- 1Faculty of Health Sciences, Centre of Reproduction, Development and Aging, University of Macau, Taipa, China
- 2Shenzhen Key Lab of Marine Genomics, Guangdong Provincial Key Lab of Molecular Breeding in Marine Economic Animals, Beijing Genomics Institute, BGI Academy of Marine Sciences, BGI Marine, Shenzhen, China
- 3College of Life Sciences, University of Chinese Academy of Sciences, Beijing, China
Melanin is the most prevalent pigment in animals. Its synthesis involves a series of functional genes. Particularly, teleosts have more copies of these genes related to the melanin synthesis than tetrapods. Despite the increasing number of available vertebrate genomes, a few systematically genomic studies were reported to identify and compare these core genes for the melanin synthesis. Here, we performed a comparative genomic analysis on several core genes, including tyrosinase genes (tyr, tyrp1, and tyrp2), premelanosome protein (pmel), microphthalmia-associated transcription factor (mitf), and solute carrier family 24 member 5 (slc24a5), based on 90 representative vertebrate genomes. Gene number and mutation identification suggest that loss-of-function mutations in these core genes may interact to generate an albinism phenotype. We found nonsense mutations in tyrp1a and pmelb of an albino golden-line barbel fish, in pmelb of an albino deep-sea snailfish (Pseudoliparis swirei), in slc24a5 of cave-restricted Mexican tetra (Astyanax mexicanus, cavefish population), and in mitf of a transparent icefish (Protosalanx hyalocranius). Convergent evolution may explain this phenomenon since nonsense mutations in these core genes for melanin synthesis have been identified across diverse albino fishes. These newly identified nonsense mutations and gene loss will provide molecular guidance for ornamental fish breeding, further enhancing our in-depth understanding of human skin coloration.
Introduction
Pigment patterns and coloration of skin, feathers, hair and scales are among the most variable phenotypes in various vertebrates (Protas and Patel, 2008). Diverse coloration phenotypes present some substantial functions in mate selection, crypsis, aposematism of predators, and species recognition (Protas and Patel, 2008). Melanin is the most prevalent pigment in animals with the primary function of shielding against UV irradiation from sunlight. It is synthesized in pigment cells or chromatophores that are derived from neural crests (Singh and Nüsslein-Volhard, 2015). Mammals and birds exhibit only one category of pigment cells, also named melanocytes including black and brown types. Reptiles and amphibians possess xanthophores and iridophores. In fishes, there are seven different types of pigment cells, including melanophores, xanthophores, iridophores, erythrophores, leucophores, cyanophores and erythro-iridophores (Nordlund, 1992; Singh and Nüsslein-Volhard, 2015).
The black pigment, also named melanin, is generated from tyrosine in the melanosome (del Marmol and Beermann, 1996). Two types of melanin are present in mammals and birds, including black eumelanin and lighter pheomelanin. In various fishes, however, only eumelanin is found (Burton, 2011). The detailed melanin synthesis pathway is summarized in Figure 1. Disruption of the melanogenesis process causes decreased pigmentation, which may lead to complete absence of melanin (Braasch et al., 2007).
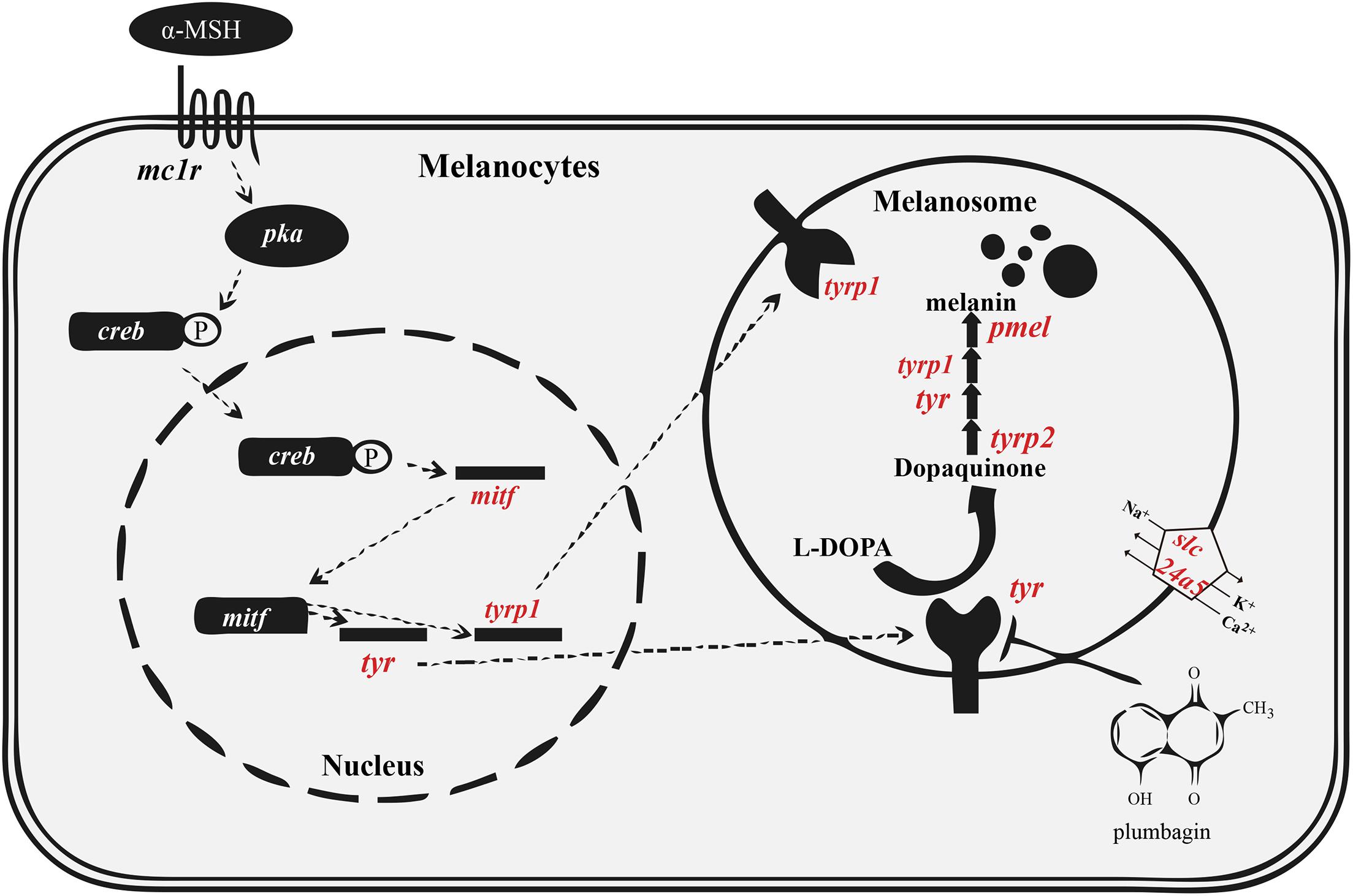
Figure 1. Melanin synthesis pathway in vertebrates. The tyrosinase family (tyr, tyrp1 and tyrp2) and pmel are essential for melanin synthesis. The melanosomal transporter (slc24a5) and regulatory factor (mitf) are also important for proper melanin synthesis. Red indicates the genes that we analyzed in detail. This figure was modified from a previous report (Oh et al., 2017). Abbreviations: α-MSH, alpha-melanocyte stimulating hormone; creb, cAMP response element binding protein; mc1r, melanocortin receptor; pka, protein kinase A. See other abbreviations in corresponding text.
