- Department of Respiratory and Critical Care Medicine, The Second Hospital of Jilin University, Changchun, China
Metabolic reprogramming is an important hallmark of malignant tumors. Serine is a non-essential amino acid involved in cell proliferation. Serine metabolism, especially the de novo serine synthesis pathway, forms a metabolic network with glycolysis, folate cycle, and one-carbon metabolism, which is essential for rapidly proliferating cells. Owing to the rapid development in metabolomics, abnormal serine metabolism may serve as a biomarker for the early diagnosis and pathological typing of tumors. Targeting serine metabolism also plays an essential role in precision and personalized cancer therapy. This article is a systematic review of de novo serine biosynthesis and the link between serine and folate metabolism in tumorigenesis, particularly in lung cancer. In addition, we discuss the potential of serine metabolism to improve tumor treatment.
Introduction
Emerging evidence suggests that metabolic reprogramming of cancer not only affects tumor progression and molecular pathways but also regulates the tumor immunochemical microenvironment and drug resistance to chemotherapy. Tumor cells satisfy specific bioenergetic and biosynthetic needs through metabolic reprogramming. However, abnormal accumulation of cellular metabolites can stimulate cell carcinogenesis and promote tumor progression (Pavlova and Thompson, 2016; Martínez-Reyes and Chandel, 2021). Metabolic reprogramming involves a series of complex metabolic changes, including increased nutrient intake, enhanced glycolytic metabolism, altered amino acid metabolism, abnormal redox homeostasis, and aberrant fatty acid oxidation (Boroughs and DeBerardinis, 2015). These metabolic changes often occur simultaneously and interact with one another. In particular, serine metabolism is closely related to glycolytic reactions and one-carbon (1C) unit metabolism and provides essential substrates for the biosynthesis of molecules required for cell proliferation, thus playing an important role in tumorigenesis and immunity (Rodriguez et al., 2019; Kurniawan et al., 2020). In this paper, we provide a detailed review of the process of serine metabolism and summarize recent advances in serine metabolism in cancer, especially lung cancer.
De novo serine synthesis pathway
Deregulation of cellular energetics is one of the most pervasive hallmarks of cancer (Hanahan and Weinberg, 2011). Compared to normal differentiated cells, cancer cells prefer aerobic glycolysis to provide energy for cell growth. This phenomenon is also called the “Warburg effect” (Vander Heiden et al., 2009). Aerobic glycolysis produces ATP inefficiently but confers many advantages to tumor cells, especially in supporting cell anabolic reactions (Lunt and Vander Heiden, 2011). Therefore, the de novo serine synthesis pathway (SSP) is an important turning point for glucose conversion. SSP is the process by which the glycolytic intermediate, 3-phosphoglycerate (3-PG), is converted to serine by 3-phosphoglycerate dehydrogenase (PHGDH), phosphoserine aminotransferase (PSAT1), and phosphoserine phosphatase (PSPH). Serine is then converted to glycine by serine hydroxymethyl transferase (SHMT) (Figure 1).
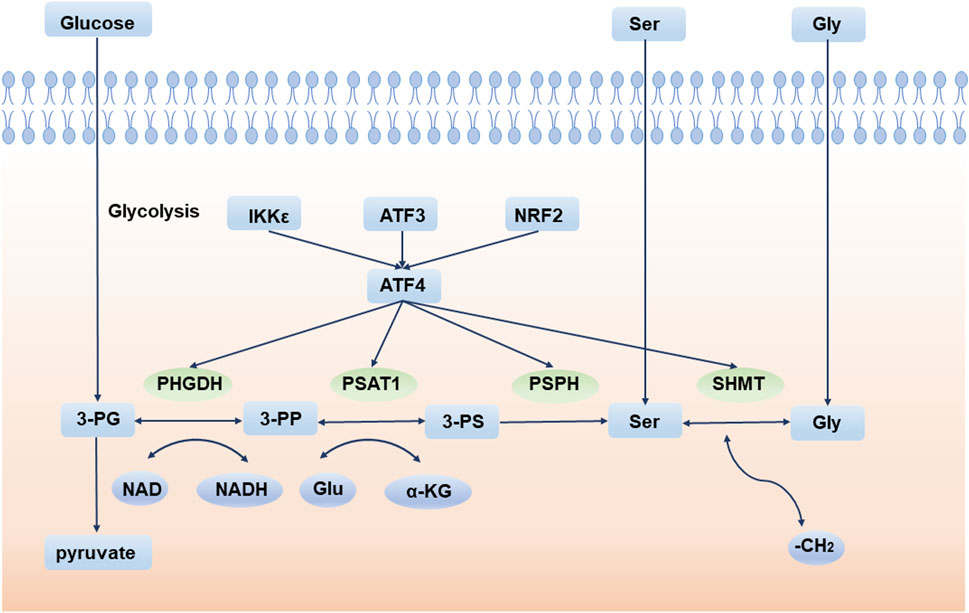
FIGURE 1. De novo serine synthesis pathway and its regulator. 3-PG is converted to serine by PHGDH, PSAT1, and PSPH. Serine is then converted to glycine by SHMT. 3-PG: 3-phosphoglycerate; 3-PP: 3-phosphohydroxypyruvate; 3-PS: 3-phosphoserine; PHGDH: 3-phosphoglycerate dehydrogenase; PSAT1: phosphoserine aminotransferase; PSPH: phosphoserine phosphatase; SHMT: serine hydroxymethyl transferase; Ser: serine; Gly: glycine; Glu: glutamate. IKKε: IκB Kinase ε; ATF3: activating transcription factor 3; NRF2: NFE2 like bZIP transcription factor 2; ATF4: activating transcription factor 4.
Glucose enters the serine pathway from the glycolysis intermediate product, 3-PG, which is the origin of SSP. Serine, the product of SSP, is a non-essential amino acid (NEAA) that is an essential precursor for protein, nucleic acid, and lipid synthesis (Amelio et al., 2014). For example, L-serine and palmitoyl CoA are critical sphingolipid units. In astrocytes, the process by which glucose metabolism produces serine and further synthesizes sphingolipids, is essential for brain development and neuronal survival (Hirabayashi and Furuya, 2008). Conversely, toxic deoxysphinganine (doxSA), which is produced upon serine depletion, damages both vascular and nervous systems (Gantner et al., 2019). More importantly, serine metabolism and glycine synthesis are inextricably linked in biology, providing 1C units for the sustainability of nucleotides, S-adenosylmethionine (SAM), reduced nicotinamide adenine dinucleotide phosphate (NADPH), and glutathione (GSH) (Amelio et al., 2014).
Under normal circumstances, serine in cells comes from two sources: food intake and intracellular synthesis from the SSP pathway. When the exogenous serine intake is insufficient, SSP is enhanced to produce more serine. Activating transcription factor 4 (ATF4) is the main regulator of SSP and directly binds to and modulates the transcription of the SSP core enzymes PHGDH, PSAT1, PSPH, and SHMT (Wortel et al., 2017). In response to amino acid starvation or endoplasmic reticulum (ER) stress, ATF4 is activated to initiate the transcription of downstream molecules and adapt to environmental stress (B'Chir et al., 2013). Furthermore, a variety of regulators indirectly regulate the SSP pathway via ATF4. For example, experiments have indicated that activating transcription factor 3 (ATF3) is crucial for the activation of the SSP pathway, especially when serine is deprived. ATF4 is first activated during serine deprivation, and then ATF3 is quickly activated depending on ATF4. ATF3 not only increases the expression of ATF4, but also promotes the expression of PHGDH, PSAT1, and PSPH by interacting with their enhancers and promoters. ATF3 also recruits E1A-binding protein p300 to transactivate key SSP enzymes (Li et al., 2021a). Another example is NFE2-like bZIP transcription factor 2 (NRF2), an essential transcription factor frequently deregulated in non-small cell lung cancer (NSCLC), which regulates SSP enzymes by targeting ATF4. Experiments show that after knockdown of NRF2, ATF4 is decreased at the protein level but does not change significantly at the transcription level. As NRF2 does not bind to key SSP enzyme promoters directly, it can be inferred that NRF2 regulates serine biosynthesis by promoting the expression of ATF4 (DeNicola et al., 2015). Additionally, IκB Kinase ε (IKKε), a key kinase that affects tumors and inflammation, is overexpressed in these tumors. IKKε reduces glucose-derived pyruvate utilization in the TCA cycle to decrease mitochondrial function, thus activating ATF4 (Xu et al., 2020). Lysine demethylase 4C (KDM4C) regulates serine pathway genes by removing the trimethylation modification of H3 lysine 9 (H3K9), which requires the involvement of ATF4 (Zhao et al., 2016). The GCN2-ATF4 (Pathria et al., 2018) and eIF2α-ATF4 (B'Chir et al., 2013) signaling pathways also play important roles in the process of tumor cells coping with amino acid starvation and maintaining cell proliferation.
SSP and one-carbon metabolism
The 1C unit refers to an organic group containing one carbon atom, including methylene, methenyl, methyl, and formyl (Locasale, 2013; Shetty and Varshney, 2021). The 1C unit cycle carried by tetrahydrofolate is the fundamental center of cellular metabolism. The 1C unit is not only a major source of purine and pyrimidine synthesis participating in DNA and RNA synthesis but also sustains GSH and contributes to cellular redox homeostasis, which is critical for maintaining rapid tumor cell proliferation (Ducker and Rabinowitz, 2017). Isotope labeling experiments implicate amino acids, especially serine, as major one-carbon sources (Davis et al., 2004; Newman and Maddocks, 2017). During the conversion of serine to glycine, a methylene group breaks off from serine and enters the folate cycle. With the conversion of the 1C unit carried on tetrahydrofolate (THF), the methylene group from serine enters the methionine cycle. Ultimately, this 1C unit acts as a methyl donor in the form of a SAM (Figure 2).
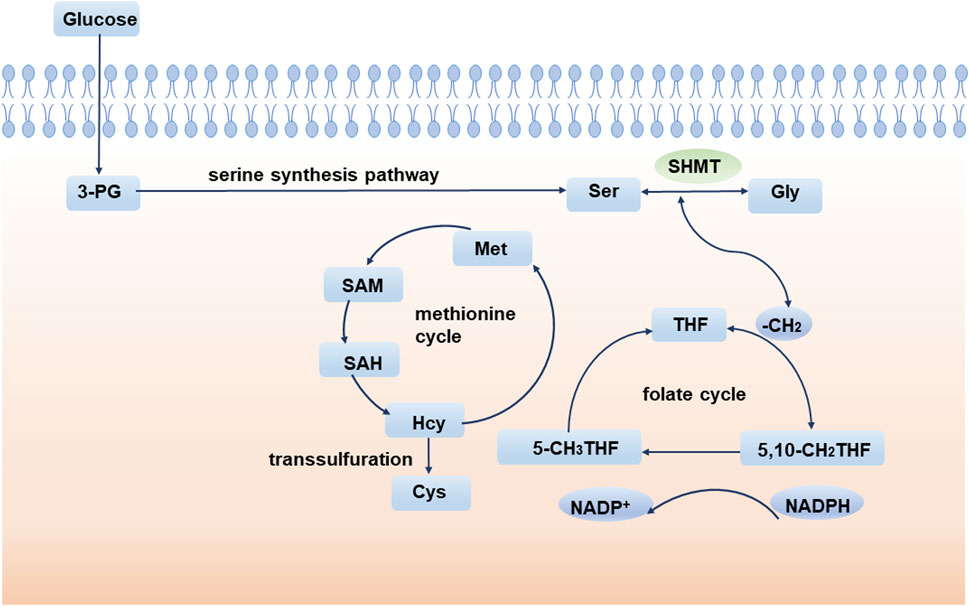
FIGURE 2. SSP and one-carbon metabolism. During the conversion of serine to glycine, a methylene group breaks off from serine and enters the folate cycle. Ultimately, this 1C unit acts as a methyl donor in the form of a SAM. 3-PG: 3-phosphoglycerate; SHMT: serine hydroxymethyl transferase; Ser: serine; Gly: glycine; THF: tetrahydrofolate; Met: methionine; SAM: S-adenosyl methionine; SAH: S-adenosyl-L-homocysteine; Hcy: homocysteine.
