- 1School of Life Science, Hunan University of Science and Technology, Xiangtan, China
- 2Hunan Key Laboratory of Economic Crops Genetic Improvement and Integrated Utilization, Xiangtan, China
- 3Oilseed Research Institute, Hunan Agricultural University, Changsha, China
- 4Key Laboratory of Biology and Genetic Improvement of Oil Crops, Ministry of Agriculture and Rural Affairs, Oil Crops Research Institute of Chinese Academy of Agricultural Sciences, Wuhan, China
Anthocyanins contribute to most colors of plants and play protective roles in response to abiotic stresses. Brassica napus is widely cultivated worldwide as both an oilseed and a vegetable. However, only several high anthocyanin-containing cultivars have been reported, and the mechanisms of anthocyanin accumulation have not been well-elucidated in B. napus. Here, the phenotype, comparative whole-genome identification, and gene expression analysis were performed to investigate the dynamic change of the anthocyanin content and the gene expression patterns of anthocyanin biosynthetic genes (ABGs) in B. napus. A total of 152 ABGs were identified in the B. napus reference genome. To screen out the critical genes involved in anthocyanin biosynthesis and accumulation, the RNA-seq of young leaves of two B. napus lines with purple leaves (PL) or green leaves (GL), and their F1 progeny at 41, 91, and 101 days were performed to identify the differentially expressed genes. The comparative expression analysis of these ABGs indicated that the upregulation of TT8 together with its target genes (such as DFR, ANS, UFGT, and TT19) might promote the anthocyanin accumulation in PL at the early developmental stage (41–91 days). While the downregulation of those ABGs and anthocyanin degradation at the late developmental stage (91–101 days) might result in the decrease in anthocyanin accumulation. Our results would enhance the understanding of the regulatory network of anthocyanin dynamic accumulation in B. napus.
Introduction
Anthocyanins are a class of natural pigments responsible for the orange, red to purple, and blue colors of many fruits and vegetables (Buer et al., 2010). Meanwhile, anthocyanins play protective roles by scavenging reactive oxygen species during abiotic stresses and may also serve to reduce cardiovascular risk factors (Butelli et al., 2008; de Pascual–Teresa et al., 2010; Kim et al., 2017).
The anthocyanin biosynthetic genes (ABGs) are well-characterized in a variety of plants, and various structural and regulatory genes play important roles in anthocyanin biosynthesis (Lepiniec et al., 2006; Xu et al., 2013; Liu et al., 2017). The structural genes leading to anthocyanin biosynthesis are usually divided into early biosynthetic genes (EBGs) and late biosynthetic genes (LBGs). EBGs (chalcone synthase, CHS; chalcone isomerase, CHI; flavanone 3-hydroxylase, F3H; flavonoid 3’-hydroxylase, F3’H) are the common flavonoid pathway genes which are involved in the biosynthesis of all downstream flavonoids. LBGs (dihydroflavonol 4-reductase, DFR; anthocyanidin synthase/leucoanthocyanin dioxygenase, ANS/LDOX; uridine diphosphate-glucose: flavonoid 3-O-glucosyltransferase, UFGT) are genes regulating the biosynthesis of anthocyanins (Petroni and Tonelli, 2011; Yan et al., 2014; Liu et al., 2018). The EBGs are regulated by a class of R2R3–MYB transcription factors, while the regulation of LBGs requires a ternary complex of MYB–bHLH–WD40 (MBWs), which binds the promoters of target genes and then activate their transcription, leading to a higher accumulation of the anthocyanin level (Lepiniec et al., 2006; Xu et al., 2014; Kim et al., 2017). In Arabidopsis thaliana, the overexpression of MYB regulators, such as PAP1, PAP2, MYB113, and MYB114 could result in a substantial increase in anthocyanins (Gonzalez et al., 2008). In Brassica, transposon insertion and point mutation independently activate the expression of the BoMYB2 gene in purple cultivars of Brassica oleracea (Yan et al., 2019), while a DNA sequence insertion in the first intron greatly reduces the transcription of BjMYB2 in green cultivars of Brassica juncea (Heng et al., 2020a). The overexpression of BjTT8 and its target genes which are involved in late anthocyanin biosynthesis and transport, account for the increasing levels of anthocyanin accumulation in purple leaves of B. juncea (Mushtaq et al., 2016; Heng et al., 2020b; Zhang et al., 2020). However, the expression of BoMYBL2-1 is inversely correlated to the anthocyanin content, and purple color in B. oleracea results from a loss of BoMYBL2-1 expression (Song et al., 2018). It has been demonstrated that some environmental factors, such as light or temperature, could also influence anthocyanin accumulation by modulating the expression of ABGs (Nguyen et al., 2015; Kim et al., 2017).
Brassica napus (AACC, 2n = 38), formed by hybridization between the diploid ancestors of B. rapa (AA, 2n = 20) and B. oleracea (CC, 2n = 18), is widely cultivated worldwide as both an oilseed and a vegetable (Fujii and Ohmido, 2011; Ton et al., 2020). The purple leaf trait and the mechanisms underlying the anthocyanin accumulation has been widely documented in B. rapa and B. oleracea (Zhang et al., 2014 and, 2015; He et al., 2016; Xie et al., 2016; Song et al., 2018; Guo et al., 2019; Yan et al., 2019); however, few studies have investigated Brassica napus anthocyanin biosynthesis. (Goswami et al., 2018). Previously, the BnaA.PL1 gene, which confers to purple leaves in B. napus, was mapped at the end of chromosome A03 (Li et al., 2016). Transcriptional analyses of a high-anthocyanin resynthesized B. napus line by crossing anthocyanin-rich B. rapa and B. oleracea suggest that MYB111, TT8, and TT19 may be responsible for the higher anthocyanin accumulation (Goswami et al., 2018). However, due to the lack of high anthocyanin mutants and genome information, the regulation of the anthocyanin biosynthetic pathway in the economically important plant B. napus has not been fully elucidated to date. With the recently released high-quality B. napus reference genomes, comparative genomic and transcriptomic analyses are now available for the genome-wide identification of anthocyanin biosynthetic genes responsible for the leaf color (Rousseau–Gueutin et al., 2020; Song et al., 2020; Mohd Saad et al., 2021). Herein, the anthocyanin contents of two B. napus lines with purple leaves or green leaves, and their F1 progeny at different developmental stages were measured to investigate the dynamic change trend. A combination of comparative genomic and transcriptomic analysis was performed to identify the ABGs in the B. napus genome and to investigate the gene expression patterns of these ABGs in leaves with different colors. Taken together, our results provide a comprehensive perspective of the transcriptional regulation of the anthocyanin biosynthetic pathway in B. napus.
Materials and Methods
Plant Materials
One B. napus inbred lines which exhibited purple leaves (abbreviated as PL) at the seedling stage and ZS11 (Zhongshuang 11) which showed green leaves (abbreviated as GL), as well as the F1 progeny by a cross between these two lines were used in this study. Seeds of PL, GL, and F1 were sown directly on September 30th, 2020, and three seedlings of each were maintained in the same pot (43 cm length × 40 cm wide × 25 cm high) to minimize the developmental or environmental effects. All the plants were grown under natural lighting in a greenhouse located at the Hunan University of Science and Technology (27°91′05″N, 112°92′60′'E, China). Considering that the leaf color of PL showed a dynamic change from light purple to dark purple and then to green, the fresh leaves at the seedling stage (<101 days after sowing) and bolting stage (150 days after sowing) were collected and stored at −80°C.