The genetic basis of pigment cell development and differentiation is largely conserved between tetrapods and fishes. It is well documented that a big proportion of genes in the melanin synthesis pathway have been duplicated and differentially retained in teleosts (Braasch et al., 2007, 2009a), possibly due to the third round of whole genome duplication (also known as the teleost-specific whole genome duplication, TWGD) approximately 250–350 million years ago (Mya) (Jaillon et al., 2004). Therefore, teleosts usually possess more melanin synthesis genes than tetrapods. Melanin biosynthesis in vertebrates depends on three members of the tyrosinase family, including tyrosinase (tyr), and tyrosinase-related protein 1 and 2 (tyrp1 and tyrp2). The tyrp1 gene might play an additional role in survival and proliferation of melanocytes. The expression of pmel (premelanosome protein) and tyrp1 genes is often regulated by mitf (microphthalmia-associated transcription factor a; see Figure 1; Braasch et al., 2009a; Bian et al., 2020).
The albinism phenotype (loss of melanin) is a fascinating trait of ornamental fishes, such as glass catfish, white cichlid fish and albino northern snakehead fish. The casper zebrafish (named after its ghost like appearance; White et al., 2008) and casper stickleback (Hart and Miller, 2017), displaying reduced pigmentation of melanosomes, can also be useful models for tumor engraftment and in vivo stem cell analyses with high sensitivity and resolution. Therefore, it is important to understand the detailed molecular mechanism and core genes involved in melanin biosynthesis, which can improve the efficiency of color breeding in ornamental fishes. This can also help to generate a wide variety of species to act as excellent research models. With an increasing number of available vertebrate genome sequences, we can analyze and identify core genes for melanin synthesis in various vertebrates. In this study, we analyzed genes involved in the melanin synthesis pathway in 90 representative vertebrates, including mammals, birds, reptiles, amphibians, Actinopterygii, and Chondrichthyes. For our better understanding of the melanin synthesis pathway in vertebrates, we explored several core genes in this pathway, such as tyr, tyrp1, tyrp2, pmel, mitf, and solute carrier family 24 member 5 (slc24a5), in various vertebrates.
Materials and Methods
Genome Data Collection and Gene Identification
In total, 90 representative vertebrate genome assemblies were selected. They were downloaded from the National Center for Biotechnology Information (NCBI; Supplementary Table 1). The scaffold N50 data of these genome assemblies was also shown in Supplementary Table 1. Each genome sequence was prepared for construction in a standard BLAST database for subsequent BLAST analyses. The published protein sequences of tyra, tyrb, tyrp1, tyrp2, pmela, pmelb, mitfa, mitfb, and slc24a5 from reference genomes (Japanese medaka Oryzias latipes, human Homo sapiens, chicken Gallus gallus, African clawed frog Xenopus laevis, and green Anoli lizard Anolis carolinensiswere) were downloaded from the public Ensembl database (Supplementary Table 2).
In detail, the protein sequences of medaka, human, chicken, frog, and green lizard were used for aligning the assemblies of Actinopterygii, mammals, birds, amphibians, and reptile species by using tBLASTn (version 2.2.28, NCBI, Bethesda, MD, United States) (Mount, 2007) with an E-value of 10–5. These alignments were further filtered and processed by using a Perl script to generate best-hit genomic regions containing putative target genes of each genome assembly with over 50% identity and aligned ratio. GeneWise v2.2.0 (Birney et al., 2004) was employed to predict target gene structures in the best-hit alignments from the 90 representative vertebrate genomes. Most importantly, if this pipeline identified a gene loss in any examined species, we then manually checked the tBLASTn alignments in this species to make sure that the data were indeed true. The gene was examined again in the final gene list from the genome annotation, if it has completed gene structure but its alignment identity and aligned ratio were between 45 and 50%. This lower identity and align ratio could be caused by rapid evolutionary rate of this gene in some species. Meanwhile, we also checked for synteny in genes form up and downstream of these genes as an additional indicator of orthology. Detailed copy numbers of above indicated genes in 90 vertebrate genomes and 9 representative genomes are provided in Tables 1, 2 and Supplementary Tables 3, 4.
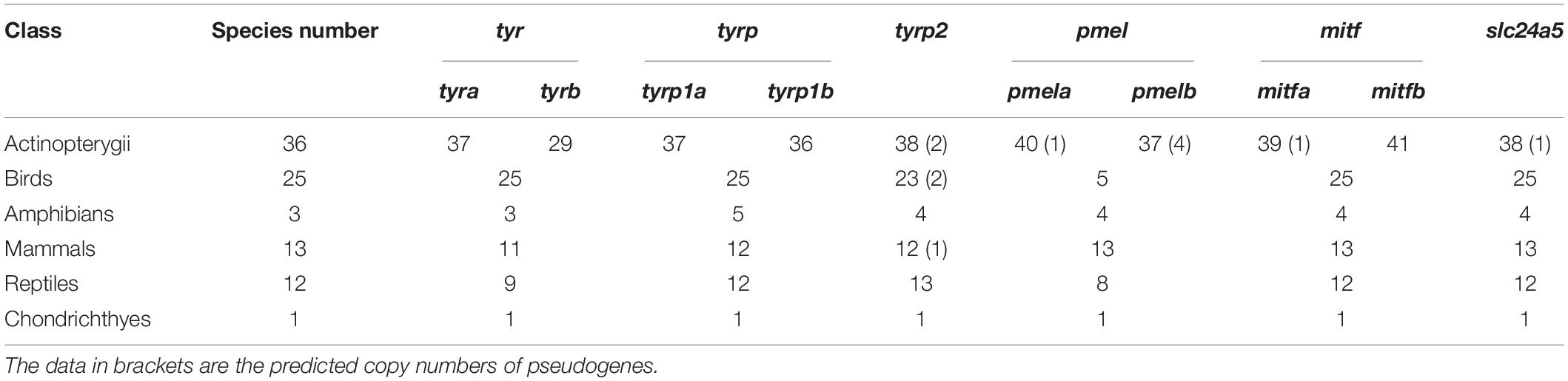
Table 1. Copy numbers of representative genes for melanin synthesis in 90 representative vertebrate genomes.
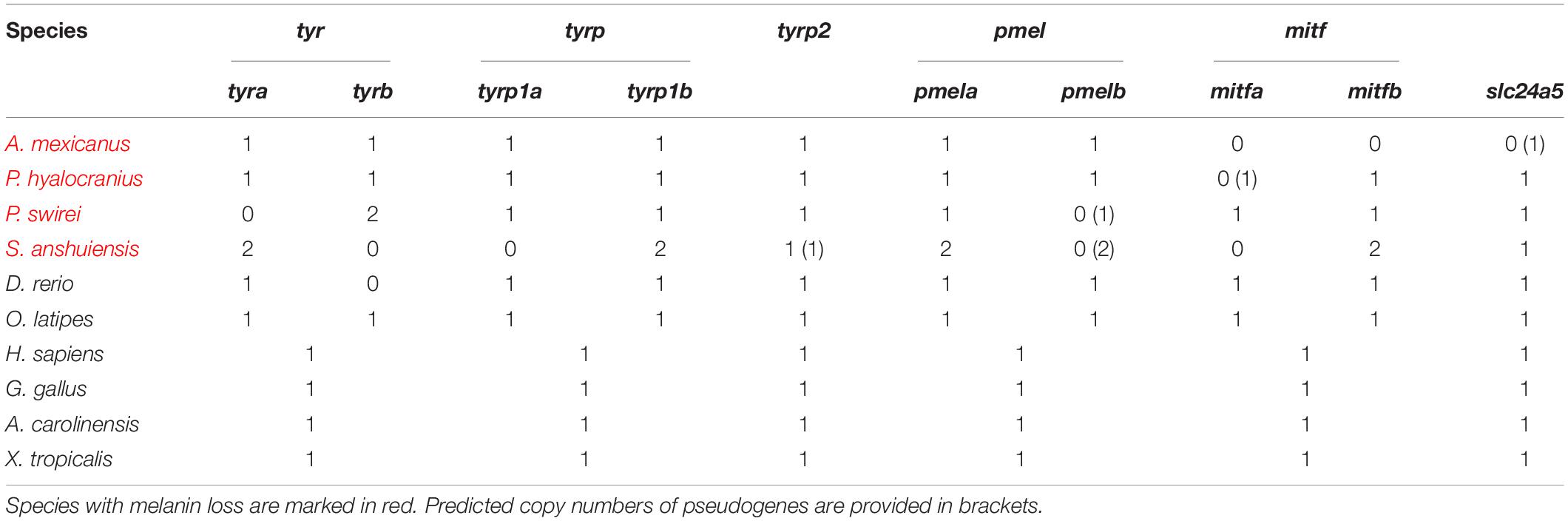
Table 2. Copy numbers of representative genes for melanin synthesis in 9 representative vertebrate species.
