- 1Department of Biological Sciences, Faculty of Science and Engineering, Chuo University, Bunkyo-ku, Tokyo, Japan
- 2Graduate School of Medicine, University of the Ryukyus, Nishihara, Japan
Chemokines, relatively small secreted proteins, are involved in cell migration and function in various biological events, including immunity, morphogenesis, and disease. Due to their nature, chemokines tend to be a target of hijacking of immunity by virus and therefore show an exceptionally high mutation rate. Xenopus laevis is considered an excellent model to investigate the effect of whole-genome duplication for gene family evolution. Because its allotetraploidization occurred around 17–18 million years ago, ancestral subgenomes L and S were well conserved. Based on the gene model of human and diploid frog Xenopus tropicalis, we identified 52 chemokine genes and 26 chemokine receptors in X. laevis. The retention rate of the gene in the X. laevis L and S subgenomes was 96% (45/47) and 68% (32/47), respectively. We conducted molecular phylogenetic analysis and found clear orthologies in all receptor genes but not in the ligand genes, suggesting rapid divergences of the ligand. dN/dS calculation demonstrated that dN/dS ratio greater than one was observed in the four ligand genes, cxcl8b.1.S, cxcl18.S, ccl21.S, and xcl1.L, but nothing in receptor genes. These results revealed that the whole-genome duplication promotes diversification of chemokine ligands in X. laevis while conserving the genes necessary for homeostasis, suggesting that selective pressure also supports a rapid divergence of the chemokines in amphibians.
Introduction
Polyploidization via whole-genome duplication (WGD) is considered a driving force of evolutionary diversification by providing new functions through genetic redundancy (Ohno, 1970; Van de Peer et al., 2009). In general, since duplicated genes have redundant functions, one of the genes degenerates to a pseudogene (or completely lost from the genome). However, duplicated genes generated by WGD show relatively high retention rates compared to duplicated genes generated by usual tandem duplications. Although this feature is explained by proposed modes, such as the duplication-degeneration-complementation (DDC) model (Force et al., 1999) or the gene balance hypothesis (Papp et al., 2003), a complete picture of evolution after WGD is still obscure.
The African clawed frog Xenopus laevis is an excellent model species to infer the evolution after WGD. They have been thought to have undergone tetraploidization around 18 million years ago (Mya) by interspecific hybridization of diploid ancestors (Session et al., 2016). In contrast with the closely related diploid species, Xenopus tropicalis, allotetraploid species X. laevis has two subgenomes, L and S (Hellsten et al., 2007; Session et al., 2016). The corresponding chromosomes of X. laevis L and S to X. tropicalis have identical numbers, except for the fused 9–10 chromosome (Matsuda et al., 2015; Session et al., 2016). The homologous genes in each subgenome are defined as homeologs, discerned by suffix .L or .S. Corresponding to protein-coding genes of X. tropicalis, X. laevis holds 88 and 66% retention rates in the L and S subgenomes, respectively, and 56% of the homeologous gene pairs (Session et al., 2016). The homology of chromosomes between X. laevis and X. tropicalis was well conserved.
Chemokines are low molecular weight cytokines that regulate cell migration through activating the G-protein coupled receptors. The importance of chemokines has been more recognized as they are involved in inflammatory and homeostatic functions, including recruiting leucocytes, cell-homing, neurogenesis, angiogenesis, and regeneration (reviewed in DeVries et al., 1999; Belperio et al., 2000; Cho and Miller, 2002; Bianchi and Mezzapelle, 2020). Depending on the sequence of the two closest cysteines in the peptide, chemokines are classified into four groups: CC, CXC, CX3C, and XC (Moser et al., 2004). Chemokines are not identified in chordates, whereas teleost fish has a broad range of numbers of chemokines, 89 in zebrafish to 20 in Tetraodon (Nomiyama et al., 2013). Chemokine receptors are also classified into four groups: CCR, CXCR, XCR, and CX3CR, according to the biding subfamily of chemokine ligands. Chemokine receptors often have binding promiscuity that the receptor binds more than one chemokine, while a single chemokine often binds to more than one receptor (Rossi and Zlotnik, 2000; Nomiyama et al., 2011). CX3C-type chemokine ligand and receptor families have not been identified in X. tropicalis and teleost (Nomiyama et al., 2013).
At least 48 chemokines have been identified in the human genome, but naturally, not all species conserved the orhologies (Zlotnik et al., 2006). For example, CXCL8 counterpart does not exist in mice (Zlotnik et al., 2006). Amphibians share the last common ancestor with mammals about 360 mya (Kumar and Hedges, 1998). Previous systemic screening exhibited 28 chemokine ligands in X. tropicalis, and they have no significant homology with those of mammalians, except for cxcl12 and cxcl14 (DeVries et al., 2006; Nomiyama et al., 2013). cxcl12 plays essential homeostatic functions with its receptors, cxcr4 and ackr3 (cxcr7) (Lataillade et al., 2004; Burns et al., 2006; Ratajczak et al., 2006; Ratajczak et al., 2012; Hattermann and Mentlein, 2013; Puchert and Engele, 2014). In Xenopus, expression and function of cxcl12 were well examined in early development, including gastrulation, germ cell migration, neural crest migration, and somitogenesis (Moepps et al., 2000; Braun et al., 2002; Fukui et al., 2007; Takeuchi et al., 2010; Mishra et al., 2013; Leal et al., 2014; Shellard and Mayor, 2016).
Chemokines can be good targets for gene evolution because they are thought to evolve relatively quickly due to competition with pathogens such as viruses and bacteria (Murphy, 2001). Although several sequences were obtained in X. laevis (Moepps et al., 2000; Braun et al., 2002; DeVries et al., 2006; Fukui et al., 2007; Cui et al., 2011; Goto et al., 2013; Mishra et al., 2013), elucidating the evolution of Xenopus chemokines will entail the whole aspect of ligands and receptors. Here, based on the latest genomic data of X. laevis and X. tropicalis, we identified all the Xenopus chemokine ligands and their receptors.
Materials and Methods
Gene Identification, Syntenic Analysis, and Phylogenetic Analysis
All identified genes were screened from gene models of the X. laevis annotation gene model v1.8 and v9.2 and genome assembly v9.1 and v9.2 and the X. tropicalis annotation v9.0 and genome assembly v9 deposit in Xenbase (www.xenbase.org), with BLAST and BLAT using known X. tropicalis and human nucleotide and peptide sequences as queries, following secondary screening by the obtained sequences. Gene model sequence errors were corrected manually using genome assemblies in GenomeMatcher (Ohtsubo et al., 2008). Syntenic analysis was performed with genome assembly of X. laevis v9.2, X. tropicalis v10, and H. sapiens GRCh38. Phylogenetic trees were generated in MEGA X (Kumar et al., 2018). Two Xenopus species (X. laevis and X. tropicalis), chicken (Gallus), and human chemokine ligand and receptor genes were aligned using CLUSTAL Omega (Sievers et al., 2011) and trimmed manually. The maximum-likelihood method was performed with 1,000 bootstraps (Felsenstein, 1985). A parameter model was estimated in MEGA X and used JTT with a gamma-distributed model for chemokine ligands and JTT with a gamma-distributed and invariable model for receptors. The inference option was a nearest-neighbor-interchange method on a neighbor-joining (NJ) tree (Saitou and Nei 1987).
dN/dS Calculation
We analyzed molecular evolution rates by computing numbers of synonymous (dS) and non-synonymous (dN) nucleotide substitutions per site for each pair of X. tropicalis versus X. laevis L or versus X. laevis S gene. A low ratio (dN/dS < 1) indicates purifying selection, which maintains similarity between orhologies, whereas a high ratio (dN/dS > 1) indicates positive selection, promoting rapid divergence of the orhologies. The dN/dS ratios were calculated by the CODEML program implemented in the PAML v. 4.9j package (Yang, 2007). We used the free ratio model (model = 1, NS site = 0, fix omega = 0) for dN/dS calculation of each branch.
Transcriptome Correlation Analysis
RNA-seq data analysis and transcriptome correlation are described previously (Session et al., 2016; Watanabe et al., 2017). Expression profiles of identified genes were extracted from the series of oocytes (oocyte stages I-II, III-IV, and V-VI), egg, early embryos (stages 8, 9, 10.5, 12, 15, 20, 25, 30, 35, and 40), and adult organs (brain, eye, lung, stomach, intestine, liver, pancreas, kidney, testis, ovary, heart, muscle, skin, and spleen) of X. laevis J-strain, analyzed by Session et al. (2016), using RNA-seq short reads deposited in NCBI Gene Expression Omnibus (accession number GSE73430 for oocytes and all embryos, GSE73419 for all adult organs). The data include biological replicates (named “Taira201203” and “Ueno201210”) for embryos and adult organs but no replicate for oocytes (only “Ueno201210”). These distinct datasets were called Clutch T and Clutch U, respectively. Transcripts per million (TPM) values of each gene in each clutch are presented in Supplementary Table S1.
Prior to transcriptome correlation analysis, all TPM values ≤ 0.5 were reduced to 0 because transcriptome data less than 0.5 TPM is considered to be irreproducible (Session et al., 2016). The transcriptomic dataset from 11 developmental stages (egg to stage 40) and 14 adult tissues were separately analyzed. Also, Clutch T and Clutch U were separately analyzed to examine the reproducibility in biological replicates. Any gene whose TPM value is ≤0.5 for all samples was removed from the analysis. Correlations of expression profiles between homeologs were examined using Pearson’s correlation and Student’s paired t-test on log2-transformed data [log2 (TPM+1)] as described by Berthelot et al. (2014). Homeologous pairs were categorized into four groups based on 1) correlation (HC: high correlation, p ≤0.05; NC: no correlation, p >0.05, Pearson’s correlation test) and 2) expression levels (SE: same expression levels, p >0.05; DE: different expression levels, p ≤0.05, Student’s paired t-test). Finally, we collected homeologous pairs which were consistently categorized into the same group in both Clutch T and Clutch U. If the category was inconsistent between Clutches, those genes were categorized as “inconsistent (inc).” Also, Clutches T and U were analyzed separately to examine reproducibility in biological replicates. Any gene with a TPM value ≤0.5 for all samples was excluded from analysis and labeled “n/a.”
