclimatization of bees to a heather region. Bee world 54, 105–111. doi:10.1080/0005772x.1973.11097464
Madella, S., Grubbs, K., and Alburaki, M. (2021). Non-invasive genotyping of honey bee queens Apis mellifera L.: Transition of the DraI mtDNA COI-COII test to in silico. Insects 12 (1), 19. doi:10.3390/insects12010019
Magnus, R. M., Tripodi, A. D., and Szalanski, A. L. (2014). Mitochondrial DNA diversity of honey bees (Apis mellifera) from unmanaged colonies and swarms in the United States. Biochem. Genet. 52, 245–257. doi:10.1007/s10528-014-9644-y
Marcelino, J., Braese, C., Christmon, K., Evans, J. D., Gilligan, T., Giray, T., et al. (2022). The movement of western honey bees (Apis mellifera L.) among U.S. States and territories: History, benefits, risks, and mitigation strategies. Front. Ecol. Evol. 10, 151–166. doi:10.3389/fevo.2022.850600
Meixner, M. D., Arias, M. C., and Sheppard, W. S. (2000). Mitochondrial DNA polymorphisms in honey bee subspecies from Kenya. Apidologie 31, 181–190. doi:10.1051/apido:2000115
Meixner, M. D., Worobik, M., Wilde, J., Fuchs, S., and Koeniger, N. (2007). Apismellifera melliferain eastern Europe – morphometric variation and determination of its range limits. Apidologie 38, 191–197. doi:10.1051/apido:2006068
Moritz, R. F. A., Cornuet, J. M., Kryger, P., Garnery, L., and Hepburn, H. R. (1994). Mitochondrial DNA variability in South African honeybees (Apis mellifera L). Apidologie 25, 169–178. doi:10.1051/apido:19940205
Munoz, I., and de la Rua, P. (2021). Correction to: Wide genetic diversity in Old World honey bees threaten by introgression. Apidologie 52, 696. doi:10.1007/s13592-021-00856-8
Nei, M., and Tajima, F. (1981). DNA polymorphism detectable by restriction endonucleases. Genetics 97, 145–163. doi:10.1093/genetics/97.1.145
Palmer, M. R., Smith, D. R., and Kaftanoglu, O. (2000). Turkish honeybees: Genetic variation and evidence for a fourth lineage of Apis mellifera mtDNA. J. Hered. 91, 42–46. doi:10.1093/jhered/91.1.42
Panziera, D., Requier, F., Chantawannakul, P., Pirk, C. W. W., and Blacquière, T. (2022). The diversity decline in wild and managed honey bee populations urges for an integrated conservation approach. Front. Ecol. Evol. 10. doi:10.3389/fevo.2022.767950
Paradis, E. (2010). pegas: an R package for population genetics with an integrated-modular approach. Bioinformatics 26, 419–420. doi:10.1093/bioinformatics/btp696
Paradis, E., and Schliep, K. (2019). Ape 5.0: an environment for modern phylogenetics and evolutionary analyses in R. Bioinformatics 35, 526–528. doi:10.1093/bioinformatics/bty633
Pinto, M. A., Henriques, D., Chávez-Galarza, J., Kryger, P., Garnery, L., van der Zee, R., et al. (2014). Genetic integrity of the dark European honey bee (Apis mellifera mellifera) from protected populations: A genome-wide assessment using SNPs and mtDNA sequence data. J. Apic. Res. 53, 269–278. doi:10.3896/ibra.1.53.2.08
Pinto, M. A., Sheppard, W. S., Johnston, J. S., Rubink, W. L., Coulson, R. N., Schiff, N. M., et al. (2007). Honey bees (hymenoptera: Apidae) of african origin exist in non-africanized areas of the southern United States: Evidence from mitochondrial DNA. Annals . Entomol. Soc. Am. 100, 289–295. doi:10.1603/0013-8746(2007)100[289:hbhaoa]2.0.co;2
R Core Team (2022). R: A language and environment for statistical computing. Vienna, Austria: R Foundation for Statistical Computing. Available at: https://www.R-project.org/.
