- 1Laboratorio de Dinámica de Redes Genéticas, Centro de Investigación en Dinámica Celular, Universidad Autónoma del Estado de Morelos, Cuernavaca, Mexico
- 2Centro de Ciencias de la Complejidad, Universidad Nacional Autónoma de México, Ciudad de México, Mexico
- 3Laboratorio de Genética Molecular, Epigenética, Desarrollo y Evoluciónde Plantas, Instituto de Ecología, Universidad Nacional Autónoma de México, Ciudad de México, Mexico
Current therapeutic strategies and vaccines against SARS-CoV-2 are mainly focused on the Spike protein despite there are other viral proteins with important roles in COVID-19 pathogenicity. For example, ORF8 restructures vesicular trafficking in the host cell, impacts intracellular immunity through the IFN-I signaling, and growth pathways through the mitogen-activated protein kinases (MAPKs). In this mini-review, we analyze the main structural similarities of ORF8 with immunological molecules such as IL−1, contributing to the immunological deregulation observed in COVID-19. We also propose that the blockage of some effector functions of ORF8 with Rapamycin, such as the mTORC1 activation through MAPKs 40 pathway, with Rapamycin, can be a promising approach to reduce COVID-19 mortality.
Introduction
The SARS-CoV-2 appeared in Wuhan at the end of December 2019 with the consequent crisis in the health systems due to the lack of an effective treatment to face a then unknown disease with a mortality of 10%. The implementation of physical distancing leads to an overall reduction in incidence by 13% (Islam et al., 2020). At the time of writing, 190 million infections and 4.14 million deaths have been reported. Although the mortality rate is reducing the number of infected patients is increasing, and there is still no effective pharmacological protocol against the disease. More than a year after the start of the pandemic, available vaccines are still uncertain since the virus genome has shown high genetic variability (Islam et al., 2020). Therefore, new drug strategies are needed for the prevention and treatment of the infection caused by this virus and the aftereffects of the disease. One of the most relevant characteristics of the virus is the strong immune response in some patients, in addition to some long-lasting pathologic consequences observed in convalescence patients.
The proteins encoded in the nine open reading frames (ORFs) of SARS-CoV-2 do not appear to be necessary for viral replication. However, they participate in the modulation of the metabolism of the infected host cells, the vesicular trafficking and packing of new viral particles, and the modification of the innate immunity (Gordon et al., 2020). From this group of proteins, ORF8 is the most connected hub with 47 links, and one of these links is the Tor1a (Torsin-1a) protein, that is involved in the quality control of protein folding in the ER (Hill et al., 2018; Gordon et al., 2020). ORF8 acts on ER to modulate the unfolded protein response (UPR) by up regulation of the ER-resident chaperones GRP78 and GRP94 leading to stimulate ATF6 and IRE1 pathways. Although, it does not seem to have any influence on the PERK pathway (Rashid et al., 2021; Figure 1). Thus, during SARS-CoV-2 infection, ORF8 takes the role of a central organizer of the activity of the virus-host hybrid network (the interactome model of viral components with the host proteins) toward the production of new virions (Díaz, 2020).
