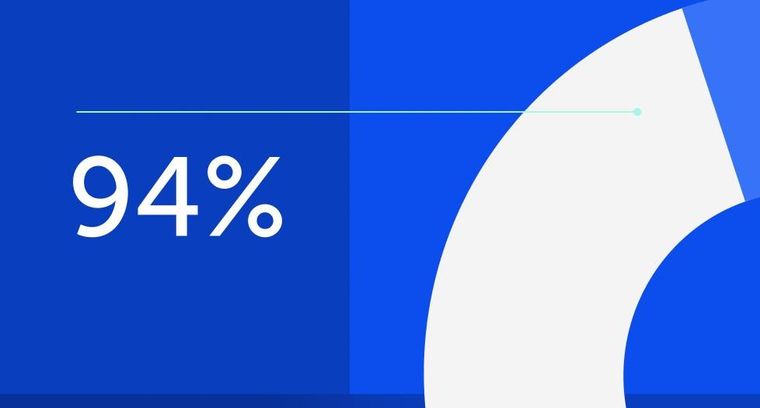
94% of researchers rate our articles as excellent or good
Learn more about the work of our research integrity team to safeguard the quality of each article we publish.
Find out more
ORIGINAL RESEARCH article
Front. Genet., 26 September 2022
Sec. Epigenomics and Epigenetics
Volume 13 - 2022 | https://doi.org/10.3389/fgene.2022.1008700
This article is part of the Research TopicRecent Advances in Epigenetic Mechanisms Controlling Plant Growth and DevelopmentView all 4 articles
DNA methylation patterns in plants are dynamically shaped by the antagonistic actions of DNA methylation and demethylation pathways. Although the DNA methylation pathway has been well studied, the DNA demethylation pathway, however, are not fully understood so far. To gain deeper insights into the mechanisms of DNA demethylation pathway, we conducted a genetic screening for proteins that were involved in preventing epigenetic gene silencing, and then the ones, which were also implicated in DNA demethylation pathway, were used for further studies. Eventually, a mutant with low luciferase luminescence (low LUC luminescence) was recovered, and named reduced LUC luminescence 6–1 (rll6-1). Map-based cloning revealed that rll6-1 mutation was located on chromosome 4, and there were a total of 10 candidate genes residing within such a region. Analyses of genome-wide methylation patterns of rll6-1 mutant showed that mutation of RLL6 locus led to 3,863 hyper-DMRs (DMRs for differentially methylated regions) throughout five Arabidopsis chromosomes, and elevated DNA methylation level of 2 × 35S promoter, which was similar to that found in the ros1 (repressor of silencing 1) mutant. Further analysis demonstrated that there were 1,456 common hyper-DMRs shared by rll6-1 and ros1-7 mutants, suggesting that both proteins acted together in a synergistic manner to remove DNA methylation. Further investigations demonstrated that mutation of RLL6 locus did not affect the expression of the four genes of the DNA glycosylase/lyase family. Thus, our results demonstrate that RLL6 locus-encoded protein not only participates in transcriptional anti-silencing of a transgene, but is also involved in DNA demethylation pathway.
DNA methylation is a conserved epigenetic mark that plays important roles in plant and vertebrate development, genome stability, and gene regulation (Law and Jacobsen, 2010). In animals, 5-methylcytisines (5-meCs) predominantly occur at CG dinucleotides, whereas in plants 5-meCs are found in CG, CHG and CHH contexts (where H is A, C or T) (He et al., 2011). RNA-directed DNA methylation (RdDM) is able to establish the de novo DNA methylation in all sequence contexts (Matzke and Mosher, 2014). Methylation in each context is mainly maintained by three types of DNA methyltransferases: the CG and CHG methylation is maintained by methyltransferase 1 (MET1) and chromomethylase 3 (CMT3), respectively, while the CHH methylation is maintained by both DOMAINS REARRANGED methylase 2 (DRM2) and chromomethylase 2 (CMT2) (Cao and Jacobsen, 2002; Law and Jacobsen, 2010; Zemach et al., 2013).
However, DNA methylation can be counteracted by DNA demethylation in vivo. According to the way it happens, the DNA demethylation can be classified into two categories: passive DNA demethylation and active DNA demethylation (Wu and Zhang, 2010). The former occurs during DNA replication when the activity of a DNA methyltransferase is inhibited (Wu and Zhang, 2010). On the other hand, the active DNA demethylation is catalyzed by DNA demethylases, and has been found to perform prominent functions in preventing the spread of DNA methylation to the neighboring regions (Zhu et al., 2007). In mammals, active DNA demethylation is initiated by TEN-ELEVEN-TRANSLOCATION (TET) enzymes or ACTIVATION INDUCED deaminase/APOLIPOPROTEIN B RNA-EDITING CATALYTIC COMPONENT-1 (AID/APOBEC), followed by the actions of DNA glycosylase THYMINE DNA glycosylase (TDG) or METHYL-CpG-BINDING DOMAIN 4 (MBD4) (Kohli and Zhang, 2013). In Arabidopsis, REPRESSOR OF SILENCING 1 (ROS1) and its paralogs DEMETER (DME), DEMETER-LIKE 2 (DML2) and DEMETER-LIKE 3 (DML3) are required for preventing DNA hypermethylation occurring at thousands of genomic regions (Gong et al., 2002; Law and Jacobsen, 2010). Transcript level of the ROS1 is regulated by MET1 and some RdDM components (Huettel et al., 2006), and ros1 mutation causes the silencing of two expression cassettes (RD29A-LUC and 35S-NPTII) in a transgene (Gong et al., 2002; Zheng et al., 2008).