Phylogenetic Analysis
Phylogenetic analysis was performed on the protein sequences. Multiple sequence alignments of amino acid sequences were conducted across various species using the Muscle software (Kumar et al., 2016). The trimAl software (Capella-Gutierrez et al., 2009) with default parameters was employed for alignment trimming. Phylogenetic trees were constructed by PhyML (version 3.1) (Guindon et al., 2010) with the amino acid substitution model of JTT + I + G and the maximum likelihood (ML) method. The protein sequences of ghost shark (Callorhynchus milii) were used as the outgroups. A total of 1,000 bootstrap replicates were applied for evaluation of their branch supports. The trees were displayed by FigTree.1
Pseudogene Identification and Protein Sequence Alignment
We focused on identification of the core genes for melanin synthesis in several representative species with a loss-of-melanin phenotype. These vertebrates included Astyanax mexicanus, Sinocyclocheilus anshuiensis, Protosalanx hyalocranius, Pseudoliparis swirei, and Danio rerio (nacre/casper mutants). The corresponding nucleotide sequences of three-spined stickleback (Gasterosteus aculeatus), D. rerio (Tu strain), Oryzias latipes, Anolis carolinensis, Homo sapiens, and Gallus gallus were used as references for comparison. Multiple sequence alignments of the above-mentioned species were produced with the Muscle software in MEGA package (Kumar et al., 2016). Codon-based alignments were utilized to examine irregular shifts in the open reading frame for identification of possible pseudogenes, which were characterized by codon frameshifts or premature stop codon(s).
Results
We screened 90 vertebrate genomes for tyr, tyrp1, tyrp2, pmel, mitf, and slc24a5 genes, representing 36 Actinopterygii species, 13 mammals, 25 birds, 12 reptiles, three amphibians, and one chondrichthyan fish. Copy numbers of each gene are summarized in Table 1.
Tyrosinase Gene Family
Melanin synthesis in vertebrates involves several important tyrosinase family members, including tyr, tyrp1, and tyrp2 (Oetting and Setaluri, 2007). The tyr and tyrp1 genes have been doubled and subsequently preserved in the teleost ancestor after the TWGD. The protein product of tyr catalyzes the first two rate-limiting steps of melanin synthesis by converting tyrosine to DOPA, and then catalyzes dopaquinone to melanin (Oetting and Setaluri, 2007). Mutations in the tyr gene of the sandy zebrafish strain caused melanin loss (Kelsh et al., 1996). Similarly, mutations in tyr lead to oculocutaneous albinism type 1 (OCA1) in humans (Dessinioti et al., 2009). The protein product of tyrp2 catalyzes dopachrome to DHI-2-carboxylic acid (DHICA), while the tyrp1 could participate not only in stabilizing the tyr in melanosome membranes (Kobayashi and Hearing, 2007; Krauss et al., 2014), but also in the formation of indole-5,6-quinone carboxylic acid from DHICA. A mutation in the coding region of tyrp1a caused melanophore death in zebrafish (Krauss et al., 2014). The double knock-down experiment of tyrp1a and tyrp1b in zebrafish led to hypo-pigmented melanophores and brown pigment (Braasch et al., 2009b). The oculocutaneous albinism type 3 (OCA3) of human, also known as Rufous albinism, is caused by mutations (Arg93Cys) in tyrp1 (Dessinioti et al., 2009). The mutations of tyrp1 gene also generally caused chocolate or brown coat color in many mammals and birds, like mice (Zdarsky et al., 1990), dog (Hrckova Turnova et al., 2017), chicken (Li et al., 2019), and Japanese quail (Nadeau et al., 2007).
We found that most of the examined teleost fishes possessed two copies of tyr (tyra and tyrb) and tyrp1 (tyrp1a and tyrp1b), but only one copy of tyrp2. However, in all examined actinopterygian genomes, only the cave-restricted S. anshuiensis lost the tyrp1a gene; however, nine fish species have lost the tyrb gene (see more details in Supplementary Table 3). Both pufferfishes (fugu and Tetraodon) have lost the tyrp1b gene, which is consistent with a previous study (Braasch et al., 2007). The three Chinese golden-line barbel fishes (Sinocyclocheilus anshuiensis, S. grahami and S. rhinocerous) have two tyrp1b copies, which are consistent with their shared lineage-specific whole genome duplication event. Two forms of tyrp2 were retained in the three golden-line barbel fishes too; however, one tyrp2 was a pseudogene with a premature stop codon in S. anshuiensis and S. rhinocerous. On the other hand, the majority of tetrapods have only one copy of tyr, tyrp1, and tyrp2. According to our reconstructed phylogenetic trees for the tyr, tyrp1, and tyrp2 genes (Supplementary Figures 1–3), tyr genes could be clearly divided into five main groups to represent actinopterygians, amphibians, mammals, reptiles, and birds. Similar to a previous study (Braasch et al., 2007), the tyr and tyrp1 phylogenetic trees (Supplementary Figures 1, 2) clearly revealed that tyr and tyrp1 were doubled in teleosts, consistent with the TWGD event.
mitf
The mitf gene encodes a vital transcription factor that up-regulates tyr, tyrp1, tyrp2, and pmel expression for melanin synthesis (Hsiao and Fisher, 2014). Most of tetrapods possess only one mitf gene in their genomes (Steingrimsson et al., 2004). In mice, a representative tetrapod model, mitf was determined to be related to development of coat color, eye, osteoclasts, and mast cells (Hodgkinson et al., 1993; Widlund and Fisher, 2003; Steingrimsson et al., 2004).
However, duplicated copies of mitf genes (named mitfa and mitfb) are present in the majority of teleost genomes (Steingrimsson et al., 2004). These mitf genes have undergone subfunctionalization after genome duplication at least 100 Mya (Altschmied et al., 2002). It was reported that the mitfa gene was involved in melanin synthesis, and the mitfb gene coexpressed with mitfa in the retinal pigment epithelium at an appropriate time to compensate for loss of mitfa function in the nacre mutant (Lister et al., 2001). A premature stop codon, identified in the mitfa exon of nacre zebrafish mutants (see more details in Figure 2), caused the loss of melanin pigments in this mutant trunk (Lister et al., 1999; White et al., 2008).