Results
Overview of Gene Annotation and Identities of Xenopus Chemokine Ligand and Receptor Genes
Based on the gene model of human and X. tropicalis, we screened 52 chemokine ligand genes that contained 44 homeologs (22 pairs) and 8 singletons from X. laevis genome assembly (Table 1, Supplementary Data S1, S2). We also reidentified 30 chemokine genes in X. tropicalis assemblies and represented the retention rate of the gene in X. laevis L subgenome as 93% (28/30) and that of S as 79% (23/30) (Figure 1). Furthermore, 26 chemokine receptors were identified in X. laevis genome, including 18 homeologs (9 pairs) and 8 singletons (Table 1, Figure 2, Supplementary Data S3, S4). The retention rate of the X. tropicalis genes in X. laevis L and S subgenomes was 100% (17/17) and 53% (9/17), respectively (Figure 2). The average amino acid sequence homology between homeologs was 88 and 77% for the receptors and ligands, respectively (Table 1). We conducted a molecular phylogenetic analysis using four vertebrate species (H. sapiens, G. gallus, X. laevis, and X. tropicalis). We found that all receptor genes (17/17) showed clear orthology in the phylogenic tree among species, but only 43% (13/30) of the ligand genes retained clear orthology (Figures 3, 4). Further, dN/dS analysis against X. tropicalis sequences indicated that the dN/dS ratio greater than one of either homeologs was found in 19% (4/21) of the ligands but not in all of the receptors (0/8) (Table 1). These findings demonstrate that the mutation rates remarkably increased in the ligand. RNAseq analysis indicated the expression of eight chemokine ligands and five receptors in embryogenesis (TPM value >5), and only cxcl2.S revealed S dominant expression (Figure 5). In adult tissues, L dominant expression of most genes was observed, but some showed S dominant expression described in distinct. Transcriptome correlation analysis indicated six high correlation-similar expression (HCSE), 10 high correlation-different expression (HCDE), 0 no correlation-similar expression (NCSE), and three no correlation-different expression (NCDE), with 11 inconsistent expression (inc.) in adult tissues (Table 1, Figure 6). We describe the chemokine ligands and receptors below in order of chromosome numbers.
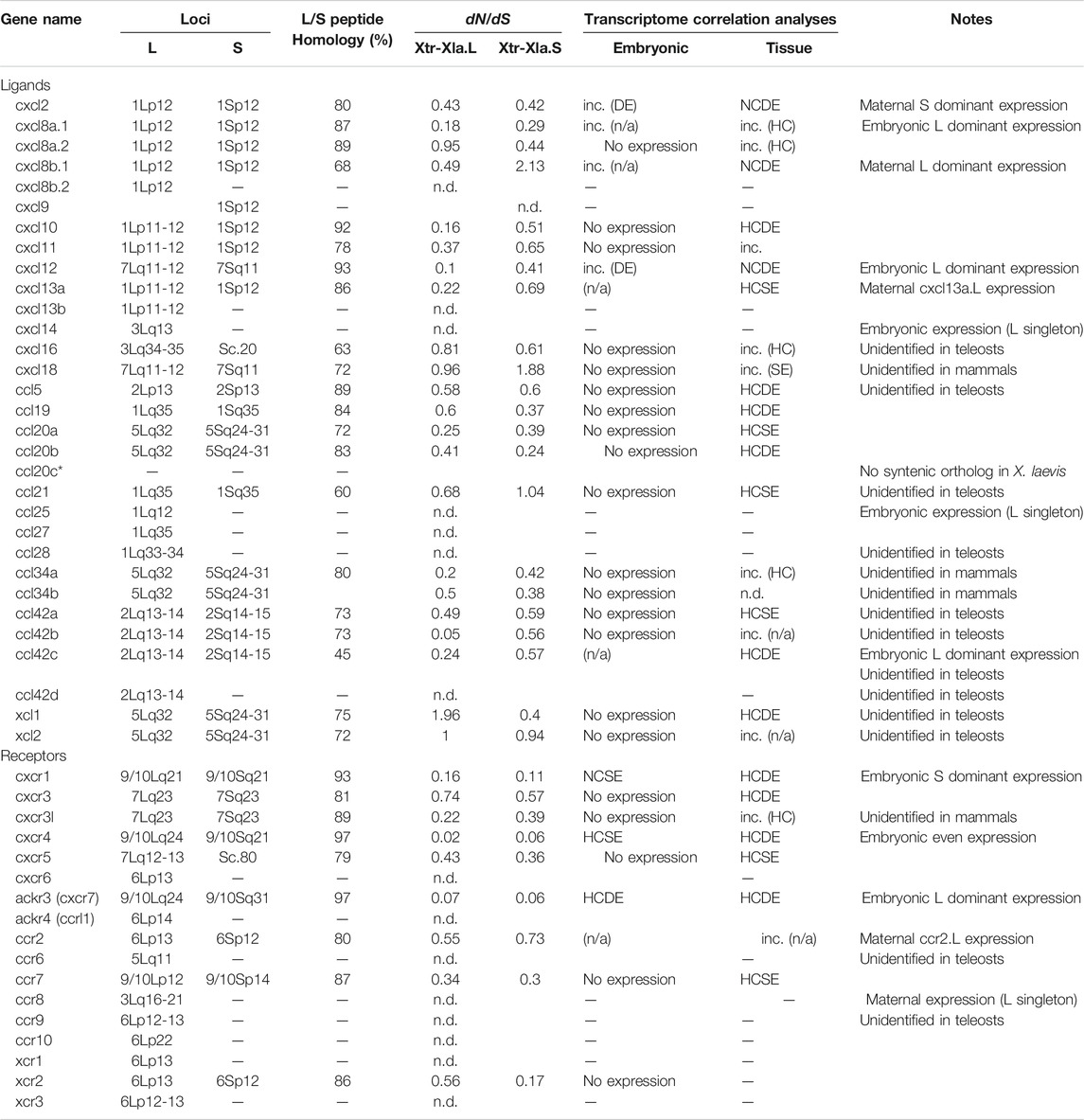
TABLE 1. Review of chemokine ligands and receptors in Xenopus laevis. Loci were estimated by the closest locus of FISH results demonstrated in Session et al. (2016). Orthologies were obtained from molecular phylogenetic analysis and syntenic analysis. Peptide sequence homology between L and S homeologous genes was calculated by CLUSTAL omega using full-length predicted peptide. Columns of transcriptome correlation analyses show the categories of HC: high correlation; NC: no correlation; SE: same expression levels; DE: different expression levels. “inc.” indicates inconsistent categories (see Materials and Methods). Note that cxcl16, ccl5, ccl21, ccl28, ccl42a, ccl42b, ccl42c, ccl42d, xcl1, xcl2, ccr2, and ccr8 genes are unidentified in teleosts, and cxcl18, ccl34a, ccl34b, and cxcr3l genes are unidentified in mammals (Nomiyama et al., 2013).
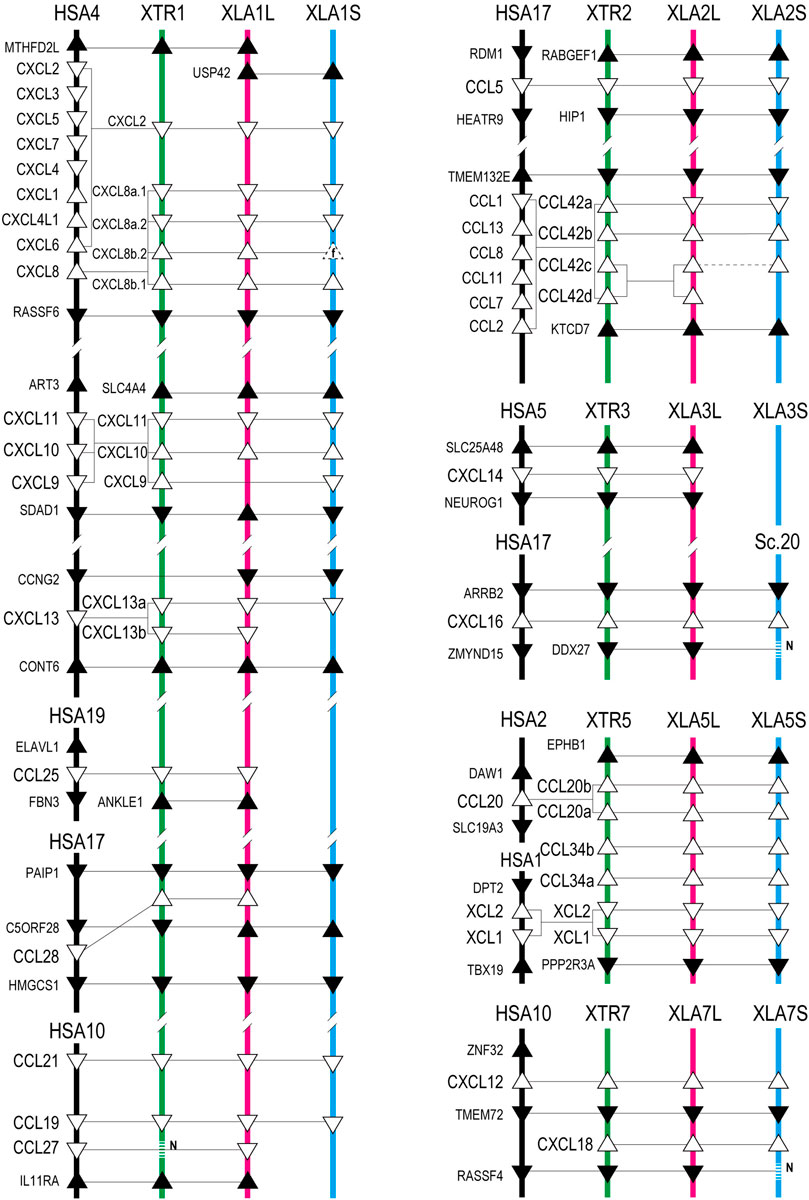
FIGURE 1. Genomic organization of Xenopus chemokines. Positions of chemokine genes (open triangles) and flanking genes (closed triangles) with direction are indicated in the order of Xenopus chromosome numbers. Chromosomes Abbreviations. HSA: H. sapiens (black lines); XTR: X. tropicalis (green lines); XLA_L and XLA_S: X. laevis L and S subgenome (red and blue lines), respectively. Sc is a scaffold number that is unbuilt in the chromosome assembly. The homologous relationship presented by connected lines was analyzed phylogenetically. The dotted line with N represents the genes unidentified with N-gap. Triangles drawn with dotted line show fossil genes (f).