Homocysteine (Hcy) is an important component of the methionine cycle and is a precursor of cysteine (Cys) biosynthesis. Glutathione (GSH) is an important low molecular antioxidant in cells, which is composed of glutamic acid, cysteine, and glycine (Forman et al., 2009). Studies have shown that cysteine synthesis mediated by transsulfuration is very important to promote tumor cell growth, especially when extracellular cysteine uptake is limited (Zhu et al., 2019). GSH is one of the most abundant metabolites in cells and can maintain the redox balance of cells by scavenging and reducing reactive oxygen species and maintaining an appropriate NADPH/NADP + ratio (Forman et al., 2009; Fan et al., 2014). GSH has been implicated in aging and various human diseases, such as Alzheimer’s disease (Mandal et al., 2015; Mahajan et al., 2020), Parkinson’s disease (Li et al., 1997), and diabetes (Sekhar et al., 2011). Since GSH affects the oxidation, differentiation, proliferation, and apoptosis of cells, abnormalities in GSH are also closely related to the occurrence and development of various cancers (Traverso et al., 2013). In conclusion, abnormal 1C metabolism and intracellular redox abnormalities are important mechanisms by which serine metabolism affects tumor progression.
Key enzymes in SSP in lung cancer
The morbidity and mortality rates of lung cancer are the highest among all malignant tumors. Recent research suggests that numerous metabolic abnormalities are involved in the development of lung cancer (Ma et al., 2021; Morrissey et al., 2021). Abnormal metabolism promotes the progression of lung cancer, and this dependence on abnormal metabolism also provides the biochemical basis for the specific killing of lung cancer. Compared with other types of cancer, lung cancer has more targeted therapy and immunotherapy drugs, and its treatment plan is more complex. However, lung cancer cells are often resistant to conventional antitumor therapies because the metabolic heterogeneity of lung cancer leads to metabolic symbiosis and therefore causes poor treatment outcomes (Yoshida, 2015). Abnormal serine metabolism, particularly the enhancement of SSP, is prevalent in lung cancer (Chen et al., 2019). Elevation of key SSP enzymes, such as PHGDH, PSAT1, and PSPH, is an important factor in the malignant progression of lung cancer cells and cancer drug resistance. In addition, these key enzymes can interact with other signaling pathways to promote lung cancer progression (Figure 3). Thus, the study of abnormal serine metabolism in lung cancer is a new way to solve the problems of lung cancer-targeted therapy resistance and immunotherapy tolerance.
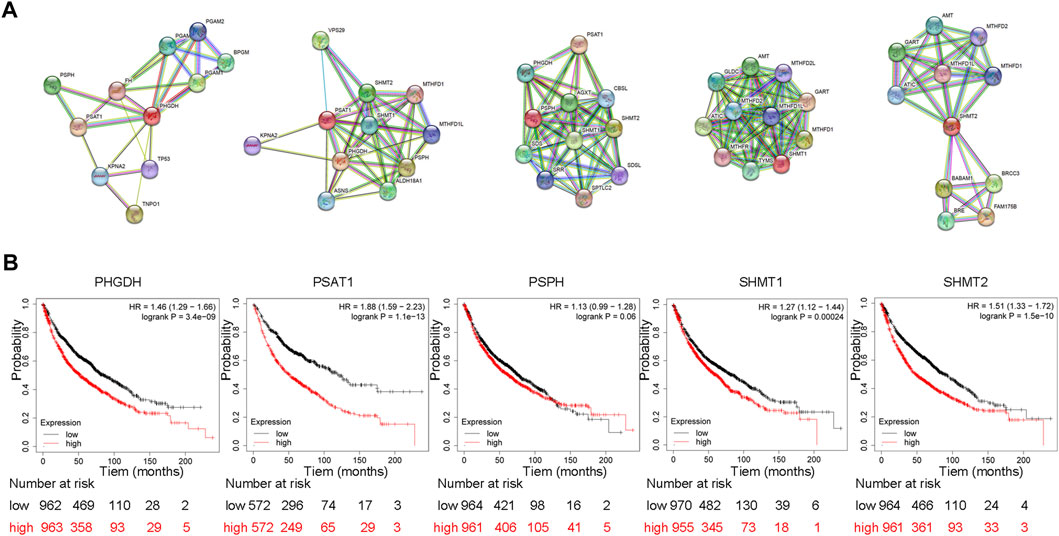
FIGURE 3. Protein interactions of SSP core enzymes and their effect on patient’s overall survival (A) Protein interactions of SSP core enzymes. We retrieved PHGDH, PSAT1, PSPH, SHMT1 and SHMT2 on STRING (https://cn.string-db.org/) and choose Homo sapiens to get the protein interaction network of SSP key enzymes in human cells. (B) SSP core enzyme’s function influences patient survival rates in lung cancer. The prognostic value of PHGDH, PSAT1, PSPH, SHMT1 and SHMT2 expression was analyzed using Kaplan-Meier Plotter (http://kmplot.com/analysis/). The results showed that the overall survival of patients with high expression of PHGDH, PSAT1, SHMT1 and SHMT2 was significantly decreased.
PHGDH
PHGDH is the rate-limiting enzyme in the first step of SSP and catalyzes the conversion of 3-PG, an intermediate in glycolysis and gluconeogenesis, to 3-phosphohydroxypyruvate (3-PP). NAD is an important cofactor for this process. PHGDH is highly expressed in various tumors and is associated with tumorigenesis, drug resistance, and a poor prognosis (Possemato et al., 2011; Ma et al., 2021) (Table 1). For example, PHGDH is upregulated in platin-resistant ovarian cancer and is regulated by the RNA-binding protein DDX3X and lncRNA RMRP (Bi et al., 2021). PHGDH is an indispensable factor in breast cancer pulmonary metastasis, which elevates the mTOR complex 1 (mTORC1) signaling pathway and defines sensitivity to rapamycin in tumor metastases (Rinaldi et al., 2021). Meanwhile, PHGDH is also a critical molecule of hepatocellular carcinoma resistance to sorafenib. High expression of PHGDH inhibits sorafenib-induced apoptosis of liver cancer cells by activating SSP and inhibiting alpha-ketoglutarate (α-KG), serine, and NADPH (Wei et al., 2019).
PHGDH is also a high-expression proto-oncogene in lung cancer that promotes cancer progression by activating serine synthesis. Proteomic analysis of six small cell lung carcinoma (SCLC) and six pulmonary carcinoid tumor (PCT) tissues indicated that PHGDH overexpression is significantly associated with cancer metabolism and poor overall survival (OS) (Fujii et al., 2018). Quantitative reverse transcriptase polymerase chain reaction (qRT-PCR) and immunohistochemistry experiments in 319 NSCLC samples and 143 control samples further showed that high expression of PHGDH was significantly correlated with pathological features of patients, such as lymph node metastasis (p = .021) and TNM stage (p = .016) (Zhu et al., 2016).
Bioinformatics analysis of 720 lung adenocarcinoma patients and tissue microarray analysis of 75 lung adenocarcinoma (LUAD) and adjacent normal tissues revealed that PHGDH is a metabolic subtype of LUAD. 13C isotopomer flux analysis demonstrated that these cells maintain a higher level of glycolysis and generate more serine from glucose. Compared with low-PHGDH-expressing cells, high-PHGDH-expressing cells maintain the characteristics of rapid proliferation and migration. This is mainly because enhanced serine metabolism promotes purine and pyrimidine precursor synthesis for massive DNA synthesis in rapidly proliferating tumor cells (Zhang et al., 2017). Moreover, the expression of PHGDH is required for the erlotinib-resistant LUAD cell lines PC9ER4 and HCC827ER9 (Dong et al., 2018). Inhibition of PHGDH re-sensitizes LUAD cells resistant to erlotinib treatment. Bioinformatic analysis and experiments showed that overexpression of PHGDH promoted GSH metabolism through the SSP pathway and downstream methionine cycle. GSH counteracts reactive oxygen species (ROS) and further inhibits the damage caused by chemotherapeutic drugs to the DNA, proteins and lipids of tumor cells.
Regulation of epigenetic pathways is an essential mode of regulation of PHGDH. Parkin, an E3 ubiquitin ligase, ubiquitinates PHGDH at lysine 330, causing PHGDH degradation and attenuating serine synthesis. Consequently, low expression of Parkin in lung cancer contributes to the high expression of PHGDH (Liu et al., 2020). In colorectal cancer, PHGDH is also monoubiquitinated by cullin 4A–based (Cul4A-based) E3 ligase complex at lysine 146. Conversely, by recruiting DnaJ homolog subfamily A member 1 (DNAJA1), K146 monoubiquitination (K146mUb) enhances PHGDH activity rather than promotes PHGDH degradation (Zhang et al., 2021a).
Post-transcriptional regulation by non-coding RNAs also plays a pivotal role in modulating PHGDH expression. Studies have shown that the circ_0062682/miR-940/PHGDH axis promotes serine metabolism and tumorigenesis in colorectal cancer, which may be a potential novel therapeutic target (Sun et al., 2021).
PSAT1
PSAT1 is the second key enzyme in SSP and catalyzes the conversion of 3-PP to 3-phosphoserine (3-PS). The essential transamination reaction in which glutamate is converted to α-KG occurs simultaneously. PSAT1 is generally overexpressed in malignant tumors (Feng et al., 2022), and plays an important role in regulating tumor progression (Table 2). For instance, PSAT1 is highly expressed in ovarian cancer and is a candidate subtype-specific biomarker suggesting that the tumor is most likely a clear cell carcinoma (Toyama et al., 2012; Zheng et al., 2019a). PSAT1 is also the top-ranked upregulated gene in colorectal cancer (CRC), which is relevant to poor chemotherapy response and prognosis of patients (Qian et al., 2017). Cell cycle analysis suggested that PSAT1 modulates chemotherapy sensitivity by inhibiting cell death and promoting recovery from oxaliplatin-induced G2/M arrest (Zhang et al., 2020).