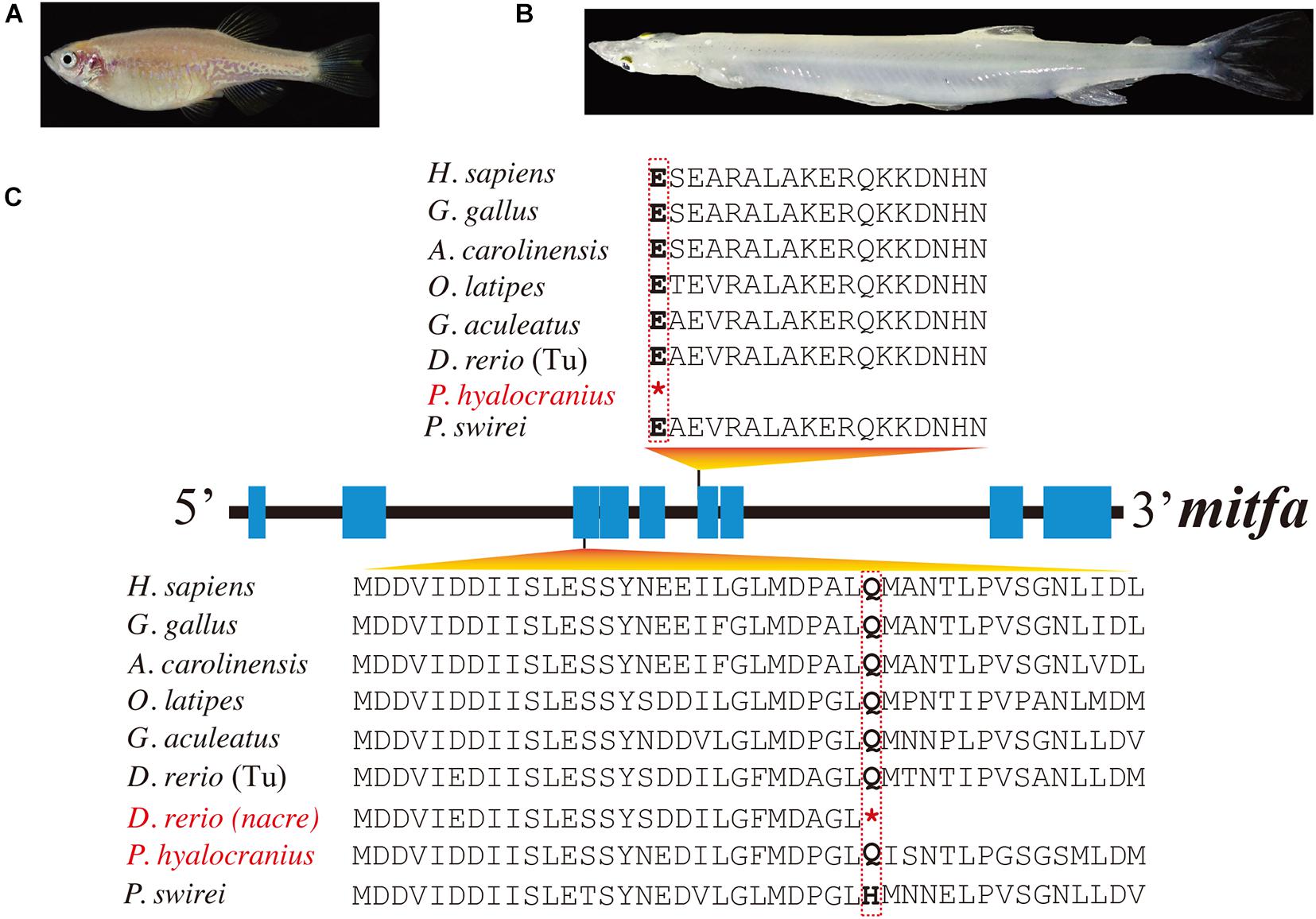
Figure 2. Multiple sequence alignments of mitfa of nine representative species. (A,B) Photos of transparent nacre zebrafish and Chinese clearhead icefish (P. hyalocranius). (C) Nonsense mutations in the mitfa exons of both species. Species with melanin loss are marked in red. *Represents the premature stop codon.
In this study, we identified 135 mitf genes in 90 representative vertebrate species (see detailed copy numbers in Supplementary Table 3). Among these examined species, the mitfa gene was lost in the cavefish A. mexicanus, while it harbored a premature stop codon in P. hyalocranius (Figure 2). The phylogenetic tree of mitfa and mitfb across 90 vertebrate species (Supplementary Figure 4) clearly placed teleosts into one main branch that was split from the common ancestor of teleosts and tetrapods. Subsequently, the teleost branch was divided into mitfa and mitfb clades, independently.
pmel
The pmel gene (also known as silver locus) encodes a type I integral membrane protein that can catalyze eumelanin production (see Figure 1) from indole-5,6-quinone carboxylic acid during melanin synthesis (Chakraborty et al., 1996). Duplication of this gene has been reported in zebrafish (Schonthaler et al., 2005). pmela is expressed in melanophores and retinal pigment epithelium, but pmelb is expressed exclusively in retinal pigment epithelium (Schonthaler et al., 2005), which is similar to the previously reported subfunctionalization and distributions of the mitfa gene and mitfb gene in zebrafish (Lister et al., 2001). In mammals, pmel transcription is regulated by mitf (Du et al., 2003). Several missense mutations in horse pmel lead to a phenotype with a characteristic mixture of white and gray hairs (Brunberg et al., 2006). Similarly, a nonsense mutation in the pmel gene of Japanese quail caused a completely yellowish plumage phenotype (Ishishita et al., 2018). Furthermore, a 9-bp insertion in the exon 10 of chicken pmel led to a Dominant white phenotype (Kerje et al., 2004).
In our present study, we found that most teleosts have two pmel genes (Supplementary Table 3). Related phylogenetic data confirmed the duplication of pmel in teleosts after their divergence from tetrapods (Supplementary Figure 5). In contrast, pmel was lost in the majority of birds. Intriguingly, we observed that the pmel genes of P. swirei and S. anshuiensis commonly harbored premature stop codon mutations. More particularly, the two pmelb genes were truncated by nonsense mutations in S. anshuiensis (see more details in Figure 3).
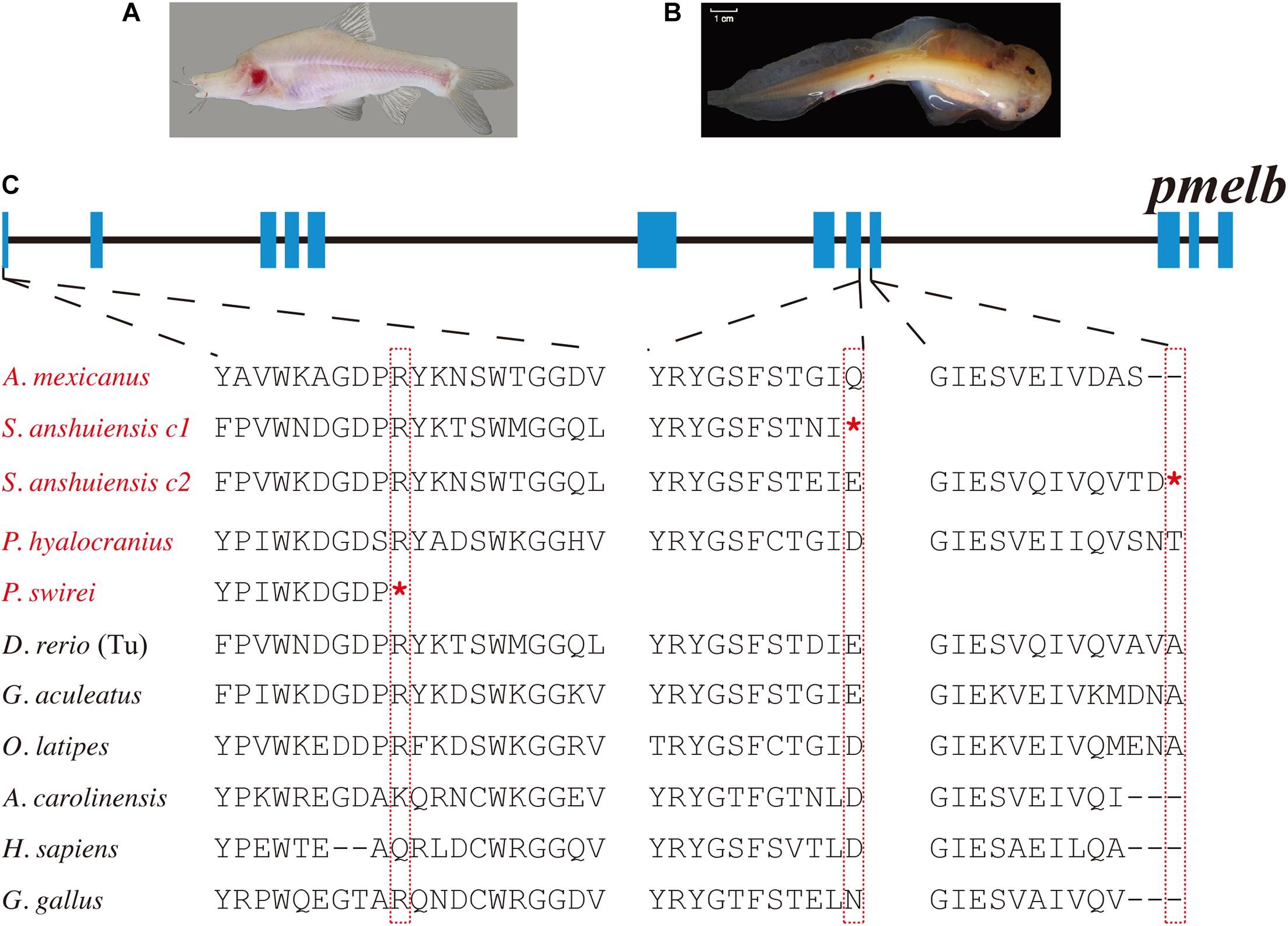
Figure 3. Multiple sequence alignments of pmelb in ten representative species. (A,B) Photos of a cave-restricted golden-line barbel fish (S. anshuiensis) and a deep-sea snailfish (P. swirei). This deep-sea snailfish figure was adopted from Wang et al. (2019) with permission. (C) Nonsense mutations in the pmelb exons of S. anshuiensis and P. swirei. Species with melanin loss were marked in red. c1 and c2 represent copy 1 and copy 2. *Represents the premature stop codon.