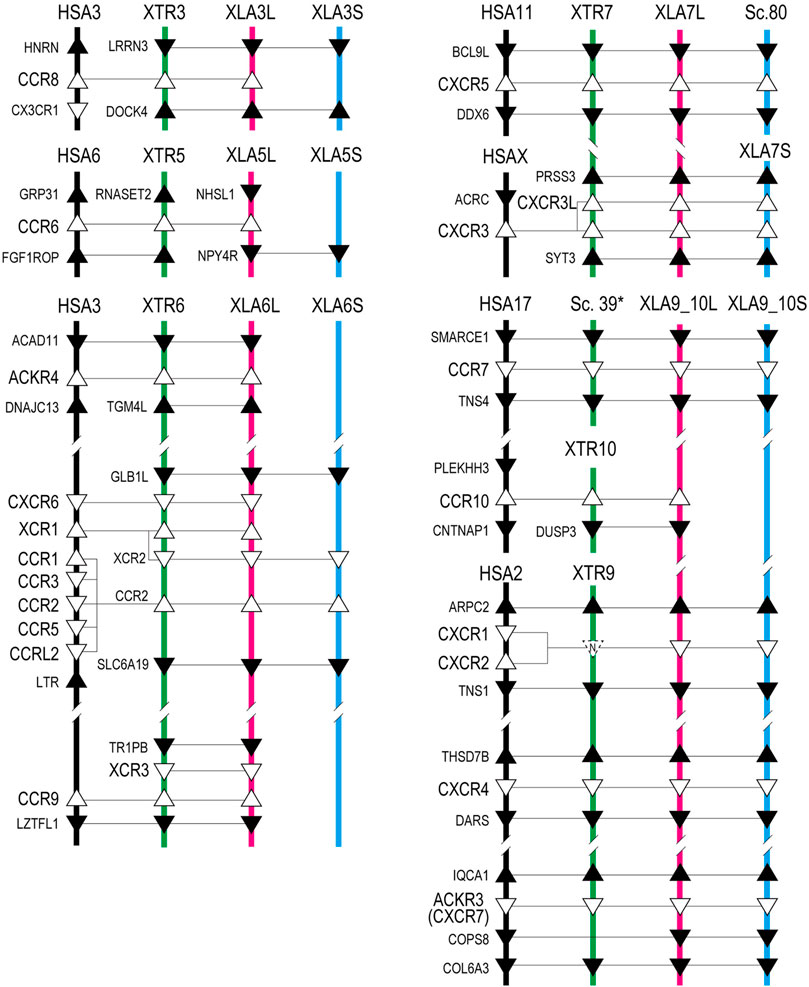
FIGURE 2. Genomic organization of Xenopus chemokine receptors. Representation is the same as Figure 1.
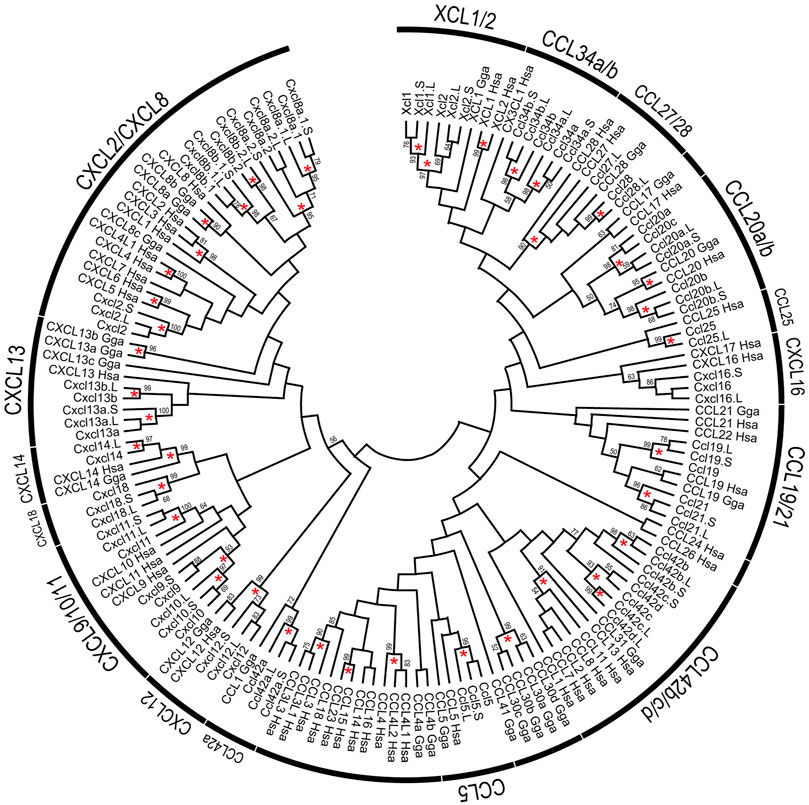
FIGURE 3. Phylogenetic tree of chemokine ligands. The tree indicates 154 chemokine proteins, including 52 of X. laevis, 30 of X. tropicalis, 24 of G. Gallus, and 48 of H. sapiens genes. Chemokine names related to Xenopus represented on the arcs. Bootstrap values greater than 50% were indicated, and asterisks show values greater than 90%. The alignment of chemokine proteins was prepared using CLUSTAL omega. Maximum likelihood methods using full-length were performed with 1,000 bootstraps using JTT with Gamma-distributed model, and inference option was a nearest-neighbor interchange method on NJ tree.
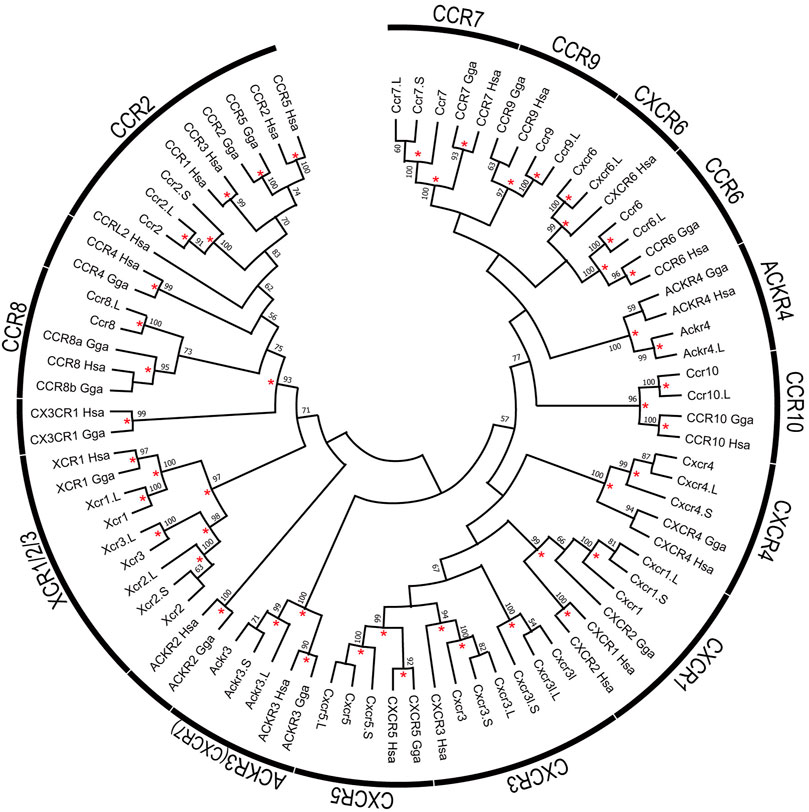
FIGURE 4. Phylogenetic tree of chemokine receptors. The tree indicates 82 chemokine receptor proteins, including 26 of X. laevis, 17 of X. tropicalis, 17 of G. Gallus, and 22 of H. sapiens genes. Receptor names represented on the arcs. Bootstrap values greater than 50% were indicated, and asterisks show values greater than 90%. The alignment of the receptor proteins was prepared using clustal omega and trimmed manually as 297 peptides with gaps. Maximum likelihood methods were performed with 1,000 bootstraps using the JTT model with Gamma distribution and invariant sites and complete deletion of gaps/missing data and inference option was a nearest-neighbor interchange method on NJ tree.