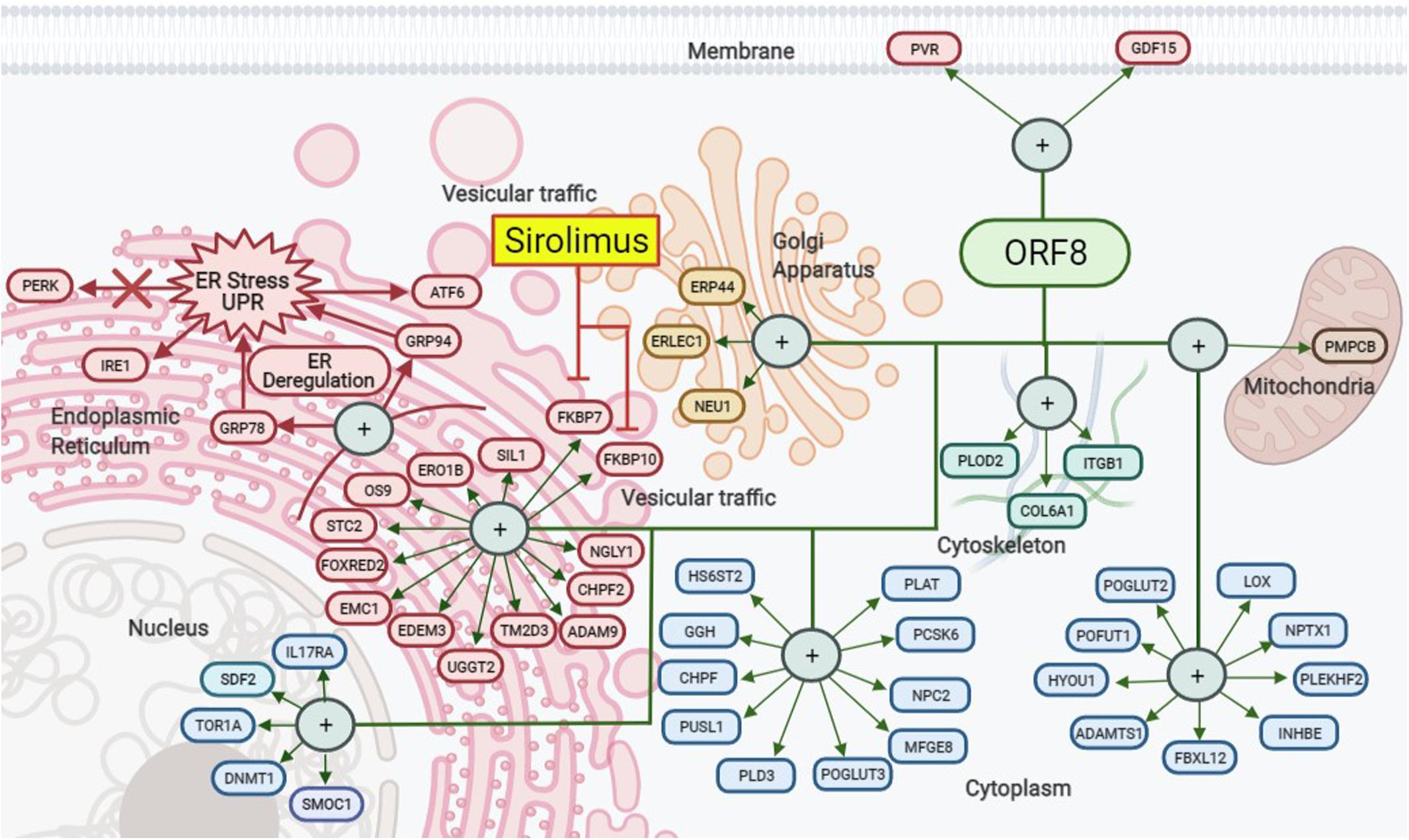
Figure 1. SARS-CoV-2 ORF8 protein interaction map. ORF8 modulates vesicular traffic through the unfolded protein response (UPR) and, therefore, ER stress by stimulating the ATF6 and IRE1 pathways through the upregulation of the GRP78 and GRP94 chaperones. Likewise, this process is linked to other intracellular interactions in the nucleus, Golgi apparatus, cytoskeleton, mitochondria, cytoplasm, and membrane. Rapamycin (Sirolimus) could indirectly decrease the effects of ORF8 by blocking FKBP7 and FKBP10 but not PERK pathway. Created with BioRender.com.