Analysis of Anthocyanins
The total anthocyanin content was measured using the method described previously (Li et al., 2016; Zhang et al., 2020). In brief, the young leaves PL, GL, and F1 with three replicates were ground into powder after low-temperature freeze drying (SJIA-10N, Ningbo, China). Then 0.1 g powder was mixed with 1 ml of 95% ethanol: 1.5 M HCl (85:15, v/v) for 20 h at 4°C in the dark with moderate shaking. The aqueous phase was collected after centrifuging at 12,000 ×g for 10 min, and the absorbance was subsequently measured at 530 nm using a Cary 60 UV-Vis (Agilent Technologies, Palo Alto, United States).
Identification of Anthocyanin Biosynthetic Genes in B. napus
Previously, the ABGs in B. rapa were well-characterized based on the syntenic analysis results between B. rapa and A. thaliana (Guo et al., 2014). Following the method provided by Cheng et al. (2012) and Guo et al. (2014 and 2019), syntenic analysis across B. napus, A. thaliana, B. rapa, and B. oleracea was performed, and ABGs were identified using SynOrths (http://brassicadb.cn/#/Download/) with the same parameters (NumQ = 20, NumR = 100, RatioQR = 0.2). Gene pairs that are the best hits or with Blastp e-values <1E−20 were selected for further analysis. The reference genome of A. thaliana was downloaded from BRAD (http://brassicadb.cn), as described before. The B. oleracea (genotype HDEM) and B. rapa (genotype Z1) reference genomes were obtained from https://www.genoscope.cns.fr/externe/plants (Rousseau-Gueutin et al., 2020). The B. napus (genotype ZS11) reference genome was obtained from http://cbi.hzau.edu.cn/bnapus/(Song et al., 2020), respectively. Integrative software TBtools was used for determining the chromosomal locations of ABGs of B. napus (Chen et al., 2020).
RNA Extraction and RNA-Seq
The entire of fully expanded young leaves from PL, GL, and F1 with three biological replicates at 41, 91, and 101 days after sowing were collected for further RNA-seq, respectively. The total RNA of samples was extracted using the RNA Easy Fast Plant Tissue Kit (TIANGEN, Beijing, China) according to a standard protocol. After that, 1.5% agarose gel, a NanoPhotometer® spectrophotometer (IMPLEN, CA, United States), and a Bioanalyzer 2,100 system (Agilent Technologies, CA, United States) were also used to check the purity and integrity of RNA. Samples with RIN scores≥7.5 and OD260/280 = 1.8–2.2 were utilized for further sequencing. Sequencing libraries were generated using the NEBNext® UltraTM RNA Library Prep Kit for Illumina® (NEB, United States), following the manufacturer’s recommendations. The libraries were sequenced on an Illumina NovaSeq 6,000 platform and 150-bp paired-end reads were generated by Shanghai Majorbio Bio-pharm Technology Co., Ltd.
Reads Filtering and Mapping
After the high-throughput sequencing, the low-quality reads which contained adapters and ploy-N were trimmed using Trimmomatic. Then, the clean reads were mapped to the high-quality B. napus (genotype ZS11; http://cbi.hzau.edu.cn/bnapus/) reference genomes using TopHat2 (http://tophat.cbcb.umd.edu/) with the default parameters. Software RSEM was used for read counting, and DESeq2 was used for differential expression analysis. Genes with adjusted p-values < 0.05 were classified as differentially expressed. The expression levels of individual genes were also quantified using the transcripts per million (TPM) method. The KEGG enrichment analysis of differentially expressed genes was implemented using the free online platform of Majorbio Cloud Platform (www.majorbio.com). KEGG terms with corrected p-values less than 0.05 were considered to be significantly enriched.
Validation of the Expression Data by qRT-PCR
The extracted RNA was also used for qRT–PCR. First-strand cDNA synthesis was performed using a Tsingke Goldenstar RT6 cDNA Synthesis Kit ver.2 (Tsingke, Beijing, China). Differentially expressed ABGs were selected, and specific primers were designed for qRT–PCR using Oligo 7 and NCBI Primer-BLAST (https://www.ncbi.nlm. nih. gov/tools/primer-blast/). The β-actin gene (GenBank accession No. AF111812) was used as an internal control, and SYBR Premix Ex TaqII with a Bio-Rad CFX96 Real-Time Detection System was used as described previously (Zhang et al., 2018).
Results
Phenotypic Characterization of Purple Leaves and Green Leaves
During the seedling stage, the PL displayed purple pigments in the surface and thin vein of leaves, while the F1 only showed faint purple in the leaf surface (Figures 1A,B). The microscopic observation of sections revealed that the purple pigments were mainly accumulated in the adaxial epidermis and subepidermal cells of PL leaves, while it decreased in the top cell layer of F1 (Figures 1C,D). In contrast, GL with common green color only exhibited green chlorophyll layers in leaves (Figure 1E).
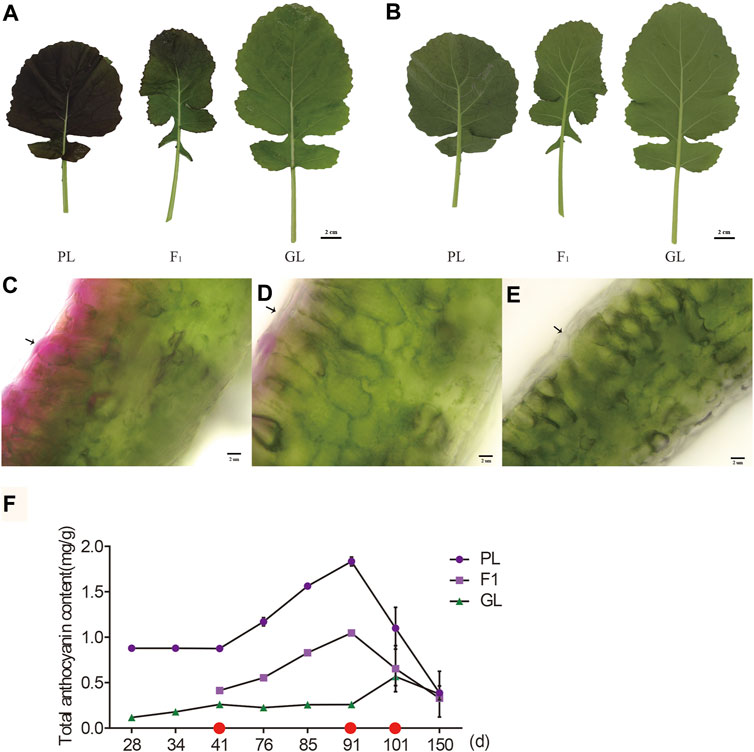
FIGURE 1. Phenotypes of B. napus with different leaf colors. The upper (A) and lower epidermis (B) of PL, F1, and GL (scale bar = 2 cm). Transverse section of leaf from PL (C), F1 (D), and GL (E) with different colors (scale bar = 2 μm). (F) The content of total anthocyanin accumulation at different stages. Values represent means ± SD from three biological replicates. Red dots in the x-axis indicate days for RNA-seq sampling.
Although the average anthocyanin contents in PL (1.09 mg/kg DW) were about 3.9-fold higher than those in GL (0.28 mg/kg DW, p < 0.05, paired Students’ t-test), they varied at different developmental stages in the leaves of PL (Figure 1F). During the seedling stage (<101 days), the total anthocyanin contents of PL were stable at initial weeks (0.88 mg/kg DW, 28–41 days after sowing) and increased after that (76–91 days) but dropped dramatically from 1.83 mg/kg (91 days) to 1.10 mg/kg (101 days). No significant difference (p > 0.05, t-test) was observed between PL (0.39 mg/kg) and GL (0.37 mg/kg) at the bolting stage (150 days), which was correlated with the color change from purple to green in the leaves of PL. The average anthocyanin contents in F1 (0.64 mg/kg DW) were higher than those in GL but significantly lower than those in PL (p < 0.05, paired t-test), suggesting that incompletely dominant gene or genes might control the purple leaf trait in B. napus.
