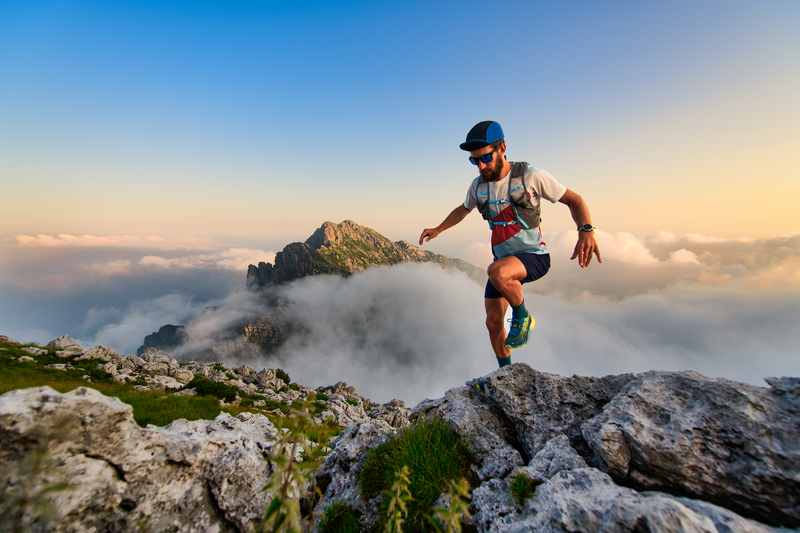
95% of researchers rate our articles as excellent or good
Learn more about the work of our research integrity team to safeguard the quality of each article we publish.
Find out more
ORIGINAL RESEARCH article
Front. Genet. , 25 November 2021
Sec. Evolutionary and Population Genetics
Volume 12 - 2021 | https://doi.org/10.3389/fgene.2021.788346
This article is part of the Research Topic The Evolution of Biomineralization in Metazoans View all 16 articles
While cartilage is an ancient tissue found both in protostomes and deuterostomes, its mineralization evolved more recently, within the vertebrate lineage. SPARC, SPARC-L, and the SCPP members (Secretory Calcium-binding PhosphoProtein genes which evolved from SPARC-L) are major players of dentine and bone mineralization, but their involvement in the emergence of the vertebrate mineralized cartilage remains unclear. We performed in situ hybridization on mineralizing cartilaginous skeletal elements of the frog Xenopus tropicalis (Xt) and the shark Scyliorhinus canicula (Sc) to examine the expression of SPARC (present in both species), SPARC-L (present in Sc only) and the SCPP members (present in Xt only). We show that while mineralizing cartilage expresses SPARC (but not SPARC-L) in Sc, it expresses the SCPP genes (but not SPARC) in Xt, and propose two possible evolutionary scenarios to explain these opposite expression patterns. In spite of these genetic divergences, our data draw the attention on an overlooked and evolutionarily conserved peripheral cartilage subdomain expressing SPARC or the SCPP genes and exhibiting a high propensity to mineralize.
The evolution of a mineralized skeleton occurred in early vertebrates, in a variety of tissues including superficial dermal scales and teeth, together with internal cartilages, and perichondral bones (Ørvig, 1951; Donoghue and Sansom, 2002). In the internal skeleton, several cell types are associated with biomineralization, and the most studied cell model in mammalian organisms is the osteoblast active in the endochondral ossification process (Long and Ornitz, 2013). These osteoblasts are derived from periosteal tissues or from hypertrophic transdifferentiated chondrocytes (Tsang et al., 2015). The process of endochondral ossification, or replacement of cartilage matrix by bone marrow and bone trabeculae, is absent from chondrichthyans and has long been thought to be a derived feature specific to osteichthyans (reviewed in Donoghue and Sansom, 2002; Hirasawa and Kuratani, 2015), although recent paleontological data has challenged this view (Brazeau et al., 2020). Also known to mineralize their matrix are the chondrocytes, not only at the ossification front of endochondral bone growth (in the case of hyaline cartilage), but also in stable forms of mineralized cartilage such as fibrocartilages and other forms of cartilage displaying striking similarities to bony tissues (Beresford, 1981; Dyment et al., 2015; Paul et al., 2016; Pears et al., 2020; Berio et al., 2021). Even though both perichondral bones and cartilaginous tissues displayed mineralization in the earliest forms of mineralized internal skeletons (Ørvig, 1951; Min and Janvier, 1998; Donoghue et al., 2006; Johanson et al., 2010, 2012; Pears et al., 2020), mineralizing cartilages have been understudied from a genetic and evolutionary perspective in extant vertebrates. A better understanding of the genetic underpinning of the mineralizing chondrocytes is therefore necessary to understand the early steps of the evolution of endoskeletal mineralization in vertebrates.
The evolution of vertebrate endoskeletal mineralization has been discussed in the light of the two rounds of whole-genome duplication (2Rs). These duplications occurred before the diversification of extant jawed vertebrates (Nakatani et al., 2021) and generated gene families with diverging gene functions which may have produced the genetic toolkit required for the cellular ability to mineralize an extracellular matrix (Zhang and Cohn, 2008). The evolution of the SPARC/SPARC-L/SCPP gene family has been of great interest in this perspective (Kawasaki and Weiss, 2003; Kawasaki et al., 2005; Kawasaki, 2009; Bertrand et al., 2013; Enault et al., 2018), and is summarized in Figure 1. SPARC-L and SPARC are two paralogues having originated from the 2Rs (Kawasaki and Weiss, 2003; Kawasaki et al., 2005; Kawasaki, 2009; Bertrand et al., 2013; Enault et al., 2018). In bony fishes, independent local duplications at the SPARC-L locus generated SPARC-L1 and SPARC-L2 and a variable number of tandemly located genes coding for Secretory Calcium-binding PhosphoProteins (SCPPs) that have evolved rapidly since their origin (see Supplementary Figure S1 and Kawasaki and Weiss, 2003; Kawasaki et al., 2005; Enault et al., 2018). Hence outside of amniotes, homology relationships between SCPP duplicates are obscured by independent gene gains and losses together with a high rate of sequence divergence (Kawasaki, 2009). No SCPP genes have been identified in cartilaginous fish genomes, making the chondrichthyan SPARC-L gene the single orthologue to all SCPP genes of bony vertebrates (see Figure 1, Supplementary Figure S1 and Ryll et al., 2014; Venkatesh et al., 2014; Enault et al., 2018).
FIGURE 1. A simplified evolutionary scenario for the SPARC/SPARC-L/SCPP family. Vertebrate-specific whole genome duplications produced the ancestral SPARC (S) and SPARC-L (L) paralogues. These loci were not overtly altered in the chondrichthyan lineage as both genes are clearly identifiable in sharks. In the osteichthyan lineage, local duplications at the SPARC-L locus produced SPARC-L1 (L1), SPARC-L2 (L2) and the SCPP members (triangles). Triangles in different orientations symbolize the fact that SCPP genes are subject to independent local duplications events and a high rate of evolutionary divergence, hindering homology relationships. SPARC-L2 was independently lost in tetrapods and teleosts and SPARC-L1 was also lost in amphibians. See text for details and references.