PSAT1 is highly expressed in NSCLC and promotes cell cycle progression, cell proliferation, and tumorigenesis. Cyclin D1 is a type of D-type cyclin that regulates the cell cycle in G1 phase and G1-S phase, thereby controlling cell proliferation (Tchakarska and Sola, 2020). Western blotting (WB) experiments suggest that knockdown of PSAT1 inhibits cyclin D1 expression and decreases Rb phosphorylation and early 2 factor (E2F) transcription activity. PSAT1 inhibits the activity of serine/threonine protein kinase glycogen synthase kinase 3b (GSK-3B) by promoting its phosphorylation at Ser-9. Phosphorylation of GSK-3B can inhibit the degradation of cyclin D1 by inhibiting its phosphorylation at Thr-286. Therefore, the overexpression of PSAT1 promotes the Cyclin D1 activity but does not affect cyclin D1 expression at the mRNA level (Yang et al., 2015). In addition, PSAT1 promotes the nuclear translocation of pyruvate kinase M2 (PKM2) in response to EGFR activation, thus promoting lung cancer progression (Biyik-Sit et al., 2021).
Furthermore, PSAT1 is highly expressed in LUAD and is correlated with clinicopathological events and poor clinical outcomes. By analyzing PSAT1-based transcriptomics microarray chips, it was found that interferon regulatory factor 1 (IRF1) and its downstream protein, interferon induced with helicase C domain 1 (IFIH1), are inhibited by the overexpression of PSAT1. The results of Ingenuity Pathway Analysis (IPA) and relative luciferase activity further suggest that when PSAT1 is overexpressed, the activities of interferon-γ (IFNγ) and signal transducer and activator of transcription 1 (STAT1), the upstream factors of IRF1, are inhibited. Further studies have found that overexpression of PSAT1, inhibits the phosphorylation of STAT1 at Y701 and S727, resulting in the suppression of dimerization and DNA binding of STAT1. In conclusion, PSAT1 inhibits LUAD metastasis by inhibiting the IFNγ/STAT1/IRF1/IFIH1-axis and leads to poor prognosis in LUAD patients (Chan et al., 2020). Furthermore, in patients with EGFR inhibitor resistance, the abnormal upregulation of PSAT1 inhibits the ROS-dependent JNK/c-Jun pathway, thereby inhibiting cell apoptosis. PSAT1 interacts with IQGAP1 and stimulates STAT3-mediated cell migration. In general, high expression of PSAT1 not only promotes tumor metastasis, but also causes resistance to EGFR inhibitors, which together leads to poor prognosis in LUAD patients (Luo et al., 2022).
The expression of PSAT1 is also regulated by multiple mechanisms. As mentioned earlier, ATF4 is a canonical regulator that promotes PSAT1 overexpression (Gao et al., 2017). In addition, the transcriptional regulators TAZ and YAP (TAZ/YAP) induce PSAT1 expression to drive oncogenic traits, such as metastasis and drug resistance (Mohamed et al., 2016; Yang et al., 2018a; Kim et al., 2022). Moreover, the luciferase assay suggested that miRNAs miR-145–5p (Ding et al., 2022), miR-340 (Yan et al., 2015), and miR-424 (Fang et al., 2018) inhibit the expression of PSAT1 by directly targeting the 3′untranslated regions. There are also studies indicating that long non-coding RNA RP4-694A7.2 (Fan et al., 2021), MEG3 (Li et al., 2020a) and MEG8 (Guo et al., 2021) modulate PSAT1 expression.
PSPH
PSPH, also known as PSP or PSPHD, belongs to a subfamily of phosphotransferases. PSPH is the last rate-limiting enzyme of SSP and is responsible for the conversion of phospho-L-serine to L-serine. Recent studies have shown that PSPH is highly expressed in a variety of cancers and mediates malignant behaviors such as tumor proliferation, metastasis, and poor prognosis (Jovov et al., 2012; Parada et al., 2017) (Table 3).
PSPH is also an upregulated oncogene in NSCLC that regulates tumor progression and correlates with the clinical stage and pathological features. Using qRT-PCR experiments in 73 pairs of NSCLC and adjacent non-tumorous tissues, it was found that PSPH expression level is associated with TNM stage (p < .01) and lymph node and/or distal metastasis (p < .05). Cell function experiments suggested that PSPH enhance NSCLC cell proliferation and migration and promote the cell cycle in the G2-M phase. WB experiments showed that after inhibiting PSPH expression, phosphorylation rather than total protein levels of ERK, MEK, and P38 is suppressed, indicating that PSPH promotes NSCLC metastasis through the MAPK signaling pathway (Liao et al., 2019).
Insulin receptor substrate 1 (IRS1) is a protein phosphorylated by insulin receptor tyrosine kinase, which acts as an essential regulator in the progression of metabolic diseases (Copps and White, 2012). Moreover, IRS1 regulates the development of drug resistance in various tumors, such as breast cancer (Choi et al., 2019), and has attracted widespread attention as a target for tumor-targeted therapy (Hao et al., 2014). Proteomic analyses indicated that IRS1 might be a specific substrate of PSPH. Furthermore, immunoprecipitation and immunoblot assays validated that PSPH regulates IRS1 dephosphorylation at Ser-794, and that the D20 of PSPH is the active site. The Ser-794 site of IRS1 is an inhibitor of the downstream molecules Akt and the p70 S6 kinase phosphorylation site. Therefore, overexpression of PSPH promotes activation of the PI3K/Akt/mTOR signaling pathway by inhibiting the phosphorylation of IRS1. This is an important mechanism by which PSPH promotes lung cancer progression in vitro and in vivo (Park et al., 2019).
SHMT
SHMT sustains cell growth and proliferation in normal and tumor tissues by regulating one-carbon metabolism (Guiducci et al., 2019). In human cells, SHMT has two isoforms: SHMT1 in the cytosol and SHMT2 in the mitochondria (Tramonti et al., 2018). In the cytosol and mitochondria, SHMT1 and SHMT2 catalyze the conversion of serine and THF to glycine and 5,10-methylene tetrahydrofolates (5, 10-CH2-THF), respectively. In general, the 1C unit required for the proliferation of various cancer cells is produced by serine metabolism in the mitochondria and transported by folate. During 1C unit generation, SHMT2 directly catalyzes the conversion of serine to glycine and directs the folate cycle production. SHMT1 is likely to regulate the folate cycle and one-carbon metabolism (Giardina et al., 2018). However, studies have shown that cells with low expression of the reduced folate carrier (RFC) solute carrier family 19 member 1 (SLC19A1) are more dependent on the cytoplasmic folate cycle regulated by SHMT1 (Lee et al., 2021). This result supports the view that mitochondrial folic acid metabolism is not the sole contributor to 1C units in tumors. In summary, both SHMT1 and SHMT2 play important roles in the development of various tumors (Tables 4, 5).
In lung cancer, high SHMT1 expression promotes cell proliferation. SHMT1 knockdown causes cell cycle arrest and p53-dependent apoptosis. Further experiments have indicated that reducing the expression of SHMT1 can reduce dTMP synthesis, leading to increased abnormal uracil accumulation during DNA replication. This causes high genomic instability and DNA strand breaks. More importantly, this type of apoptosis cannot be rescued by adding glycine or serine to the medium. These results suggest that targeting SHMT1 may contribute to the treatment of patients with lung cancer (Pandey et al., 2014; Paone et al., 2014).
SHMT2 is a proto-oncogene that is highly expressed in various human carcinogenesis (Lee et al., 2014), such as breast cancer (Bernhardt et al., 2017), kidney renal papillary cell carcinoma, liver hepatocellular carcinoma, and gastrointestinal tumors (Liu et al., 2019). Kyoto Encyclopedia of Genes and Genomes (KEGG) pathway analysis indicates that SHMT2 is enriched in 5 pathways including one carbon pool by folate (hsa00670), metabolic pathways (hsa01100), biosynthesis of antibiotics (hsa01130), glyoxylate and dicarboxylate metabolism (hsa00630), and glycine, serine, and threonine metabolism (hsa00260). Moreover, the expression of SHMT2 is related to the clinicopathological characteristics of the tumor, such as the patient’s age and tumor stages (Usman et al., 2022).
In lung cancer, SHMT2 is also a highly expressed oncogene, and its expression is highly related to tumor-infiltrating lymphoytes (Luo et al., 2021) and shorter OS (Koseki et al., 2018). SHMT2 maintains lung cancer development mainly through classical regulation of 1C metabolism. Other specific mechanisms have not yet been identified. SIRT5, a sirtuin, binds to SHMT2 and mediates its desuccinylation at lysine 280. Since hypersuccinylation of SHMT2 inhibits its enzymatic activity, SIRT5 can enhance the promotion of SHMT2 on tumor proliferation (Yang et al., 2018b).
Targeting SSP in the treatment of tumor
Many tumors show serine dependence and serine starvation triggers serine synthesis from glucose and glycine, which causes altered metabolism compared to that in normal tissues (Li and Ye, 2020; Tajan et al., 2021). Serine/glycine uptake is thought to be closely related to cancer cell proliferation, as serine and glycine are interconverted under the catalysis of SHMT. Further experiments have shown that the uptake of serine, rather than glycine, supported the 1C unit metabolism. The uptake of glycine cannot substitute serine to promote cell proliferation alone (Labuschagne et al., 2014). Studies have shown that both serine deprivation and excess glycine inhibit cell proliferation (Li et al., 2021a). This phenomenon is related to the production of 5,10-methylene-tetrahydrofolate, which is supported during the conversion of serine to glycine and is depleted when glycine is converted to serine (Maddocks et al., 2013). However, high expression of PHGDH, PSAT1, and PSPH is ubiquitous in tumor cells, which activates endogenous serine metabolism and thus weakens the effect of serine starvation on tumor treatment. PHGDH is the first key enzyme of SSP that plays a cancer-promoting role in multiple tumors. Experiments show that a combination of PHGDH inhibitor (PHGDHi) and medium lacking serine and glycine (-SG) impedes tumor growth by inhibiting DNA, purine and GSH synthesis (Montrose et al., 2021). More importantly, supplementation with 1C unit or glycine alone did not rescue tumor cell proliferation. Adding glycine and 1C units simultaneously can partially rescue cell proliferation by recovering ATP and GTP synthesis. Furthermore, PHGDHi/-SG treatment reduced global protein synthesis, the protective response to serine depletion in vitro and showed better therapeutic results than monotherapy in vivo (Tajan et al., 2021).