In DNA methylation pathway, enzymes responsible for methylating DNA are guided to specific loci by base-pairing between small RNAs and scaffold transcripts (Law and Jacobsen, 2010; He et al., 2011). Recent studies showed that the functioning of ROS1 requires a protein complex containing INCREASED DNA METHYLATION 1 (IDM1), INCREASED DNA METHYLATION 2 (IDM2), INCREASED DNA METHYLATION 3 (IDM3), and METHYL-CpG-BINDING DOMAIN 7 (MBD7) (Qian et al., 2012; Qian et al., 2014; Lang et al., 2015). Of these four proteins, the MBD7 binds to hypermethylated CG-dense region and interacts with IDM2 and IDM3 (Wang et al., 2015). Both the IDM2 and IDM3 are α-crystallin domain-containing protein and interact with the IDM1 (Wang et al., 2015). The IDM1 encodes a histone acetyltransferase and such a protein is considered essential for ROS1-mediated DNA demethylation at certain loci (Wang et al., 2015). These four proteins form a functional complex and recruit ROS1 to suppress spread of DNA methylation (Wang et al., 2015). Besides, REPRESSOR OF SILENCING 3 (ROS3) was identified to bind to single-stranded RNAs and to be co-localized with ROS1 in nucleus. Moreover, both the ros3 and ros1 mutants exhibited concomitantly increased DNA methylation at certain loci, indicative of ROS3 acting in a same genetic pathway as did ROS1 (Zheng et al., 2008). Nevertheless, the mechanisms of how these DNA demethylases are specifically recruited to such targets remain largely unclear.
In order to gain deeper insights into the DNA demethylation pathway in Arabidopsis, in this study, we performed a genetic screening for candidate mutants with lowered LUC luminescence from an EMS (ethyl methanesulfonate)-mutagenized F2 population, which was derived from a parental line Col-LUC that carried a 2 × 35S-LUC transgene and a homozygous rdr6-11 mutation (which was able to minimize recovery of post-transcriptional gene silencing mutants) (Supplementary Figure S1). In the end, a low-LUC-luminescence mutant named reduced LUC luminescence 6–1 (rll6-1) was obtained, and genome-wide DNA methylation profiling showed that mutation of RLL6 locus led to a large number of hyper-DMRs throughout the Arabidopsis chromosomes, and there were 1,456 hyper-DMRs overlapping between rll6-1 and ros1-7 mutants, collectively suggesting that RLL6 locus-encoded protein participates in both anti-silencing of a transgene and demethylation of endogenous loci through the DNA demethylation pathway.
In order to identify more proteins participating in DNA demethylation pathway, we mutagenized a Col-LUC transgenic line by EMS and then conducted genetic screening for low-LUC-luminescence mutants; in this way, a few anti-silencing genes or DNA demethylation genes, such as ROS1 and IDM1, had been recovered (Supplementary Figure S1). Our screening led to isolation of one candidate mutant, hereafter named rll6-1, from such a mutagenized population in the M2 generation (Figure 1A). When compared to the two parental lines (Col-LUC and Ler-LUC), the rll6-1 mutant plants emitted a significantly low level of LUC luminescence, which was similar to that emitted by ros1-7 or idm1-4 mutant plants (Figure 1B). RT-PCR and RT-qPCR analyses showed that the expression of LUC gene in the rll6-1 mutant plants was significantly reduced relative to that in Col-LUC plants, but it showed somewhat higher than in ros1-7 mutant plants (Figures 1C,D), which was in good agreement with intensity of LUC luminescence observed in such genotypes. Thus, RLL6 locus-encoded protein seemed to play a similar role in repressing gene silencing as did ROS1.
FIGURE 1. rll6-1 mutation causes transcriptional gene silencing. (A) Genetic screening for low-LUC-luminescence mutants from an EMS-mutagenized M2 population. A candidate mutant was boxed with red and named rll6-1. (B) LUC luminescence performance of seedlings from rll6-1 mutant compared to Col-LUC, Ler-LUC, ros1-7 and idm1-4 seedlings. Left panel: 10-day-old seedlings grown on 1/2 MS medium; right panel: 30-day-old detached leaves. (C–D) Semi-quantitative RT-PCR (C) and RT-qPCR (D) analyses of LUC expression in the Col-LUC and rll6-1 mutant genotypes. The ros1-7 mutant served as a low-LUC-luminescence control. Asterisks indicate a significant difference from the Col-LUC by Student’s t-test (**, p < 0.01). Data are the means ± SD for three biological replicates.
Morphological phenotypes of the rll6-1 mutant plants exhibited no visible differences from those of Col-LUC plants at both juvenile and bolting stages (Figure 2A and Supplementary Figure S2A); however, areas of the third and fourth leaves from rll6-1 mutant plants were significantly larger than those from Col-LUC plants (Figures 2B,C). To know if rll6-1 mutant plants exhibited similar MMS (methyl methanesulfonate)-sensitive phenotype as did the ros1 mutant plants (Gong et al., 2002), the seeds from the Col-LUC and rll6-1 mutant plants were sown on 1/2 Murashige and Skoog (MS) agar plates containing 50 mg/L MMS, and such plates were placed under normal growth conditions for 2 weeks. As shown in Supplementary Figure S2B, the rll6-1 mutant plants did not show observable sensitivity to MMS, which was opposite to the MMS-sensitive phenotype displayed by the ros1 mutant plants. Moreover, as was the case with MMS treatment, rll6-1 mutant plants did also not show differences in sensitivity from Col-LUC plants when treated with NaCl or ABA (Supplementary Figure S2B).