The SPARC gene (Secreted Protein Acidic and Rich in Cysteine, formerly coined Osteonectin) encodes a matricellular protein which is one of the most abundant non-collagenous matrix proteins in mammalian and teleost bone (Schreiweis et al., 2007; Kessels et al., 2014). Secreted by osteoblasts, the SPARC protein functions in mineralized tissues by binding both collagen fibrils and calcium, but also by signaling to bone cells (reviewed by Rosset and Bradshaw, 2016). In osteichthyans, the expression of SPARC is evolutionary conserved in osteoblasts as well as in odontoblasts (Holland et al., 1987; Li et al., 2009; Espinoza et al., 2010; Enault et al., 2018). In chondrichthyans having secondarily lost the bone tissue (and the osteoblast cell type), SPARC is highly expressed in odontoblasts (Enault et al., 2018). The single SPARC-L gene in cartilaginous fishes is expressed in enameloid secreting cells in teeth and scales of the catshark Scyliorhinus canicula (Enault et al., 2018). In osteichthyans it seems that SPARC-L1 and SPARC-L2 are not specifically expressed nor functionally required in the skeleton (McKinnon et al., 2000; Bertrand et al., 2013). In addition, SPARC-L2 was independently lost in tetrapods and teleosts, and SPARC-L1 was also lost in amphibians (see Figure 1 and Kawasaki et al., 2007; Bertrand et al., 2013; Enault et al., 2018), suggesting that these two genes are functionally dispensable. Rather, in osteichthyans, SCPP family members are key players of skeletal mineralization. Within amniote SCPP genes, Bone sialoprotein (BSP), Osteopontin (OPN or SPP1) and Dentin matrix protein 1 (DMP1) are strongly expressed by osteoblasts and their protein products are stored in the mineral phase of bone tissue (Ustriyana et al., 2021). Most members of this family are also expressed and functional during tooth development in mammals (either in the production of enamel or/and dentin, reviewed by Nikoloudaki, 2021). In the clawed frog Xenopus tropicalis and the zebrafish Danio rerio the expression of distinct SCPP members has been reported in ameloblasts, odontoblasts, and osteoblasts (Kawasaki et al., 2005; Kawasaki, 2009; Espinoza et al., 2010; Enault et al., 2018). Overall, our knowledge of the evolution of the expression of SPARC, SPARC-L and the SCPP members during cartilage mineralization remains limited, and, in this study, we examined the expression of these genes during endoskeletal development in Xenopus tropicalis and Scyliorhinus canicula.
Lesser spotted catshark (Scyliorhinus canicula) embryos were maintained at 17°C at the University of Montpellier, France, until they reached development stage 32 (Ballard et al., 1993; Maxwell et al., 2008). Embryos were taken out of their eggshell, anesthetized and subsequently euthanized by overdose of MS-222 (Sigma) following European animal-care specifications. As substantial growth occurs during stage 32, each individual was measured before fixation and classified into early, intermediate and late stages whose body length measured respectively 5.3, 6.6, and 8.5 cm for histological analyses, and respectively 5.0, 6.3, and 7.9 cm for the Alizarin red S and in situ hybridization procedures. Abdominal vertebral portions were fixed 48 h in PFA 4% in PBS 1× at 4°C and were subsequently transferred in ethanol and stored at − 20°C until needed.
Adult Xenopus tropicalis were maintained following standard protocols established for this species, at the University of Concepcion. Embryos and tadpoles were raised after natural mating and staged according to the Nieuwkoop and Faber developmental table (Nieuwkoop and Faber, 1967). Anesthesia of tadpoles was performed with a MS-222 (Sigma) solution at 2 mg/ml and each specimen was subsequently decapitated in agreement with international bioethical recommendations (Close et al., 1996; Ramlochansingh et al., 2014).
Dissected organs of both species were embedded in paraffin to generate 7 μm-thick histological sections that were stained with standard protocols [eosin, hematoxylin and safran reaction for catshark (RHEM platform at IRCM, Montpellier); hematoxylin and chromotrope 2R (C3143 Sigma) for frog sections]. The von Kossa protocol was used on paraffin sections of Xenopus tropicalis to detect calcium on tissue sections (#10241, Diapath, Italy) following the manufacturer’s instruction. Briefly, the von Kossa method is based on the transformation of calcium ions, bound to phosphates, into silver ions brought by a solution of silver nitrate.
Spotted catshark alizarin red S and in situ hybridizations were performed on serial, 14 μm thick cryostat sections, cut transversal in the body trunk, at the level of the pectoral fins. Parts of the specimens that were not used for this study were conserved in ethanol at − 20°C for further studies on gene expression. Alizarin red S staining was used to detect calcium deposits with a single bath of 0.05% Alizarin Red S (Sigma) in KOH 0.5%, 5 min, before mounting in Mowiol. All slides generated with catshark samples were scanned on a Hamamatsu nanozoomer.
All probes and in situ hybridization procedures used here with Scyliorhinus canicula and Xenopus tropicalis were previously described (Espinoza et al., 2010; Enault et al., 2018), except for the frog SCPPA2 gene (GenBank EU642617) for which a 968 bp product was amplified and cloned into pBluescript using the following primers (5′ to 3′) Forward- GAG TCA TAC TAC AGG ACC TGC, Reverse-CAT GCA ACT CAG CCA AAG CT.
The catshark vertebral tissue mineralizes at the level of the neural arches and of the vertebral body (Enault et al., 2015). In the neural arches, mineralization occurs at two juxtaposed sites: the matrix of the most peripheral chondrocytes and the fibrous perichondrial tissue surrounding each neural arch (Figures 2A–H). In the neural arch peripheral cartilage, a faint Alizarin red-positive signal is observable in early stage 32 embryos (Figures 2A,B,E,F), and becomes more intense in intermediate and late stage 32 embryos (Figures 2C,D,G,H). Note that neural arch mineralization never extends to the center of the cartilaginous scaffold (Figures 2A–H and Berio et al., 2021). In addition, the fibrous perichondrial tissue surrounding each neural arch displays a robust mineralization in intermediate and late stage 32 embryos. In the vertebral body, a mineralization ring appears in the cartilage surrounding the notochord of intermediate stage 32 embryos and becomes more mineralized in late stage 32 embryos (Figures 2F–H).