slc24a5
slc24a5, encoding a transporter protein in the melanosome membrane, is essential for melanin synthesis (Figure 1). It has a pigmentation related function that was firstly identified in teleosts and later in mammals. Loss-of-function mutations in this gene led to reduced melanin concentration in zebrafish (Lamason et al., 2005). However, a non-synonymous mutation in the exon 2 of slc24a5 in a horse generated bright orange-colored eyes (Mack et al., 2017). A recent study also reported that a non-synonymous mutation in slc24a5 significantly correlated to skin color in African human populations (Crawford et al., 2017).
In the present study, we identified a total of 94 slc24a5 genes (Supplementary Tables 3, 4). It has been clearly shown that most of the examined teleosts only have one copy of the slc24a5 gene, suggesting that its duplicated paralogous gene was lost in the teleost ancestor after the TWGD. The A. mexicanus is a unique species that harbors a premature stop codon mutation in its slc24a5 coding regions (Figure 4 and Table 1). Interestingly, gene copy number and phylogenetic analyses demonstrated the unique presence of three copies of the slc24a5 gene in Nile tilapia (Oreochromis niloticus, Supplementary Figure 6).
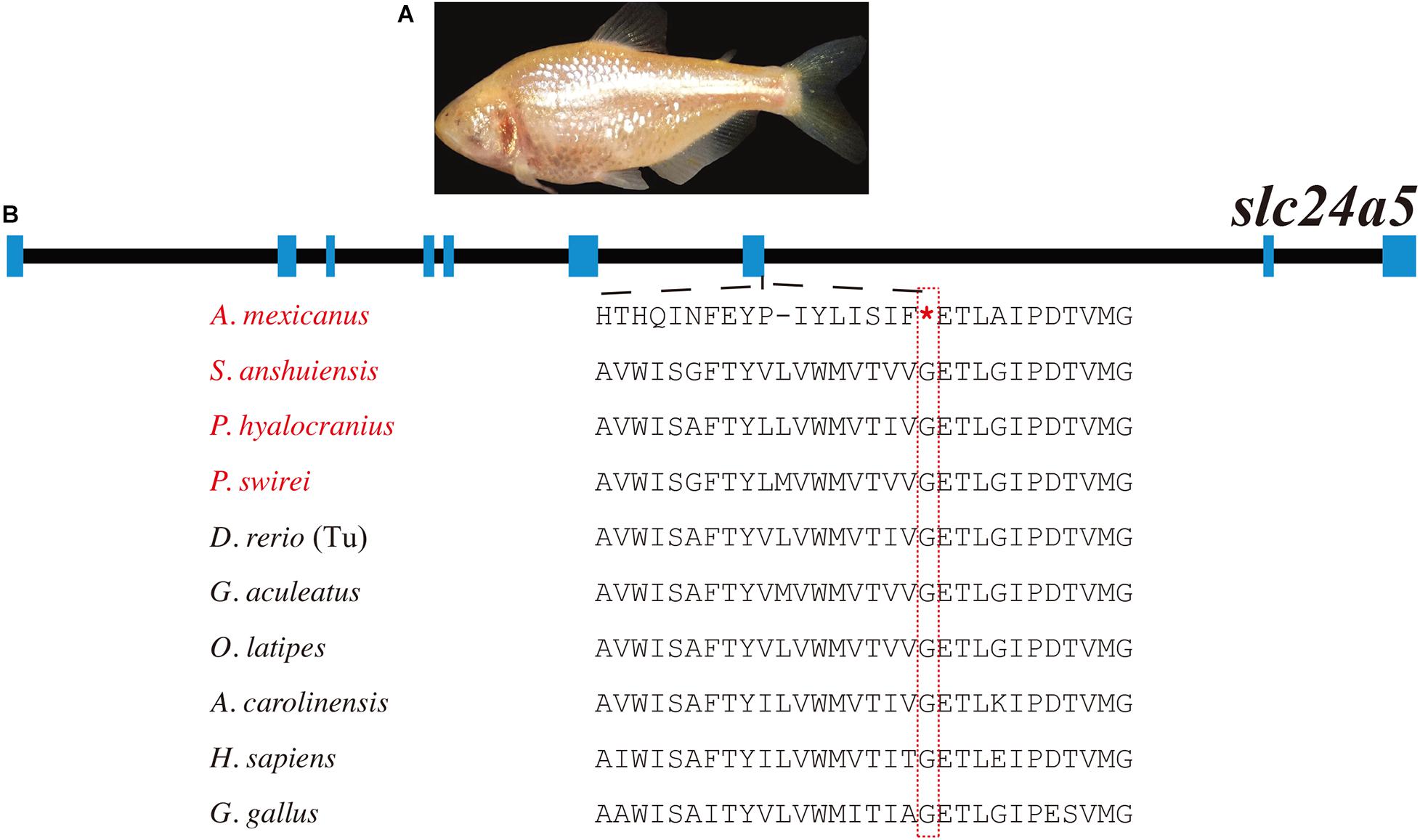
Figure 4. Multiple sequence alignments of slc24a5 in ten representative species. (A) Photo of A. mexicanus (cave population), which was downloaded from Wikipedia Commons (NIH Image Gallery, Bethesda, MD, United States). (B) A nonsense mutation in the slc24a5 exon of A. mexicanus. Species with melanin loss were marked in red. *Represents the premature stop codon.
Discussion
Possible Molecular Clues Regarding the Melanin Loss Phenotype
Animals that are not exposed to light, such as cavefishes, are often colorless or transparent. The cave ecosystem is associated with several traits that are decreased or degenerated over time (i.e., “regressive” traits) (Protas et al., 2006). Notably, the reduced skin pigmentation in cavefishes is an important adaptation that has independently arisen in diverse species, such as S. anshuiensis (Yang et al., 2016) and A. mexicanus (Gross, 2012).