FIGURE 2. Developmental phenotypes of the rll6-1 mutant plants under the conditions with or without a variety of abiotic stresses. (A) Comparisons of plant morphologies between 3-week-old rll6-1 mutant and Col-LUC control plants. (B) Morphological comparisons between individual leaves from a representative rll6-1 mutant and the counterparts from a Col-LUC plant. Both rll6-1 mutant and Col-LUC plants were at 3 weeks of age. (C) Comparisons of areas of the third, fourth and fifth leaves from the rll6-1 mutant and Col-LUC plant as shown in (B). Asterisks indicate a significant difference from the Col-LUC by Student’s t-test (**, p < 0.01). (D) Comparisons of CdCl2 tolerance among rll6-1 as well as ros1-7 mutant plants and Col-LUC control plants. 1/2 MS: seeds were germinated on 1/2 MS medium, and the resulting seedlings were continuously grown on the same medium until they were photographed. 40 μM CdCl2: seeds were germinated on 1/2 MS medium supplemented with 40 μM CdCl2, and allowed the seedlings to continuously grow on the same medium until they were photographed. 1/2 MS to 40 μM CdCl2: seeds were germinated on 1/2 MS and continuously grown for 1 week, and then the seedlings were transferred to 1/2 medium supplemented with 40 μM CdCl2 to allow them to grow for another 1 week. 40 uM CdCl2 to 1/2 MS: seeds were germinated on 1/2 MS supplemented with 40 μM CdCl2 and allowed them continuously grow for 1 week, and then the seedlings were transferred to 1/2 MS medium to leave them growing for another 1 week. All seedlings were grown for a total of 2 weeks post-germination before photography. (E) Comparisons of lengths of roots from three genotypes (rll6-1, ros1-7 and Col-LUC) that were grown on media as indicated in (D). Asterisks indicate a significant difference from the Col-LUC genotype under the same treatment conditions by Student’s t-test (**, p < 0.01). Data are the means ± SD for three biological replicates.
A previous study showed that rdd triple mutant (for ros1 dml2 dml3) exhibited an elevated tolerance to CdCl2 stress (Fan et al., 2020). We found that, however, rll6-1 mutant plants showed evidently enhanced sensitivity to 40 μM CdCl2 treatment, as shown by more retarded growth observed for the rll6-1 mutant seedlings, when rll6-1 mutant seeds as well as ros1-7 and Col-LUC seeds were germinated on CdCl2-containing medium in parallel (Figure 2D); however, when the rll6-1 mutant seeds were germinated on CdCl2-containing medium and the seedlings were then transferred to 1/2 MS, their roots grew longer than those from ros1-7 mutant and Col-LUC plants (Figures 2D,E).
To know if rll6-1 locus was allelic to known mutations of genes associated with DNA demethylation pathway, rll6-1 mutant was crossed with Ler-LUC, ros1-7, idm1-4 or rdr6-11, respectively, to produce F1 seeds. The resulting F1 seeds were subsequently sown on 1/2 MS medium, and the seedlings were subjected to imaging of LUC luminescence following growth for 2 weeks. It was abundantly clear that the F1 seedlings from the crosses rll6-1 × ros1-7 and rll6-1 × idm1-4 all displayed high LUC luminescence as Col-LUC seedlings did, suggesting that rll6-1 mutation was not allelic to ros1 or idm1 mutation (Figure 3A). The low-LUC-luminescence phenotype of the rll6-1 mutant plants did also not result from mutation of the LUC gene because the seedlings from the cross rll6-1 × rdr6-11 (rdr6-11 mutant did not carry 2 × 35S-LUC transgene) exhibited obviously increased LUC luminescence in comparison with those from rll6-1 mutant seedlings (Figure 3A). Altogether, these results indicated that RLL6 locus appears to encode a protein that participates in anti-silencing of a transgene.
FIGURE 3. Allelic tests and map-based cloning of rll6-1 mutation. (A) Left panel: Allelic tests between rll6-1 and ros1 or idm1 mutant genotypes. Right panel: Relative luminescence intensities between Col-LUC and other seven genotypes. The luminescence intensities were quantified by ImageJ software. Data are the means ± SD for three biological replicates. Significant differences from Col-LUC were determined by Student’s t-test. **, p < 0.01. (B) Approximate location of rll6-1 locus on Arabidopsis chromosome 4, which was identified by a map-based cloning approach. The recombination fractions in the genetic mapping were shown in parentheses.
To map rll6-1 locus, we crossed the rll6-1 mutant plants with Ler-LUC plants, and the resulting F2 population was used for such a purpose. It was evident that the F2 individuals showed a phenotype segregation of 3:1 (high-LUC-luminescence: low-LUC-luminescence = 408:120; χ2 = 1.34), implicating that the rll6-1 mutant carried a recessive mutation occurring in a single nuclear gene. Primary mapping was conducted by selecting 120 stably-low-LUC-luminescence plants for genetic linkage analysis (Supplementary Figure S3A). The results indicated that the rll6-1 locus was mapped to the short arm of chromosome 4, which was in the vicinity of BAC clone F6N15 (Supplementary Figure S3B). For fine mapping the rll6-1 locus, 386 low-LUC-luminescence plants out of 1659 F2 progenies were used. Eventually, the rll6-1 locus was narrowed down to an ∼80-kb region within the BAC clone F5I10 (Figure 3B). Gene annotation from TAIR website revealed that there were 10 candidate genes residing in such a region (Table 1), which encoded proteins of different molecular functions, including transcriptional regulation, carbohydrate binding, methylated CpG binding, RNA binding, and so on. Further studies are needed to determine which one is the true gene responsible for maintenance of LUC luminescence.
To know whether the silencing of LUC transgene in rll6-1 mutant plants resulted from enhanced DNA methylation occurring on the promoter of such a transgene, rll6-1 mutant seedlings were treated with DNA methyltransferase inhibitor 5-Aza-2′-deoxycytodine which was known to cause global DNA hypomethylation when they were used to treat plants. It was apparent that the LUC luminescence emitted from the rll6-1 mutant seedlings was obviously enhanced after the treatment (which was similar to that emitted from Col-LUC seedlings), as it was in ros1-7 (Figure 4A). Therefore, this result suggested that mutation of RLL6 locus very likely led to an elevation of DNA methylation level on the 2 × 35S promoter situated in front of LUC gene.