FIGURE 2. Histology, mineralization dynamics and SPARC and SPARC-L expression pattern in the vertebrae and scales of the small-spotted catshark Scyliorhinus canicula. (A–D) Hematoxylin-Eosin Safran (HES) histological staining on transverse sections at the level of thoracic vertebrae: A, general view with location of the neural tube (nt) and notochord (nc); B-D, closer views on vertebral tissues as boxed in A, with identification of the fibrous perichondrium (fp), unmineralized cartilage (uc) and peripheral chondrocytes (pc) of the neural arch (na), as well as the unmineralized cartilage (uc) and fibrous cartilage (fc) of the vertebral body (vb); insets in B-D, closer view of the dorsal scales as boxed in A, indicating the location of the epithelial (e) and mesenchymal (m) compartments of scale buds. Stage 32 embryos were staged according to their total length into “early,” “intermediate,” and “late” categories as described in the Material and Method section. (E) Periodic Acid Schiff-Alcian Blue (PAS-BA) histological staining of a section consecutive to A: BA (blue) stains the acid glycosaminoglycans of the hyaline cartilage and PAS (pink) stains the fibrous content of the perichondrium. (F–H) Alizarin red S staining locates calcium deposits in mineralizing matrices [of the peripheral chondrocytes (pc), fibrous perichondrium (fp) or fibrous cartilage (fc), and scale enameloid/dentin], in embryos of similar total length as A-D. (I–L) SPARC gene expression patterns, for sections that are consecutive to those shown in (F–H) respectively. (M–P) SPARC-L gene expression patterns for sections that are consecutive to those shown in (F–H) respectively. Scales represent 200 μm, except in scale insets where they represent 50 µm.
The expression of the SPARC gene was detected in the neural tube and several connective tissues such as the dermis and perimysium at all tested developmental stages (Figures 2I–L). We report three major sites of SPARC expression in the Sc developing vertebrae: the neural arch chondrocytes, the neural arch fibrous perichondrium, and the vertebral body. In the neural arches of early stage 32 embryos, SPARC expression localizes to peripheral chondrocytes (i.e., specifically to the mineralizing cartilage) and to the cells of the fibrous perichondrium (Figures 2I,J). In intermediate stage 32 embryos SPARC expression extends to most chondrocytes of the neural arches (Figure 2K). Cells of the mineralized fibrous perichondrial tissue surrounding the neural arches also express SPARC in intermediate and late stage 32 embryos (Figures 2J,K). In the vertebral body from early and intermediate stage 32 embryos, SPARC is detected as a ring of expression in chondrocytes surrounding the notochord (Figures 2I–K). Our results on late stage 32 embryos show little gene expression of SPARC in the vertebral tissues, as only a faint signal was observed in some chondrocytes, (Figure 2L), revealing that the expression of this gene is dynamic and transient in relation to the mineralization processes. We had previously shown that SPARC is expressed in developing scales (Enault et al., 2018), and the expected signal in odontoblasts presents on the same section strongly argues against a possible technical problem for the detection method in late stage 32 embryos (Figure 2L, inset).
On the other hand, the expression of SPARC-L could not be detected in any endoskeletal tissues, while its expression in the epithelium (i.e., the ameloblast layer) of developing and mineralized scales was observable at all stages (Figures 2M–P), as expected (Enault et al., 2018).
We examined gene expression in NF58 Xt limbs because at this stage hypertrophic cartilage is in its most mature state and becomes eliminated and replaced by bone marrow at the diaphysis (Figure 3A). von Kossa staining showed an intense signal in the bone matrix and revealed that the Xt hypertrophic cartilage does not mineralize at the diaphysis of NF58 femoral bones (Figure 3B). SPARC transcripts were specifically detected in osteocytes and in osteoblasts of the periosteum and endosteum, but not in the cartilage (Figures 3C,D). A similar situation was observed for BSP (Figure 3E). DMP1 was detected in osteocytes as well as in some chondrocytes of the diaphysis (Figure 3F). Transcripts from the SCPPA2 gene were detected in osteocytes and some osteoblasts, and in many chondrocytes located at the cartilage to bone marrow transition and in the vicinity of the bone matrix of the diaphysis (Figure 3G) and of the epiphysis (Figure 3G′).
FIGURE 3. SPARC and SCPP gene expression in Xenopus tropicalis stage NF58 hindlimbs. Longitudinal sections of Xenopus tropicalis stage NF58 femoral bones were subjected to Hematoxylin-Eosin staining (A), von Kossa staining (B), or in situ hybridization using a negative control [SPARC sense probe, (C)] or an antisense probe for SPARC (D), BSP (E), DMP1 (F), and SCPPA2 (G, G′). Pictures show the diaphysis in (A–G) and the epiphysis in (G′). White and black arrowheads show in situ hybridization signal in osteoblasts and chondrocytes, respectively. Abbreviations: BM, bone marrow, Oc osteocytes showing in situ hybridization signal. Scale bar in (A) represents 100 µm and applies to all panels. The asterisk shows an artifact due to the cartilage which teared apart and contracted in this region of the section.
Stage NF58 vertebrae (Figure 4A) were subjected to von Kossa staining, revealing cartilage mineralization in the dorsal region of the neural arches (Figures 4B,C), as well as in the ventral region located between the neural tube and the notochord (Figures 4B′,C′), in agreement with previous observations performed with Alizarin red S (Enault et al., 2015). We found that each of the examined genes displays a distinctive expression pattern. SPARC is specifically expressed in osteoblasts of the dorsal neural arches and of the ventral region, but not in chondrocytes (Figures 4D,D′,E,E′). BSP is expressed in osteoblasts and chondrocytes of both regions, albeit its expression is much stronger in the cartilage of the ventral vertebrae (Figures 4F,F′). DMP1 is expressed in osteocytes and in chondrocytes located close to the bone matrix of the dorsal neural arch, but is not expressed in the ventral vertebra at this stage (Figures 4G,G′). SCPPA2 is moderately expressed in some osteocytes and osteoblasts of the dorsal neural arch, and very strongly in chondrocytes of the mineralizing cartilage of both vertebral regions (Figures 4H,H′).
FIGURE 4. SPARC and SCPP gene expression in Xenopus tropicalis stage NF58 vertebrae. Transversal sections of Xenopus tropicalis stage NF58 vertebrae were subjected to Hematoxylin-Eosin staining (A,B,B′), von Kossa staining (C,C′), or in situ hybridization using a negative control [SPARC sense probe, (D,D′)] or an antisense probe for SPARC (E,E′), BSP (F,F′), DMP1 (G, G′), and SCPPA2 (H,H′). Pictures are orientated with dorsal to the top and show the whole vertebrae (A), the neural arch (B–H) or the vertebral body (B′–H′). White and black arrowheads show in situ hybridization signal in osteoblasts and chondrocytes, respectively. Abbreviations: uc, unmineralized cartilage, Oc osteocytes showing in situ hybridization signal. Scale bars: (A), 100 μm; (B), 100 µm in (B–D′); (E), 50 µm in (E–H′).