Even if exogenous serine is sufficient, inhibiting the expression of key SSP enzymes has anti-tumor effects on some specific cancers, which provides new targets for tumor precision therapy (Pacold et al., 2016). The currently studied drugs that inhibit key SSP enzymes are summarized in Table 6. For instance, NCT-503 (IC50 = 2.5 ± .6 μM) is synthesized and identified as a potent PHGDH inhibitor that inhibits intracellular serine and glycine in stably overexpressing PHGDH MDA-MB-231 cells (MDA-MB-231-PHGDH). NCT-503 does not regulate other amino acids such as aspartate. Similarly, isotope labeling experiments suggest that NCT-503 only controls SSP and 1C unit metabolism, but does not affect the process of glycolysis to 3-PG. Cellular experiments indicated that knockout of PHGDH by NCT-503 is selectively toxic to PHGDH-dependent cell lines, but not to other PHGDH-independent cell lines. Therefore, NCT-503 may be used as a targeted therapy for tumors, such as lung cancer, breast cancer, and other tumors that highly express PHGDH (Pacold et al., 2016). Notably, most drugs identified as SSP inhibitors have not yet been used in clinical treatments. Studies on drug reuse have also identified some clinical drugs that inhibit SSP, but this pharmacological effect has not been incorporated into clinical treatment.
Moreover, the combination of SSP key enzyme inhibitors and chemotherapy drugs will achieve better therapeutic effects (Jing et al., 2015; Ross et al., 2017; Li et al., 2021b). For example, in bladder cancer, compared to individual agents, PHGDH inhibition promotes gemcitabine/cisplatin-induced antitumor effects by suppressing serine biosynthesis and inhibiting cancer cell viability (Yoshino et al., 2020).
Drugs can also regulate the SSP pathway by affecting the post-translational modification of key enzymes, thereby exerting tumor suppressor effects. P300/CBP is a crucial epigenetic regulator of glycolysis and SSP metabolic enzymes that acetylate histone H3K18/K27 during transcriptional activation. A-485 and B029-2 are two selective and highly potent p300 inhibitors that suppress tumor cell metabolism and tumor progression by targeting p300/CBP and reducing the levels of key metabolic enzyme genes, such as PSPH, PSAT1 promoter region H3K18Ac, and H3K27Ac. Therefore, A-485 and B029-2 could be used as potential therapeutic strategies for lung cancer, liver cancer, and other metabolically abnormal tumors (Cai et al., 2021).
Remarkably, most drugs targeting key SSP enzymes inhibit tumor cell proliferation by inhibiting endogenous serine metabolism, but there are also some special mechanisms (Zhao et al., 2020; Arlt et al., 2021). For example, the multi-kinase inhibitor regorafenib directly stabilizes PSAT1 to maintain its high expression, thereby activating PRKAA-dependent autophagy. Therefore, high PSAT1 expression is essential for regorafenib to kill tumor cells (Jiang et al., 2020).
Conclusion
Studies have shown that even if exogenous serine is sufficient, de novo serine biosynthesis is required in tumor cells (Reid et al., 2018). Serine is an important node in cancer cell metabolism (Mattaini et al., 2016). SSP provides serine to cancer cells for protein synthesis. SSP, together with glycolysis and 1C metabolism, forms a metabolic network that is crucial for the occurrence of tumors (Reina-Campos et al., 2020). Specifically, SSP is an important destination for the glycolytic intermediate 3-PG. After 3-PG enters SSP, it no longer provides energy for cancer cells but is converted into serine through a three-step catalytic reaction, and finally provides a carbon source for 1C metabolism. Thus, cellular glucose participates in molecular synthesis rather than providing energy. Although cell replication requires energy, to maintain a high level of 3-PG to support anabolic reactions in cells, aerobic glycolysis is also important for cancer cells with rapid proliferation (Li and Zhang, 2016). Consequently, this metabolic mode partly explains the Warburg effect in tumor cells. Notably, although de novo serine synthesis may provide a type of flow for glycolytic intermediates, this requirement for serine synthesis is not the only reason for the unusual uptake of glucose in tumors.
PHGDH is the first rate-limiting enzyme of SSP and largely determines its flow. The key SSP enzymes PHGDH, PSAT1, and PSPH are significantly overexpressed in a variety of cancers and are associated with poor patient outcomes. In the past, mechanistic research and drug development have mainly targeted PHGDH. No known metabolic reaction can bypass PSAT1 or PSPH to complete the downstream reaction catalyzed by PHGDH (Buqué et al., 2021). Drugs targeting PSAT1 and PSPH may function similarly to those targeting PHGDH. Here, we propose that the use of targeted drugs according to the different expression levels of key SSP enzymes in different tumors is the next research direction.
The study of tumor metabolism originated with the discovery of the Warburg effect (Warburg et al., 1927; Warburg, 1956). With the discovery of numerous oncogenes, cancer is recognized as a genetic disease (Bignell et al., 2010). The discovery of oncogenes has greatly expanded tumor treatment and improved patient survival. However, the mortality rate of cancer is still high, especially for patients who fail to be diagnosed early (Miller et al., 2022; Siegel et al., 2022). In recent years, owing to the application of metabolomics and the discovery of “oncometabolites,” cancer, as a kind of metabolic disorder, has attracted the attention of researchers. This is of great significance in the diagnosis and treatment of cancer. For example, the detection of metabolic abnormalities can indicate early cancer development. Drugs targeting key enzymes involved in abnormal metabolism can also inhibit the progression of some tumors (Wishart, 2015).
Metabolomics studies enable the simultaneous detection of many small-molecule metabolites, thus helping to assess metabolic abnormalities in lung cancer (Noreldeen et al., 2020). It is now clear that there are many metabolic abnormalities in lung cancer, such as abnormal energy metabolism (Vanhove et al., 2019), dysregulation of lipid metabolism (Wang et al., 2022), and glucose metabolism disorder (Ding et al., 2019; Smolle et al., 2020). Current research focuses on the occurrence and development mechanism of abnormal metabolism in lung cancer, its impact on tumor progression, and its clinical application. It is of great significance to promote metabolomic measurement data to provide evidence for early diagnosis and pathological classification of lung cancer. According to the different types and stages of lung cancers with different metabolic abnormalities, research on new targeted drugs and reducing drug resistance during lung cancer treatment has very broad prospects for clinical application.
Author contributions
All authors listed have made a substantial, direct, and intellectual contribution to the work and approved it for publication.
Funding
This study was supported by the Technology Research Funds of Jilin Province (20190303162SF), the Medical and Health Project Funds of Jilin Province (20200708083YY and 2020SCZT019), and the Disciplinary Crossing and Integration and Innovation Cultivation Project of Jilin University (JLUXKJC2020212).
Conflict of interest
The authors declare that the research was conducted in the absence of any commercial or financial relationships that could be construed as a potential conflict of interest.
Publisher’s note
All claims expressed in this article are solely those of the authors and do not necessarily represent those of their affiliated organizations, or those of the publisher, the editors and the reviewers. Any product that may be evaluated in this article, or claim that may be made by its manufacturer, is not guaranteed or endorsed by the publisher.
References
Amelio, I., Cutruzzola, F., Antonov, A., Agostini, M., and Melino, G. (2014). Serine and glycine metabolism in cancer. Trends Biochem. Sci. 39, 191–198. doi:10.1016/j.tibs.2014.02.004
Arlt, B., Zasada, C., Baum, K., Wuenschel, J., Mastrobuoni, G., Lodrini, M., et al. (2021). Inhibiting phosphoglycerate dehydrogenase counteracts chemotherapeutic efficacy against MYCN-amplified neuroblastoma. Int. J. Cancer 148, 1219–1232. doi:10.1002/ijc.33423
B'Chir, W., Maurin, A. C., Carraro, V., Averous, J., Jousse, C., Muranishi, Y., et al. (2013). The eIF2α/ATF4 pathway is essential for stress-induced autophagy gene expression. Nucleic Acids Res. 41, 7683–7699. doi:10.1093/nar/gkt563
Bachelor, M. A., Lu, Y., and Owens, D. M. (2011). L-3-Phosphoserine phosphatase (PSPH) regulates cutaneous squamous cell carcinoma proliferation independent of L-serine biosynthesis. J. dermatological Sci. 63, 164–172. doi:10.1016/j.jdermsci.2011.06.001
Bernhardt, S., Bayerlova, M., Vetter, M., Wachter, A., Mitra, D., Hanf, V., et al. (2017). Proteomic profiling of breast cancer metabolism identifies SHMT2 and ASCT2 as prognostic factors. Breast cancer Res. BCR 19, 112. doi:10.1186/s13058-017-0905-7
Bi, F., An, Y., Sun, T., You, Y., and Yang, Q. (2021). PHGDH is upregulated at translational level and implicated in platin-resistant in ovarian cancer cells. Front. Oncol. 11, 643129. doi:10.3389/fonc.2021.643129