Genome-Wide Identification of Anthocyanin Biosynthetic Genes in B. napus
Based on the method from a previous study (Guo et al., 2019; Zhang et al., 2020), a total of 74, 93, and 152 genes were identified to be ABGs in B. rapa, B. oleracea, and B. napus (Supplementary Table S1). As compared with 41 ABGs in A. thaliana, FLS2, FLS4, FLS5, FLS6, CPC, MYB111, MYB113, and MYB114 have not been identified in B. rapa, B. oleracea, and B. napus; these observations suggested that these genes were lost following the whole-genome triplication. Among the 152 genes identified in B. napus, 98 were classified into structural genes, 50 were regulatory genes, and 4 were transport genes. The number of these three types of genes was quadrupled as compared with A. thaliana, which may be caused by whole-genome triplication and subsequent allopolyploidization. Using the total A. thaliana and B. napus genes as references, the 152 ABGs represent 0.15% of the 100,919 annotated B. napus genes, which was similar to that in A. thaliana (0.15%). Meanwhile, the overall expansion levels of structural, regulatory, and transport genes also exhibited no significant difference to the whole genome (Table 1, chi-squared test, p > 0.05).

TABLE 1. Comparison of the number of ABGs in Arabidopsis thaliana, Brassica rapa, Brassica oleracea, and Brassica napus.
Among these 152 ABGs, 150 were anchored to the 19 chromosomes of B. napus, in which 66 genes were distributed on the A subgenome and other 84 genes were located on the C subgenome, ranging from 1 to 16 on each chromosome (Figure 2A). Among the 150 anchored ABGs, 33.3% (50/150) were originated from tandem duplication events. The 50 tandemly duplicated genes could be divided into 22 groups, with 9 groups on the A subgenome (19/50) and 13 groups on the C subgenome (31/50), of which 19 groups contained two genes each, two groups contained 3 genes each, and 1 group contained 6 genes each. Except for one set of tandemly duplicated gene on the chromosome A02 and two set of tandemly duplicated genes on chromosome C02 and chromosome C05, respectively, all the tandemly duplicated genes had homologous copies both in A and C subgenomes. These indicated that most tandem duplication events occurred before polyploidization. The syntenic analysis of ABGs among A. thaliana, B. rapa, B. oleracea, and B. napus showed that the whole-genome triplication of Brassica and subsequent allopolyploidization contributed to the expansion of ABGs in B. napus (Figure 2B). Although some genes were lost as a result of gene fractionation that occurred following the whole-genome triplication, a large proportion of ABGs exhibited syntenic between B. napus and B. rapa/B. oleracea.
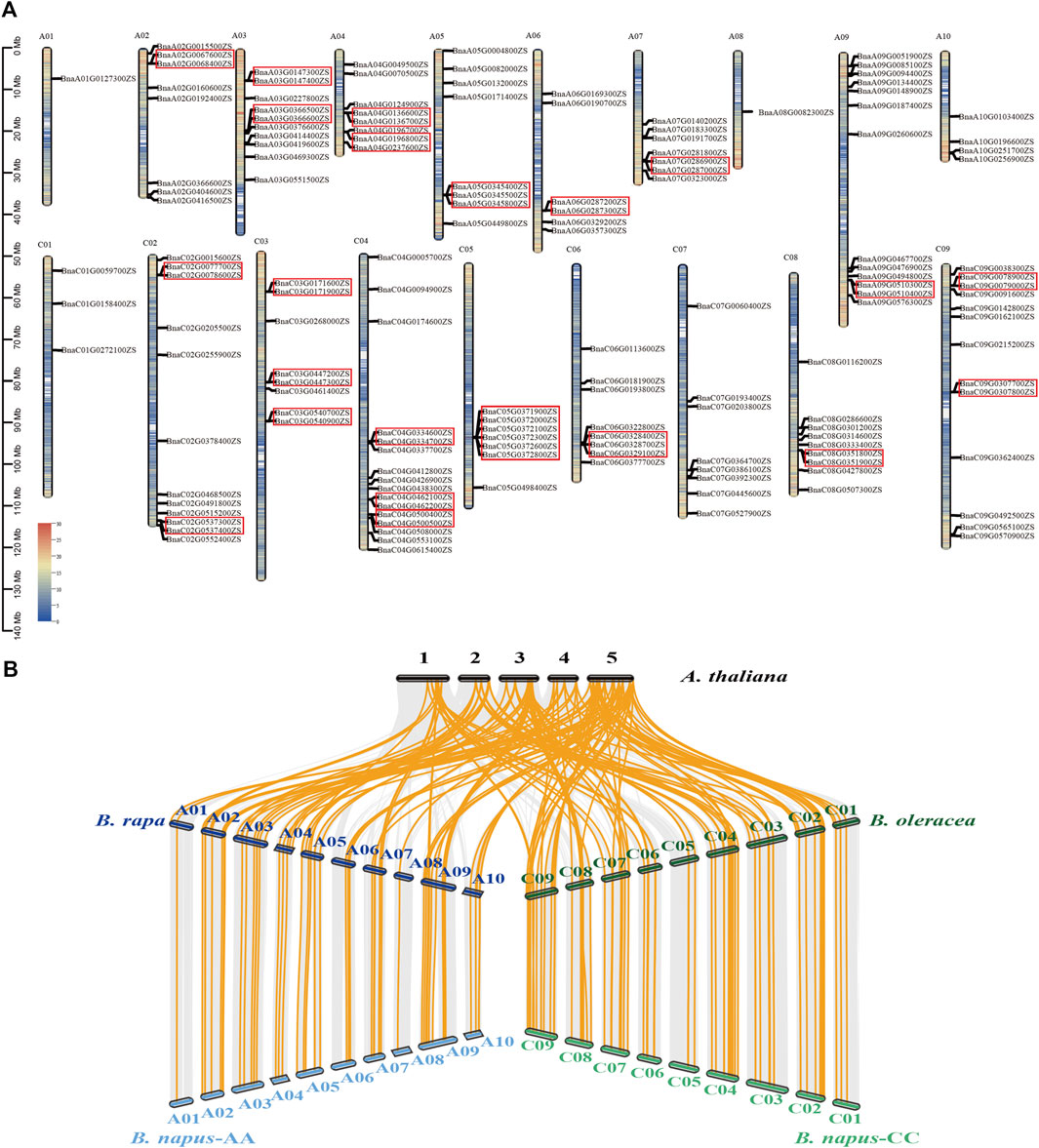
FIGURE 2. Distribution and syntenic analysis of ABGs in Brassica napus. (A) The color on the chromosome indicates the gene density of all the genes. Redder color indicates the higher gene density, while the bluer color indicates lower gene density. The red boxes indicate tandem duplications. (B) The orange lines indicate the ABGs exhibited syntenic between A. thaliana, B. rapa, B. oleracea, and B. napus.