We identified premature stop variations and gene loss that are potentially leading to melanin loss in fishes with the albinism phenotype, including Mexican tetra (McGaugh et al., 2014), zebrafish (Danio rerio, nacre/casper mutants) (Howe et al., 2013; Bian et al., 2020), Chinese clearhead icefish (Liu et al., 2017), deep-sea Mariana snailfish (Pseudoliparis swirei), and cave-restricted golden-line barbel fish (Sinocyclocheilus anshuiensis; Yang et al., 2016). These premature stop variants and gene loss could provide novel molecular interpretations for melanin loss and albinism in various vertebrates.
Three high-quality genome assemblies of Sinocyclocheilus fishes (Cypriniformes: Cyprinidae), including S. grahami, S. rhinocerous, and S. anshuiensis have been reported before by us (Yang et al., 2016). S. grahami is a surface-dwelling species, S. rhinocerous is a semi-cave-dwelling species, while S. anshuiensis is a cave-restricted counterpart with an albinism phenotype (Figure 3A). These genome data of the three tetraploid fishes were useful for comparative identification of genetic evidence for melanin loss. By comparing these genome assemblies, we found that the tyrp1a gene has been lost in the cavefish S. anshuiensis. It has been well confirmed that a mis-sense mutation in the tyrp1a leads to melanophore death (Krauss et al., 2014). This loss-of-function tryp1a could be one potential cue for the melanin loss phenotype in S. anshuiensis.
Moreover, both pmelb genes in S. anshuiensis have a premature stop codon; however, in the other two Sinocyclocheilus fish species, pmelb genes are commonly normal. In the former case, this may result in functional loss of these genes (to be potential pseudogenes). In fact, many genes for regulating the development of some features may accumulate mutations, ultimately resulting in the loss of functions or even the disappearance of specific traits. The loss of these melanin synthesis genes in S. anshuiensis could be important for the white-skin phenotype [15] in this species. A similar nonsense mutation in the pmelb coding region is visible in a deep-sea snailfish, P. swirei, with a similar melanin loss phenotype (Figure 3). This could be a good example of convergent evolution for mutations in the pmelb genes of both white-skinned species that exhibit a melanin pigment loss phenotype.
A. mexicanus (cave population) is a perfect diploid model for studying the regressive genetics in cavefishes. A previous study has reported that the albino cave population of A. mexicanus harbored deletions in its oca2 gene (Protas et al., 2006) when compared with its surface counterparts. However, roles of these mutations cannot be critically validated by the gene knockout experiments; in fact, the experimental fish will lose the melanin, when each gene encoding the rate-limiting factors like mitfa (Koludrovic and Davidson, 2013), slc24a5 (Lamason et al., 2005), oca2 (Protas et al., 2006; Klaassen et al., 2018), mc1r (Gross et al., 2009), tyr (Kobayashi and Hearing, 2007) for melanin synthesis was knocked out or knocked down. Similarly, the gene gain or loss cannot be correctly identified by only using the QTL (quantitative trait locus) method (Protas et al., 2006) in diverse populations of A. mexicanus.
By comparing genes across fish species, we observed that the A. mexicanus cave population has lost the mitfa gene and harbors a nonsense mutation in slc24a5. We therefore speculate that the loss of mitfa could be a potential reason for melanin loss in this species and this gene loss may be resulted from several changes. The first step involved the accumulation of mutations in one gene, thereby resulting in missense or nonsense mutations. Since this would have caused a loss of function for this gene, and even the gene eventually disappeared from the genome. Therefore, the loss of mitfa gene could have appeared earlier than the nonsense mutation in slc24a5. A possible hypothesis could be considered as follows: the loss of mitfa led to a defect in the melanin synthesis pathway, resulting in the inactivation and gradual accumulation of neutral mutations in the up- and downstream genes of this pathway.
On the other hand, in addition to the black pigmentation defect, the A. mexicanus (cave population) exhibits remarkable yellow or golden skin (Figure 4A), which is similar to the reported golden zebrafish mutant with a mutation in slc24a5, thereby leading to more lightly pigmented and “golden” fish (Lamason et al., 2005). This similar phenotype provides additional genetic evidence to support the theory that the mutation of slc24a5 could be possibly involved in this phenotype of A. mexicanus (cave population).
Another fish with an albinism phenotype, P. hyalocranius, has been reported in our previous paper (Liu et al., 2017). We also found a nonsense mutation in the sixth exon of mitfa (Figure 2). It has been well documented that the mitfa gene plays a core role in neural crest cell fate specification and melanocyte development (Levy et al., 2006; Wan et al., 2011; Koludrovic and Davidson, 2013). The nacre/casper zebrafish strain with a transparent phenotype also harbored a nonsense mutation in its mitfa gene (Lister et al., 1999; White et al., 2008). Therefore, the loss-of-function mutation of mitfa in P. hyalocranius could be the primary molecular mechanism for its melanin loss. Interestingly, the similar mutations in the mitfa genes from both P. hyalocranius and nacre/casper zebrafish were determined, and both fish species present with a similar phenotype of melanin loss in trunk.
Conclusion
Large numbers of sequenced vertebrate genomes have provided us a great opportunity to perform comparative genomics studies on some interesting genes. We collected 90 representative vertebrate genome assemblies with high quality to analyze detailed copy numbers and gene structures of core genes for melanin synthesis including tyr, pmel, mitf, and slc24a5. Phylogenetic analysis and genomic alignments were also performed in this study. We identified some novel genetic evidences that loss or nonsense mutations of these core melanin synthesis genes may contribute to melanin loss in these white-skinned fishes. These genetic resources will help to improve the practical breeding of ornamental fishes and create novel transparent models for theoretical researches. Our interesting findings in this study are also instructive for in-depth investigations of human skin coloration.
Data Availability Statement
The original contributions presented in the study are included in the article/Supplementary Material, further inquiries can be directed to the corresponding authors.
Ethics Statement
All animal experiments were performed in accordance with the guidelines of the Animal Ethics Committee and were approved by the Institutional Review Board on Bioethics and Biosafety of BGI.
Author Contributions
QS and WG conceived the project. CB, RL, and ZW performed the data analysis and figure preparation. CB prepared the manuscript. QS and WG revised the manuscript. All authors contributed to the article and approved the submitted version.
Funding
This work was financially supported by the University of Macau (MYRG2016-00072-FHS, MYRG2017-00157-FHS, and CPG2014-00014-FHS) and the Macau Fund for Development of Science and Technology (FDCT089/2014/A2 and FDCT173/2017/A3) to WG, and Shenzhen Grant Plan for Demonstration City Project for Marine Economic Development in Shenzhen (No. 86) to QS.
Conflict of Interest
The authors declare that the research was conducted in the absence of any commercial or financial relationships that could be construed as a potential conflict of interest.
Publisher’s Note
All claims expressed in this article are solely those of the authors and do not necessarily represent those of their affiliated organizations, or those of the publisher, the editors and the reviewers. Any product that may be evaluated in this article, or claim that may be made by its manufacturer, is not guaranteed or endorsed by the publisher.
Supplementary Material
The Supplementary Material for this article can be found online at: https://www.frontiersin.org/articles/10.3389/fgene.2021.707228/full#supplementary-material
Footnotes
References
Altschmied, J., Delfgaauw, J., Wilde, B., Duschl, J., Bouneau, L., Volff, J. N., et al. (2002). Subfunctionalization of duplicate mitf genes associated with differential degeneration of alternative exons in fish. Genetics 161, 259–267.