Our findings in Xt reveal an evolutionary conservation of the cartilaginous expression of the SCPP genes in tetrapods. Indeed, similarly to the situation in Xt, SPARC is not expressed in mouse chondrocytes (Holland et al., 1987). Rather, SCPP genes such as DMP1 and BSP are expressed and required for mouse cartilage development (Chen et al., 1991; Ye et al., 2005; Bouleftour et al., 2014; Fujikawa et al., 2015). As indicated by other studies (Yagami et al., 1999; Bandyopadhyay et al., 2008), gene expression in cartilaginous elements can be subdivided in two distinct domains which we will use to discuss our results. First, SCPP genes become activated at late stages of hypertrophy, when the cartilage matrix becomes replaced by bone marrow at the mammalian diaphysis (Chen et al., 1991; Fujikawa et al., 2015). Likewise, in Xt, DMP1 is exclusively expressed at the diaphysis (Figure 3F), and SCPPA2 exhibits a much stronger expression at the diaphysis than the epiphysis region (Figures 3G,G′). A similar situation is observed at the level of the Xt vertebrae, where the expression of SCPP genes tightly correlates with cartilage maturation and mineralization in the neural arch (for BSP, DMP1, and SCPPA2) as well as in the ventral vertebral region (for BSP and SCPPA2). Second, the SCPP genes harbor a stronger expression in the non-mineralized peripheral cartilage, as observed in mouse for osteopontin (Heilig et al., 2016) and DMP1 (Fujikawa et al., 2015). This situation is similar to the expression of Xt SSCP genes in chondrocytes located in the vicinity of the bone matrix in long bones and vertebrae (Figures 3, 4). Such a peripheral cartilage domain expresses specific genes, as reported in chick (Bandyopadhyay et al., 2008), and undergoes ectopic mineralization in mutant mouse animals for the Mgp (Marulanda et al., 2017) and Trps1 (Napierala et al., 2008) genes. In summary, SCPP genes from frog and mouse are expressed in the mature cartilage of the diaphysis and neural arches, as well as in peripherally located chondrocytes.
Available expression analyses did not report any cartilaginous expression for SCPP genes in teleosts (Kawasaki et al., 2005; Kawasaki, 2009; Weigele et al., 2015). Rather, the expression of the SPARC gene has been associated to cartilage development in zebrafish, gilthead seabream and the cichlid mouth breeder (Estevao et al., 2005; Redruello et al., 2005; Rotllant et al., 2008; Estevao et al., 2011; Weigele et al., 2015), albeit not in medaka, at least at the examined stages (Renn et al., 2006). Hence the reported cartilaginous expression patterns in teleosts (SPARC positive and SCPP negative) are opposite to the tetrapod situation (SPARC negative and SCPP positive), which might be related to drastic difference in the mode of endochondral ossification between these two groups (Cervantes-Diaz et al., 2017). In this respect, our data in the chondrichthyan representative Sc is instrumental to understand the evolution of the expression of these genes in the jawed vertebrate endoskeleton.
As no SCPP genes have been reported in chondrichthyan genomes to date, we focused on the evolutionarily related gene SPARC-L (Kawasaki and Weiss, 2003; Bertrand et al., 2013; Venkatesh et al., 2014; Enault et al., 2018). Our finding that Sc SPARC-L is not expressed in the vertebral cartilage is further confirmed by the robust and expected Sc SPARC-L expression in the odontodes present on the same sections and serving as convenient internal positive controls (Enault et al., 2018). By contrast, SPARC expression clearly co-localizes with sites of vertebral mineralization. In the neural arches of early stage 32 embryos, SPARC is restricted to the mineralizing peripheral cartilage matrix, thereby paralleling the expression of SCPP genes in frog (Figures 2, 4) and mouse (Fujikawa et al., 2015; Heilig et al., 2016). Hence, we uncover a novel evolutionarily conserved cartilage domain, as defined by peripherally located chondrocytes expressing SPARC in chondrichthyans and the SCPP genes in tetrapods. One difference is that this domain mineralizes in chondricthyans, but not in tetrapods. We propose that dosage variations between pro- and anti-mineralizing proteins might explain evolutionary differences between vertebrate lineages, as might be the case for instance for MGP which is a well-conserved cartilage mineralization inhibitor (Yagami et al., 1999; Espinoza et al., 2010; Viegas et al., 2013; Marulanda et al., 2017; Leurs et al., 2021). By examining the centrum of Sc specimens from different developmental stages we show that a ring of SPARC expression is already present in Alizarin red-negative early stage 32 embryos, suggesting that the presence of the SPARC protein plays a crucial role in the initiation of mineralization. The functional interaction between the mammalian SPARC and collagen 1 proteins is important for mineralization (Termine et al., 1981). However, as the shark chondrocytes embedded within a mineralizing cartilage matrix neither expresses collagen 1a1 nor collagen 1a2 (Enault et al., 2015), Sc SPARC function might be related to other aspects of matrix mineralization, such as its interaction with calcium and hydroxyapatite crystals (Engel et al., 1987; Maurer et al., 1995; Fujisawa et al., 1996).
Our data suggest that chondrichthyans are more similar to teleosts than to tetrapods because the Sc mineralizing cartilage is SPARC positive and SPARC-L negative. Here, we propose two evolutionary models to explain these divergent expression patterns (see Figure 5 and Supplementary Figure S1). Both models are based on the assumption that SPARC, SPARC-L, and SCPP share some level of functional redundancy, at least during chondrogenesis, as suggested by the fact that both SPARC and SCPP proteins are extracellular transglutaminase substrates (Aeschlimann et al., 1995; Forsprecher et al., 2011) and bind calcium ions (Engel et al., 1987; Chen et al., 1992; Maurer et al., 1995; Klaning et al., 2014). The “multiple losses” model is reminiscent of the Duplication Degeneration Complementation (DDC) phenomenon (Force et al., 1999) and involves at least three independent changes which abrogated the cartilaginous expression of SPARC, of SPARC-L or of the SCPP genes in distinct lineages (Figure 5, left panel). The “expression swap” model involves two changes and implies that, in the tetrapod lineage, cartilaginous expression was gained for the SCPP genes and lost for SPARC (Figure 5, right panel). While the SPARC/SPARC-L/SCPP family exhibit a unique evolutionary trajectory (Bertrand et al., 2013; Enault et al., 2018), the “expression swap” model would be similar to the dynamic expression turnover observed between members of the Keratin, Vitellogenin, and Globin vertebrate families (Finn et al., 2009; Vandebergh and Bossuyt, 2012; Opazo et al., 2015). Both scenarios imply regulatory changes that switched off (“multiple losses model”) or on (“expression swap model”) several SCPP genes (Figure 5). From a regulatory perspective, the idea of coordinated change in the expression of tandemly located SCPP genes is consistent with the fact that BSP and DMP1 are included within the same topological association domain in human chromatin (Krietenstein et al., 2020), and that multiple genes can be co-regulated by the same enhancer (reviewed in Zheng and Xie, 2019). To the best of our knowledge, current experimental evidence is not sufficient to discriminate between the two models shown in Figure 5. Hence, a detailed analysis of the expression and regulation of SPARC/SPARC-L/SCPP genes in chondrocyte from a broader array of chondrichthyan and osteichthyan species will be required to shed light on the genetic mechanisms involved in the evolution of cartilage mineralization that originated deep in the vertebrate lineage (Min and Janvier, 1998; Donoghue et al., 2006; Johanson et al., 2010; 2012).