Comparative Transcriptome Analysis of B. napus With Different Leaf Colors
To investigate the molecular mechanisms of anthocyanin accumulation, young leaves with three biological replicates at three different developmental stages (41, 91, 101 days) from GL, F1, and PL were collected for library construction (3 × 3 × 3 = 27 libraries) and high-throughput RNA sequencing (Supplementary Table S2). In summary, about 127.0 G clean reads were obtained after filtering, and more than 90% of them can map on the B. napus reference genome released recently (Song et al., 2020). Gene expression levels were calculated using the TPM methods, and gene expression correlation showed good consistence between replications (Supplementary Figure S1).
The comparative transcriptome analysis of B. napus among GL, F1, and PL showed that the number of DEGs was varied in different comparisons at different developmental stages (Figure 3A). The highest number of DEGs was found between PL and GL at 91 days when PL showed highest anthocyanin content, for 6,263 genes were upregulated and 3,795 genes were downregulated. While the number of DEGs were decreased between F1 and the parental lines (F1 vs GL and PL vs F1) with the development of plants, and the lowest number of DEGs was found between F1 and GL at 101 days when the anthocyanin content showed no significant difference. About 8,167, 10,058, and 8,291 genes were differentially expressed between PL and GL at 41, 91, and 101 days after sowing, and 4,054 of them were shared in the aforementioned comparisons (Figure 3B). The KEGG analysis of those DEGs between PL and GL suggested that the genes involved in anthocyanin biosynthesis were significantly enriched at 41, 91, and 101 days (Figure 3C).
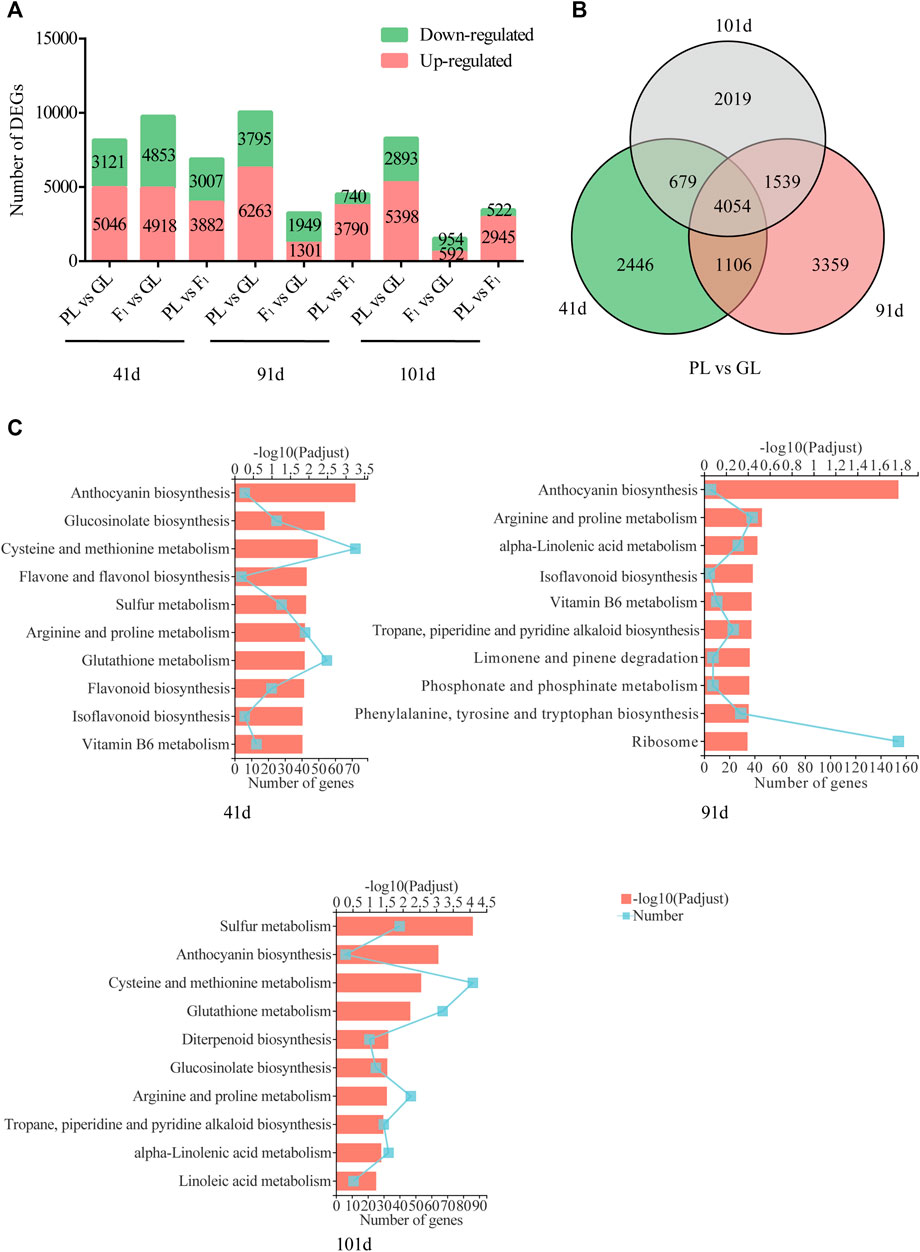
FIGURE 3. Summary of differentially expressed genes between GL and PL. (A) The number of DEGs among GL, F1, and PL. (B) Venn diagrams of differentially expressed genes between PL and GL shared at different developmental stages. (C) The KEGG enrichment of DEGs between PL and GL at different developmental stages.
The Expression Analysis of Genes in the Anthocyanin Biosynthetic Pathway in the Leaves of B. napus
To identify key genes responsible for leaf color variation in B. napus, the expression patterns of ABGs were investigated. Among the 152 ABGs, the expression levels of 57 genes were extremely low (average TPM value < 1) or undetected (Supplementary Table S3). Of the other 95 expressed ABGs, 22 genes were differentially expressed between PL and GL. These 22 DEGs are equally distributed in the A subgenome (11/22) and C subgenome (11/22), while the average expression level of the 11 DEGs in C subgenome was higher than that in the A subgenome (p < 0.05, two-tailed Student’s t-test; Figure 4B). Based on these results, the expressed levels of DEGs in the anthocyanin biosynthetic pathway from different leaf color samples at different stages were demonstrated (Figure 4). The majority of upstream genes in the anthocyanin biosynthetic pathway (45/46 in the phenylpropanoid pathway and 32/35 in EBGs) were not differentially expressed, implying that these genes might not play important roles in anthocyanin accumulation differences. While these genes which are involved in LBGs, such as DFR (3/3), ANS (3/3), and UFGT (6/9), as well as TT19 (3/4) and TT8 (2/3) were significantly upregulated in PL, as compared with GL at 41 and 91 days after sowing (Supplementary Table S3, Figure 4A). As to F1, the overall expression level of 22 ABGs was significantly higher than that in GL at 41, 91, and 101 days but lower than that in PL at 41 and 91 days (p < 0.05, two-tailed Student’s t-test; Figure 4B). Except for one C4H gene (BnaA03G0147400ZS), all the other genes (21/22) involved in early or late anthocyanin biosynthetic exhibited the similar trend of expression level PL > F1>GL at 41 and 91 days (Figure 4A). Quantitative RT-PCR results also confirmed the overexpression of these genes in PL and F1, suggesting that these genes might be the essential factors for anthocyanin accumulation at the early stage (Supplementary Figure S2, Supplementary Table S4). It is also worthy to note that the expression levels of most upregulated (p < 0.01, paired t-test) genes in PL at 41 and 91 days were decreased due to the similar levels of GL (p = 0.1175, paired t-test) at 101 days, which was consistent with the decrease in anthocyanin contents and the leaf color phenotype in PL (Figure 4B and Figure 1F).