Bignell, G. R., Greenman, C. D., Davies, H., Butler, A. P., Edkins, S., Andrews, J. M., et al. (2010). Signatures of mutation and selection in the cancer genome. Nature 463, 893–898. doi:10.1038/nature08768
Biyik-Sit, R., Kruer, T., Dougherty, S., Bradley, J. A., Wilkey, D. W., Merchant, M. L., et al. (2021). Nuclear pyruvate kinase M2 (PKM2) contributes to phosphoserine aminotransferase 1 (PSAT1)-Mediated cell migration in EGFR-activated lung cancer cells. Cancers (Basel) 13, 3938. doi:10.3390/cancers13163938
Bjelosevic, S., Gruber, E., Newbold, A., Shembrey, C., Devlin, J. R., Hogg, S. J., et al. (2021). Serine biosynthesis is a metabolic vulnerability in FLT3-ITD-driven acute myeloid leukemia. Cancer Discov. 11, 1582–1599. doi:10.1158/2159-8290.Cd-20-0738
Boroughs, L. K., and DeBerardinis, R. J. (2015). Metabolic pathways promoting cancer cell survival and growth. Nat. Cell Biol. 17, 351–359. doi:10.1038/ncb3124
Buqué, A., Galluzzi, L., and Montrose, D. C. (2021). Targeting serine in cancer: Is two better than one? Trends cancer 7, 668–670. doi:10.1016/j.trecan.2021.06.004
Cai, L. Y., Chen, S. J., Xiao, S. H., Sun, Q. J., Ding, C. H., Zheng, B. N., et al. (2021). Targeting p300/CBP attenuates hepatocellular carcinoma progression through epigenetic regulation of metabolism. Cancer Res. 81, 860–872. doi:10.1158/0008-5472.CAN-20-1323
Chan, Y. C., Chang, Y. C., Chuang, H. H., Yang, Y. C., Lin, Y. F., Huang, M. S., et al. (2020). Overexpression of PSAT1 promotes metastasis of lung adenocarcinoma by suppressing the IRF1-IFNγ axis. Oncogene 39, 2509–2522. doi:10.1038/s41388-020-1160-4
Chen, C., Zhu, T., Liu, X., Zhu, D., Zhang, Y., Wu, S., et al. (2022). Identification of a novel PHGDH covalent inhibitor by chemical proteomics and phenotypic profiling. Acta Pharm. Sin. B 12, 246–261. doi:10.1016/j.apsb.2021.06.008
Chen, J., Na, R., Xiao, C., Wang, X., Wang, Y., Yan, D., et al. (2021). The loss of SHMT2 mediates 5-fluorouracil chemoresistance in colorectal cancer by upregulating autophagy. Oncogene 40, 3974–3988. doi:10.1038/s41388-021-01815-4
Chen, P. H., Cai, L., Huffman, K., Yang, C., Kim, J., Faubert, B., et al. (2019). Metabolic diversity in human non-small cell lung cancer cells. Mol. Cell 76, 838–851. e835. doi:10.1016/j.molcel.2019.08.028
Choi, H. J., Jin, S., Cho, H., Won, H. Y., An, H. W., Jeong, G. Y., et al. (2019). CDK12 drives breast tumor initiation and trastuzumab resistance via WNT and IRS1-ErbB-PI3K signaling. EMBO Rep. 20, e48058. doi:10.15252/embr.201948058
Copps, K. D., and White, M. F. (2012). Regulation of insulin sensitivity by serine/threonine phosphorylation of insulin receptor substrate proteins IRS1 and IRS2. Diabetologia 55, 2565–2582. doi:10.1007/s00125-012-2644-8
Davis, S. R., Stacpoole, P. W., Williamson, J., Kick, L. S., Quinlivan, E. P., Coats, B. S., et al. (2004). Tracer-derived total and folate-dependent homocysteine remethylation and synthesis rates in humans indicate that serine is the main one-carbon donor. Am. J. physiology. Endocrinol. metabolism 286, E272–E279. doi:10.1152/ajpendo.00351.2003
Dekhne, A. S., Shah, K., Ducker, G. S., Katinas, J. M., Wong-Roushar, J., Nayeen, M. J., et al. (2019). Novel pyrrolo[3, 2-d]pyrimidine compounds target mitochondrial and cytosolic one-carbon metabolism with broad-spectrum antitumor efficacy. Mol. Cancer Ther. 18, 1787–1799. doi:10.1158/1535-7163.MCT-19-0037
DeNicola, G. M., Chen, P. H., Mullarky, E., Sudderth, J. A., Hu, Z., Wu, D., et al. (2015). NRF2 regulates serine biosynthesis in non-small cell lung cancer. Nat. Genet. 47, 1475–1481. doi:10.1038/ng.3421
Ding, M., Li, F., Wang, B., Chi, G., and Liu, H. (2019). A comprehensive analysis of WGCNA and serum metabolomics manifests the lung cancer-associated disordered glucose metabolism. J. Cell. Biochem. 120, 10855–10863. doi:10.1002/jcb.28377
Ding, R., Hong, W., Huang, L., Shao, J., Yu, W., and Xu, X. (2022). Examination of the effects of microRNA-145-5p and phosphoserine aminotransferase 1 in colon cancer. Bioengineered 13, 12794–12806. doi:10.1080/21655979.2022.2071010
Dong, J. K., Lei, H. M., Liang, Q., Tang, Y. B., Zhou, Y., Wang, Y., et al. (2018). Overcoming erlotinib resistance in EGFR mutation-positive lung adenocarcinomas through repression of phosphoglycerate dehydrogenase. Theranostics 8, 1808–1823. doi:10.7150/thno.23177
Ducker, G. S., Ghergurovich, J. M., Mainolfi, N., Suri, V., Jeong, S. K., Hsin-Jung Li, S., et al. (2017). Human SHMT inhibitors reveal defective glycine import as a targetable metabolic vulnerability of diffuse large B-cell lymphoma. Proc. Natl. Acad. Sci. U. S. A. 114, 11404–11409. doi:10.1073/pnas.1706617114
Ducker, G. S., and Rabinowitz, J. D. (2017). One-carbon metabolism in Health and disease. Cell Metab. 25, 27–42. doi:10.1016/j.cmet.2016.08.009
Fan, J., Ye, J., Kamphorst, J. J., Shlomi, T., Thompson, C. B., and Rabinowitz, J. D. (2014). Quantitative flux analysis reveals folate-dependent NADPH production. Nature 510, 298–302. doi:10.1038/nature13236
Fan, Y., Wang, L., Ding, Y., Sheng, Q., Zhang, C., Li, Y., et al. (2021). Long non-coding RNA RP4-694a7.2 promotes hepatocellular carcinoma cell proliferation and metastasis through the regulation of PSAT1. J. Cancer 12, 5633–5643. doi:10.7150/jca.59348
Fang, Y., Liang, X., Xu, J., and Cai, X. (2018). miR-424 targets AKT3 and PSAT1 and has a tumor-suppressive role in human colorectal cancer. Cancer Manag. Res. 10, 6537–6547. doi:10.2147/cmar.S185789
Feng, M., Cui, H., Tu, W., Li, L., Gao, Y., Chen, L., et al. (2022). An integrated pan-cancer analysis of PSAT1: A potential biomarker for survival and immunotherapy. Front. Genet. 13, 975381. doi:10.3389/fgene.2022.975381
Forman, H. J., Zhang, H., and Rinna, A. (2009). Glutathione: Overview of its protective roles, measurement, and biosynthesis. Mol. aspects Med. 30, 1–12. doi:10.1016/j.mam.2008.08.006
Fujii, K., Miyata, Y., Takahashi, I., Koizumi, H., Saji, H., Hoshikawa, M., et al. (2018). Differential proteomic analysis between small cell lung carcinoma (SCLC) and pulmonary carcinoid tumors reveals molecular signatures for malignancy in lung cancer. Proteomics Clin. Appl. 12, e1800015. doi:10.1002/prca.201800015
Gantner, M. L., Eade, K., Wallace, M., Handzlik, M. K., Fallon, R., Trombley, J., et al. (2019). Serine and lipid metabolism in macular disease and peripheral neuropathy. N. Engl. J. Med. 381, 1422–1433. doi:10.1056/NEJMoa1815111
Gao, S., Ge, A., Xu, S., You, Z., Ning, S., Zhao, Y., et al. (2017). PSAT1 is regulated by ATF4 and enhances cell proliferation via the GSK3β/β-catenin/cyclin D1 signaling pathway in ER-negative breast cancer. J. Exp. Clin. cancer Res. CR 36, 179. doi:10.1186/s13046-017-0648-4
García-Cañaveras, J. C., Lancho, O., Ducker, G. S., Ghergurovich, J. M., Xu, X., da Silva-Diz, V., et al. (2021). SHMT inhibition is effective and synergizes with methotrexate in T-cell acute lymphoblastic leukemia. Leukemia 35, 377–388. doi:10.1038/s41375-020-0845-6
Geeraerts, S. L., Kampen, K. R., Rinaldi, G., Gupta, P., Planque, M., Louros, N., et al. (2021). Repurposing the antidepressant sertraline as SHMT inhibitor to suppress serine/Glycine synthesis-addicted breast tumor growth. Mol. Cancer Ther. 20, 50–63. doi:10.1158/1535-7163.Mct-20-0480
Giardina, G., Paone, A., Tramonti, A., Lucchi, R., Marani, M., Magnifico, M. C., et al. (2018). The catalytic activity of serine hydroxymethyltransferase is essential for de novo nuclear dTMP synthesis in lung cancer cells. FEBS J. 285, 3238–3253. doi:10.1111/febs.14610
Guiducci, G., Paone, A., Tramonti, A., Giardina, G., Rinaldo, S., Bouzidi, A., et al. (2019). The moonlighting RNA-binding activity of cytosolic serine hydroxymethyltransferase contributes to control compartmentalization of serine metabolism. Nucleic Acids Res. 47, 4240–4254. doi:10.1093/nar/gkz129
Guo, J., Gu, X., Zheng, M., Zhang, Y., Chen, L., and Li, H. (2019). Azacoccone E inhibits cancer cell growth by targeting 3-phosphoglycerate dehydrogenase. Bioorg. Chem. 87, 16–22. doi:10.1016/j.bioorg.2019.02.037
Guo, K., Qi, D., and Huang, B. (2021). LncRNA MEG8 promotes NSCLC progression by modulating the miR-15a-5p-miR-15b-5p/PSAT1 axis. Cancer Cell Int. 21, 84. doi:10.1186/s12935-021-01772-8
Gupta, R., Yang, Q., Dogra, S. K., and Wajapeyee, N. (2017). Serine hydroxymethyl transferase 1 stimulates pro-oncogenic cytokine expression through sialic acid to promote ovarian cancer tumor growth and progression. Oncogene 36, 4014–4024. doi:10.1038/onc.2017.37
Hanahan, D., and Weinberg, R. A. (2011). Hallmarks of cancer: The next generation. Cell 144, 646–674. doi:10.1016/j.cell.2011.02.013
Hao, Y., Zhao, S., and Wang, Z. (2014). Targeting the protein-protein interaction between IRS1 and mutant p110α for cancer therapy. Toxicol. Pathol. 42, 140–147. doi:10.1177/0192623313506794
Hirabayashi, Y., and Furuya, S. (2008). Roles of l-serine and sphingolipid synthesis in brain development and neuronal survival. Prog. Lipid Res. 47, 188–203. doi:10.1016/j.plipres.2008.01.003
Huang, M. Y., Liu, X. Y., Shao, Q., Zhang, X., Miao, L., Wu, X. Y., et al. (2022). Phosphoserine phosphatase as a prognostic biomarker in patients with gastric cancer and its potential association with immune cells. BMC Gastroenterol. 22, 1. doi:10.1186/s12876-021-02073-0