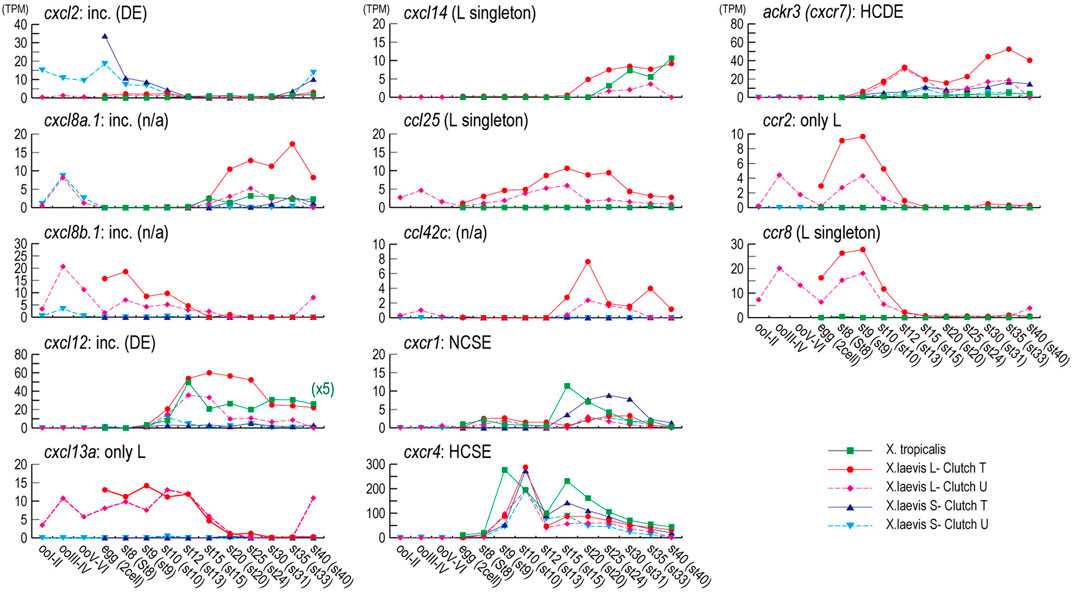
FIGURE 5. Expression profiles during oogenesis and embryogenesis. Genes with a max TMP value of 5 or higher during oogenesis and embryogenesis are presented. Expression profiles for X. tropicalis were obtained from Tan et al. (2013). Unfortunately, cxcl8, cxcl13a, and ccl42c have no expression profile of X. tropicalis. The vertical axis shows the expression level (TPM), and the horizontal axis is the developmental stages of X. laevis, X. tropicalis indicated within parentheses. All TPM values are shown in Supplementary Table S1. Orthologous family names and results of transcriptome correlation analysis are indicated on the upper left of each graph. Symbols. Square: X. tropicalis (green); circle,: X. laevis L-clutch T (red); diamond: X. laevis L-clutch U (magenta); triangle: X. laevis S-clutch T (blue); reverse triangle: X. laevis S-clutch U (cyan). Note that the TPM value of X. tropicalis in cxcl12 was indicated one-fifth scale to increase the resolution (x5).
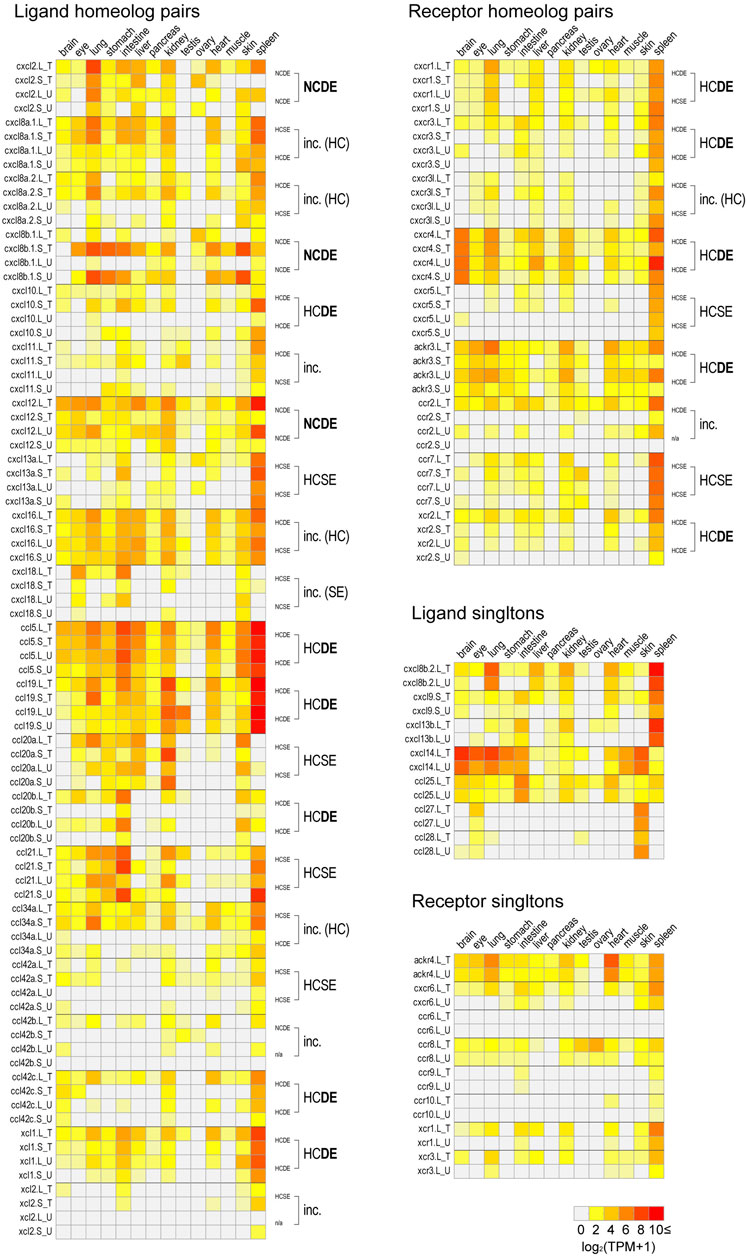
FIGURE 6. Expression profiles in adult tissues. log2 transformed TPM values in Clutch T and Clutch U [log2 (TPM+1)] of all chemokine ligand and receptor genes of brain, eye, lung, stomach, intestine, liver, pancreas, kidney, testis, ovary, heart, muscle, skin, and spleen are presented with heat maps. For each homeologous pair, transcriptome correlation groups are indicated on the right side of panels. In cases of the “inc.” group, their details are described with parentheses. If results from two clutches showed half-consistency, their common results are indicated (HC or SE). Singletons were presented in the separated panels. All TPM values are shown in Supplementary Table S1.
Chemokine Ligands of Xenopus Laevis
A large cluster of CXC-type chemokines was found between flanking genes rasssf6 and usp42 in Xenopus chromosome 1, which contained four homeologous pairs and one L singleton (Figure 1). They have no one-to-one relationship with human orhologies indicated by molecular phylogenetic analysis (Figure 3). Among cxcl8 homeologous genes, cxcl8b.1 were distinct in sequence homology (68%) and expression patterns, with L dominant in embryos and S dominant in tissues (Table 1, Figures 5, 6). Since the X residue of the CXC motif has been noted for altering the binding ability to the receptor (Wedemeyer et al., 2020), we examined that, of the cxcl8 chemokine family, four cxcl8 homologs between X. laevis and X. tropicalis were conserved as CQC in cxcl8b.2 and cxcl2, and CLC in cxcl8a.2 and cxcl8a.1 homologous genes. However, cxcl8b.1 homologs presented unique sequences as CKC in cxcl8b.1, CRC in cxcl8b.1.L, and CQC in cxcl8b.1.S, respectively. Finally, a higher dN/dS ratio (2.32) of cxcl8b.1.S exhibited potentially positive selection or relaxation, markedly suggesting that this gene experienced unusual evolution.
cxcl9, cxcl10, and cxcl11 exhibited the cluster on chromosome 1, and cxcl9.S retained the only cxcl9.S singleton among the chemokine ligands. They have no one-to-one relationship with human orhologies (Figure 3). Relatively S dominant expression of these genes was observed in tissues (Figure 6).
cxcl13 paralogs showed tandem duplication in the Xenopus genome. Their synteny is consistent with human, located between flanking genes ccng2 and cont6. Expression levels during oogenesis and embryogenesis presented L dominant expression, whereas tissue expression was highly correlated (HCSE) (Figures 5, 6).
ccl25 had no synteny conservation between Xenopus and human. This gene was adjacent to ankle1 in Xenopus, whereas fbn3 and elavl1 were in humans (Figure 1). Broad expressions were found through oogenesis and embryogenesis and among adult tissues (Figures 5, 6), suggesting homeostatic function.
ccl28 was adjacent to the c5orf28 in both Xenopus and human, but their positions were rearranged (Figure 1). ccl28 formed a clade with ccl27 in phylogenetic analysis (Figure 3). Both ccl27 and ccl28 were L singleton and expressed in the skin dominantly (Figure 6).
ccl21, ccl19, and ccl27 formed a small cluster flanked by 1l11ra in chromosome 1 and was observed in human. Note that il11ra.S was not found, probably due to gene loss in the S subgenome. Unfortunately, ccl27 of X. tropicalis was unidentified with N deletion in the genome sequence. ccl21 and ccl19 formed a clade in phylogenetic analysis (Figure 3). ccl19 homeologous pair revealed different expression (HCDE). ccl19.L was most highly expressed in testis among the chemokines examined (Figure 6). ccl21.L was dominantly expressed in lung, stomach, kidney, and S dominant expression in intestine and spleen (Figure 6).