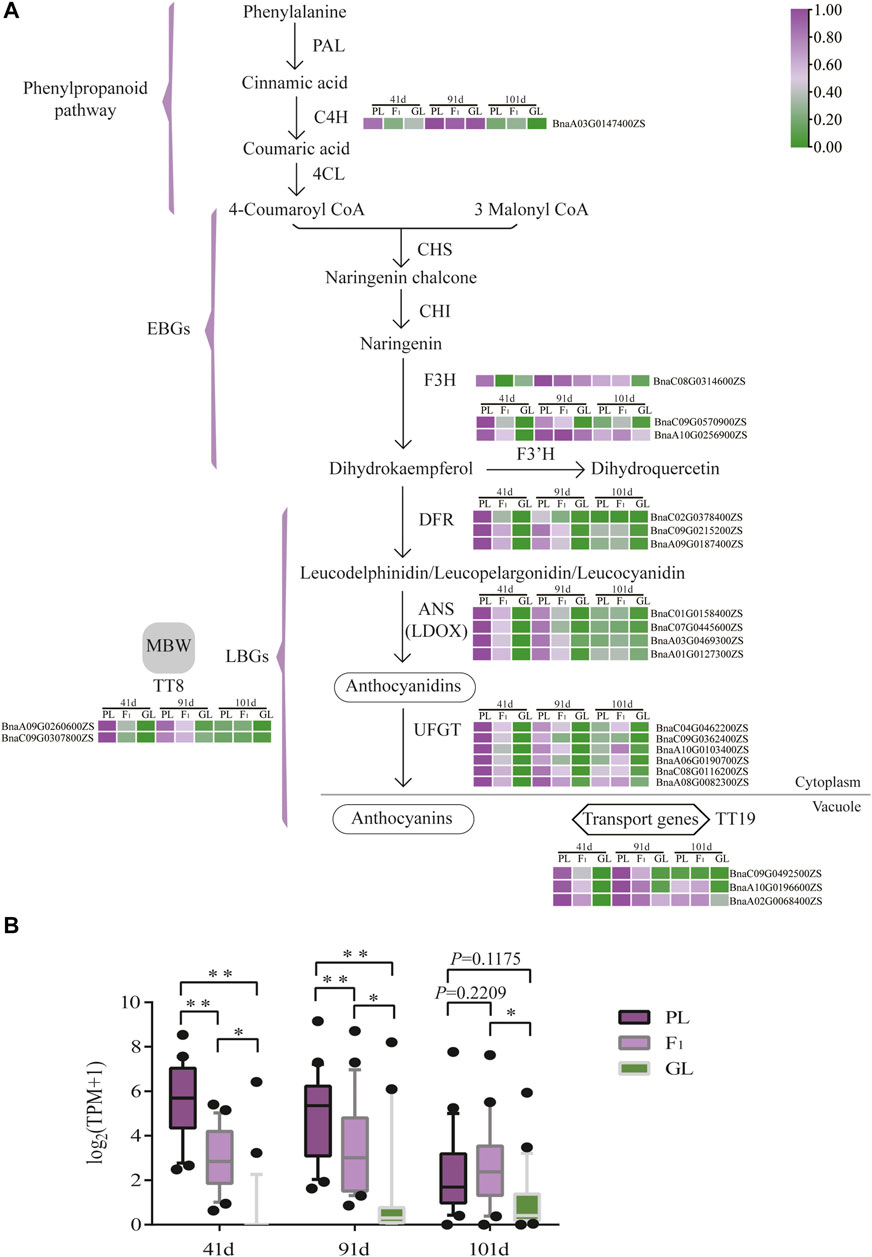
FIGURE 4. Anthocyanin biosynthetic pathway and expression patterns of ABGs in B. napus. (A) The expression level of ABGs which are differentially expressed between PL and GL is shown in the pathway. Purple and green colors are used to represent high to low expression levels, respectively. The color scale corresponds to the mean-centered log2-transformed average TPM values from three replicates. (B) The overview of ABG expression, which was differentially expressed at different stages. Asterisks indicate statistical differences between each other (two-tailed Student’s t-test, ** indicates p < 0.01; * indicates p < 0.05).
To further understand the relationship between expression patterns of the 22 differentially expressed ABGs and anthocyanin accumulation, correlation coefficients were calculated (Supplementary Figure S3). Significant correlations (p < 0.05, Pearson correlation coefficient) between expression and anthocyanin contents were observed in UFGT (BnaA08G0082300ZS, r = 0.60), TT8 (BnaA09G0260600ZS, r = 0.62), TT19 (BnaC09G0492500ZS, r = 0.76; BnaA10G0196600ZS, r = 0.82; BnaA02G0068400ZS r = 0.72), F3H (BnaC08G0314600ZS, r = 0.79), and F3 'H (BnaA10G0256900ZS, r = 0.64).
Discussion
In plants, anthocyanins not only confer colorful appearances but also play protective roles against various abiotic and biotic stresses; thus, the mechanisms underlying anthocyanin accumulation have been widely studied in many plant systems (Liu et al., 2015; Xu et al., 2015; Zhang et al., 2017; Castillejo et al., 2020; Yan et al., 2011). In Brassica, the drastic differences in anthocyanin accumulation and distribution could arise from cultivar and genetic discrepancies. Herein, our results found that the anthocyanins were mainly accumulated in the adaxial epidermis of PL leaves, which was consistent with the findings in B. rapa but inconsistent with that in B. juncea and B. oleracea (Zhang et al., 2015; He et al., 2016; Mushtaq et al., 2016; Heng et al., 2020b; Zhang et al., 2020). The anthocyanin contents in PL were significantly higher than those in GL but varied at different developmental stages. The highest content in PL was found 91 days after sowing at the seedling stage but dropped dramatically to the same level of GL after 150 days at the bolting stage (Figure 1). The dynamic changes in the anthocyanin content were correlated with the phenotype change from purple to green in the leaves, suggesting that anthocyanin accumulation was a response to leaf color in B. napus and influenced by the environmental and developmental signals. Previous studies demonstrated that anthocyanin biosynthesis could be regulated by many environmental factors, such as light, temperature, and various types of biotic and abiotic stresses, via a network comprising many transcription factors (Nguyen et al., 2015; He et al., 2016; Wang et al., 2016; Kim et al., 2017). For example, flavonoid biosynthesis might be induced to accumulate anthocyanin in the red-leaved accessions of ornamental kale (B. oleracea var. acephala), when plants were under low temperature (Guo et al., 2019). Consistent with those studies, the anthocyanin content in PL also increased with the decrease in temperature (from 22°C at 41 days to 7°C at 91 days) and decreased with the increase in temperature (7°C at 91 days to 11°C at 101 days). Thus, we hypothesized that the purple color intensity in B. napus leaves, which was caused by differences in total anthocyanin content, could be regulated by a diverse array of exogenous and endogenous environmental factors.