Itoyama, R., Yasuda-Yoshihara, N., Kitamura, F., Yasuda, T., Bu, L., Yonemura, A., et al. (2021). Metabolic shift to serine biosynthesis through 3-PG accumulation and PHGDH induction promotes tumor growth in pancreatic cancer. Cancer Lett. 523, 29–42. doi:10.1016/j.canlet.2021.09.007
Jiang, J., Zhang, L., Chen, H., Lei, Y., Zhang, T., Wang, Y., et al. (2020). Regorafenib induces lethal autophagy arrest by stabilizing PSAT1 in glioblastoma. Autophagy 16, 106–122. doi:10.1080/15548627.2019.1598752
Jing, Z., Heng, W., Xia, L., Ning, W., Yafei, Q., Yao, Z., et al. (2015). Downregulation of phosphoglycerate dehydrogenase inhibits proliferation and enhances cisplatin sensitivity in cervical adenocarcinoma cells by regulating Bcl-2 and caspase-3. Cancer Biol. Ther. 16, 541–548. doi:10.1080/15384047.2015.1017690
Jovov, B., Araujo-Perez, F., Sigel, C. S., Stratford, J. K., McCoy, A. N., Yeh, J. J., et al. (2012). Differential gene expression between African American and European American colorectal cancer patients. PloS one 7, e30168. doi:10.1371/journal.pone.0030168
Kampen, K. R., Fancello, L., Girardi, T., Rinaldi, G., Planque, M., Sulima, S. O., et al. (2019). Translatome analysis reveals altered serine and glycine metabolism in T-cell acute lymphoblastic leukemia cells. Nat. Commun. 10, 2542. doi:10.1038/s41467-019-10508-2
Kim, Y. J., Jang, S. K., Hong, S. E., Park, C. S., Seong, M. K., Kim, H. A., et al. (2022). Knockdown of YAP/TAZ sensitizes tamoxifen-resistant MCF7 breast cancer cells. Biochem. biophysical Res. Commun. 601, 73–78. doi:10.1016/j.bbrc.2022.02.083
Koseki, J., Konno, M., Asai, A., Colvin, H., Kawamoto, K., Nishida, N., et al. (2018). Enzymes of the one-carbon folate metabolism as anticancer targets predicted by survival rate analysis. Sci. Rep. 8, 303. doi:10.1038/s41598-017-18456-x
Kurniawan, H., Franchina, D. G., Guerra, L., Bonetti, L., -Baguet, L. S., Grusdat, M., et al. (2020). Glutathione restricts serine metabolism to preserve regulatory T cell function. Cell Metab. 31, 920–936. e927. doi:10.1016/j.cmet.2020.03.004
Labuschagne, C. F., van den Broek, N. J., Mackay, G. M., Vousden, K. H., and Maddocks, O. D. (2014). Serine, but not glycine, supports one-carbon metabolism and proliferation of cancer cells. Cell Rep. 7, 1248–1258. doi:10.1016/j.celrep.2014.04.045
Lee, G. Y., Haverty, P. M., Li, L., Kljavin, N. M., Bourgon, R., Lee, J., et al. (2014). Comparative oncogenomics identifies PSMB4 and SHMT2 as potential cancer driver genes. Cancer Res. 74, 3114–3126. doi:10.1158/0008-5472.Can-13-2683
Lee, W. D., Pirona, A. C., Sarvin, B., Stern, A., Nevo-Dinur, K., Besser, E., et al. (2021). Tumor reliance on cytosolic versus mitochondrial one-carbon flux depends on folate availability. Cell Metab. 33, 190–198.e6. doi:10.1016/j.cmet.2020.12.002
Li, A. M., and Ye, J. (2020). Reprogramming of serine, glycine and one-carbon metabolism in cancer. Biochimica biophysica acta. Mol. basis Dis. 1866, 165841. doi:10.1016/j.bbadis.2020.165841
Li, M. K., Liu, L. X., Zhang, W. Y., Zhan, H. L., Chen, R. P., Feng, J. L., et al. (2020). Long non‑coding RNA MEG3 suppresses epithelial‑to‑mesenchymal transition by inhibiting the PSAT1‑dependent GSK‑3β/Snail signaling pathway in esophageal squamous cell carcinoma. Oncol. Rep. 44, 2130–2142. doi:10.3892/or.2020.7754
Li, M., Wu, C., Yang, Y., Zheng, M., Yu, S., Wang, J., et al. (2021). 3-Phosphoglycerate dehydrogenase: A potential target for cancer treatment. Cell. Oncol. Dordr. 44, 541–556. doi:10.1007/s13402-021-00599-9
Li, X., Gracilla, D., Cai, L., Zhang, M., Yu, X., Chen, X., et al. (2021). ATF3 promotes the serine synthesis pathway and tumor growth under dietary serine restriction. Cell Rep. 36, 109706. doi:10.1016/j.celrep.2021.109706
Li, X., Xun, Z., and Yang, Y. (2016). Inhibition of phosphoserine phosphatase enhances the anticancer efficacy of 5-fluorouracil in colorectal cancer. Biochem. biophysical Res. Commun. 477, 633–639. doi:10.1016/j.bbrc.2016.06.112
Li, X., Zhang, K., Hu, Y., and Luo, N. (2020). ERRα activates SHMT2 transcription to enhance the resistance of breast cancer to lapatinib via modulating the mitochondrial metabolic adaption. Biosci. Rep. 40, BSR20192465. doi:10.1042/bsr20192465
Li, Y., Maher, P., and Schubert, D. (1997). A role for 12-lipoxygenase in nerve cell death caused by glutathione depletion. Neuron 19, 453–463. doi:10.1016/s0896-6273(00)80953-8
Li, Z., and Zhang, H. (2016). Reprogramming of glucose, fatty acid and amino acid metabolism for cancer progression. Cell. Mol. life Sci. CMLS 73, 377–392. doi:10.1007/s00018-015-2070-4
Liao, L., Ge, M., Zhan, Q., Huang, R., Ji, X., Liang, X., et al. (2019). PSPH mediates the metastasis and proliferation of non-small cell lung cancer through MAPK signaling pathways. Int. J. Biol. Sci. 15, 183–194. doi:10.7150/ijbs.29203
Liu, B., Jia, Y., Cao, Y., Wu, S., Jiang, H., Sun, X., et al. (2016). Overexpression of phosphoserine aminotransferase 1 (PSAT1) predicts poor prognosis and associates with tumor progression in human esophageal squamous cell carcinoma. Cell Physiol. Biochem. 39, 395–406. doi:10.1159/000445633
Liu, C., Wang, L., Liu, X., Tan, Y., Tao, L., Xiao, Y., et al. (2021). Cytoplasmic SHMT2 drives the progression and metastasis of colorectal cancer by inhibiting β-catenin degradation. Theranostics 11, 2966–2986. doi:10.7150/thno.48699
Liu, J., Zhang, C., Wu, H., Sun, X. X., Li, Y., Huang, S., et al. (2020). Parkin ubiquitinates phosphoglycerate dehydrogenase to suppress serine synthesis and tumor progression. J. Clin. Invest. 130, 3253–3269. doi:10.1172/JCI132876
Liu, Y., Yin, C., Deng, M. M., Wang, Q., He, X. Q., Li, M. T., et al. (2019). High expression of SHMT2 is correlated with tumor progression and predicts poor prognosis in gastrointestinal tumors. Eur. Rev. Med. Pharmacol. Sci. 23, 9379–9392. doi:10.26355/eurrev_201911_19431
Locasale, J. W. (2013). Serine, glycine and one-carbon units: Cancer metabolism in full circle. Nat. Rev. Cancer 13, 572–583. doi:10.1038/nrc3557
Lunt, S. Y., and Vander Heiden, M. G. (2011). Aerobic glycolysis: Meeting the metabolic requirements of cell proliferation. Annu. Rev. Cell Dev. Biol. 27, 441–464. doi:10.1146/annurev-cellbio-092910-154237
Luo, L., Zheng, Y., Lin, Z., Li, X., Li, X., Li, M., et al. (2021). Identification of SHMT2 as a potential prognostic biomarker and correlating with immune infiltrates in lung adenocarcinoma. J. Immunol. Res. 2021, 6647122. doi:10.1155/2021/6647122
Luo, M. Y., Zhou, Y., Gu, W. M., Wang, C., Shen, N. X., Dong, J. K., et al. (2022). Metabolic and nonmetabolic functions of PSAT1 coordinate signaling cascades to confer EGFR inhibitor resistance and drive progression in lung adenocarcinoma. Cancer Res. 82, 3516–3531. doi:10.1158/0008-5472.Can-21-4074
Ma, C., Zheng, K., Jiang, K., Zhao, Q., Sha, N., Wang, W., et al. (2021). The alternative activity of nuclear PHGDH contributes to tumour growth under nutrient stress. Nat. Metab. 3, 1357–1371. doi:10.1038/s42255-021-00456-x
Ma, X., Li, B., Liu, J., Fu, Y., and Luo, Y. (2019). Phosphoglycerate dehydrogenase promotes pancreatic cancer development by interacting with eIF4A1 and eIF4E. J. Exp. Clin. cancer Res. CR 38, 66. doi:10.1186/s13046-019-1053-y
Macfarlane, A. J., Perry, C. A., McEntee, M. F., Lin, D. M., and Stover, P. J. (2011). Shmt1 heterozygosity impairs folate-dependent thymidylate synthesis capacity and modifies risk of Apc(min)-mediated intestinal cancer risk. Cancer Res. 71, 2098–2107. doi:10.1158/0008-5472.Can-10-1886
Maddocks, O. D., Berkers, C. R., Mason, S. M., Zheng, L., Blyth, K., Gottlieb, E., et al. (2013). Serine starvation induces stress and p53-dependent metabolic remodelling in cancer cells. Nature 493, 542–546. doi:10.1038/nature11743
Mahajan, U. V., Varma, V. R., Griswold, M. E., Blackshear, C. T., An, Y., Oommen, A. M., et al. (2020). Dysregulation of multiple metabolic networks related to brain transmethylation and polyamine pathways in alzheimer disease: A targeted metabolomic and transcriptomic study. PLoS Med. 17, e1003012. doi:10.1371/journal.pmed.1003012
Mandal, P. K., Saharan, S., Tripathi, M., and Murari, G. (2015). Brain glutathione levels--a novel biomarker for mild cognitive impairment and Alzheimer's disease. Biol. psychiatry 78, 702–710. doi:10.1016/j.biopsych.2015.04.005
Martínez-Reyes, I., and Chandel, N. S. (2021). Cancer metabolism: Looking forward. Nat. Rev. Cancer 21, 669–680. doi:10.1038/s41568-021-00378-6
Mattaini, K. R., Sullivan, M. R., and Vander Heiden, M. G. (2016). The importance of serine metabolism in cancer. J. Cell Biol. 214, 249–257. doi:10.1083/jcb.201604085
Metcalf, S., Dougherty, S., Kruer, T., Hasan, N., Biyik-Sit, R., Reynolds, L., et al. (2020). Selective loss of phosphoserine aminotransferase 1 (PSAT1) suppresses migration, invasion, and experimental metastasis in triple negative breast cancer. Clin. Exp. metastasis 37, 187–197. doi:10.1007/s10585-019-10000-7
Miller, K. D., Nogueira, L., Devasia, T., Mariotto, A. B., Yabroff, K. R., Jemal, A., et al. (2022). Cancer treatment and survivorship statistics. CA a cancer J. Clin. 72, 409–436. doi:10.3322/caac.21731
Mohamed, A., Sun, C., De Mello, V., Selfe, J., Missiaglia, E., Shipley, J., et al. (2016). The Hippo effector TAZ (WWTR1) transforms myoblasts and TAZ abundance is associated with reduced survival in embryonal rhabdomyosarcoma. J. pathology 240, 3–14. doi:10.1002/path.4745