FIGURE 5. A model for the evolution of the SPARC/SPARC-L/SCPP cartilaginous expression in jawed vertebrates. Evolutionary changes are polarized onto vertebrate cladograms. According to the “multiple losses” model (left), the mineralizing cartilage of the jawed vertebrate last common ancestor expressed both the SPARC and SPARC-L ancestral genes (A). This expression was inherited by the SCPP members when they evolved through SPARC-L local duplications. Expression losses occurred at least three times independently in distinct lineages: SPARC-L expression was lost in chondrichthyans (B), SCPP expression was lost in teleosts (C) and SPARC expression was lost in tetrapods (D). According to the “expression swap” model (right), the mineralizing cartilage of the jawed vertebrate last common ancestor only expressed SPARC (E), a situation which remained unchanged in the chondrichthyan and teleost lineages. However, the tetrapod lineage experienced drastic regulatory changes at both loci, leading to the activation of the SCPP genes (F) and the repression of SPARC (G) in chondrocytes.
The raw data supporting the conclusion of this article will be made available by the authors, without undue reservation.
Handling of small-spotted catshark embryos followed all institutional, national, and international guidelines [European Communities Council Directive of September 22, 2010 (2010/63/UE)]: no further approval by an ethics committee was necessary as the biological material is embryonic and no live experimental procedures were carried out. The Ethics Committee of the University of Concepcion (Concepcion, Chile) approved all experimental procedures carried out on Xenopus tropicalis tadpoles during this study, which were performed following the guidelines outlined in the Biosafety and Bioethics Manual of the National Commission of Scientific and Technological Research (CONICYT, Chilean Government).
AR and DM performed the histological analyses and in situ hybridization procedures in Xenopus tropicalis. NL and MD-T performed the histological analyses and in situ hybridization procedures in Scyliorhinus canicula. SM and MD-T wrote the manuscript and prepared the figures. All authors analyzed and interpreted the data and read and approved the manuscript.
We acknowledge the MRI platform, member of the national infrastructure France-BioImaging supported by the French National Research Agency (ANR-10-INBS-04, «Investments for the future»), the labex CEMEB (ANR-10-LABX-0004), and NUMEV (ANR-10-LABX-0020). We also wish to acknowledge the “Réseau d’Histologie Expérimentale de Montpellier”—RHEM facility supported by the SIRIC Montpellier Cancer (Grant INCa_Inserm_DGOS_12553), the european regional development foundation and the occitanian region (FEDER-FSE 2014-2020 Languedoc Roussillon) for processing our animal tissues, histology techniques, and expertise. This study was partially funded by the IRP (LIA) MAST (CNRS). Fondecyt 1190926 to SM. AR and DM were ANID fellows 21170393 and 21160069 respectively.
The authors declare that the research was conducted in the absence of any commercial or financial relationships that could be construed as a potential conflict of interest.
All claims expressed in this article are solely those of the authors and do not necessarily represent those of their affiliated organizations, or those of the publisher, the editors and the reviewers. Any product that may be evaluated in this article, or claim that may be made by its manufacturer, is not guaranteed or endorsed by the publisher.
We thank Stéphanie Ventéo and Camille Martinand-Mari for their help with the biological material and in situ hybridization technique.
The Supplementary Material for this article can be found online at: https://www.frontiersin.org/articles/10.3389/fgene.2021.788346/full#supplementary-material
Aeschlimann, D., Kaupp, O., and Paulsson, M. (1995). Transglutaminase-catalyzed Matrix Cross-Linking in Differentiating Cartilage: Identification of Osteonectin as a Major Glutaminyl Substrate. J. Cel Biol 129, 881–892. doi:10.1083/jcb.129.3.881
Ballard, W., Mellinger, J., and Lechenault, H. (1993). A Series of Stages for Development of Scyliorhinus canicula the Lesser Spotted Dogfish (Chondrichthyes: Scyliorhinidae). J. Exp. Zool 267, 1–43. doi:10.1002/jez.1402670309
Bandyopadhyay, A., Kubilus, J. K., Crochiere, M. L., Linsenmayer, T. F., and Tabin, C. J. (2008). Identification of Unique Molecular Subdomains in the Perichondrium and Periosteum and Their Role in Regulating Gene Expression in the Underlying Chondrocytes. Develop. Biol. 321, 162–174. doi:10.1016/j.ydbio.2008.06.012
Beresford, W. A. (1981). Chondroid Bone, Secondary Cartilage, and Metaplasia. The American Journal of Surgical Pathology 5, 405.