Coronaviruses show high genetic variability, and the structure of the SARS-CoV-2 genome consist of a set of conserved genes with an exceptionally low or null rate of mutation, together with a set of genes with high rate of variation. For example, of the 11,113 ORF8 sequences analyzed by Pereira (2020), the L84S substitution is the mutation that has been positively selected during the course of the pandemic. In 58 sites with this mutation the change in position 84 from leucine (observed in 85% of the sequences) to serine (observed in 15% of the sequences) stands up (Vilar and Isom, 2021; Zinzula, 2021). In the last group, the gene ORF8 (ORF8) has a notable tendency to recombine and undergo deletions that exceed the evolutionary capacity of its analogs in other coronaviruses, facilitating SARS-CoV-2 adaptability to new reservoirs and hosts (Abdelrahman et al., 2020; Zinzula, 2021). Despite the fact that truncations in ORF8 become more common as the pandemic progresses, and that these changes have apparently no influence on the replication of the virus, they are associated with non-synonymous mutations that increases the affinity of protein S for its receptor producing genetic variants with greater contagion capacity and an increased epidemiological persistence (Pereira, 2020).
During the first 6 months of the 2020 pandemic, 240 different non-synonymous mutations and 2 deletions in ORF8 have been found in 45,400 sequences. Approximately, 50% of these mutations are detrimental to the ORF8 protein, and 25% of them are among the conserved amino acids of other variants of coronavirus in animals. These mutations, regardless of their effects on ORF8 itself, can influence the biology of SARS-CoV-2 and slow down the discovery of new drugs, vaccines, and diagnostics against this coronavirus (Chan et al., 2020; Velazquez-Salinas et al., 2020; Alkhansa et al., 2021). An observational cohort study made in Singapore in the first 3 months of 2020, highlighted that an infection process with the D382 ORF8 variant induced late onset of pneumonia with milder symptoms, compared to the patients infected with the wild type (WT) ORF8. This result was associated with a lower probability of developing hypoxia and a better recovery from the disease (Young et al., 2020), possibly due to an elicited immune response in the absence of a fully functional ORF8. The most distinctive characteristic of severe COVID-19 is the accumulation of high levels of pro-inflammatory cytokines, chemokines, and growth factors that are systemically released and are associated with lung injury. However, in patients infected with the D382 ORF8 variant all these molecules were found in lower concentrations together with high levels of gamma interferon (IFN-γ), and other cytokines responsible for the activation of T cells, in contrast with patients infected with WT (Su et al., 2020). In 2018, a 29-nucleotide deletion in ORF8 was reported in the SARS-CoV genome, which was acquired during the first stage of person-to-person transmission. These observations point to the fact that these genomic changes relate to the deletion mutations of ORF8, given SARS-CoV-2 some advantage in its process of adaptation to humans (Muth et al., 2018).
The new ORF8 encodes a 121 amino acid secretory protein with 55.4% nucleotide similarity, and 30% protein identity with SARS-CoV counterpart. However, despite this genomic divergence, they share structural similarities as they both present a cavity with adequate electrostatic charges for protein–protein interaction (Neches et al., 2021). Structurally, SARS-CoV-2 ORF8 is a dimer in which each chain is made up of an alpha helix, followed by six-stranded chain β sheet, and an N-terminal hydrophobic signal peptide (1–15 aa of length) that promotes its import into the ER lumen where it can interact with a wide range of host proteins (Gordon et al., 2020; Rashid et al., 2021). This, together with two dimerization interfaces, means that ORF8 has a high possibility of forming unique complexes that can take part in immunological activity. This dimerization is probably an adaptative characteristic absent in homologs from other coronaviruses (Flower et al., 2020).
Among the functions that ORF8 plays in the evasion of the immune system are the activation of IL-17 signaling pathway, and the promotion of the expression of pro-inflammatory factors, supporting the lower intensity and late response to pneumonia caused by the D382 ORF8 variant. Additionally, association of ORF8 deletion variant (D382 variant) with milder disease outcome strongly supports the importance of ORF8 protein as a therapeutic target against SARS-CoV-2 (Sharma et al., 2021). However, the search for direct inhibition drugs of ORF8 is difficult due to the globular structure and high variability of this viral component. Another distinct function of ORF8 protein, different form SARS-CoV 29 nucleotide deleted versions- ORF8a and ORF8b (Pereira, 2021), is the regulation of the amount of MHC-I on the surface of the infected cell through a mechanism of lysosomal degradation dependent on autophagy. This results in dysregulated and deficient antigen presentation, hindering the recognition and elimination of infected cells (Zhang et al., 2020; de Sousa et al., 2020). Recently, computational experiments of homology modeling and molecular coupling suggested that a high expression of ORF8 and the surface glycoprotein may interact with heme porphyrin in the 1-beta chain of hemoglobin, resulting in a significant decrease in gas exchange processes and aggravating hypoxia in patients with severe disease (Liu and Li, 2020). However, these observations are still under investigation because of their clinical implications.