FIGURE 4. Effects of 5-Aza-2′-deoxycytodine on LUC expression in rll6-1 mutant plants. (A) Performance of LUC luminescence of 14-day-old seedlings from rll6-1, ros1-7 and Col-LUC genotypes under the conditions with or without 7 μg/ml 5-Aza-2′-deoxycytodine treatments. Left panel: image of LUC luminescence from seedlings of the three genotypes as indicated grown on 1/2 MS medium for 1 week. Right panel: image of LUC luminescence from seedlings of three genotypes as indicated grown on 1/2 MS medium, which was supplemented with 7 μg/ml 5-Aza-2′-deoxycytodine, for 1 week. (B) Examination of DNA methylation status at several endogenous loci in ros1-7 and rll6-1 mutants by Chop-PCR method. HpaII, ClaI and HpyCH4IV were methylation-sensitive restriction enzymes used to digest genomic DNA. Undigested DNA served as a control. (C) Detection of expression levels of several essential genes, which were involved in ROS1-mediated DNA demethylation pathway, in rll6-1 mutant by semi-quantitative RT-PCR method.
In order to find if mutation of RLL6 locus results in increased DNA methylation levels at endogenous genomic loci as well, we examined the DNA methylation status of a few particular loci (which showed noticeable hypermethylation in ros1-7 mutant) in rll6-1 mutant by using the Chop-PCR method (Figure 4B). It was interesting that the rll6-1 mutant, as did the ros1-7, exhibited elevated level of DNA methylation at the aforementioned loci, suggesting that RLL6 locus-encoded protein presumably works in close collaboration with ROS1 to counteract DNA methylation in such loci. To ascertain if the increases of DNA methylation levels at those loci were the result of downregulation of ROS1 expression, we examined the expression of genes involved in ROS1-mediated DNA demethylation pathway in the rll6-1 mutant by RT-PCR assays. Surprisingly, there were no significant expression changes for the nine genes detected, such as ROS1, ROS3, IDM1, DME and MBD7, etc., in the rll6-1 mutant plants (Figure 4C), clearly indicating that the elevated DNA methylation at certain loci caused by mutation of RLL6 locus may be not a result of deficiency or downregulation of the expression of DNA demethylation pathway genes.
Given the fact that a few genomic loci, which showed increased DNA methylation in ros1-7 mutant, exhibited prominent DNA hypermethylation in rll6-1 mutant, we wondered whether there were more DNA hypermethylated loci shared by both mutants on a genome-wide scale. Therefore, both mutants were subjected to the whole-genome bisulfite sequencing. Analyses of DNA methylation profiling data revealed noticeable increases in DNA methylation in three sequence contexts (CG, CHG, and CHH) on the 2 × 35S promoters in the rll6-1 mutant; moreover, the levels of DNA methylation in each of the three sequence contexts were virtually the same between rll6-1 and ros1-7 mutants (Figure 5A). Further analysis indicated that genome-wide DNA hypermethylation occurred at the three sequence contexts (Supplementary Figure S4A), and the percentage proportional distributions of hyper-DMRs on each of constituents of genome [gene, TE, and intergenic region (IG)] were quite similar between the rll6-1 and ros1-7 mutants, with approximately 38% of hyper-DMRs concentrated on TEs in both mutants (Supplementary Figure S4B); furthermore, it was remarkable that the largest proportion of the hyper-DMRs overlapping with TEs appeared to cluster on 0–0.5-kb TEs in both the mutants (Supplementary Figure S4C). The analyses also demonstrated that there was a total of 3,863 hyper-DMRs and 700 hypomethylated differentially methylated regions (hypo-DMRs) were identified in the rll6-1 mutant relative to Col-LUC genotype, while 7,098 hyper-DMRs and 410 hypo-DMRs were identified in the ros1-7 mutant versus the same Col-LUC plant (Supplementary Table S1). We then compared the hyper-DMRs between rll6-1 and ros1-7 mutants and obtained 1,456 common hyper-DMRs, which accounted for about 37.7% and 20.5% of total hyper-DMRs in the rll6-1 and ros1-7 mutants, respectively (Figure 5B). Boxplot analysis indicated that for such 1,456 common hyper-DMRs, their methylation levels in the three sequence contexts were all obviously increased in both mutant genotypes by comparison with Col-LUC genotype (Figure 5B); for example, a few loci (chr1: 9,564,000–9,566,000, chr2: 9,946,000–9,949,000, chr3: 2,887,000–2,890,000, chr3: 4,647,000–4,649,000, etc.) showed clear hypermethylation in the rll6-1 and ros1-7 mutants, when compared to the Col-LUC control (Figure 5C). Moreover, as for the hyper-DMRs unique to rll6-1 mutant, the elevation of DNA methylation levels in the three sequence contexts in the rll6-1 mutant genotype versus Col-LUC genotype was coincident with the increase of those in ros1-7; however, as regards the 5,642 hyper-DMRs exclusive to the ros1-7, although the rise of those in the ros1-7 mutant genotype versus the Col-LUC genotype was significant, the methylation levels in the three sequence contexts in the rll6-1 mutant genotype was just marginally increased compared to the Col-LUC genotype (Figure 5B). Taken together, these results further supported the above-mentioned notion that the RLL6 locus-encoded protein presumably acts in close collaboration with ROS1 to antagonize DNA methylation in thousands of loci at a genome-wide level.