Berio, F., Broyon, M., Enault, S., Pirot, N., López-Romero, F. A., and Debiais-Thibaud, M. (2021). Diversity and Evolution of Mineralized Skeletal Tissues in Chondrichthyans. Front. Ecol. Evol. 9, 1–19. doi:10.3389/fevo.2021.660767
Bertrand, S., Fuentealba, J., Aze, A., Hudson, C., Yasuo, H., Torrejon, M., et al. (2013). A Dynamic History of Gene Duplications and Losses Characterizes the Evolution of the SPARC Family in Eumetazoans. Proc. R. Soc. B. 280, 20122963. doi:10.1098/rspb.2012.2963
Bouleftour, W., Boudiffa, M., Wade-Gueye, N. M., Bouët, G., Cardelli, M., Laroche, N., et al. (2014). Skeletal Development of Mice Lacking Bone Sialoprotein (BSP) - Impairment of Long Bone Growth and Progressive Establishment of High Trabecular Bone Mass. PLoS One 9, e95144. doi:10.1371/journal.pone.0095144
Brazeau, M. D., Giles, S., Dearden, R. P., Jerve, A., Ariunchimeg, Y., Zorig, E., et al. (2020). Endochondral Bone in an Early Devonian 'placoderm' from Mongolia. Nat. Ecol. Evol. 4, 1477–1484. doi:10.1038/s41559-020-01290-2
Cervantes-Diaz, F., Contreras, P., and Marcellini, S. (2017). Evolutionary Origin of Endochondral Ossification: the Transdifferentiation Hypothesis. Dev. Genes Evol. 227, 121–127. doi:10.1007/s00427-016-0567-y
Chen, J., Shapiro, H. S., Wrana, J. L., Reimers, S., Heersche, J. N. M., and Sodek, J. (1991). Localization of Bone Sialoprotein (BSP) Expression to Sites of Mineralized Tissue Formation in Fetal Rat Tissues by In Situ Hybridization. Matrix 11, 133–143. doi:10.1016/s0934-8832(11)80217-9
Chen, Y., Bal, B. S., and Gorski, J. P. (1992). Calcium and Collagen Binding Properties of Osteopontin, Bone Sialoprotein, and Bone Acidic Glycoprotein-75 from Bone. J. Biol. Chem. 267, 24871–24878. doi:10.1016/s0021-9258(18)35844-7
Close, B., Banister, K., Baumans, V., Bernoth, E.-M., Bromage, N., Bunyan, J., et al. (1996). Recommendations for Euthanasia of Experimental Animals: Part 1. Lab. Anim. 30, 293–316. doi:10.1258/002367796780739871
Donoghue, P. C. J., Sansom, I. J., and Downs, J. P. (2006). Early Evolution of Vertebrate Skeletal Tissues and Cellular Interactions, and the Canalization of Skeletal Development. J. Exp. Zool. 306B, 278–294. doi:10.1002/jez.b.21090
Donoghue, P. C. J., and Sansom, I. J. (2002). Origin and Early Evolution of Vertebrate Skeletonization. Microsc. Res. Tech. 59, 352–372. doi:10.1002/jemt.10217
Dyment, N. A., Breidenbach, A. P., Schwartz, A. G., Russell, R. P., Aschbacher-Smith, L., Liu, H., et al. (2015). Gdf5 Progenitors Give Rise to Fibrocartilage Cells that Mineralize via Hedgehog Signaling to Form the Zonal Enthesis. Develop. Biol. 405, 96–107. doi:10.1016/j.ydbio.2015.06.020
Enault, S., Muñoz, D. N., Silva, W. T. A. F., Borday-Birraux, V., Bonade, M., Oulion, S., et al. (2015). Molecular Footprinting of Skeletal Tissues in the Catshark Scyliorhinus canicula and the Clawed Frog Xenopus Tropicalis Identifies Conserved and Derived Features of Vertebrate Calcification. Front. Genet. 6, 283. doi:10.3389/fgene.2015.00283
Enault, S., Muñoz, D., Simion, P., Ventéo, S., Sire, J.-Y., Marcellini, S., et al. (2018). Evolution of Dental Tissue Mineralization: an Analysis of the Jawed Vertebrate SPARC and SPARC-L Families. BMC Evol. Biol. 18, 127. doi:10.1186/s12862-018-1241-y
Engel, J., Taylor, W., Paulsson, M., Sage, H., and Hogan, B. (1987). Calcium Binding Domains and Calcium-Induced Conformational Transition of SPARC/BM-40/osteonectin, an Extracellular Glycoprotein Expressed in Mineralized and Nonmineralized Tissues. Biochemistry 26, 6958–6965. doi:10.1021/bi00396a015
Espinoza, J., Sanchez, M., Sanchez, A., Hanna, P., Torrejon, M., Buisine, N., et al. (2010). Two Families of Xenopus Tropicalis Skeletal Genes Display Well-Conserved Expression Patterns with Mammals in Spite of Their Highly Divergent Regulatory Regions. Evol. Dev. 12, 541–551. doi:10.1111/j.1525-142X.2010.00440.x
Estêvão, M. D., Redruello, B., Canario, A. V. M., and Power, D. M. (2005). Ontogeny of Osteonectin Expression in Embryos and Larvae of Sea Bream (Sparus Auratus). Gen. Comp. Endocrinol. 142, 155–162. doi:10.1016/j.ygcen.2004.11.018
Estêvão, M. D., Silva, N., Redruello, B., Costa, R., Gregório, S., Canário, A. V. M., et al. (2011). Cellular Morphology and Markers of Cartilage and Bone in the marine Teleost Sparus Auratus. Cell Tissue Res 343, 619–635. doi:10.1007/s00441-010-1109-y
Finn, R., Kolarevic, J., Kongshaug, H., and Nilsen, F. (2009). Evolution and Differential Expression of a Vertebrate Vitellogenin Gene Cluster. BMC Evol. Biol. 9, 2. doi:10.1186/1471-2148-9-2
Force, A., Lynch, M., Pickett, F. B., Amores, A., Yan, Y.-l., and Postlethwait, J. (1999). Preservation of Duplicate Genes by Complementary, Degenerative Mutations. Genetics 151, 1531–1545. doi:10.1093/genetics/151.4.1531
Forsprecher, J., Wang, Z., Goldberg, H. A., and Kaartinen, M. T. (2011). Transglutaminase-mediated Oligomerization Promotes Osteoblast Adhesive Properties of Osteopontin and Bone Sialoprotein. Cell Adhes. Migration 5, 65–72. doi:10.4161/cam.5.1.13369
Fujikawa, K., Yokohama-Tamaki, T., Morita, T., Baba, O., Qin, C., and Shibata, S. (2015). An In Situ Hybridization Study of Perlecan, DMP1, and MEPE in Developing Condylar Cartilage of the Fetal Mouse Mandible and Limb Bud Cartilage. Eur. J. Histochem. 59, 2553. doi:10.4081/ejh.2015.2553
Fujisawa, R., Wada, Y., Nodasaka, Y., and Kuboki, Y. (1996). Acidic Amino Acid-Rich Sequences as Binding Sites of Osteonectin to Hydroxyapatite Crystals. Biochim. Biophys. Acta (Bba) - Protein Struct. Mol. Enzymol. 1292, 53–60. doi:10.1016/0167-4838(95)00190-5