A previous study suggested that 73 ABGs in B. rapa, 81 genes in B. oleracea, and 129 genes in B. juncea were found as orthologs of 41 ABGs in A. thaliana, respectively (Guo et al. 2014; Guo et al. 2019; Zhang et al. 2020). In the present study, a total of 74, 93, and 152 genes were identified to be ABGs in B. rapa, B. oleracea, and B. napus using the reference genome released recently (Rousseau-Gueutin et al., 2020; Song et al., 2020; Supplementary Table S1). The B. napus genome has undergone a whole-genome triplication of Brassica and recent allopolyploidy between B. rapa and B. oleracea because of its divergence from the A. thaliana; thus, the copy number of ABGs in B. napus has been expanded following the whole-genome duplication events (Chalhoub et al., 2014). However, these events were followed by diploidization that involved substantial genome reshuffling and gene losses, which might lead to different copy number retention of the homologous genes of A. thaliana (Wang et al., 2011; Liu et al., 2014). The comparative and syntenic analyses of ABGs between Brassica and A. thaliana genome suggested that gene losses have mainly occurred following the whole-genome triplication between B. rapa/B. oleracea and A. thaliana, rather than the allopolyploidy between B. napus and B. rapa/B. oleracea (Figure 2), which was in accordance with the findings in B. juncea (Zhang et al., 2020).
The comparative transcriptome analysis of B. napus with different leaf colors found that about 22 ABGs were upregulated in purple leaves relative to green leaves. As compared with two parental lines, the expression level of 21 ABGs in F1 was also higher than that in GL but lower than that in PL at 41 and 91 days, which was in accordance with the phenotype of the intermediate anthocyanin content (Figure 1F, Figure 4). Among the 22 upregulated genes, late ABGs (DFR, ANS, and UFGT), positive regulatory genes (TT8), and transport genes (TT19) exhibited significant correlations between their expression levels and anthocyanin contents, indicating that these genes might play important roles in anthocyanin accumulation differences (Supplementary Figure S3). The overexpression of genes involved in late anthocyanin biosynthesis, positive regulatory as well as transport genes, is usually responsible for increased levels of anthocyanin accumulation in plants (Sun et al., 2012; Zhang et al., 2015; He et al., 2016; Kim et al., 2017; Goswami et al., 2018; Heng et al., 2020b). The ectopic expression of AtDFR resulted in increased accumulation of anthocyanins in B. napus plants (Kim et al., 2017). While AtTT19 functioned as a carrier to transport anthocyanin from the cytosol to tonoplasts, and the tt19 mutant barely accumulates anthocyanins (Sun et al., 2012). In vegetative tissues, DFR, ANS, UFGT, and TT19 were the direct targets of the MBW ternary complex, and the expression of these genes relied on the transcriptional activity of R2R3-MYB, bHLH, and WDR proteins (Xu et al., 2014). As a central component in the MBW complex, TT8 was a key regulator and could activate the anthocyanin biosynthesis in B. rapa, B. oleracea, and B. juncea (He et al., 2016; Li et al., 2016; Guo et al., 2019; Heng et al., 2020b). Consistent with previous studies, TT8 and TT19 displayed higher regulation levels in PL at 41 and 91 days, as well as significantly high correlation between the expression and anthocyanin contents at different stages, suggesting that TT8 and TT19 might play vital roles in anthocyanin accumulation (Figures 4,5). Hence, it is speculated that the upregulation of TT8 together with its target genes (such as DFR, ANS, UFGT, and TT19) might promote the anthocyanin accumulation in B. napus under low temperature at the early stage (41–91 days). Notably, the expression levels of most upregulated genes in PL at 91 days were decreased to similar levels of GL at 101 days, which was consistent with the decrease in anthocyanin contents in PL (Figure 5). The downregulation of those ABGs and time-consuming anthocyanin degradation under higher temperature at the late developmental stage (91–101 days) might reduce the total anthocyanin accumulation, leading to a color change from purple to green in the leaves of PL.
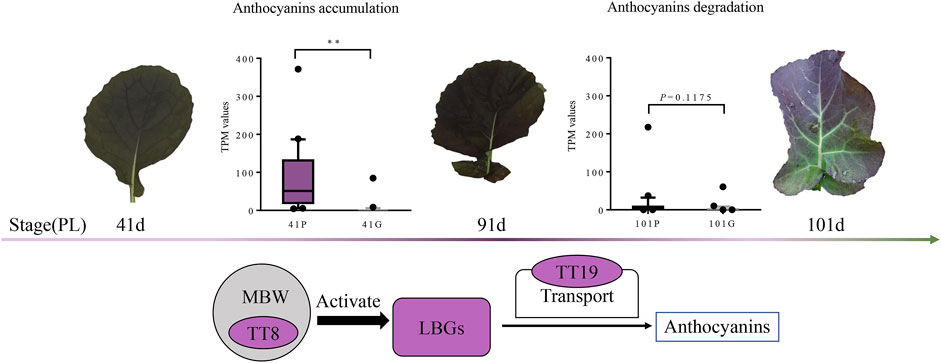
FIGURE 5. Model of anthocyanins accumulation in Brassica napus. The TT8, TT19, and LBGs are significantly expressed in PL compared with those in GL from 41 to 91 days, (p < 0.01). At these developmental stages, anthocyanin contents increase, and the purple pigments are accumulated in the adaxial epidermis and subepidermal cells. The expression levels of these genes are decreased in PL and exhibited no significant difference between that of GL from 91 to 101 days, (p = 0.1175). The anthocyanin degradation at this developmental stage might reduce the total anthocyanin accumulation and result in the leaves to gradually turn to green. Circles filled with purple color indicate upregulated genes expressed in PL at 41 and 91 days.
Conclusion
We found that the anthocyanins were mainly accumulated in the adaxial epidermis and could be influenced by the environmental and developmental signals. A total of 152 ABGs were identified in the B. napus genome, and they have been expanded during the whole-genome duplication events, but some of the genes were lost following genome diploidization. The expression patterns of these ABGs in different leaf types indicated that the upregulation of TT8 together with its target genes (such as DFR, ANS, UFGT, and TT19) might promote the anthocyanin accumulation in B. napus at early stages (41–91 days), while the downregulation of those ABGs and anthocyanin degradation at late developmental stages (91–101 days) might result in the reduction of anthocyanin accumulation. Taken together, our results enhance the understanding of the genetic mechanisms and regulatory network of anthocyanin accumulation in B. napus.
Data Availability Statement
The datasets presented in this study can be found in online repositories. The names of the repository/repositories and accession number(s) can be found at https://ngdc.cncb.ac.cn/gsa/, CRA004724.
Author Contributions
MY, ZL, and DwZ contributed to conception and design of the study. DH, LL, DgZ, and TL performed the sample collection, RNA isolation and gene expression analysis. DwZ, DH, LK, and JW performed the bioinformatics analysis. DwZ and DH wrote the manuscript. All authors read and approved the final manuscript.
Funding
This work was funded by the National Key Research and Development Program of China (2018YFD1000904), National Natural Science Foundation of China (U19A2029), Open Project of Key Laboratory of Biology and Genetic Improvement of Oil Crops, Ministry of Agriculture and Rural Affairs (KF2020011), Open Funding of Hunan Key Laboratory of Economic Crops Genetic Improvement and Integrated Utilization (E22115), and Postgraduate Scientific Research Innovation Project of Hunan Province (CX20201007).
Conflict of Interest
The authors declare that the research was conducted in the absence of any commercial or financial relationships that could be construed as a potential conflict of interest.
Publisher’s Note
All claims expressed in this article are solely those of the authors and do not necessarily represent those of their affiliated organizations, or those of the publisher, the editors, and the reviewers. Any product that may be evaluated in this article, or claim that may be made by its manufacturer, is not guaranteed or endorsed by the publisher.
Acknowledgments
The authors are grateful to the reviewers for their constructive comments for revisions.