ccl5 was one of the abundant CC-type chemokines in Xenopus (Figure 6 and Supplementary Table S1) and exhibited HCDE in transcriptome correlation analysis. Synteny of ccl5 was inconsistent between Xenopus and human as flanking genes of ccl5 were rabgef1 and hip1 for Xenopus, but RDM1 and HEATR9 for human (Figure 1). Intriguingly, CCL5 can bind to three receptors, CCR1, CCR3, and CCR5 (Zlotnik et al., 2006), but all orhologies were not identified in Xenopus.
ccl42a, ccl42b, ccl42c, and ccl42d formed a cluster (Figure 1). The nomenclature of these genes depends on Nomiyama et al. (2013). ccl42c and ccl42d have no obvious orthology in phylogenetic analysis (Figure 3), which may be due to gene conversion or species-specific tandem duplication. Notably, the ccl42 cluster was adjacent to tmem132e, as well as one of the human chemokine clusters. Further, ccl42a clade included G. gallus ccl1 with the bootstrap value of 72%, and ccl42b, ccl42c, and ccl42d formed a clade with human CCL1, CCL13, CCL8, CCL11, CCL7, and CCL2. These findings suggest that ccl42 chemokines were orhologies to mammalian ccl cluster genes. ccl42c.L was slightly expressed in embryogenesis, and ccl42a.L, ccl42b.L, and ccl42c.L were expressed in spleen L dominantly (Figures 5, 6).
cxcl14 is one of the most conserved chemokine genes among vertebrates and has been reported as a novel ligand of cxcr4 similar to cxcl12 (Tanegashima et al., 2013). RNAseq analysis demonstrated that the expression was detected from neurula, and relatively higher expressions were observed in the brain, skin, lung, stomach, eye, and muscle (Figures 5, 6).
cxcl16 is a transmembrane-type chemokine (Matloubian et al., 2000; Abel et al., 2004), and the CXC motif is replaced by the CC motif in the Xenopus genus. The peptide sequence homology between homeologs was relatively low (68%).
xcl1, xcl2, ccl34a, ccl34b, ccl20a, and ccl20b formed a cluster in Xenopus genomes, whereas human orhologies XCL1, XCL2, and CCL20 were scattered in different chromosomes (Figure 1). Further, the flanking gene of ccl20a is ppp2r3a, whereas those of human CCL20 are SLC19A3 and DAW1. Phylogenetic analysis indicated ccl20b, not ccl20a, was relatively similar to human and bird CCL20. A distinct expression pattern was observed between ccl20a and ccl20b of a higher level of ccl20a homeologs in the liver and kidney and ccl20b homeologs in the stomach (Figure 6). ccl20a.S was dominantly expressed in the kidney. ccl20b homeologous pair exhibited different expression patterns (HCDE). ccl20c identified in X. tropicalis (Nomiyama et al., 2013) had no syntenic ortholog in X. laevis.
ccl34a and ccl34b formed a clade with xcl1 and xcl2 in molecular phylogenetic analysis (Figure 3). The gene name of ccl34 depends on Nomiyama et al. (2013). ccl34a.L exhibited expression in the lung and spleen dominantly. There are no expression data of cxcl34b because this is a newly identified gene in this study after Session et al. (2016).
xcl1 and xcl2 were clustering genes likewise orhologies of human. However, molecular phylogenetic analysis exhibited separated branches of Xenopus and human orhologies (Figure 3), and the Xenopus xcl1 and xcl2 were separated by each gene. This no one-to-one relationship suggests species-specific tandem duplication in the Xenopus ancestor. Intriguingly, dN/dS ratio of 1.96 and 1.00 in xcl1.L and xcl2.L, respectively, indicated higher relaxation in both L homeologs. Transcriptome correlation analysis demonstrated different expression (HCDE) between xcl1 homeologs. xcl2.S was expressed S dominantly in the spleen (Figure 6).
cxcl12 and cxcl18 were adjacent to tmem72 and on the opposite side (Figure 1). Expression profiles of cxcl12 homeologs demonstrated L dominant expressions in embryogenesis and adult tissues (Figures 5, 6). cxcl18 ortholog was found in the teleost, was unidentified in mammals, and has been not yet reported function (Nomiyama et al., 2013). cxcl18.L was dominantly expressed in the eye and intestine. dN/dS ratio of 1.85 in cxcl18.S indicated relaxation or positive selection.
Chemokine Receptors of Xenopus Laevis
ccr8 was L singleton gene located between flanking genes lrrn3 and dock4 on chromosome 3, whereas the gene order was not conserved in human (Figure 1). RNAseq analysis demonstrated the unique pattern of ccl8.L. This gene was expressed in oocytes to blastula through embryogenesis and testis and ovary of adult tissues (Figure 6). ccr8 is a candidate receptor for ccl1 (Tiffany et al., 1997), and the phylogenetic analysis indicated G. gallus ccl1 organizes a clade with ccl42a (Figure 3), suggesting a functional similarity between ccl42a and ccl1.
ccr6 was located between flanking genes lrrn3 and dock4 in X. tropicalis and nhsl1 and npy4r in X. laevis on chromosome 5, respectively (Figure 1). Partial synteny of X. tropicalis was conserved in human but not in X. laevis subgenomes. Phylogenetic analysis indicated clear orthology between species (Figure 4). Therefore, syntenic inconsistency may be due to chromosome rearrangement (Session et al., 2016). RNAseq analysis indicated no expression of ccr6 in all tissues. Human CCR6 is identified as a CCL20 receptor (Baba et al., 1997; Hieshima et al., 1997).
ackr4, also known as ccrl1, is a decoy receptor that controls chemokine levels by sequestrating the ligands. Xenopus ackr4 gene was located between acad11 and tgm4l on chromosome 7 as L singleton. Locus around ackr4 and its surrounding genes were not identified in the available S subgenome. RNAseq analysis demonstrated broad expression in adult tissues with a higher level in the heart, except for the ovary. Since human ACKR4 can bind to CCL2, CCL8, CCL13, CCL19, CCl20, CCL21, and CCL25 (Gosling et al., 2000; Schweickart et al., 2000; Matti et al., 2020), these chemokines might be the candidate ligands for Xenopus ackr4. Note that ccl42b, ccl42c, ccl42d were candidate orhologies for ccl2, ccl8, and ccl13 (see ligands sections).
cxcr6, xcr1, xcr2, and ccr2 formed a cluster in Xenopus chromosome 6. cxcr6 and xcr1 were L singleton, whereas xcr2 and ccr2 retained both homeologs. Flanking genes of this cluster were different between Xenopus and human, but synteny within-cluster was well-conserved. xcr1 seems to be duplicated in the ancestral Xenopus genome. Notably, three xcr1-type receptors, xcr1, xcr2, and xcr3, existed in the Xenopus genome with clear orthology (Figure 2). In contrast, synteny and phylogenetic analysis demonstrated that Xenopus ccr2 corresponds to a single ortholog for human CCR1, CCR2, CCR3, CCR5, and CCRL2 (Figures 2, 4). RNAseq analysis of ccr2 homeologs indicated L dominant expression in embryogenesis and adult tissues.
ccr9 was next to xcr3 between tr1pb and lztfl1 in chromosome 6 as L singleton and weakly expressed in the lung and spleen. The candidate ligand ccl25 existed in the Xenopus genome.
Human CXCR5 is a candidate receptor for CXCL13 and has been reported to be essential for B cell migration (Förster et al., 1996). Surrounding synteny of this gene was conserved between Xenopus and human. Expression of both cxcr5.L and cxcr5.S was found in the spleen.
Xenopus cxcr3 and cxcr3l were tandemly aligned between prss3 and syt3 in chromosome 7. There was no syntenic conservation with human. Phylogenetic analysis revealed that cxcr3, cxcr3l, and cxcr5 clades form a clade with a bootstrap value of 57% (Figure 4). L dominant expression of cxcr3 was observed in the lung, intestine, kidney, and spleen with different expression (HCDE), whereas S dominant expression of cxcr3l was observed in the lung and spleen.
ccr7 and its surrounding genes (smarce1 and tns4) were conserved between human and X. laevis. Unfortunately, locus in X. tropicalis was not identified in available genome sequences. RNAseq analysis demonstrated expression in the spleen and dominant expression of ccr7.L in the intestine and ccr7.S in the testis (Figure 6). CCR7 is a candidate receptor for CCL19 and CCL21 in human (Förster et al., 2008). Interestingly, dominant expression of ccl21.S in the intestine and ccl19.L and ccl21.L in the testis was observed. This inconsistent expression pattern of receptor and ligand in L versus S may serve as a model for crosstalk between subgenomes.
ccr10 was L singleton and gene order around Xenopus ccr10 was inconsistent with human. CCR10 binds to CCL27 in human (Homey et al., 2000). Slightly expression was observed in the heart and spleen.
cxcr1 is a candidate for the receptor of the cxcl8 cluster genes. Synteny was conserved in human adjacent to arpc2 and tns1, although human ortholog was tandemly duplicated as cxcr1 and cxcr2 (Figure 2). RNAseq analysis demonstrated S dominant expression in embryogenesis and spleen, and transcriptome analysis indicated different expression (HCDE).
cxcr4 and ackr3 were receptors for cxcl12. cxcr4 was located between thsd8b and dars. In X. tropicalis, cops8 was translocated within X. tropicalis chromosome 9 (XTR9). Almost similar embryonic expressions of both cxcr4.L and cxcr4.S were detected from stage 9 (late blastula). In adult tissues, transcriptome correlation analysis indicated different expression (HCDE) as L dominant expression in the intestine, liver, heart, and spleen. ackr3 was located between iqca1 and cops8, and L-dominant expression was detected from stage 9. Almost L dominant expression was observed among adult tissues. However, S was dominant in testis. Transcriptome analysis indicated different expression (HCDE).