Montrose, D. C., Saha, S., Foronda, M., McNally, E. M., Chen, J., Zhou, X. K., et al. (2021). Exogenous and endogenous sources of serine contribute to colon cancer metabolism, growth, and resistance to 5-fluorouracil. Cancer Res. 81, 2275–2288. doi:10.1158/0008-5472.CAN-20-1541
Morrissey, S. M., Zhang, F., Ding, C., Montoya-Durango, D. E., Hu, X., Yang, C., et al. (2021). Tumor-derived exosomes drive immunosuppressive macrophages in a pre-metastatic niche through glycolytic dominant metabolic reprogramming. Cell Metab. 33, 20402040–20402058.e10. doi:10.1016/j.cmet.2021.09.002
Mullarky, E., Lucki, N. C., Beheshti Zavareh, R., Anglin, J. L., Gomes, A. P., Nicolay, B. N., et al. (2016). Identification of a small molecule inhibitor of 3-phosphoglycerate dehydrogenase to target serine biosynthesis in cancers. Proc. Natl. Acad. Sci. U. S. A. 113, 1778–1783. doi:10.1073/pnas.1521548113
Newman, A. C., and Maddocks, O. D. K. (2017). One-carbon metabolism in cancer. Br. J. Cancer 116, 1499–1504. doi:10.1038/bjc.2017.118
Noreldeen, H. A. A., Liu, X., and Xu, G. (2020). Metabolomics of lung cancer: Analytical platforms and their applications. J. Sep. Sci. 43, 120–133. doi:10.1002/jssc.201900736
Pacold, M. E., Brimacombe, K. R., Chan, S. H., Rohde, J. M., Lewis, C. A., Swier, L. J. Y. M., et al. (2016). A PHGDH inhibitor reveals coordination of serine synthesis and one-carbon unit fate. Nat. Chem. Biol. 12, 452–458. doi:10.1038/nchembio.2070
Pandey, S., Garg, P., Lee, S., Choung, H. W., Choung, Y. H., Choung, P. H., et al. (2014). Nucleotide biosynthesis arrest by silencing SHMT1 function via vitamin B6-coupled vector and effects on tumor growth inhibition. Biomaterials 35, 9332–9342. doi:10.1016/j.biomaterials.2014.07.045
Paone, A., Marani, M., Fiascarelli, A., Rinaldo, S., Giardina, G., Contestabile, R., et al. (2014). SHMT1 knockdown induces apoptosis in lung cancer cells by causing uracil misincorporation. Cell death Dis. 5, e1525. doi:10.1038/cddis.2014.482
Parada, H., Sun, X., Fleming, J. M., Williams-DeVane, C. R., Kirk, E. L., Olsson, L. T., et al. (2017). Race-associated biological differences among luminal A and basal-like breast cancers in the Carolina Breast Cancer Study. Breast cancer Res. BCR 19, 131. doi:10.1186/s13058-017-0914-6
Park, S. M., Seo, E. H., Bae, D. H., Kim, S. S., Kim, J., Lin, W., et al. (2019). Phosphoserine phosphatase promotes lung cancer progression through the dephosphorylation of IRS-1 and a noncanonical L-serine-independent pathway. Mol. Cells 42, 604–616. doi:10.14348/molcells.2019.0160
Parsa, S., Ortega-Molina, A., Ying, H. Y., Jiang, M., Teater, M., Wang, J., et al. (2020). The serine hydroxymethyltransferase-2 (SHMT2) initiates lymphoma development through epigenetic tumor suppressor silencing. Nat. cancer 1, 653–664. doi:10.1038/s43018-020-0080-0
Pathria, G., Scott, D. A., Feng, Y., Sang Lee, J., Fujita, Y., Zhang, G., et al. (2018). Targeting the Warburg effect via LDHA inhibition engages ATF4 signaling for cancer cell survival. EMBO J. 37, e99735. doi:10.15252/embj.201899735
Pavlova, N. N., and Thompson, C. B. (2016). The emerging hallmarks of cancer metabolism. Cell Metab. 23, 27–47. doi:10.1016/j.cmet.2015.12.006
Pikman, Y., Ocasio-Martinez, N., Alexe, G., Dimitrov, B., Kitara, S., Diehl, F. F., et al. (2022). Targeting serine hydroxymethyltransferases 1 and 2 for T-cell acute lymphoblastic leukemia therapy. Leukemia 36, 348–360. doi:10.1038/s41375-021-01361-8
Possemato, R., Marks, K. M., Shaul, Y. D., Pacold, M. E., Kim, D., Birsoy, K., et al. (2011). Functional genomics reveal that the serine synthesis pathway is essential in breast cancer. Nature 476, 346–350. doi:10.1038/nature10350
Qian, C., Xia, Y., Ren, Y., Yin, Y., and Deng, A. (2017). Identification and validation of PSAT1 as a potential prognostic factor for predicting clinical outcomes in patients with colorectal carcinoma. Oncol. Lett. 14, 8014–8020. doi:10.3892/ol.2017.7211
Rawat, V., Malvi, P., Della Manna, D., Yang, E. S., Bugide, S., Zhang, X., et al. (2021). PSPH promotes melanoma growth and metastasis by metabolic deregulation-mediated transcriptional activation of NR4A1. Oncogene 40, 2448–2462. doi:10.1038/s41388-021-01683-y
Reid, M. A., Allen, A. E., Liu, S., Liberti, M. V., Liu, P., Liu, X., et al. (2018). Serine synthesis through PHGDH coordinates nucleotide levels by maintaining central carbon metabolism. Nat. Commun. 9, 5442. doi:10.1038/s41467-018-07868-6
Reina-Campos, M., Diaz-Meco, M. T., and Moscat, J. (2020). The complexity of the serine glycine one-carbon pathway in cancer. J. Cell Biol. 219, e201907022. doi:10.1083/jcb.201907022
Rinaldi, G., Pranzini, E., Van Elsen, J., Broekaert, D., Funk, C. M., Planque, M., et al. (2021). In vivo evidence for serine biosynthesis-defined sensitivity of lung metastasis, but not of primary breast tumors, to mTORC1 inhibition. Mol. Cell 81, 386–397.e7. doi:10.1016/j.molcel.2020.11.027
Rodriguez, A. E., Ducker, G. S., Billingham, L. K., Martinez, C. A., Mainolfi, N., Suri, V., et al. (2019). Serine metabolism supports macrophage IL-1β production. Cell Metab. 29, 1003–1011. e1004. doi:10.1016/j.cmet.2019.01.014
Ross, K. C., Andrews, A. J., Marion, C. D., Yen, T. J., and Bhattacharjee, V. (2017). Identification of the serine biosynthesis pathway as a critical component of BRAF inhibitor resistance of melanoma, pancreatic, and non-small cell lung cancer cells. Mol. Cancer Ther. 16, 1596–1609. doi:10.1158/1535-7163.Mct-16-0798
Rossi, M., Altea-Manzano, P., Demicco, M., Doglioni, G., Bornes, L., Fukano, M., et al. (2022). PHGDH heterogeneity potentiates cancer cell dissemination and metastasis. Nature 605, 747–753. doi:10.1038/s41586-022-04758-2
Sato, K., Masuda, T., Hu, Q., Tobo, T., Kidogami, S., Ogawa, Y., et al. (2017). Phosphoserine phosphatase is a novel prognostic biomarker on chromosome 7 in colorectal cancer. Anticancer Res. 37, 2365–2371. doi:10.21873/anticanres.11574
Scaletti, E., Jemth, A. S., Helleday, T., and Stenmark, P. (2019). Structural basis of inhibition of the human serine hydroxymethyltransferase SHMT2 by antifolate drugs. FEBS Lett. 593, 1863–1873. doi:10.1002/1873-3468.13455
Sekhar, R. V., McKay, S. V., Patel, S. G., Guthikonda, A. P., Reddy, V. T., Balasubramanyam, A., et al. (2011). Glutathione synthesis is diminished in patients with uncontrolled diabetes and restored by dietary supplementation with cysteine and glycine. Diabetes care 34, 162–167. doi:10.2337/dc10-1006
Shetty, S., and Varshney, U. (2021). Regulation of translation by one-carbon metabolism in bacteria and eukaryotic organelles. J. Biol. Chem. 296, 100088. doi:10.1074/jbc.REV120.011985
Siegel, R. L., Miller, K. D., Fuchs, H. E., and Jemal, A. (2022). Cancer statistics, 2022. CA a cancer J. Clin. 72, 7–33. doi:10.3322/caac.21708
Skibola, C. F., Smith, M. T., Hubbard, A., Shane, B., Roberts, A. C., Law, G. R., et al. (2002). Polymorphisms in the thymidylate synthase and serine hydroxymethyltransferase genes and risk of adult acute lymphocytic leukemia. Blood 99, 3786–3791. doi:10.1182/blood.v99.10.3786
Smolle, E., Leko, P., Stacher-Priehse, E., Brcic, L., El-Heliebi, A., Hofmann, L., et al. (2020). Distribution and prognostic significance of gluconeogenesis and glycolysis in lung cancer. Mol. Oncol. 14, 2853–2867. doi:10.1002/1878-0261.12780
Song, Z., Feng, C., Lu, Y., Lin, Y., and Dong, C. (2018). PHGDH is an independent prognosis marker and contributes cell proliferation, migration and invasion in human pancreatic cancer. Gene 642, 43–50. doi:10.1016/j.gene.2017.11.014
Strunck, E., Frank, K., Tan, M. I., and Vollmer, G. (2001). Expression of l-3-phosphoserine phosphatase is regulated by reconstituted basement membrane. Biochem. biophysical Res. Commun. 281, 747–753. doi:10.1006/bbrc.2001.4403
Sun, S., Li, C., Cui, K., Liu, B., Zhou, M., Cao, Y., et al. (2021). Hsa_circ_0062682 promotes serine metabolism and tumor growth in colorectal cancer by regulating the miR-940/PHGDH Axis. Front. Cell Dev. Biol. 9, 770006. doi:10.3389/fcell.2021.770006
Tajan, M., Hennequart, M., Cheung, E. C., Zani, F., Hock, A. K., Legrave, N., et al. (2021). Serine synthesis pathway inhibition cooperates with dietary serine and glycine limitation for cancer therapy. Nat. Commun. 12, 366. doi:10.1038/s41467-020-20223-y
Tan, Y., Zhou, X., Gong, Y., Gou, K., Luo, Y., Jia, D., et al. (2021). Biophysical and biochemical properties of PHGDH revealed by studies on PHGDH inhibitors. Cell. Mol. life Sci. CMLS 79, 27. doi:10.1007/s00018-021-04022-2
Tchakarska, G., and Sola, B. (2020). The double dealing of cyclin D1. Cell cycle 19, 163–178. doi:10.1080/15384101.2019.1706903
Toyama, A., Suzuki, A., Shimada, T., Aoki, C., Aoki, Y., Umino, Y., et al. (2012). Proteomic characterization of ovarian cancers identifying annexin-A4, phosphoserine aminotransferase, cellular retinoic acid-binding protein 2, and serpin B5 as histology-specific biomarkers. Cancer Sci. 103, 747–755. doi:10.1111/j.1349-7006.2012.02224.x
Tramonti, A., Cuyas, E., Encinar, J. A., Pietzke, M., Paone, A., Verdura, S., et al. (2021). Metformin is a pyridoxal-5'-phosphate (PLP)-Competitive inhibitor of SHMT2. Cancers (Basel) 13, 4009. doi:10.3390/cancers13164009