FIGURE 5. Analysis of hyper-DMRs identified in rll6-1 and ros1-7 mutants. (A) DNA methylation levels of 2 × 35S promoter in rll6-1 as well as ros1-7 mutant and Col-LUC control genotypes. Left panel: a screenshot of DNA methylation status in 2 × 35S promoter regions from the three genotypes as indicated. Right panel: quantification of methylation levels of the 2 × 35S promoter regions shown in (A). (B) Overlap of hyper-DMRs between the rll6-1 and ros1-7 mutants. Boxplots showed methylation levels (for CG, CHG and CHH sequence contexts) of each class of hyper-DMRs (1,456 for overlapped hyper-DMRs; 5,642 and 2,407 for hyper-DMRs unique to ros1-7 and rll6-1 mutants, respectively). (C) Screenshots of DNA methylation status of several endogenous genomic loci from the rll6-1 as well as ros1-7 mutant and Col-LUC control genotypes.
In this study, we identified one new low-LUC-luminescence mutant, and mapped the mutation to a region in bacterial artificial chromosome (BAC) clones F5I10 on Arabidopsis chromosome 4 (Figures 1, 3). Our mapping of rll6 locus led to the identification of a region including 10 candidate genes (Table 1). Among the 10 genes, the MBD3 (AT4G00416), MYB3R2 (AT4G00540) and double-stranded RNA-binding domain-containing protein (AT4G00420) appeared to be quite interesting. There are 13 Methyl-CpG-binding domain-containing proteins present in Arabidopsis genome, three of which, i.e. MBD5, MBD6 and MBD7, were found to bind specifically to methylated CG sites in vitro (Zemach and Grafi, 2007). Recent studies showed that MBD7 was physically associated with the histone acetyltransferase IDM1, and it participated in active DNA demethylation in Arabidopsis (Lang et al., 2015; Wang et al., 2015). Thus, MBD3 seems to be a potential candidate involved in inhibiting DNA methylation and preventing transcriptional gene silencing. MYB proteins are known to generally function as transcription factors engaging in the defense responses of plants (Zheng et al., 2012). In Arabidopsis, MYB74 belongs to the R2R3-MYB protein family, and such a protein was transcriptionally regulated by RdDM pathway (Xu et al., 2015). As a putative c-myb-like transcription factor, whether the MYB3R2 (AT4G00540) is involved in the RdDM pathway or DNA demethylation pathway remains unclear. Moreover, the double-stranded RNA-binding domain-containing protein (AT4G00420) appeared to be also a candidate because, previous research showed that an RNA-binding protein ROS3 was required for DNA demethylation pathway in Arabidopsis (Zheng et al., 2008); hence, such a protein is worth being further examined to find whether it participates in the DNA demethylation pathway.
Previous research showed that there existed the same and different mechanisms underlying the silencing of 2 × 35S promoter when compared to RD29A promoter (Gong et al., 2002). Using the same genetic screening system, a ros1-7 mutant allele was identified, in which the increased DNA methylation level on the 2 × 35S promoter resulted in transcriptional silencing of LUC gene (Hou et al., 2022). However, SAC3B dysfunction resulted in LUC gene silencing without obviously altering promoter DNA methylation level of the transgene 2 × 35S-LUC (Yang et al., 2017). Our study revealed that RLL6 locus-encoded protein was also required to protect the 2 × 35S promoter from being silenced just like ROS1 did, so the two anti-silencing factors seemed to play similar roles in anti-silencing of such a promoter as DNA methylation patterns at the 2 × 35S-LUC promoter were increased both in the ros1 and in rll6-1 mutants (Figure 5A). Moreover, the silencing state of such a promoter in rll6-1 mutant seedlings could be released by 5-Aza-2′-deoxycytodine, similar to what was observed in ros1-7 seedlings (Figure 4A). Therefore, these results indicate that both RLL6 locus-encoded protein and ROS1 participate in inhibiting transcriptional gene silencing through a similar mechanism.
Epigenetic mutations are usually accompanied by developmental defects in Arabidopsis. DEMETER (DME) demethylated small transposons and edges of long transposons (Gehring et al., 2006; Schoft et al., 2011; Ibarra et al., 2012; Park et al., 2017; Frost et al., 2018), and mutation of DME resulted in seed abortion and abnormal germination of pollen tubes (Choi et al., 2002; Schoft et al., 2011). Mutation of increase IN BONSAI METHYLATION 1 (IBM1) induced a variety of developmental phenotypes, including smaller leaves, abnormal flower development and reduced fertility, which depended on methylation status of histone H3 at lysine 9 (Saze et al., 2008). Arabidopsis SAC3B dysfunction caused elevation in the repressive histone mark H3K9me2, accompanied by shorter roots, smaller leaves and shorter inflorescence (Yang et al., 2017). In this study, we observed that rll6-1 mutant showed enlarged third and fourth leaves, although the effect was weaker than that in other mutants mentioned above, implicating that RLL6 locus-encoded protein may be also involved in the regulation of plant development (Figures 2A,B).
One of the most important roles of DNA methylation was to silence TEs, which exist extensively in plant and animal genomes and tend to spread to adjacent genes (Bennetzen and Wang, 2014). Previous research showed that transcription of EPF2 gene, which was near a methylated TE in Arabidopsis, relied on the demethylation of ROS1, which played a critical role in preventing spread of DNA methylation from methylated TEs to adjacent sequences (Yamamuro et al., 2014). Our findings revealed that RLL6 locus-encoded protein also limited the spread of DNA methylation from high methylated regions to neighboring regions (Figure 5C).