Heilig, J., Paulsson, M., and Zaucke, F. (2016). Insulin-like Growth Factor 1 Receptor (IGF1R) Signaling Regulates Osterix Expression and Cartilage Matrix Mineralization during Endochondral Ossification. Bone 83, 48–57. doi:10.1016/j.bone.2015.10.007
Hirasawa, T., and Kuratani, S. (2015). Evolution of the Vertebrate Skeleton: Morphology, Embryology, and Development. Zoolog. Lett 1. doi:10.1186/s40851-014-0007-7
Holland, P. W., Harper, S. J., Mcvey, J. H., and Hogan, B. L. (1987). In Vivo expression of mRNA for the Ca++-Binding Protein SPARC (Osteonectin) Revealed by In Situ Hybridization. J. Cel Biol 105, 473–482. doi:10.1083/jcb.105.1.473
Johanson, Z., Kearsley, A., Den Blaauwen, J., Newman, M., and Smith, M. M. (2010). No Bones about it: An Enigmatic Devonian Fossil Reveals a New Skeletal Framework-A Potential Role of Loss of Gene Regulation. Semin. Cel Develop. Biol. 21, 414–423. doi:10.1016/j.semcdb.2009.10.011
Johanson, Z., Kearsley, A., Den Blaauwen, J., Newman, M., and Smith, M. M. (2012). Ontogenetic Development of an Exceptionally Preserved Devonian Cartilaginous Skeleton. J. Exp. Zool. 318B, 50–58. doi:10.1002/jez.b.21441
Kawasaki, K., Buchanan, A. V., and Weiss, K. M. (2007). Gene Duplication and the Evolution of Vertebrate Skeletal Mineralization. Cells Tissues Organs 186, 7–24. doi:10.1159/000102678
Kawasaki, K., Suzuki, T., and Weiss, K. M. (2005). Phenogenetic Drift in Evolution: the Changing Genetic Basis of Vertebrate Teeth. Proc. Natl. Acad. Sci. 102, 18063–18068. doi:10.1073/pnas.0509263102
Kawasaki, K. (2009). The SCPP Gene Repertoire in Bony Vertebrates and Graded Differences in Mineralized Tissues. Dev. Genes Evol. 219, 147–157. doi:10.1007/s00427-009-0276-x
Kawasaki, K., and Weiss, K. M. (2003). Mineralized Tissue and Vertebrate Evolution: the Secretory Calcium-Binding Phosphoprotein Gene Cluster. Proc. Natl. Acad. Sci. 100, 4060–4065. doi:10.1073/pnas.0638023100
Kessels, M. Y., Huitema, L. F. A., Boeren, S., Kranenbarg, S., Schulte-Merker, S., Van Leeuwen, J. L., et al. (2014). Proteomics Analysis of the Zebrafish Skeletal Extracellular Matrix. PLoS One 9, e90568. doi:10.1371/journal.pone.0090568
Kläning, E., Christensen, B., Sørensen, E. S., Vorup-Jensen, T., and Jensen, J. K. (2014). Osteopontin Binds Multiple Calcium Ions with High Affinity and Independently of Phosphorylation Status. Bone 66, 90–95. doi:10.1016/j.bone.2014.05.020
Krietenstein, N., Abraham, S., Venev, S. V., Abdennur, N., Gibcus, J., Hsieh, T.-H. S., et al. (2020). Ultrastructural Details of Mammalian Chromosome Architecture. Mol. Cel 78, 554–565. e557. doi:10.1016/j.molcel.2020.03.003
Leurs, N., Martinand-Mari, C., Ventéo, S., Haitina, T., and Debiais-Thibaud, M. (2021). Evolution of Matrix Gla and Bone Gla Protein Genes in Jawed Vertebrates. Front. Genet. 12, 620659. doi:10.3389/fgene.2021.620659
Li, N., Felber, K., Elks, P., Croucher, P., and Roehl, H. H. (2009). Tracking Gene Expression during Zebrafish Osteoblast Differentiation. Dev. Dyn. 238, 459–466. doi:10.1002/dvdy.21838
Long, F., and Ornitz, D. M. (2013). Development of the Endochondral Skeleton. Cold Spring Harbor Perspect. Biol. 5, a008334. doi:10.1101/cshperspect.a008334
Marulanda, J., Eimar, H., Mckee, M. D., Berkvens, M., Nelea, V., Roman, H., et al. (2017). Matrix Gla Protein Deficiency Impairs Nasal Septum Growth, Causing Midface Hypoplasia. J. Biol. Chem. 292, 11400–11412. doi:10.1074/jbc.M116.769802
Maurer, P., Hohenadl, C., Hohenester, E., Göhring, W., Timpl, R., and Engel, J. (1995). The C-Terminal Portion of BM-40 (SPARC/osteonectin) Is an Autonomously Folding and Crystallisable Domain that Binds Calcium and Collagen IV. J. Mol. Biol. 253, 347–357. doi:10.1006/jmbi.1995.0557
Maxwell, E. E., Fröbisch, N. B., and Heppleston, A. C. (2008). Variability and Conservation in Late Chondrichthyan Development: Ontogeny of the winter Skate (Leucoraja ocellata). Anat. Rec. 291, 1079–1087. doi:10.1002/ar.20719
Mckinnon, P. J., Mclaughlin, S. K., Kapsetaki, M., and Margolskee, R. F. (2000). Extracellular Matrix-Associated Protein Sc1 Is Not Essential for Mouse Development. Mol. Cel Biol 20, 656–660. doi:10.1128/mcb.20.2.656-660.2000
Min, Z., and Janvier, P. (1998). The Histological Structure of the Endoskeleton in Galeaspids (Galeaspida, Vertebrata). J. Vertebr. Paleontol. 18, 650–654. doi:10.1080/02724634.1998.10011091
Nakatani, Y., Shingate, P., Ravi, V., Pillai, N. E., Prasad, A., Mclysaght, A., et al. (2021). Reconstruction of Proto-Vertebrate, Proto-Cyclostome and Proto-Gnathostome Genomes Provides New Insights into Early Vertebrate Evolution. Nat. Commun. 12, 4489. doi:10.1038/s41467-021-24573-z
Napierala, D., Sam, K., Morello, R., Zheng, Q., Munivez, E., Shivdasani, R. A., et al. (2008). Uncoupling of Chondrocyte Differentiation and Perichondrial Mineralization Underlies the Skeletal Dysplasia in Tricho-Rhino-Phalangeal Syndrome. Hum. Mol. Genet. 17, 2244–2254. doi:10.1093/hmg/ddn125
Nieuwkoop, P. D., and Faber, J. (1967). Normal Table of Xenopus laevis (Daudin). Netherlands: North Holland.
Nikoloudaki, G. (2021). Functions of Matricellular Proteins in Dental Tissues and Their Emerging Roles in Orofacial Tissue Development, Maintenance, and Disease. Ijms 22, 6626. doi:10.3390/ijms22126626
Opazo, J. C., Hoffmann, F. G., Natarajan, C., Witt, C. C., Berenbrink, M., and Storz, J. F. (2015). Gene Turnover in the Avian Globin Gene Families and Evolutionary Changes in Hemoglobin Isoform Expression. Mol. Biol. Evol. 32, 871–887. doi:10.1093/molbev/msu341
Ørvig, T. (1951). Histologic Studies of Placoderms and Fossil Elasmobranchs. I: the Endoskeleton, with Remarks on the Hard Tissues of Lower Vertebrates in General. Arkiv Zool 2.