Discussion
This study comprehensively identified and analyzed chemokine ligands and their receptors in X. laevis genome. L subgenome retained genes are dominant as 13 for L singleton genes versus one for S singleton of the identified genes, consistent with the S subgenome having a faster rate of pseudogenization than the L after allopolyploidization Xenopus species (Furman et al., 2018). Transcriptome correlation analysis suggests that the genes of 13 different expression (DE) homeologous pairs include potential candidates for subfunctionalization or neofunctionalization.
For the retention rates of homeologous gene pairs, all ligand genes in X. laevis genome showed 71% (22/31) in this study. This rate was higher than all analyzed genes (56%; 8,806/15,613) reported by Session et al. (2016). The details of that were 71% (10/14) for CXC-type, 71% (10/14) for CC-type, and 100% (2/2) for XC-type ligands; no significant differences between them was observed, suggesting that WGD promotes constant evolutionary divergence of ligands because it ensured diversity and increased the likelihood of acquiring novel functions such as antibacterial activity (Hieshima et al., 2003). This idea may be supported by transcriptome correlation analysis that revealed a higher rate of different expression and L or S dominant expression pairs in chemokine ligands (9 of DE vs. 2 of SE, one L-dominant and one S-dominant, Table 1).
The retention rate of all chemokine receptor homeologous pairs (53%, 9/17) was similar to all analyzed genes. However, details were 86% (6/7) for CXC-type (including ackr3), 29% (2/7) for CC-type (including ackr4), and 33% (1/3) for XC-type chemokine receptor. S subgenome gene loss of CC and XC may depend on “genome fractionation” (Schnable et al., 2011; Sankoff et al., 2012; Garsmeur et al., 2014). In contrast, the CXC-type receptors and the candidate CXC-type ligands tended to have higher retention rates, suggesting selective pressure for dosage compensation or subfunctionalization in their expression domain or target specificity (Session et al., 2016; Watanabe et al., 2017). As another example, the homeologous pairs of the ligands and receptors involved in growth factors showed the highest retention rate for TGF, FGF, and Wnt signaling (Michiue et al., 2017; Suzuki et al., 2017).
dN/dS analysis revealed four genes, cxcl8b.1.S, cxcl18.S, ccl21.S, and xcl1.L, had a dN/dS ratio greater than one, and four genes, cxcr4.L, cxcr4.S, ackr3.L, and ackr3.S, had shallow ratios of less than 0.1. Referring to ratios from automatically calculated results from Session et al. (2016), the homeologous genes with dN/dS ratios greater than one were only 0.3% [45 in 17,590 genes (8,795 homeologous pairs)] and less than 0.1 were 32% (5,561 in 17,590). These findings suggest a higher tendency of relaxation in the chemokine genes among homeologous genes.
Regarding cxcl8 genes, the homeologs of cxcl8a.1 and cxcl8a.2 showed a high correlation (Table 1), and the pattern of the expressed organs was also similar. These genes possess ELR motifs and are predicted to promote the migration of neutrophils (Strieter et al., 1995). Since cxcl8a.1 gene expression was upregulated by virus infection (Koubourli et al., 2018), it may function in the early response to infection and inflammation in Xenopus. In contrast, cxc8b.2.L recruited anti-inflammatory macrophages, which expressed genes associated with immune suppression (Koubourli et al., 2018). During inflammation and tissue repair, there is the recruitment of proinflammatory M1 macrophage, followed by anti-inflammatory M2 macrophage (Murray and Wynn, 2011). Diversified cxcl8b genes in X. laevis may play a different role in regeneration and tissue repair.
We found the expression of ccr2 and ccr8 in oogenesis, suggesting that these genes act in oogenesis or as a maternal factor. ccr2 was also broadly expressed in adult tissues (Figure 6), reflecting its expression in macrophages and lymphocytes. Although a ligand for ccr2 was not identified in Xenopus, ccl42b, ccl42c, and ccl42d conserved synteny and retained similarity with the CCL2, which is human CCR2 ligand (Zlotnik and Yoshie, 2000). Therefore, some of these may be candidates for the ligand of Xenopus ccr2. Actually, not in the oocyte, but the weak expression of ccl42b.S was detected in the ovary (Figure 6). Next, regarding ccr8, among all the receptors examined in this study, only ccr8.L showed dominant expression in the testis and ovary. CCL1 and CCL18 were known as ligands for CCR8 (Garlisi et al., 1999; Islam et al., 2013), but both have been unidentified in Xenopus. Interestingly, although ccr2 and ccr8 are not identified in teleosts (Table 1), CCR2 and CCR8 RNAs have also been detected in the human oocyte (Zhao et al., 2020). Although their role in oocytes is still unclear, both genes may have evolutionarily conserved functions.
cxcl12, cxcr4, and ackr3 had been examined their expression and function in the early development of X. laevis (Moepps et al., 2000; Braun et al., 2002; Fukui et al., 2007; Takeuchi et al., 2010; Mishra et al., 2013; Shellard and Mayor, 2016). Because sequence homology of each ortholog is relatively well conserved among vertebrates (DeVries et al., 2006; Nomiyama et al., 2013), cxcl12, cxcr4, and ackr3 are anticipated to undergo intense purifying selection. This prediction was also supported in this study. In contrast, the expression levels of cxcl12.S and ackr3.S were reduced compared to L counterparts in early development, and all three homeologous pairs indicated HCDE in adult tissues. These findings suggest that the potential subfunctionalization/pseudogenization is progressing in homeologs of cxcl12, cxcr4, and ackr3.
Data Availability Statement
The datasets presented in this study can be found in online repositories. The names of the repository/repositories and accession number(s) can be found in the article/Supplementary Material.
Ethics Statement
Ethical review and approval were not required for the animal study because we used only genome assembly datum, not organisms.
Author Contributions
AF did gene model screening, manual annotation validation, and transcriptome analysis. MM conducted dN/dS analysis. All authors contributed to the phylogenetic analysis and manuscript writing.
Funding
This study was supported by the Joint Research Grant from the Institute of Science and Engineering, Chuo University.
Conflict of Interest
The authors declare that the research was conducted in the absence of any commercial or financial relationships that could be construed as a potential conflict of interest.
Publisher’s Note
All claims expressed in this article are solely those of the authors and do not necessarily represent those of their affiliated organizations or those of the publisher, the editors, and the reviewers. Any product that may be evaluated in this article, or claim that may be made by its manufacturer, is not guaranteed or endorsed by the publisher.
Acknowledgments
We would like to thank all members of the X. laevis genome project consortium in Japan. We also thank Atsushi Toyoda, Yutaka Suzuki, Taejoon Kwon, Atsushi Suzuki, Naoto Ueno, and Masanori Taira for RNA-seq experiments and TPM calculations. Thanks also are to Yoshinobu Uno for the FISH experiment. We acknowledge the Hiroshima University Amphibian Research Center through the National BioResource Project (NBRP) of AMED for providing the useful genome browser for X. tropicalis and X. laevis under Grant Number JP20km0210085.