In plants, active DNA demethylation is initiated by the ROS1/DME family of 5-methylcytosine DNA glycosylases (He et al., 2009). In this study, many hypermethylated loci were identified in rll6-1 mutant, and more than one third were overlapped with those of ros1-7 mutant (Figure 5B). Moreover, both mutants showed similar increased DNA methylation patterns in many representative loci, suggesting that both proteins synergistically regulated DNA hypermethylation in some loci (Figure 5B). To date, how the ROS1 was targeted to specific genomic loci remains largely unclear. Our data indicated that RLL6 locus-encoded protein had a very close relationship with ROS1, whereas mutation of the RLL6 locus did not affect expression of ROS1 as well as other ROS1/DME family genes (Figure 4C). Recent research showed that the IDM1-IDM2-IDM3-MBD7 complex played an important role in facilitating active DNA demethylation through ROS1 (Lang et al., 2015; Wang et al., 2015); however, mutation of RLL6 locus did not affect the expression of these genes (Figure 4C), demonstrating that RLL6 locus-encoded protein inhibited DNA hypermethylation not by affecting the expression of those genes participating in ROS1-mediated DNA demethylation. Moreover, the 2,407 unique hyper-DMRs existing in the rll6-1 mutant indicated that the RLL6 locus-encoded protein could inhibit DNA hypermethylation independently of ROS1 (Figure 5B). So, it is necessary to pinpoint the mutation site on RLL6 locus to further understand the roles of the RLL6 locus-encoded protein in ROS1-dependent DNA demethylation pathway and ROS1-independent DNA demethylation process.
The wild-type plants in this study were from the Col-LUC transgenic line bearing 2 × 35S-LUC in the rdr6-11 mutant background. The Ler-LUC line was the Landsberg-0 (Ler-0) plant harboring the same 2 × 35S-LUC transgene and rdr6-11 mutation as those present in Col-LUC line, which was generated by backcrossing Col-LUC with Ler-0 six times. All the seeds we used were surface-sterilized with 0.8% sodium hypochlorite (NaClO) before sown on Murashige and Skoog (MS) medium containing 0.8% (w/v) agar and 2% (w/v) sucrose. For NaCl, ABA, MMS, CdCl2 or 5-Aza-2′-deoxycytodine treatments, seeds were sown on 1/2 MS medium supplemented with 0.8% (w/v) agar and 2% (w/v) sucrose, with 50 mg/L MMS, 0.1 M NaCl, 0.2 μM ABA, or 7 μg/ml 5-Aza. Each treatment was repeated three times (three replicates), and 150 seeds were sown for each replicate. After vernalization at 4°C for 48 h, the plants were transferred to a long-day photoperiod (16-h light/8-h dark) at 22°C in a growth room. EMS mutagenesis and screening of mutants with reduced LUC luminescence were conducted as described previously (Yang et al., 2017).
For LUC luminescence imaging, seedlings were sown on 1/2 MS medium, which contained 0.8% (w/v) agar and 2% (w/v) sucrose. Prior to the imaging, two-week-old seedlings were placed in the dark for 5 min after being sprayed with 1 mM luciferin. Then the plants were put in a Princeton Dark Box equipped with a Roper VersArray1300B camera controlled by the WinView32 software, and was imaged with a 30-s exposure time.
To map the rll6-1 mutation, rll6-1 mutant plants (female) were crossed with Ler-LUC plants (male), and the resulting F1 seeds were selfed to produce an F2 population. The low-LUC-luminescence F2 individuals were selected to form a mapping population. Primary mapping with 120 F2 individuals delimited the rll6-1 locus to the top of the chromosome four in the vicinity of the BAC clone F6N15. Fine mapping further narrowed down the rll6-1 locus to an ∼80-kb region on the BAC clone F5I10 by using 386 F2 individuals.
Gene expression analysis was carried out according to the methods described previously (Lei et al., 2014). Briefly, RNA was extracted from 14-days-old seedlings, and about 1 μg of total RNA was used to synthesize the first-strand cDNA using One-step gDNA removal and cDNA Synthesis SuperMix kits (Transgen, Beijing, China). Subsequently, the cDNA was used for RT-PCR or qRT-PCR analysis for LUC expression; a housekeeping gene ACTIN2 served as internal controls for all reactions.
For Chop-PCR assays, approximately 600 ng of genomic DNA was digested by methylation-sensitive enzymes for 16 h, and then they were subjected to PCR with different primer sets. All the primers used were listed in the Supplementary Table S2. For whole-genome bisulfite sequencing, DNA samples were extracted from two-week-old seedlings by the CTAB method, then used for bisulfite treatment and DNA sequencing [Novogene (Beijing, China)] as previously described (Duan et al., 2015). Differentially methylated regions (DMRs) were identified as described previously (Duan et al., 2015).
The datasets presented in this study can be found in online repositories. The names of the repository/repositories and accession number(s) can be found in the article/Supplementary Material.
XW and HL designed the research plan; XW and MW conducted the experiments; XW and JD analyzed the data; XW, QW, and HL wrote and revised the manuscript. All authors have read and approved the final version of the manuscript.
This study was funded by the National Natural Science Foundation of China (31970582) and the National Natural Science Foundation of China (31771427) to HL.
Data analysis was supported by the high-performance computing platform of Bioinformatics Center, Nanjing Agricultural University.
The authors declare that the research was conducted in the absence of any commercial or financial relationships that could be construed as a potential conflict of interest.
All claims expressed in this article are solely those of the authors and do not necessarily represent those of their affiliated organizations, or those of the publisher, the editors and the reviewers. Any product that may be evaluated in this article, or claim that may be made by its manufacturer, is not guaranteed or endorsed by the publisher.