Tramonti, A., Nardella, C., di Salvo, M. L., Barile, A., Cutruzzola, F., and Contestabile, R. (2018). Human cytosolic and mitochondrial serine hydroxymethyltransferase isoforms in comparison: Full kinetic characterization and substrate inhibition properties. Biochemistry 57, 6984–6996. doi:10.1021/acs.biochem.8b01074
Traverso, N., Ricciarelli, R., Nitti, M., Marengo, B., Furfaro, A. L., Pronzato, M. A., et al. (2013). Role of glutathione in cancer progression and chemoresistance. Oxidative Med. Cell. Longev. 2013, 972913. doi:10.1155/2013/972913
Usman, M., Hameed, Y., Ahmad, M., Iqbal, M. J., Maryam, A., Mazhar, A., et al. (2022). SHMT2 is associated with tumor purity, CD8+ T immune cells infiltration, and a novel therapeutic target in four different human cancers. Curr. Mol. Med. 23, 161–176. doi:10.2174/1566524022666220112142409
Vander Heiden, M. G., Cantley, L. C., and Thompson, C. B. (2009). Understanding the Warburg effect: The metabolic requirements of cell proliferation. Science 324, 1029–1033. doi:10.1126/science.1160809
Vanhove, K., Derveaux, E., Graulus, G. J., Mesotten, L., Thomeer, M., Noben, J. P., et al. (2019). Glutamine addiction and therapeutic strategies in lung cancer. Int. J. Mol. Sci. 20, 252. doi:10.3390/ijms20020252
Vié, N., Copois, V., Bascoul-Mollevi, C., Denis, V., Bec, N., Robert, B., et al. (2008). Overexpression of phosphoserine aminotransferase PSAT1 stimulates cell growth and increases chemoresistance of colon cancer cells. Mol. cancer 7, 14. doi:10.1186/1476-4598-7-14
Wang, G., Qiu, M., Xing, X., Zhou, J., Yao, H., Li, M., et al. (2022). Lung cancer scRNA-seq and lipidomics reveal aberrant lipid metabolism for early-stage diagnosis. Sci. Transl. Med. 14, eabk2756. doi:10.1126/scitranslmed.abk2756
Wang, H., Chong, T., Li, B. Y., Chen, X. S., and Zhen, W. B. (2020). Evaluating the clinical significance of SHMT2 and its co-expressed gene in human kidney cancer. Biol. Res. 53, 46. doi:10.1186/s40659-020-00314-2
Wang, Q., Liberti, M. V., Liu, P., Deng, X., Liu, Y., Locasale, J. W., et al. (2017). Rational design of selective allosteric inhibitors of PHGDH and serine synthesis with anti-tumor activity. Cell Chem. Biol. 24, 55–65. doi:10.1016/j.chembiol.2016.11.013
Warburg, O. (1956). On the origin of cancer cells. Science 123, 309–314. doi:10.1126/science.123.3191.309
Warburg, O., Wind, F., and Negelein, E. (1927). The metabolism of tumors in the body. J. general physiology 8, 519–530. doi:10.1085/jgp.8.6.519
Wei, L., Lee, D., Law, C. T., Zhang, M. S., Shen, J., Chin, D. W. C., et al. (2019). Genome-wide CRISPR/Cas9 library screening identified PHGDH as a critical driver for Sorafenib resistance in HCC. Nat. Commun. 10, 4681. doi:10.1038/s41467-019-12606-7
Weinstabl, H., Treu, M., Rinnenthal, J., Zahn, S. K., Ettmayer, P., Bader, G., et al. (2019). Intracellular trapping of the selective phosphoglycerate dehydrogenase (PHGDH) inhibitor BI-4924 disrupts serine biosynthesis. J. Med. Chem. 62, 7976–7997. doi:10.1021/acs.jmedchem.9b00718
Wilke, A. C., Doebele, C., Zindel, A., Lee, K. S., Rieke, S. A., Ceribelli, M., et al. (2022). SHMT2 inhibition disrupts the TCF3 transcriptional survival program in Burkitt lymphoma. Blood 139, 538–553. doi:10.1182/blood.2021012081
Wishart, D. S. (2015). Is cancer a genetic disease or a metabolic disease? EBioMedicine 2, 478–479. doi:10.1016/j.ebiom.2015.05.022
Wortel, I. M. N., van der Meer, L. T., Kilberg, M. S., and van Leeuwen, F. N. (2017). Surviving stress: Modulation of ATF4-mediated stress responses in normal and malignant cells. Trends Endocrinol. Metab. 28, 794–806. doi:10.1016/j.tem.2017.07.003
Xu, R., Jones, W., Wilcz-Villega, E., Costa, A. S., Rajeeve, V., Bentham, R. B., et al. (2020). The breast cancer oncogene IKKε coordinates mitochondrial function and serine metabolism. EMBO Rep. 21, e48260. doi:10.15252/embr.201948260
Yan, S., Jiang, H., Fang, S., Yin, F., Wang, Z., Jia, Y., et al. (2015). MicroRNA-340 inhibits esophageal cancer cell growth and invasion by targeting phosphoserine aminotransferase 1. Cell Physiol. Biochem. 37, 375–386. doi:10.1159/000430361
Yang, C. S., Stampouloglou, E., Kingston, N. M., Zhang, L., Monti, S., and Varelas, X. (2018). Glutamine-utilizing transaminases are a metabolic vulnerability of TAZ/YAP-activated cancer cells. EMBO Rep. 19, e43577. doi:10.15252/embr.201643577
Yang, X., Wang, Z., Li, X., Liu, B., Liu, M., Liu, L., et al. (2018). SHMT2 desuccinylation by SIRT5 drives cancer cell proliferation. Cancer Res. 78, 372–386. doi:10.1158/0008-5472.Can-17-1912
Yang, Y., Wu, J., Cai, J., He, Z., Yuan, J., Zhu, X., et al. (2015). PSAT1 regulates cyclin D1 degradation and sustains proliferation of non-small cell lung cancer cells. Int. J. Cancer 136, E39–E50. doi:10.1002/ijc.29150
Yoshida, G. J. (2015). Metabolic reprogramming: The emerging concept and associated therapeutic strategies. J. Exp. Clin. cancer Res. CR 34, 111. doi:10.1186/s13046-015-0221-y
Yoshino, H., Enokida, H., Osako, Y., Nohata, N., Yonemori, M., Sugita, S., et al. (2020). Characterization of PHGDH expression in bladder cancer: Potential targeting therapy with gemcitabine/cisplatin and the contribution of promoter DNA hypomethylation. Mol. Oncol. 14, 2190–2202. doi:10.1002/1878-0261.12697
Zhang, B., Zheng, A., Hydbring, P., Ambroise, G., Ouchida, A. T., Goiny, M., et al. (2017). PHGDH defines a metabolic subtype in lung adenocarcinomas with poor prognosis. Cell Rep. 19, 2289–2303. doi:10.1016/j.celrep.2017.05.067
Zhang, H., Che, Y., Xuan, B., Wu, X., and Li, H. (2022). Serine hydroxymethyltransferase 2 (SHMT2) potentiates the aggressive process of oral squamous cell carcinoma by binding to interleukin enhancer-binding factor 2 (ILF2). Bioengineered 13, 8785–8797. doi:10.1080/21655979.2022.2051886
Zhang, J., Wang, E., Zhang, L., and Zhou, B. (2021). PSPH induces cell autophagy and promotes cell proliferation and invasion in the hepatocellular carcinoma cell line Huh7 via the AMPK/mTOR/ULK1 signaling pathway. Cell Biol. Int. 45, 305–319. doi:10.1002/cbin.11489
Zhang, P., and Yang, Q. (2021). Overexpression of SHMT2 predicts a poor prognosis and promotes tumor cell growth in bladder cancer. Front. Genet. 12, 682856. doi:10.3389/fgene.2021.682856
Zhang, Y., Li, J., Dong, X., Meng, D., Zhi, X., Yuan, L., et al. (2020). PSAT1 regulated oxidation-reduction balance affects the growth and prognosis of epithelial ovarian cancer. Onco Targets Ther. 13, 5443–5453. doi:10.2147/OTT.S250066
Zhang, Y., Liu, Z., Wang, X., Jian, H., Xiao, H., and Wen, T. (2022). SHMT2 promotes cell viability and inhibits ROS-dependent, mitochondrial-mediated apoptosis via the intrinsic signaling pathway in bladder cancer cells. Cancer gene Ther. 29, 1514–1527. doi:10.1038/s41417-022-00470-5
Zhang, Y., Yu, H., Zhang, J., Gao, H., Wang, S., Li, S., et al. (2021). Cul4A-DDB1-mediated monoubiquitination of phosphoglycerate dehydrogenase promotes colorectal cancer metastasis via increased S-adenosylmethionine. J. Clin. Invest. 131, e146187. doi:10.1172/jci146187
Zhao, E., Ding, J., Xia, Y., Liu, M., Ye, B., Choi, J. H., et al. (2016). KDM4C and ATF4 cooperate in transcriptional control of amino acid metabolism. Cell Rep. 14, 506–519. doi:10.1016/j.celrep.2015.12.053
Zhao, X., Fu, J., Tang, W., Yu, L., and Xu, W. (2020). Inhibition of serine metabolism promotes resistance to cisplatin in gastric cancer. Onco Targets Ther. 13, 4833–4842. doi:10.2147/ott.S246430
Zheng, M., Guo, J., Xu, J., Yang, K., Tang, R., Gu, X., et al. (2019). Ixocarpalactone A from dietary tomatillo inhibits pancreatic cancer growth by targeting PHGDH. Food & Funct. 10, 3386–3395. doi:10.1039/c9fo00394k
Zheng, M. J., Li, X., Hu, Y. X., Dong, H., Gou, R., Nie, X., et al. (2019). Identification of molecular marker associated with ovarian cancer prognosis using bioinformatics analysis and experiments. J. Cell. physiology 234, 11023–11036. doi:10.1002/jcp.27926
Zhu, J., Berisa, M., Schworer, S., Qin, W., Cross, J. R., and Thompson, C. B. (2019). Transsulfuration activity can support cell growth upon extracellular cysteine limitation. Cell Metab. 30, 865–876. e865. doi:10.1016/j.cmet.2019.09.009
Zhu, J., Ma, J., Wang, X., Ma, T., Zhang, S., Wang, W., et al. (2016). High expression of PHGDH predicts poor prognosis in non-small cell lung cancer. Transl. Oncol. 9, 592–599. doi:10.1016/j.tranon.2016.08.003
Keywords: serine metabolism, lung cancer, PHGDH, PSAT1, PSPH
Citation: Zhou X, Tian C, Cao Y, Zhao M and Wang K (2023) The role of serine metabolism in lung cancer: From oncogenesis to tumor treatment. Front. Genet. 13:1084609. doi: 10.3389/fgene.2022.1084609
Received: 30 October 2022; Accepted: 22 December 2022;
Published: 09 January 2023.
Edited by:
Sergey A. Krupenko, University of North Carolina at Chapel Hill, United StatesReviewed by:
Natalia Oleinik, Medical University of South Carolina, United StatesAndrew Wolfe, The City University of New York, United States
Copyright © 2023 Zhou, Tian, Cao, Zhao and Wang. This is an open-access article distributed under the terms of the Creative Commons Attribution License (CC BY). The use, distribution or reproduction in other forums is permitted, provided the original author(s) and the copyright owner(s) are credited and that the original publication in this journal is cited, in accordance with accepted academic practice. No use, distribution or reproduction is permitted which does not comply with these terms.
*Correspondence: Ke Wang, d2tlQGpsdS5lZHUuY24=