Bian, C., Chen, W., Ruan, Z., Hu, Z., Huang, Y., Lv, Y., et al. (2020). Genome and transcriptome sequencing of casper and roy zebrafish mutants provides novel genetic clues for iridophore loss. Int. J. Mol. Sci. 21:2385. doi: 10.3390/ijms21072385
Birney, E., Clamp, M., and Durbin, R. (2004). GeneWise and Genomewise. Genome Res. 14, 988–995. doi: 10.1101/gr.1865504
Braasch, I., Brunet, F., Volff, J. N., and Schartl, M. (2009a). Pigmentation pathway evolution after whole-genome duplication in fish. Genome Biol. Evol. 1, 479–493. doi: 10.1093/gbe/evp050
Braasch, I., Liedtke, D., Volff, J. N., and Schartl, M. (2009b). Pigmentary function and evolution of tyrp1 gene duplicates in fish. Pigment Cell Melanoma Res. 22, 839–850. doi: 10.1111/j.1755-148X.2009.00614.x
Braasch, I., Schartl, M., and Volff, J. N. (2007). Evolution of pigment synthesis pathways by gene and genome duplication in fish. BMC Evol. Biol. 7:74. doi: 10.1186/1471-2148-7-74
Brunberg, E., Andersson, L., Cothran, G., Sandberg, K., Mikko, S., and Lindgren, G. (2006). A missense mutation in PMEL17 is associated with the Silver coat color in the horse. BMC Genet. 7:46. doi: 10.1186/1471-2156-7-46
Burton, D. (2011). “THE skin | coloration and chromatophores in fishes,” in Encyclopedia of Fish Physiology: from Genome to Environment, ed. A. P. Farrell (Amsterdam: Elsevier), 489–496. doi: 10.1016/b978-0-12-374553-8.00041-1
Capella-Gutierrez, S., Silla-Martinez, J. M., and Gabaldon, T. (2009). trimAl: a tool for automated alignment trimming in large-scale phylogenetic analyses. Bioinformatics 25, 1972–1973. doi: 10.1093/bioinformatics/btp348
Chakraborty, A. K., Platt, J. T., Kim, K. K., Kwon, B. S., Bennett, D. C., and Pawelek, J. M. (1996). Polymerization of 5,6-dihydroxyindole-2-carboxylic acid to melanin by the pmel 17/silver locus protein. Eur. J. Biochem. 236, 180–188. doi: 10.1111/j.1432-1033.1996.t01-1-00180.x
Crawford, N., Kelly, D., Hansen, M., Holsbach Beltrame, M., Fan, S., Bowman, S., et al. (2017). Loci associated with skin pigmentation identified in African populations. Science 358:eaan8433. doi: 10.1126/science.aan8433
del Marmol, V., and Beermann, F. (1996). Tyrosinase and related proteins in mammalian pigmentation. FEBS Lett. 381, 165–168. doi: 10.1016/0014-5793(96)00109-3
Dessinioti, C., Stratigos, A. J., Rigopoulos, D., and Katsambas, A. D. (2009). A review of genetic disorders of hypopigmentation: lessons learned from the biology of melanocytes. Exp. Dermatol. 18, 741–749. doi: 10.1111/j.1600-0625.2009.00896.x
Du, J., Miller, A. J., Widlund, H. R., Horstmann, M. A., Ramaswamy, S., and Fisher, D. E. (2003). MLANA/MART1 and SILV/PMEL17/GP100 are transcriptionally regulated by MITF in melanocytes and melanoma. Am. J. Pathol. 163, 333–343. doi: 10.1016/S0002-9440(10)63657-7
Gross, J. B. (2012). The complex origin of Astyanax cavefish. BMC Evol. Biol. 12:105. doi: 10.1186/1471-2148-12-105
Gross, J. B., Borowsky, R., and Tabin, C. J. (2009). A novel role for Mc1r in the parallel evolution of depigmentation in independent populations of the cavefish Astyanax mexicanus. PLoS Genet. 5:e1000326. doi: 10.1371/journal.pgen.1000326
Guindon, S., Dufayard, J. F., Lefort, V., Anisimova, M., Hordijk, W., and Gascuel, O. (2010). New algorithms and methods to estimate maximum-likelihood phylogenies: assessing the performance of PhyML 3.0. Syst. Biol. 59, 307–321. doi: 10.1093/sysbio/syq010
Hart, J. C., and Miller, C. T. (2017). Sequence-based mapping and genome editing reveal mutations in stickleback Hps5 cause oculocutaneous albinism and the casper phenotype. G3 7, 3123–3131. doi: 10.1534/g3.117.1125
Hodgkinson, C. A., Moore, K. J., Nakayama, A., Steingrimsson, E., Copeland, N. G., Jenkins, N. A., et al. (1993). Mutations at the mouse microphthalmia locus are associated with defects in a gene encoding a novel basic-helix-loop-helix-zipper protein. Cell 74, 395–404. doi: 10.1016/0092-8674(93)90429-t
Howe, K., Clark, M. D., Torroja, C. F., Torrance, J., Berthelot, C., Muffato, M., et al. (2013). The zebrafish reference genome sequence and its relationship to the human genome. Nature 496, 498–503. doi: 10.1038/nature12111
Hrckova Turnova, E., Majchrakova, Z., Bielikova, M., Soltys, K., Turna, J., and Dudas, A. (2017). A novel mutation in the TYRP1 gene associated with brown coat colour in the Australian shepherd dog breed. Anim. Genet. 48:626. doi: 10.1111/age.12563
Hsiao, J. J., and Fisher, D. E. (2014). The roles of microphthalmia-associated transcription factor and pigmentation in melanoma. Arch. Biochem. Biophys. 563, 28–34. doi: 10.1016/j.abb.2014.07.019
Ishishita, S., Takahashi, M., Yamaguchi, K., Kinoshita, K., Nakano, M., Nunome, M., et al. (2018). Nonsense mutation in PMEL is associated with yellowish plumage colour phenotype in Japanese quail. Sci. Rep. 8:16732. doi: 10.1038/s41598-018-34827-4
Jaillon, O., Aury, J. M., Brunet, F., Petit, J. L., Stange-Thomann, N., Mauceli, E., et al. (2004). Genome duplication in the teleost fish Tetraodon nigroviridis reveals the early vertebrate proto-karyotype. Nature 431, 946–957. doi: 10.1038/nature03025
Kelsh, R. N., Brand, M., Jiang, Y. J., Heisenberg, C. P., Lin, S., Haffter, P., et al. (1996). Zebrafish pigmentation mutations and the processes of neural crest development. Development 123, 369–389. doi: 10.1242/dev.123.1.369
Kerje, S., Sharma, P., Gunnarsson, U., Kim, H., Bagchi, S., Fredriksson, R., et al. (2004). The Dominant white, Dun and Smoky color variants in chicken are associated with insertion/deletion polymorphisms in the PMEL17 gene. Genetics 168, 1507–1518. doi: 10.1534/genetics.104.027995
Klaassen, H., Wang, Y., Adamski, K., Rohner, N., and Kowalko, J. E. (2018). CRISPR mutagenesis confirms the role of oca2 in melanin pigmentation in Astyanax mexicanus. Dev. Biol. 441, 313–318. doi: 10.1016/j.ydbio.2018.03.014
Kobayashi, T., and Hearing, V. J. (2007). Direct interaction of tyrosinase with Tyrp1 to form heterodimeric complexes in vivo. J. Cell Sci. 120(Pt 24), 4261–4268. doi: 10.1242/jcs.017913
Koludrovic, D., and Davidson, I. (2013). MITF, the Janus transcription factor of melanoma. Future Oncol. 9, 235–244. doi: 10.2217/fon.12.177
Krauss, J., Geiger-Rudolph, S., Koch, I., Nusslein-Volhard, C., and Irion, U. (2014). A dominant mutation in tyrp1A leads to melanophore death in zebrafish. Pigment Cell Melanoma Res. 27, 827–830. doi: 10.1111/pcmr.12272