The Supplementary Material for this article can be found online at: https://www.frontiersin.org/articles/10.3389/fgene.2022.1008700/full#supplementary-material
AID/APOBEC, activation induced deaminase/apolipoprotein B RNA-editing catalytic component-1; BAC, bacterial artificial chromosome; CMT2, Chromomethylase two; CMT3, Chromomethylase three; DRM2, domains rearranged methylase two; CpG-binding domain four; DMRs, differentially methylated regions; DME, demeter; DML2, demeter-like two; DML3, demeter-like three; EMS, ethyl methanesulfonate; rll6-1, reduced LUC luminescence six to one; \5-meC, 5-methylcytisine; MMS, methyl methanesulfonate; MS, Murashige and Skoog; MET1, Methyltransferase one; MBD4, methyl-ROS1, repressor of silencing one; IDM1, increased DNA methylation one; RdDM, RNA-directed DNA methylation; IDM2, increased DNA methylation two; IDM3, increased DNA methylation three; MBD7, methyl-CpG-binding domain seven; ROS3, repressor of silencing one; rdd, ros1 dml2 dml3 triple mutant; IG, intergenic region; IBM1, Increase in bonsai methylation one; TDG, thymine DNA glycosylase; TET, ten-eleven-translocation; TFEB, transcription factor EB
Bennetzen, J. L., and Wang, H. (2014). The contributions of transposable elements to the structure, function, and evolution of plant genomes. Annu. Rev. Plant Biol. 65, 505–530. doi:10.1146/annurev-arplant-050213-035811
Cao, X., and Jacobsen, S. E. (2002). Role of the arabidopsis DRM methyltransferases in de novo DNA methylation and gene silencing. Curr. Biol. 12, 1138–1144. doi:10.1016/s0960-9822(02)00925-9
Choi, Y., Gehring, M., Johnson, L., Hannon, M., Harada, J. J., Goldberg, R. B., et al. (2002). DEMETER, a DNA glycosylase domain protein, is required for endosperm gene imprinting and seed viability in arabidopsis. Cell 110, 33–42. doi:10.1016/s0092-8674(02)00807-3
Duan, C. G., Wang, X., Tang, K., Zhang, H., Mangrauthia, S. K., Lei, M., et al. (2015). MET18 connects the cytosolic iron-sulfur cluster Assembly pathway to active DNA demethylation in arabidopsis. PLoS Genet. 11, e1005559. doi:10.1371/journal.pgen.1005559
Fan, S. K., Ye, J. Y., Zhang, L. L., Chen, H. S., Zhang, H. H., Zhu, Y. X., et al. (2020). Inhibition of DNA demethylation enhances plant tolerance to cadmium toxicity by improving iron nutrition. Plant Cell Environ. 43, 275–291. doi:10.1111/pce.13670
Frost, J. M., Kim, M. Y., Park, G. T., Hsieh, P. H., Nakamura, M., Lin, S. J. H., et al. (2018). FACT complex is required for DNA demethylation at heterochromatin during reproduction in Arabidopsis. Proc. Natl. Acad. Sci. U. S. A. 115, E4720–e4729. doi:10.1073/pnas.1713333115
Gehring, M., Huh, J. H., Hsieh, T. F., Penterman, J., Choi, Y., Harada, J. J., et al. (2006). DEMETER DNA glycosylase establishes MEDEA polycomb gene self-imprinting by allele-specific demethylation. Cell 124, 495–506. doi:10.1016/j.cell.2005.12.034
Gong, Z., Morales-ruiz, T., Ariza, R. R., Roldan-Arjona, T., David, L., and Zhu, J. K. (2002). ROS1, a repressor of transcriptional gene silencing in Arabidopsis, encodes a DNA glycosylase/lyase. Cell 111, 803–814. doi:10.1016/s0092-8674(02)01133-9
He, X. J., Chen, T., and Zhu, J. K. (2011). Regulation and function of DNA methylation in plants and animals. Cell Res. 21, 442–465. doi:10.1038/cr.2011.23
He, X. J., Hsu, Y. F., Zhu, S., Wierzbicki, A. T., Pontes, O., Pikaard, C. S., et al. (2009). An effector of RNA-directed DNA methylation in arabidopsis is an ARGONAUTE 4- and RNA-binding protein. Cell 137, 498–508. doi:10.1016/j.cell.2009.04.028
Hou, Z., Dai, J., Wang, C., Cheng, Y., La, Y., Niu, J., et al. (2022). RLL5, an F-box-containing protein, involved in preventing transgene silencing and in maintaining global DNA methylation in Arabidopsis. Biochem. Biophys. Res. Commun. 609, 1–8. doi:10.1016/j.bbrc.2022.03.135
Huettel, B., Kanno, T., Daxinger, L., Aufsatz, W., Matzke, A. J., and Matzke, M. (2006). Endogenous targets of RNA-directed DNA methylation and pol IV in arabidopsis. EMBO J. 25, 2828–2836. doi:10.1038/sj.emboj.7601150
Ibarra, C. A., Feng, X., Schoft, V. K., Hsieh, T. F., Uzawa, R., Rodrigues, J. A., et al. (2012). Active DNA demethylation in plant companion cells reinforces transposon methylation in gametes. Science 337, 1360–1364. doi:10.1126/science.1224839
Kohli, R. M., and Zhang, Y. (2013). TET enzymes, TDG and the dynamics of DNA demethylation. Nature 502, 472–479. doi:10.1038/nature12750
Lang, Z., Lei, M., Wang, X., Tang, K., Miki, D., Zhang, H., et al. (2015). The methyl-CpG-binding protein MBD7 facilitates active DNA demethylation to limit DNA hyper-methylation and transcriptional gene silencing. Mol. Cell 57, 971–983. doi:10.1016/j.molcel.2015.01.009
Law, J. A., and Jacobsen, S. E. (2010). Establishing, maintaining and modifying DNA methylation patterns in plants and animals. Nat. Rev. Genet. 11, 204–220. doi:10.1038/nrg2719