Paul, S., Schindler, S., Giovannone, D., De Millo Terrazzani, A., Mariani, F. V., and Crump, J. G. (2016). Ihha Induces Hybrid Cartilage-Bone Cells during Zebrafish Jawbone Regeneration. Development 143, 2066–2076. doi:10.1242/dev.131292
Pears, J. B., Johanson, Z., Trinajstic, K., Dean, M. N., and Boisvert, C. A. (2020). Mineralization of the Callorhinchus Vertebral Column (Holocephali; Chondrichthyes). Front. Genet. 11, 571694. doi:10.3389/fgene.2020.571694
Ramlochansingh, C., Branoner, F., Chagnaud, B. P., and Straka, H. (2014). Efficacy of Tricaine Methanesulfonate (MS-222) as an Anesthetic Agent for Blocking Sensory-Motor Responses in Xenopus laevis Tadpoles. PLoS One 9, e101606. doi:10.1371/journal.pone.0101606
Redruello, B., Estêvão, M. D., Rotllant, J., Guerreiro, P. M., Anjos, L. I., Canário, A. V., et al. (2005). Isolation and Characterization of Piscine Osteonectin and Downregulation of its Expression by PTH-Related Protein. J. Bone Miner Res. 20, 682–692. doi:10.1359/JBMR.041201
Renn, J., Schaedel, M., Volff, J.-N., Goerlich, R., Schartl, M., and Winkler, C. (2006). Dynamic Expression of Sparc Precedes Formation of Skeletal Elements in the Medaka (Oryzias latipes). Gene 372, 208–218. doi:10.1016/j.gene.2006.01.011
Rosset, E. M., and Bradshaw, A. D. (2016). SPARC/osteonectin in Mineralized Tissue. Matrix Biol. 52-54, 78–87. doi:10.1016/j.matbio.2016.02.001
Rotllant, J., Liu, D., Yan, Y.-L., Postlethwait, J. H., Westerfield, M., and Du, S.-J. (2008). Sparc (Osteonectin) Functions in Morphogenesis of the Pharyngeal Skeleton and Inner Ear. Matrix Biol. 27, 561–572. doi:10.1016/j.matbio.2008.03.001
Ryll, B., Sanchez, S., Haitina, T., Tafforeau, P., and Ahlberg, P. E. (2014). The Genome of Callorhinchus and the Fossil Record: a New Perspective on SCPP Gene Evolution in Gnathostomes. Evol. Develop. 16, 123–124. doi:10.1111/ede.12071
Schreiweis, M. A., Butler, J. P., Kulkarni, N. H., Knierman, M. D., Higgs, R. E., Halladay, D. L., et al. (2007). A Proteomic Analysis of Adult Rat Bone Reveals the Presence of Cartilage/chondrocyte Markers. J. Cel. Biochem. 101, 466–476. doi:10.1002/jcb.21196
Termine, J. D., Kleinman, H. K., Whitson, S. W., Conn, K. M., Mcgarvey, M. L., and Martin, G. R. (1981). Osteonectin, a Bone-specific Protein Linking mineral to Collagen. Cell 26, 99–105. doi:10.1016/0092-8674(81)90037-4
Tsang, K. Y., Chan, D., and Cheah, K. S. E. (2015). Fate of Growth Plate Hypertrophic Chondrocytes: Death or Lineage Extension? Develop. Growth Differ. 57, 179–192. doi:10.1111/dgd.12203
Ustriyana, P., Schulte, F., Gombedza, F., Gil-Bona, A., Paruchuri, S., Bidlack, F. B., et al. (2021). Spatial Survey of Non-collagenous Proteins in Mineralizing and Non-mineralizing Vertebrate Tissues Ex Vivo. Bone Rep. 14, 100754. doi:10.1016/j.bonr.2021.100754
Vandebergh, W., and Bossuyt, F. (2012). Radiation and Functional Diversification of Alpha Keratins during Early Vertebrate Evolution. Mol. Biol. Evol. 29, 995–1004. doi:10.1093/molbev/msr269
Venkatesh, B., Lee, A. P., Ravi, V., Maurya, A. K., Lian, M. M., Swann, J. B., et al. (2014). Elephant Shark Genome Provides Unique Insights into Gnathostome Evolution. Nature 505, 174–179. doi:10.1038/nature12826
Viegas, C. S. B., Simes, D. C., Williamson, M. K., Cavaco, S., Laizé, V., Price, P. A., et al. (2013). Sturgeon Osteocalcin Shares Structural Features with Matrix Gla Protein. J. Biol. Chem. 288, 27801–27811. doi:10.1074/jbc.M113.450213
Weigele, J., Franz‐Odendaal, T. A., and Hilbig, R. (2015). Expression of SPARC and the Osteopontin‐like Protein during Skeletal Development in the Cichlid Fish Oreochromis mossambicus. Dev. Dyn. 244, 955–972. doi:10.1002/dvdy.24293
Yagami, K., Suh, J.-Y., Enomoto-Iwamoto, M., Koyama, E., Abrams, W. R., Shapiro, I. M., et al. (1999). Matrix GLA Protein Is a Developmental Regulator of Chondrocyte Mineralization and, when Constitutively Expressed, Blocks Endochondral and Intramembranous Ossification in the Limb. J. Cel Biol 147, 1097–1108. doi:10.1083/jcb.147.5.1097
Ye, L., Mishina, Y., Chen, D., Huang, H., Dallas, S. L., Dallas, M. R., et al. (2005). Dmp1-deficient Mice Display Severe Defects in Cartilage Formation Responsible for a Chondrodysplasia-like Phenotype. J. Biol. Chem. 280, 6197–6203. doi:10.1074/jbc.M412911200
Zhang, G., and Cohn, M. J. (2008). Genome Duplication and the Origin of the Vertebrate Skeleton. Curr. Opin. Genet. Develop. 18, 387–393. doi:10.1016/j.gde.2008.07.009
Keywords: SPARC, SPARC-L, SCPP, cartilage mineralization, Xenopus tropicalis, Scyliorhinus canicula, vertebrate evolution
Citation: Romero A, Leurs N, Muñoz D, Debiais-Thibaud M and Marcellini S (2021) Divergent Expression of SPARC, SPARC-L, and SCPP Genes During Jawed Vertebrate Cartilage Mineralization. Front. Genet. 12:788346. doi: 10.3389/fgene.2021.788346
Received: 02 October 2021; Accepted: 10 November 2021;
Published: 25 November 2021.
Edited by:
Claudio Oliveira, São Paulo State University, BrazilReviewed by:
Anthony K. Redmond, Trinity College Dublin, IrelandCopyright © 2021 Romero, Leurs, Muñoz, Debiais-Thibaud and Marcellini. This is an open-access article distributed under the terms of the Creative Commons Attribution License (CC BY). The use, distribution or reproduction in other forums is permitted, provided the original author(s) and the copyright owner(s) are credited and that the original publication in this journal is cited, in accordance with accepted academic practice. No use, distribution or reproduction is permitted which does not comply with these terms.
*Correspondence: Mélanie Debiais-Thibaud, bWVsYW5pZS5kZWJpYWlzLXRoaWJhdWRAdW1vbnRwZWxsaWVyLmZy; Sylvain Marcellini, c21hcmNlbGxpbmlAdWRlYy5jbA==
†These authors have contributed equally to this work
Disclaimer: All claims expressed in this article are solely those of the authors and do not necessarily represent those of their affiliated organizations, or those of the publisher, the editors and the reviewers. Any product that may be evaluated in this article or claim that may be made by its manufacturer is not guaranteed or endorsed by the publisher.
Research integrity at Frontiers
Learn more about the work of our research integrity team to safeguard the quality of each article we publish.