Kumar, S., Stecher, G., and Tamura, K. (2016). MEGA7: molecular evolutionary genetics analysis version 7.0 for bigger datasets. Mol. Biol. Evol. 33, 1870–1874. doi: 10.1093/molbev/msw054
Lamason, R. L., Mohideen, M. A., Mest, J. R., Wong, A. C., Norton, H. L., Aros, M. C., et al. (2005). SLC24A5, a putative cation exchanger, affects pigmentation in zebrafish and humans. Science 310, 1782–1786. doi: 10.1126/science.1116238
Levy, C., Khaled, M., and Fisher, D. E. (2006). MITF: master regulator of melanocyte development and melanoma oncogene. Trends Mol. Med. 12, 406–414. doi: 10.1016/j.molmed.2006.07.008
Li, J., Bed’hom, B., Marthey, S., Valade, M., Dureux, A., Moroldo, M., et al. (2019). A missense mutation in TYRP1 causes the chocolate plumage color in chicken and alters melanosome structure. Pigment Cell Melanoma Res. 32, 381–390. doi: 10.1111/pcmr.12753
Lister, J. A., Close, J., and Raible, D. W. (2001). Duplicate mitf genes in zebrafish: complementary expression and conservation of melanogenic potential. Dev. Biol. 237, 333–344. doi: 10.1006/dbio.2001.0379
Lister, J. A., Robertson, C. P., Lepage, T., Johnson, S. L., and Raible, D. W. (1999). nacre encodes a zebrafish microphthalmia-related protein that regulates neural-crest-derived pigment cell fate. Development 126, 3757–3767. doi: 10.1242/dev.126.17.3757
Liu, K., Xu, D., Li, J., Bian, C., Duan, J., Zhou, Y., et al. (2017). Whole genome sequencing of Chinese clearhead icefish, Protosalanx hyalocranius. Gigascience 6, 1–6. doi: 10.1093/gigascience/giw012
Mack, M., Kowalski, E., Grahn, R., Bras, D., Penedo, M. C. T., and Bellone, R. (2017). Two variants in SLC24A5 are associated with “Tiger-Eye” Iris pigmentation in Puerto Rican Paso Fino horses. G3 7, 2799–2806. doi: 10.1534/g3.117.043786
McGaugh, S., Gross, J., Aken, B., Blin, M., Borowsky, R., Chalopin, D., et al. (2014). The cavefish genome reveals candidate genes for eye loss. Nat. Comm. 5:5307. doi: 10.1038/ncomms6307
Mount, D. W. (2007). Using the basic local alignment search tool (BLAST). CSH Protoc. 2007:dbto17. doi: 10.1101/pdb.top17
Nadeau, N. J., Mundy, N. I., Gourichon, D., and Minvielle, F. (2007). Association of a single-nucleotide substitution in TYRP1 with roux in Japanese quail (Coturnix japonica). Anim. Genet. 38, 609–613. doi: 10.1111/j.1365-2052.2007.01667.x
Nordlund, J. J. (1992). The pigmentary system and inflammation. Pigment Cell Res. 5(5 Pt 2), 362–365. doi: 10.1111/j.1600-0749.1992.tb00563.x
Oetting, W., and Setaluri, V. (2007). “The tyrosinase gene family,” in The Pigmentary System: Physiology and Pathophysiology, 2nd Edn, eds J. J. Nordlund, R. E. Boissy, V. J. Hearing, R. A. King, W. S. Oetting, J.-P. Ortonne, et al. (Hoboken, NJ: Blackwell Publishing Ltd), 213–229. doi: 10.1002/9780470987100.ch11
Oh, T. I., Yun, J. M., Park, E. J., Kim, Y. S., Lee, Y. M., and Lim, J. H. (2017). Plumbagin suppresses alpha-MSH-induced melanogenesis in B16F10 mouse melanoma cells by inhibiting tyrosinase activity. Int. J. Mol. Sci. 18:320. doi: 10.3390/ijms18020320
Protas, M., and Patel, N. (2008). Evolution of coloration patterns. Ann. Rev. Cell Dev. Biol. 24, 425–446. doi: 10.1146/annurev.cellbio.24.110707.175302
Protas, M. E., Hersey, C., Kochanek, D., Zhou, Y., Wilkens, H., Jeffery, W. R., et al. (2006). Genetic analysis of cavefish reveals molecular convergence in the evolution of albinism. Nat. Genet. 38, 107–111. doi: 10.1038/ng1700
Schonthaler, H. B., Lampert, J. M., von Lintig, J., Schwarz, H., Geisler, R., and Neuhauss, S. C. (2005). A mutation in the silver gene leads to defects in melanosome biogenesis and alterations in the visual system in the zebrafish mutant fading vision. Dev. Biol. 284, 421–436. doi: 10.1016/j.ydbio.2005.06.001
Singh, A., and Nüsslein-Volhard, C. (2015). Zebrafish stripes as a model for vertebrate colour pattern formation. Curr. Biol. 25, R81–R92. doi: 10.1016/j.cub.2014.11.013
Steingrimsson, E., Copeland, N. G., and Jenkins, N. A. (2004). Melanocytes and the microphthalmia transcription factor network. Annu. Rev. Genet. 38, 365–411. doi: 10.1146/annurev.genet.38.072902.092717
Wan, P., Hu, Y., and He, L. (2011). Regulation of melanocyte pivotal transcription factor MITF by some other transcription factors. Mol. Cell Biochem. 354, 241–246. doi: 10.1007/s11010-011-0823-4
Wang, K., Shen, Y., Yang, Y., Gan, X., Guichun, L., Hu, K., et al. (2019). Morphology and genome of a snailfish from the Mariana Trench provide insights into deep-sea adaptation. Nat. Ecol. Evol. 3, 823–833. doi: 10.1038/s41559-019-0864-8
White, R. M., Sessa, A., Burke, C., Bowman, T., LeBlanc, J., Ceol, C., et al. (2008). Transparent adult zebrafish as a tool for in vivo transplantation analysis. Cell Stem Cell 2, 183–189. doi: 10.1016/j.stem.2007.11.002
Widlund, H. R., and Fisher, D. E. (2003). Microphthalamia-associated transcription factor: a critical regulator of pigment cell development and survival. Oncogene 22, 3035–3041. doi: 10.1038/sj.onc.1206443
Yang, J., Chen, X., Bai, J., Fang, D., Qiu, Y., Jiang, W., et al. (2016). The Sinocyclocheilus cavefish genome provides insights into cave adaptation. BMC Biol. 14:1. doi: 10.1186/s12915-015-0223-4
Keywords: melanin synthesis pathway, core genes for melanin synthesis, albinism phenotype, nonsense mutation, phylogenetic analysis
Citation: Bian C, Li R, Wen Z, Ge W and Shi Q (2021) Phylogenetic Analysis of Core Melanin Synthesis Genes Provides Novel Insights Into the Molecular Basis of Albinism in Fish. Front. Genet. 12:707228. doi: 10.3389/fgene.2021.707228
Received: 09 May 2021; Accepted: 12 July 2021;
Published: 04 August 2021.
Edited by:
Liang Guo, Chinese Academy of Fishery Sciences (CAFS), ChinaReviewed by:
Deshou Wang, Southwest University, ChinaZaijie Dong, Chinese Academy of Fishery Sciences (CAFS), China
Copyright © 2021 Bian, Li, Wen, Ge and Shi. This is an open-access article distributed under the terms of the Creative Commons Attribution License (CC BY). The use, distribution or reproduction in other forums is permitted, provided the original author(s) and the copyright owner(s) are credited and that the original publication in this journal is cited, in accordance with accepted academic practice. No use, distribution or reproduction is permitted which does not comply with these terms.
*Correspondence: Wei Ge, d2VpZ2VAdW0uZWR1Lm1v; Qiong Shi, c2hpcWlvbmdAZ2Vub21pY3MuY24=