Lei, M., La, H., Lu, K., Wang, P., Miki, D., Ren, Z., et al. (2014). Arabidopsis EDM2 promotes IBM1 distal polyadenylation and regulates genome DNA methylation patterns. Proc. Natl. Acad. Sci. U. S. A. 111, 527–532. doi:10.1073/pnas.1320106110
Matzke, M. A., and Mosher, R. A. (2014). RNA-Directed DNA methylation: An epigenetic pathway of increasing complexity. Nat. Rev. Genet. 15, 394–408. doi:10.1038/nrg3683
Park, J. S., Frost, J. M., Park, K., Ohr, H., Park, G. T., Kim, S., et al. (2017). Control of DEMETER DNA demethylase gene transcription in male and female gamete companion cells in Arabidopsis thaliana. Proc. Natl. Acad. Sci. U. S. A. 114, 2078–2083. doi:10.1073/pnas.1620592114
Qian, W., Miki, D., Lei, M., Zhu, X., Zhang, H., Liu, Y., et al. (2014). Regulation of active DNA demethylation by an alpha-crystallin domain protein in Arabidopsis. Mol. Cell 55, 361–371. doi:10.1016/j.molcel.2014.06.008
Qian, W., Miki, D., Zhang, H., Liu, Y., Zhang, X., Tang, K., et al. (2012). A histone acetyltransferase regulates active DNA demethylation in Arabidopsis. Science 336, 1445–1448. doi:10.1126/science.1219416
Saze, H., Shiraishi, A., Miura, A., and Kakutani, T. (2008). Control of genic DNA methylation by a jmjC domain-containing protein in Arabidopsis thaliana. Science 319, 462–465. doi:10.1126/science.1150987
Schoft, V. K., Chumak, N., Choi, Y., Hannon, M., Garcia-Aguilar, M., Machlicova, A., et al. (2011). Function of the DEMETER DNA glycosylase in the Arabidopsis thaliana male gametophyte. Proc. Natl. Acad. Sci. U. S. A. 108, 8042–8047. doi:10.1073/pnas.1105117108
Wang, C., Dong, X., Jin, D., Zhao, Y., Xie, S., Li, X., et al. (2015). Methyl-CpG-binding domain protein MBD7 is required for active DNA demethylation in Arabidopsis. Plant Physiol. 167, 905–914. doi:10.1104/pp.114.252106
Wu, S. C., and Zhang, Y. (2010). Active DNA demethylation: Many roads lead to rome. Nat. Rev. Mol. Cell Biol. 11, 607–620. doi:10.1038/nrm2950
Xu, R., Wang, Y., Zheng, H., Lu, W., Wu, C., Huang, J., et al. (2015). Salt-induced transcription factor MYB74 is regulated by the RNA-directed DNA methylation pathway in Arabidopsis. J. Exp. Bot. 66, 5997–6008. doi:10.1093/jxb/erv312
Yamamuro, C., Miki, D., Zheng, Z., Ma, J., Wang, J., Yang, Z., et al. (2014). Overproduction of stomatal lineage cells in Arabidopsis mutants defective in active DNA demethylation. Nat. Commun. 5, 4062. doi:10.1038/ncomms5062
Yang, Y., La, H., Tang, K., Miki, D., Yang, L., Wang, B., et al. (2017). SAC3B, a central component of the mRNA export complex TREX-2, is required for prevention of epigenetic gene silencing in Arabidopsis. Nucleic Acids Res. 45, 181–197. doi:10.1093/nar/gkw850
Zemach, A., and Grafi, G. (2007). Methyl-CpG-binding domain proteins in plants: Interpreters of DNA methylation. Trends Plant Sci. 12, 80–85. doi:10.1016/j.tplants.2006.12.004
Zemach, A., Kim, M. Y., Hsieh, P. H., Coleman-Derr, D., Eshed-Williams, L., Thao, K., et al. (2013). The Arabidopsis nucleosome remodeler DDM1 allows DNA methyltransferases to access H1-containing heterochromatin. Cell 153, 193–205. doi:10.1016/j.cell.2013.02.033
Zheng, X., Pontes, O., Zhu, J., Miki, D., Zhang, F., Li, W. X., et al. (2008). ROS3 is an RNA-binding protein required for DNA demethylation in Arabidopsis. Nature 455, 1259–1262. doi:10.1038/nature07305
Keywords: arabidopsis thaliana, reduced LUC luminescence 6 (RLL6), DNA demethylation, map-based cloning, whole-genome bisulfite sequencing (WGBS), repressor of silencing 1 (ROS1)
Citation: Wang X, Wang M, Dai J, Wang Q and La H (2022) Fine mapping and characterization of RLL6 locus required for anti-silencing of a transgene and DNA demethylation in Arabidopsis thaliana. Front. Genet. 13:1008700. doi: 10.3389/fgene.2022.1008700
Received: 02 August 2022; Accepted: 29 August 2022;
Published: 26 September 2022.
Edited by:
Quanyi Zhao, Chinese Academy of Medical Sciences, ChinaReviewed by:
Qinglan Li, University of Pennsylvania, United StatesCopyright © 2022 Wang, Wang, Dai, Wang and La. This is an open-access article distributed under the terms of the Creative Commons Attribution License (CC BY). The use, distribution or reproduction in other forums is permitted, provided the original author(s) and the copyright owner(s) are credited and that the original publication in this journal is cited, in accordance with accepted academic practice. No use, distribution or reproduction is permitted which does not comply with these terms.
*Correspondence: Honggui La, aG9uZ2d1aWxhQG5qYXUuZWR1LmNu
Disclaimer: All claims expressed in this article are solely those of the authors and do not necessarily represent those of their affiliated organizations, or those of the publisher, the editors and the reviewers. Any product that may be evaluated in this article or claim that may be made by its manufacturer is not guaranteed or endorsed by the publisher.
Research integrity at Frontiers
Learn more about the work of our research integrity team to safeguard the quality of each article we publish.