Supplementary Material
The Supplementary Material for this article can be found online at: https://www.frontiersin.org/articles/10.3389/fgene.2021.787979/full#supplementary-material
References
Abel, S., Hundhausen, C., Mentlein, R., Schulte, A., Berkhout, T. A., Broadway, N., et al. (2004). The Transmembrane CXC-Chemokine Ligand 16 Is Induced by IFN-γ and TNF-α and Shed by the Activity of the Disintegrin-like Metalloproteinase ADAM10. J. Immunol. 172, 6362–6372. doi:10.4049/jimmunol.172.10.6362
Baba, M., Imai, T., Nishimura, M., Kakizaki, M., Takagi, S., Hieshima, K., et al. (1997). Identification of CCR6, the Specific Receptor for a Novel Lymphocyte-Directed CC Chemokine LARC. J. Biol. Chem. 272, 14893–14898. doi:10.1074/jbc.272.23.14893
Belperio, J. A., Keane, M. P., Arenberg, D. A., Addison, C. L., Ehlert, J. E., Burdick, M. D., et al. (2000). CXC Chemokines in Angiogenesis. J. Leukoc. Biol. 68, 1–8. doi:10.1189/jlb.68.1.1
Berthelot, C., Brunet, F., Chalopin, D., Juanchich, A., Bernard, M., Noël, B., et al. (2014). The Rainbow trout Genome Provides Novel Insights into Evolution after Whole-Genome Duplication in Vertebrates. Nat. Commun. 5, 3657. doi:10.1038/ncomms4657
Bianchi, M. E., and Mezzapelle, R. (2020). The Chemokine Receptor CXCR4 in Cell Proliferation and Tissue Regeneration. Front. Immunol. 11, 2109. doi:10.3389/fimmu.2020.02109
Braun, M., Wunderlin, M., Spieth, K., Knöchel, W., Gierschik, P., and Moepps, B. (2002). Xenopus laevis Stromal Cell-Derived Factor 1: Conservation of Structure and Function during Vertebrate Development. J. Immunol. 168, 2340–2347. doi:10.4049/jimmunol.168.5.2340
Burns, J. M., Summers, B. C., Wang, Y., Melikian, A., Berahovich, R., Miao, Z., et al. (2006). A Novel Chemokine Receptor for SDF-1 and I-TAC Involved in Cell Survival, Cell Adhesion, and Tumor Development. J. Exp. Med. 203, 2201–2213. doi:10.1084/jem.20052144
Cho, C., and Miller, R. J. (2002). Chemokine Receptors and Neural Function. J. Neurovirol. 8, 573–584. doi:10.1080/13550280290101003
Cui, X., Han, Y., Pan, Y., Xu, X., Ren, W., and Zhang, S. (2011). Molecular Cloning, Expression and Functional Analysis of Interleukin-8 (IL-8) in South African Clawed Frog (Xenopus laevis). Dev. Comp. Immunol. 35, 1159–1165. doi:10.1016/j.dci.2011.04.005
DeVries, M. E., Ran, L., and Kelvin, D. J. (1999). On the Edge: the Physiological and Pathophysiological Role of Chemokines during Inflammatory and Immunological Responses. Semin. Immunol. 11, 95–104. doi:10.1006/smim.1999.0165
DeVries, M. E., Kelvin, A. A., Xu, L., Ran, L., Robinson, J., and Kelvin, D. J. (2006). Defining the Origins and Evolution of the Chemokine/chemokine Receptor System. J. Immunol. 176, 401–415. doi:10.4049/jimmunol.176.1.401
Felsenstein, J. (1985). Confidence Limits on Phylogenies: An Approach Using the Bootstrap. Evolution 39, 783–791. doi:10.1111/j.1558-5646.1985.tb00420.x
Förster, R., Mattis, A. E., Kremmer, E., Wolf, E., Brem, G., and Lipp, M. (1996). A Putative Chemokine Receptor, BLR1, Directs B Cell Migration to Defined Lymphoid Organs and Specific Anatomic Compartments of the Spleen. Cell 87, 1037–1047. doi:10.1016/s0092-8674(00)81798-5
Förster, R., Davalos-Misslitz, A. C., and Rot, A. (2008). CCR7 and its Ligands: Balancing Immunity and Tolerance. Nat. Rev. Immunol. 8, 362–371. doi:10.1038/nri2297
Force, A., Lynch, M., Pickett, F. B., Amores, A., Yan, Y.-l., and Postlethwait, J. (1999). Preservation of Duplicate Genes by Complementary, Degenerative Mutations. Genetics 151, 1531–1545. doi:10.1093/genetics/151.4.1531
Fukui, A., Goto, T., Kitamoto, J., Homma, M., and Asashima, M. (2007). SDF-1α Regulates Mesendodermal Cell Migration during Frog Gastrulation. Biochem. Biophysical Res. Commun. 354, 472–477. doi:10.1016/j.bbrc.2007.01.007
Furman, B. L. S., Dang, U. J., Evans, B. J., and Golding, G. B. (2018). Divergent Subgenome Evolution after Allopolyploidization in African Clawed Frogs (Xenopus). J. Evol. Biol. 31, 1945–1958. doi:10.1111/jeb.13391
Garlisi, C. G., Xiao, H., Tian, F., Hedrick, J. A., Billah, M. M., Egan, R. W., et al. (1999). The Assignment of Chemokine-Chemokine Receptor Pairs: TARC and MIP-1β Are Not Ligands for Human CC-Chemokine Receptor 8. Eur. J. Immunol. 29, 3210–3215. doi:10.1002/(sici)1521-4141(199910)29:10<3210::aid-immu3210>3.0.co;2-w
Garsmeur, O., Schnable, J. C., Almeida, A., Jourda, C., D’Hont, A., and Freeling, M. (2014). Two Evolutionarily Distinct Classes of Paleopolyploidy. Mol. Biol. Evol. 31, 448–454. doi:10.1093/molbev/mst230
Gosling, J., Dairaghi, D. J., Wang, Y., Hanley, M., Talbot, D., Miao, Z., et al. (2000). Cutting Edge: Identification of a Novel Chemokine Receptor that Binds Dendritic Cell- and T Cell-Active Chemokines Including ELC, SLC, and TECK. J. Immunol. 164, 2851–2856. doi:10.4049/jimmunol.164.6.2851
Goto, T., Michiue, T., Ito, Y., and Asashima, M. (2013). Characterization of CXC-type Chemokine Molecules in Early Xenopus laevis Development. Int. J. Dev. Biol. 57, 41–47. doi:10.1387/ijdb.120223ma
Hattermann, K., and Mentlein, R. (2013). An Infernal Trio: the Chemokine CXCL12 and its Receptors CXCR4 and CXCR7 in Tumor Biology. Ann. Anat. - Anatomischer Anzeiger 195, 103–110. doi:10.1016/j.aanat.2012.10.013
Hellsten, U., Khokha, M. K., Grammer, T. C., Harland, R. M., Richardson, P., and Rokhsar, D. S. (2007). Accelerated Gene Evolution and Subfunctionalization in the Pseudotetraploid Frog Xenopus laevis. BMC Biol. 5, 31. doi:10.1186/1741-7007-5-31
Hieshima, K., Imai, T., Opdenakker, G., Van Damme, J., Kusuda, J., Tei, H., et al. (1997). Molecular Cloning of a Novel Human CC Chemokine Liver and Activation-Regulated Chemokine (LARC) Expressed in Liver. J. Biol. Chem. 272, 5846–5853. doi:10.1074/jbc.272.9.5846
Hieshima, K., Ohtani, H., Shibano, M., Izawa, D., Nakayama, T., Kawasaki, Y., et al. (2003). CCL28 Has Dual Roles in Mucosal Immunity as a Chemokine with Broad-Spectrum Antimicrobial Activity. J. Immunol. 170, 1452–1461. doi:10.4049/jimmunol.170.3.1452
Homey, B., Wang, W., Soto, H., Buchanan, M. E., Wiesenborn, A., Catron, D., et al. (2000). Cutting Edge: the Orphan Chemokine Receptor G Protein-Coupled Receptor-2 (GPR-2, CCR10) Binds the Skin-Associated Chemokine CCL27 (CTACK/ALP/ILC). J. Immunol. 164, 3465–3470. doi:10.4049/jimmunol.164.7.3465
Islam, S. A., Ling, M. F., Leung, J., Shreffler, W. G., and Luster, A. D. (2013). Identification of Human CCR8 as a CCL18 Receptor. J. Exp. Med. 210, 1889–1898. doi:10.1084/jem.20130240
Koubourli, D. V., Yaparla, A., Popovic, M., and Grayfer, L. (2018). Amphibian (Xenopus laevis) Interleukin-8 (CXCL8): A Perspective on the Evolutionary Divergence of Granulocyte Chemotaxis. Front. Immunol. 9, 2058. doi:10.3389/fimmu.2018.02058
Kumar, S., and Hedges, S. B. (1998). A Molecular Timescale for Vertebrate Evolution. Nature 392, 917–920. doi:10.1038/31927
Kumar, S., Stecher, G., Li, M., Knyaz, C., and Tamura, K. (2018). MEGA X: Molecular Evolutionary Genetics Analysis across Computing Platforms. Mol. Biol. Evol. 35, 1547–1549. doi:10.1093/molbev/msy096
Lataillade, J. J., Domenech, J., and Le Bousse-Kerdilès, M. C. (2004). Stromal Cell-Derived Factor-1 (SDF-1)\CXCR4 Couple Plays Multiple Roles on Haematopoietic Progenitors at the Border between the Old Cytokine and New Chemokine Worlds: Survival, Cell Cycling and Trafficking. Eur. Cytokine Netw. 15, 177–188.