Supplementary Material
The Supplementary Material for this article can be found online at: https://www.frontiersin.org/articles/10.3389/fgene.2021.764835/full#supplementary-material
References
Buer, C. S., Imin, N., and Djordjevic, M. A. (2010). Flavonoids: New Roles for Old Molecules. J. Integr. Plant Biol. 52 (1), 98–111. doi:10.1111/j.1744-7909.2010.00905.x
Butelli, E., Titta, L., Giorgio, M., Mock, H.-P., Matros, A., Peterek, S., et al. (2008). Enrichment of Tomato Fruit with Health-Promoting Anthocyanins by Expression of Select Transcription Factors. Nat. Biotechnol. 26 (11), 1301–1308. doi:10.1038/nbt.1506
Castillejo, C., Waurich, V., Wagner, H., Ramos, R., Oiza, N., Muñoz, P., et al. (2020). Allelic Variation of MYB10 Is the Major Force Controlling Natural Variation in Skin and Flesh Color in Strawberry (Fragaria spp.) Fruit. Plant Cell 32 (12), 3723–3749. doi:10.1105/tpc.20.00474
Chalhoub, B., Denoeud, F., Liu, S., Parkin, I. A., Tang, H., Wang, X., et al. (2014). Plant Genetics. Early Allopolyploid Evolution in the post-Neolithic Brassica Napus Oilseed Genome. Science 345 (6199), 950–953. doi:10.1126/science.1253435
Chen, C., Chen, H., Zhang, Y., Thomas, H. R., Frank, M. H., He, Y., et al. (2020). TBtools: An Integrative Toolkit Developed for Interactive Analyses of Big Biological Data. Mol. Plant 13 (8), 1194–1202. doi:10.1016/j.molp.2020.06.009
Cheng, F., Wu, J., Fang, L., and Wang, X. (2012). Syntenic Gene Analysis between Brassica Rapa and Other Brassicaceae Species. Front. Plant Sci. 3, 198. doi:10.3389/fpls.2012.00198
de Pascual-Teresa, S., Moreno, D. A., and García-Viguera, C. (2010). Flavanols and Anthocyanins in Cardiovascular Health: a Review of Current Evidence. Int. J. Mol. Sci. 11 (4), 1679–1703. doi:10.3390/ijms11041679
Fujii, K., and Ohmido, N. (2011). Stable Progeny Production of the Amphidiploid Resynthesized Brassica Napus Cv. Hanakkori, a Newly Bred Vegetable. Theor. Appl. Genet. 123 (8), 1433–1443. doi:10.1007/s00122-011-1678-5
Gonzalez, A., Zhao, M., Leavitt, J. M., and Lloyd, A. M. (2008). Regulation of the Anthocyanin Biosynthetic Pathway by the TTG1/bHLH/Myb Transcriptional Complex in Arabidopsis Seedlings. Plant J. 53 (5), 814–827. doi:10.1111/j.1365-313X.2007.03373.x
Goswami, G., Nath, U. K., Park, J.-I., Hossain, M. R., Biswas, M. K., Kim, H.-T., et al. (2018). Transcriptional Regulation of Anthocyanin Biosynthesis in a High-Anthocyanin Resynthesized Brassica Napus Cultivar. J. Biol. Res-thessaloniki 25, 19. doi:10.1186/s40709-018-0090-6
Guo, N., Cheng, F., Wu, J., Liu, B., Zheng, S., Liang, J., et al. (2014). Anthocyanin Biosynthetic Genes in Brassica Rapa. BMC Genomics 15, 426. doi:10.1186/1471-2164-15-426
Guo, N., Han, S., Zong, M., Wang, G., Zheng, S., and Liu, F. (2019). Identification and Differential Expression Analysis of Anthocyanin Biosynthetic Genes in Leaf Color Variants of Ornamental Kale. BMC genomics 20 (1), 564. doi:10.1186/s12864-019-5910-z
He, Q., Zhang, Z., and Zhang, L. (2016). Anthocyanin Accumulation, Antioxidant Ability and Stability, and a Transcriptional Analysis of Anthocyanin Biosynthesis in Purple Heading Chinese Cabbage (Brassica Rapa L. Ssp. Pekinensis). J. Agric. Food Chem. 64 (1), 132–145. doi:10.1021/acs.jafc.5b04674
Heng, S., Cheng, Q., Zhang, T., Liu, X., Huang, H., Yao, P., et al. (2020a). Fine-mapping of the BjPur Gene for Purple Leaf Color in Brassica Juncea. Theor. Appl. Genet. 133 (11), 2989–3000. doi:10.1007/s00122-020-03634-9
Heng, S., Wang, L., Yang, X., Huang, H., Chen, G., Cui, M., et al. (2020b). Genetic and Comparative Transcriptome Analysis Revealed DEGs Involved in the Purple Leaf Formation in Brassica Juncea. Front. Genet. 11, 322. doi:10.3389/fgene.2020.00322
Kim, J., Lee, W. J., Vu, T. T., Jeong, C. Y., Hong, S.-W., and Lee, H. (2017). High Accumulation of Anthocyanins via the Ectopic Expression of AtDFR Confers Significant Salt Stress Tolerance in Brassica Napus L. Plant Cel Rep 36 (8), 1215–1224. doi:10.1007/s00299-017-2147-7
Lepiniec, L., Debeaujon, I., Routaboul, J.-M., Baudry, A., Pourcel, L., Nesi, N., et al. (2006). Genetics and Biochemistry of Seed Flavonoids. Annu. Rev. Plant Biol. 57, 405–430. doi:10.1146/annurev.arplant.57.032905.105252
Li, H., Zhu, L., Yuan, G., Heng, S., Yi, B., Ma, C., et al. (2016). Fine Mapping and Candidate Gene Analysis of an Anthocyanin-Rich Gene, BnaA.PL1, Conferring Purple Leaves in Brassica Napus L. Mol. Genet. Genomics 291 (4), 1523–1534. doi:10.1007/s00438-016-1199-7
Liu, J., Osbourn, A., and Ma, P. (2015). MYB Transcription Factors as Regulators of Phenylpropanoid Metabolism in Plants. Mol. Plant 8 (5), 689–708. doi:10.1016/j.molp.2015.03.012
Liu, L. L., Huang, T., Ding, S. P., Wang, Y., and Yan, M. L. (2017). BANYULS Genes from Brassica Juncea and Brassica Nigra: Cloning, Evolution and Involvement in Seed Coat Colour. J. Agric. Sci. 155 (3), 421–430. doi:10.1017/S0021859616000435
Liu, S., Liu, Y., Yang, X., Tong, C., Edwards, D., Parkin, I. A. P., et al. (2014). The Brassica oleracea Genome Reveals the Asymmetrical Evolution of Polyploid Genomes. Nat. Commun. 5, 3930. doi:10.1038/ncomms4930
Liu, Y., Tikunov, Y., Schouten, R. E., Marcelis, L. F. M., Visser, R. G. F., and Bovy, A. (2018). Anthocyanin Biosynthesis and Degradation Mechanisms in Solanaceous Vegetables: A Review. Front. Chem. 6, 52. doi:10.3389/fchem.2018.00052
Mohd Saad, N. S., Severn-Ellis, A. A., Pradhan, A., Edwards, D., and Batley, J. (2021). Genomics Armed with Diversity Leads the Way in Brassica Improvement in a Changing Global Environment. Front. Genet. 12, 600789. doi:10.3389/fgene.2021.600789
Mushtaq, M. A., Pan, Q., Chen, D., Zhang, Q., Ge, X., and Li, Z. (2016). Comparative Leaves Transcriptome Analysis Emphasizing on Accumulation of Anthocyanins in Brassica: Molecular Regulation and Potential Interaction with Photosynthesis. Front. Plant Sci. 7, 311. doi:10.3389/fpls.2016.00311