Rangel, J., Traver, B., Stoner, M., Hatter, A., Trevelline, B., Garza, C., et al. (2020). Genetic diversity of wild and managed honey bees (Apis mellifera) in Southwestern Pennsylvania, and prevalence of the microsporidian gut pathogens Nosema ceranae and N. apis. Apidologie 51, 802–814. doi:10.1007/s13592-020-00762-5
Rortais, A., Arnold, G., Alburaki, M., Legout, H., and Garnery, L. (2011). Review of the DraI COI-COII test for the conservation of the black honeybee (Apis mellifera mellifera). Conserv. Genet. Resour. 3, 383
Ruttner, F., Tassencourt, L., and Louveaux, J. (1978). Biometrical-Statistical Analysis Of The Geographic Variability Of APIS Mellifera L. I. Material and Methods. Apidologie 9, 363–381. doi:10.1051/apido:19780408
Schiff, N. M., Sheppard, W. S., Loper, G. M., and Shimanuki, H. (1994). Genetic diversity of feral honey bee (hymenoptera: Apidae) populations in the southern United States. Ann. Entomological Soc. Am. 87, 842–848.
Schiff, N. M., and Sheppard, W. S. (1993). Mitochondrial DNA evidence for the 19th century introduction of African honey bees into the United States. Experientia 49, 530–532. doi:10.1007/bf01955156
Sheppard, W. S., and Meixner, M. (2003). Apis mellifera pomonella, a new honey bee subspecies from Central Asia. Apidologie 34, 367–375. doi:10.1051/apido:2003037
Smith, D. R., and Brown, W. M. (1988). Polymorphisms in mitochondrial DNA of European and Africanized honeybees (Apis mellifera). Experientia 44, 257–260. doi:10.1007/BF01941730
Smith, D. R., and Brown, W. M. (1990). Restriction endonuclease cleavage site and length polymorphisms in mitochondrial DNA of Apis mellifera mellifera and A. M. carnica (hymenoptera: Apidae). Ann. Entomological Soc. Am. 83, 81–88. doi:10.1093/aesa/83.1.81
Smith, D. R., Palopoli, M. F., Taylor, B. R., Garnery, L., Cornuet, J. M., SolignacM., , et al. (1991). Geographical overlap of two mitochondrial genomes in Spanish honeybees (Apis mellifera iberica). J. Hered. 82, 96–100. doi:10.1093/oxfordjournals.jhered.a111062
Tamura, K., and Nei, M. (1993). Estimation of the number of nucleotide substitutions in the control region of mitochondrial DNA in humans and chimpanzees. Mol. Biol. Evol. 10, 512–526. doi:10.1093/oxfordjournals.molbev.a040023
Keywords: A. mellifera, genetic diversity, honey bee populations, haplotype, mtDNA, evolutionary lineages, ND2 gene
Citation: Alburaki M, Madella S, Lopez J, Bouga M, Chen Y and vanEngelsdorp D (2023) Honey bee populations of the USA display restrictions in their mtDNA haplotype diversity. Front. Genet. 13:1092121. doi: 10.3389/fgene.2022.1092121
Received: 07 November 2022; Accepted: 01 December 2022;
Published: 04 January 2023.
Edited by:
Antonia Picornell, University of the Balearic Islands, SpainReviewed by:
MariaTeresa Rebelo, University of Lisbon, PortugalLuca Fontanesi, University of Bologna, Italy
Copyright © 2023 Alburaki, Madella, Lopez, Bouga, Chen and vanEngelsdorp. This is an open-access article distributed under the terms of the Creative Commons Attribution License (CC BY). The use, distribution or reproduction in other forums is permitted, provided the original author(s) and the copyright owner(s) are credited and that the original publication in this journal is cited, in accordance with accepted academic practice. No use, distribution or reproduction is permitted which does not comply with these terms.
*Correspondence: Mohamed Alburaki, bW9oYW1lZC5hbGJ1cmFraUB1c2RhLmdvdg==