Leal, M. A., Fickel, S. R., Sabillo, A., Ramirez, J., Vergara, H. M., Nave, C., et al. (2014). The Role of Sdf-1α Signaling in Xenopus laevis somite Morphogenesis. Dev. Dyn. 243, 509–526. doi:10.1002/dvdy.24092
Matloubian, M., David, A., Engel, S., Ryan, J. E., and Cyster, J. G. (2000). A Transmembrane CXC Chemokine Is a Ligand for HIV-Coreceptor Bonzo. Nat. Immunol. 1, 298–304. doi:10.1038/79738
Matsuda, Y., Uno, Y., Kondo, M., Gilchrist, M. J., Zorn, A. M., Rokhsar, D. S., et al. (2015). A New Nomenclature of Xenopus laevis Chromosomes Based on the Phylogenetic Relationship to Silurana/Xenopus Tropicalis. Cytogenet. Genome Res. 145, 187–191. doi:10.1159/000381292
Matti, C., D'Uonnolo, G., Artinger, M., Melgrati, S., Salnikov, A., Thelen, S., et al. (2020). CCL20 Is a Novel Ligand for the Scavenging Atypical Chemokine Receptor 4. J. Leukoc. Biol. 107, 1137–1154. doi:10.1002/JLB.2MA0420-295RRR
Michiue, T., Yamamoto, T., Yasuoka, Y., Goto, T., Ikeda, T., Nagura, K., et al. (2017). High Variability of Expression Profiles of Homeologous Genes for Wnt, Hh, Notch, and Hippo Signaling Pathways in Xenopus laevis. Dev. Biol. 426, 270–290. doi:10.1016/j.ydbio.2016.12.006
Mishra, S.-K., Nagata, T., Furusawa, K., Sasaki, A., and Fukui, A. (2013). Expression of xSDF-1α, xCXCR4, and xCXCR7 during Gastrulation in Xenopus laevis. Int. J. Dev. Biol. 57, 95–100. doi:10.1387/ijdb.120130af
Moepps, B., Braun, M., Knöpfle, K., Dillinger, K., Knöchel, W., and Gierschik, P. (2000). Characterization of aXenopus Laevis CXC Chemokine Receptor 4: Implications for Hemato-Poietic Cell Development in the Vertebrate Embryo. Eur. J. Immunol. 30, 2924–2934. doi:10.1002/1521-4141(200010)30:10<2924::aid-immu2924>3.0.co;2-y
Moser, B., Wolf, M., Walz, A., and Loetscher, P. (2004). Chemokines: Multiple Levels of Leukocyte Migration Control. Trends Immunol. 25, 75–84. doi:10.1016/j.it.2003.12.005
Murphy, P. M. (2001). Viral Exploitation and Subversion of the Immune System through Chemokine Mimicry. Nat. Immunol. 2, 116–122. doi:10.1038/84214
Murray, P. J., and Wynn, T. A. (2011). Protective and Pathogenic Functions of Macrophage Subsets. Nat. Rev. Immunol. 11, 723–737. doi:10.1038/Nri3073
Nomiyama, H., Osada, N., and Yoshie, O. (2011). A Family Tree of Vertebrate Chemokine Receptors for a Unified Nomenclature. Dev. Comp. Immunol. 35, 705–715. doi:10.1016/j.dci.2011.01.019
Nomiyama, H., Osada, N., and Yoshie, O. (2013). Systematic Classification of Vertebrate Chemokines Based on Conserved Synteny and Evolutionary History. Genes Cel. 18, 1–16. doi:10.1111/gtc.12013
Ohtsubo, Y., Ikeda-Ohtsubo, W., Nagata, Y., and Tsuda, M. (2008). GenomeMatcher: a Graphical User Interface for DNA Sequence Comparison. BMC Bioinformatics 9, 376. doi:10.1186/1471-2105-9-376
Papp, B., Pál, C., and Hurst, L. D. (2003). Dosage Sensitivity and the Evolution of Gene Families in Yeast. Nature 424, 194–197. doi:10.1038/nature01771
Puchert, M., and Engele, J. (2014). The Peculiarities of the SDF-1/CXCL12 System: in Some Cells, CXCR4 and CXCR7 Sing Solos, in Others, They Sing Duets. Cel. Tissue Res. 355, 239–253. doi:10.1007/s00441-013-1747-y
Ratajczak, M. Z., Zuba-Surma, E., Kucia, M., Reca, R., Wojakowski, W., and Ratajczak, J. (2006). The Pleiotropic Effects of the SDF-1-CXCR4 axis in Organogenesis, Regeneration and Tumorigenesis. Leukemia 20, 1915–1924. doi:10.1038/sj.leu.2404357
Ratajczak, M. Z., Kim, C. H., Abdel-Latif, A., Schneider, G., Kucia, M., Morris, A. J., et al. (2012). A Novel Perspective on Stem Cell Homing and Mobilization: Review on Bioactive Lipids as Potent Chemoattractants and Cationic Peptides as Underappreciated Modulators of Responsiveness to SDF-1 Gradients. Leukemia 26, 63–72. doi:10.1038/leu.2011.242
Rossi, D., and Zlotnik, A. (2000). The biology of chemokines and their receptors. Annu. Rev. Immunol. 18, 217–242. doi:10.1146/annurev.immunol.18.1.217.2000
Saitou, N., and Nei, M. (1987). The Neighbor-Joining Method: a New Method for Reconstructing Phylogenetic Trees. Mol. Biol. Evol. 4, 406–425. doi:10.1093/oxfordjournals.molbev.a040454
Sankoff, D., Zheng, C., and Wang, B. (2012). A Model for Biased Fractionation after Whole Genome Duplication. BMC Genomics 13, S8. doi:10.1186/1471-2164-13-S1-S8
Schnable, J. C., Springer, N. M., and Freeling, M. (2011). Differentiation of the maize Subgenomes by Genome Dominance and Both Ancient and Ongoing Gene Loss. Proc. Natl. Acad. Sci. 108, 4069–4074. doi:10.1073/pnas.1101368108
Schweickart, V. L., Epp, A., Raport, C. J., and Gray, P. W. (2000). CCR11 Is a Functional Receptor for the Monocyte Chemoattractant Protein Family of Chemokines. J. Biol. Chem. 275, 9550–9556. doi:10.1074/jbc.275.13.9550
Session, A. M., Uno, Y., Kwon, T., Chapman, J. A., Toyoda, A., Takahashi, S., et al. (2016). Genome Evolution in the Allotetraploid Frog Xenopus laevis. Nature 538, 336–343. doi:10.1038/nature19840
Shellard, A., and Mayor, R. (2016). Chemotaxis during Neural Crest Migration. Semin. Cel Dev. Biol. 55, 111–118. doi:10.1016/j.semcdb.2016.01.031
Sievers, F., Wilm, A., Dineen, D., Gibson, T. J., Karplus, K., Li, W., et al. (2011). Fast, Scalable Generation of High‐quality Protein Multiple Sequence Alignments Using Clustal Omega. Mol. Syst. Biol. 7, 539. doi:10.1038/msb.2011.75
Strieter, R. M., Polverini, P. J., Kunkel, S. L., Arenberg, D. A., Burdick, M. D., Kasper, J., et al. (1995). The Functional Role of the ELR Motif in CXC Chemokine-Mediated Angiogenesis. J. Biol. Chem. 270, 27348–27357. doi:10.1074/jbc.270.45.27348.1995
Suzuki, A., Yoshida, H., van Heeringen, S. J., Takebayashi-Suzuki, K., Veenstra, G. J. C., and Taira, M. (2017). Genomic Organization and Modulation of Gene Expression of the TGF-β and FGF Pathways in the Allotetraploid Frog Xenopus laevis. Dev. Biol. 426, 336–359. doi:10.1016/j.ydbio.2016.09.016
Takeuchi, T., Tanigawa, Y., Minamide, R., Ikenishi, K., and Komiya, T. (2010). Analysis of SDF-1/CXCR4 Signaling in Primordial Germ Cell Migration and Survival or Differentiation in Xenopus laevis. Mech. Dev. 127, 146–158. doi:10.1016/j.mod.2009.09.005
Tan, M. H., Au, K. F., Yablonovitch, A. L., Wills, A. E., Chuang, J., Baker, J. C., et al. (2013). RNA Sequencing Reveals a Diverse and Dynamic Repertoire of the Xenopus Tropicalis Transcriptome over Development. Genome Res. 23, 201–216. doi:10.1101/gr.141424.112
Tanegashima, K., Suzuki, K., Nakayama, Y., Tsuji, K., Shigenaga, A., Otaka, A., et al. (2013). CXCL14 Is a Natural Inhibitor of the CXCL12-CXCR4 Signaling axis. FEBS Lett. 587, 1731–1735. doi:10.1016/j.febslet.2013.04.046
Tiffany, H. L., Lautens, L. L., Gao, J.-L., Pease, J., Locati, M., Combadiere, C., et al. (1997). Identification of CCR8: a Human Monocyte and Thymus Receptor for the CC Chemokine I-309. J. Exp. Med. 186, 165–170. doi:10.1084/jem.186.1.165
Van de Peer, Y., Maere, S., and Meyer, A. (2009). The Evolutionary Significance of Ancient Genome Duplications. Nat. Rev. Genet. 10, 725–732. doi:10.1038/nrg2600
Watanabe, M., Yasuoka, Y., Mawaribuchi, S., Kuretani, A., Ito, M., Kondo, M., et al. (2017). Conservatism and Variability of Gene Expression Profiles Among Homeologous Transcription Factors in Xenopus laevis. Dev. Biol. 426, 301–324. doi:10.1016/j.ydbio.2016.09.017
Wedemeyer, M. J., Mahn, S. A., Getschman, A. E., Crawford, K. S., Peterson, F. C., Marchese, A., et al. (2020). The Chemokine X-Factor: Structure-Function Analysis of the CXC Motif at CXCR4 and ACKR3. J. Biol. Chem. 295, 13927–13939. doi:10.1074/jbc.RA120.014244
Yang, Z. (2007). PAML 4: Phylogenetic Analysis by Maximum Likelihood. Mol. Biol. Evol. 24, 1586–1591. doi:10.1093/molbev/msm088
Zhao, Z.-H., Meng, T.-G., Li, A., Schatten, H., Wang, Z.-B., and Sun, Q.-Y. (2020). RNA-Seq Transcriptome Reveals Different Molecular Responses during Human and Mouse Oocyte Maturation and Fertilization. BMC Genomics 21, 475. doi:10.1186/s12864-020-06885-4.2020
Zlotnik, A., and Yoshie, O. (2000). Chemokines: A New Classification System and their Role in Immunity. Immunity 12, 121–127. doi:10.1016/s1074-7613(00)80165-x.2000
Keywords: chemokine, homeolog, genome duplication, allotetraploid, subfunctionalization, neofunctionalization, Xenopus, amphibian
Citation: Fukui A and Matsunami M (2022) Gene Structure Analysis of Chemokines and Their Receptors in Allotetraploid Frog, Xenopus laevis. Front. Genet. 12:787979. doi: 10.3389/fgene.2021.787979
Received: 01 October 2021; Accepted: 23 December 2021;
Published: 20 January 2022.
Edited by:
Yukio Nagano, Saga University, JapanReviewed by:
Ei"ichi Iizasa, Kagoshima University, JapanLeon Grayfer, George Washington University, United States
Copyright © 2022 Fukui and Matsunami. This is an open-access article distributed under the terms of the Creative Commons Attribution License (CC BY). The use, distribution or reproduction in other forums is permitted, provided the original author(s) and the copyright owner(s) are credited and that the original publication in this journal is cited, in accordance with accepted academic practice. No use, distribution or reproduction is permitted which does not comply with these terms.
*Correspondence: Akimasa Fukui, ZnVrdWlAYmlvLmNodW8tdS5hYy5qcA==