Nguyen, N. H., Jeong, C. Y., Kang, G. H., Yoo, S. D., Hong, S. W., and Lee, H. (2015). MYBD Employed by HY 5 Increases Anthocyanin Accumulation via Repression of MYBL 2 in Arabidopsis. Plant J. 84 (6), 1192–1205. doi:10.1111/tpj.13077
Petroni, K., and Tonelli, C. (2011). Recent Advances on the Regulation of Anthocyanin Synthesis in Reproductive Organs. Plant Sci. 181 (3), 219–229. doi:10.1016/j.plantsci.2011.05.009
Rousseau-Gueutin, M., Belser, C., Da Silva, C., Richard, G., Istace, B., Cruaud, C., et al. (2020). Long-read Assembly of the Brassica Napus Reference Genome Darmor-Bzh. Gigascience 9 (12), giaa137. doi:10.1093/gigascience/giaa137
Song, H., Yi, H., Lee, M., Han, C.-T., Lee, J., Kim, H., et al. (2018). Purple Brassica oleracea Var. Capitata F. Rubra Is Due to the Loss of BoMYBL2-1 Expression. BMC Plant Biol. 18 (1), 82. doi:10.1186/s12870-018-1290-9
Song, J.-M., Guan, Z., Hu, J., Guo, C., Yang, Z., Wang, S., et al. (2020). Eight High-Quality Genomes Reveal Pan-Genome Architecture and Ecotype Differentiation of Brassica Napus. Nat. Plants 6 (1), 34–45. doi:10.1038/s41477-019-0577-7
Sun, Y., Li, H., and Huang, J.-R. (2012). Arabidopsis TT19 Functions as a Carrier to Transport Anthocyanin from the Cytosol to Tonoplasts. Mol. Plant 5 (2), 387–400. doi:10.1093/mp/ssr110
Ton, L. B., Neik, T. X., and Batley, J. (2020). The Use of Genetic and Gene Technologies in Shaping Modern Rapeseed Cultivars (Brassica Napus L.). Genes 11 (10), 1161. doi:10.3390/genes11101161
Wang, X., Wang, H., Wang, H., Wang, J., Sun, R., Wu, J., et al. (2011). The Genome of the Mesopolyploid Crop Species Brassica Rapa. Nat. Genet. 43 (10), 1035–1039. doi:10.1038/ng.919
Wang, Y., Wang, Y., Song, Z., and Zhang, H. (2016). Repression of MYBL2 by Both microRNA858a and HY5 Leads to the Activation of Anthocyanin Biosynthetic Pathway in Arabidopsis. Mol. Plant 9 (10), 1395–1405. doi:10.1016/j.molp.2016.07.003
Xie, L., Li, F., Zhang, S., Zhang, H., Qian, W., Li, P., et al. (2016). Mining for Candidate Genes in an Introgression Line by Using RNA Sequencing: The Anthocyanin Overaccumulation Phenotype in Brassica. Front. Plant Sci. 7, 1245. doi:10.3389/fpls.2016.01245
Xu, W., Dubos, C., and Lepiniec, L. (2015). Transcriptional Control of Flavonoid Biosynthesis by MYB-bHLH-WDR Complexes. Trends Plant Science 20 (3), 176–185. doi:10.1016/j.tplants.2014.12.001
Xu, W., Grain, D., Bobet, S., Le Gourrierec, J., Thévenin, J., Kelemen, Z., et al. (2014). Complexity and Robustness of the Flavonoid Transcriptional Regulatory Network Revealed by Comprehensive Analyses of MYB -b HLH - WDR Complexes and Their Targets in A Rabidopsis Seed. New Phytol. 202 (1), 132–144. doi:10.1111/nph.12620
Xu, W., Grain, D., Gourrierec, J., Harscoët, E., Berger, A., Jauvion, V., et al. (2013). Regulation of Flavonoid Biosynthesis Involves an Unexpected Complex Transcriptional Regulation of TT 8 Expression, in A Rabidopsis. New Phytol. 198 (1), 59–70. doi:10.1111/nph.12142
Yan, C., An, G., Zhu, T., Zhang, W., Zhang, L., Peng, L., et al. (2019). Independent Activation of the BoMYB2 Gene Leading to Purple Traits in Brassica oleracea. Theor. Appl. Genet. 132 (4), 895–906. doi:10.1007/s00122-018-3245-9
Yan, M., Ding, S., Liu, L., Yin, X., and Shu, J. (2014). Cloning and Expression Analysis of an Anthocyanidin Synthase Gene Homologue from Brassica Carinata. J. Genet. 93 (2), 513–516. doi:10.1007/s12041-014-0387-7
Yan, M., Liu, X., Guan, C., Chen, X., and Liu, Z. (2011). Cloning and Expression Analysis of an Anthocyanidin Synthase Gene Homolog from Brassica Juncea. Mol. Breed. 28, 313–322. doi:10.1007/s11032-010-9483-4
Zhang, D.-w., Liu, L.-l., Zhou, D.-g., Liu, X.-j., Liu, Z.-s., and Yan, M.-l. (2020). Genome-wide Identification and Expression Analysis of Anthocyanin Biosynthetic Genes in Brassica Juncea. J. Integr. Agric. 19, 1250–1260. doi:10.1016/S2095-3119(20)63172-0
Zhang, D., Pan, Q., Tan, C., Liu, L., Ge, X., Li, Z., et al. (2018). Homoeolog Expression Is Modulated Differently by Different Subgenomes in Brassica Napus Hybrids and Allotetraploids. Plant Mol. Biol. Rep. 36, 387–398. doi:10.1007/s11105-018-1087-x
Zhang, L., Su, W., Tao, R., Zhang, W., Chen, J., Wu, P., et al. (2017). RNA Sequencing Provides Insights into the Evolution of Lettuce and the Regulation of Flavonoid Biosynthesis. Nat. Commun. 8 (1), 2264. doi:10.1038/s41467-017-02445-9
Zhang, Y., Chen, G., Dong, T., Pan, Y., Zhao, Z., Tian, S., et al. (2014). Anthocyanin Accumulation and Transcriptional Regulation of Anthocyanin Biosynthesis in Purple Bok Choy (Brassica Rapa Var. Chinensis). J. Agric. Food Chem. 62 (51), 12366–12376. doi:10.1021/jf503453e
Keywords: anthocyanin biosynthetic genes, B. napus, comparative genomic analysis, transcriptome, dynamic accumulation
Citation: He D, Zhang D, Li T, Liu L, Zhou D, Kang L, Wu J, Liu Z and Yan M (2021) Whole-Genome Identification and Comparative Expression Analysis of Anthocyanin Biosynthetic Genes in Brassica napus. Front. Genet. 12:764835. doi: 10.3389/fgene.2021.764835
Received: 26 August 2021; Accepted: 11 October 2021;
Published: 18 November 2021.
Edited by:
Zefeng Yang, Yangzhou University, ChinaCopyright © 2021 He, Zhang, Li, Liu, Zhou, Kang, Wu, Liu and Yan. This is an open-access article distributed under the terms of the Creative Commons Attribution License (CC BY). The use, distribution or reproduction in other forums is permitted, provided the original author(s) and the copyright owner(s) are credited and that the original publication in this journal is cited, in accordance with accepted academic practice. No use, distribution or reproduction is permitted which does not comply with these terms.
*Correspondence: Dawei Zhang, emhhbmdkYXdlaS5obnVzdEBmb3htYWlsLmNvbQ==; Mingli Yan, eW1samFja0AxMjYuY29t