The joint action of ORF8, Nsp1, and Nsp6 results in a significant decrease in the production of IFN-I through different mechanisms to suppress signaling and produce failures and incorrect immune response, which favors the replication and transmission of the virus, to other host cells (Xia et al., 2020). Another example of this synergy is Nsp5, Nsp7, ORF3b, and M that can act together with ORF8 in more than one cell organelle, as in the case of stress-induced to ER (Figure 1; Gordon et al., 2020).
The diverse immune response evasion strategies generating an adaptative advantage for SARS-CoV-2 survival and propagation could be a result of functional mimicry that intensifies the host-pathogen interaction. An example of this functional mimicry comes from in silico simulations of ORF8-substrate complexes with F1 and C3b. The results of the coupling suggest that ORF8 can have interactions based on its mimicry with host targets inside and outside of the ER. Even a high extracellular concentration of ORF8 could have unknown interactions with other cell types different from lung alveolar type 2 cells based on possible putative functions conferred by its Ig-like structure. According to the structural alignment of the monomer of SARS-CoV-2 ORF8 (PDB: 7JTL) with SARS-CoV ORF7a (PDB: 1XAK) (Dali Server, Z-score = 4.6, RMSD = 2.4) they share two sets of structural disulfide bonds generating a fold like Ig conformation (Flower et al., 2020). Using the Dali server (Holm, 2020), result in more than one hundred immunoglobulins reporting a Z score higher than 3.9, and RMSD values between 2.7 and 3.6. The most outstanding results are shown in Table 1, in which it is possible to identify their role in mimicking possible host factors.
ORF8 mimics ALCAM (CD166), which is a structural protein that can activate ERK (Ibáñez et al., 2006). Once activated, ERK stimulates cell growth through the indirect activation of mTORC1 (Saxton and Sabatini, 2017). Some observations in vitro from MERS-CoV replication determine that mTORC1 activity is crucial for viral replication, and that the drug Rapamycin can abrogate 60% of the production of new virions (Kindrachuk et al., 2015). Additionally, ORF8 also mimics DNAM-1 (CD266), which is an important molecule that activates Natural Killer (NK) cells (Zhang et al., 2015; Wang et al., 2019), and has been implicated in the regulation of T CD8+ activation (Gilfillan et al., 2008), which can be used by the virus as a potent mechanism to evade the immune response. Moreover, ORF8 also has similarities with OX-2 (CD200) (Hatherley et al., 2013), which is an inhibitory molecule of macrophages (Gordon et al., 2020). Other effect of the structural mimicry of ORF8 is its ability to activate the immune response by itself due to its similarity with the soluble IL-1β receptor and IL-1RA agonists, stimulating the inflammation process. ORF8 also mimics CD79B (3KG5-A) and CD80 (1DR9-A), which are antigens required to activate B and T cell effector functions, respectively (Vasile et al., 1994; Trzupek et al., 2019). However, ORF8 is not precisely equal to such antigens, and can produce an incomplete stimulation of the receptors. In a biological context, incomplete stimulation produces anergy (Rollins and Gibbons, 2017), which may be used by SARS-CoV-2 to enhance its replication.
Discussion
Current pharmacological strategies to control SARS-CoV-2 infection are mainly focused on inhibiting spike-ACE2 interaction, and to block viral RNA synthesis. Some examples of these drugs are Remdesivir, Lopinavir and Ritonavir, which have been tested on many clinical trials around the world (McKee et al., 2020). Unfortunately, these drugs have little or no effect on patients of COVID-19 (WHO, 2021). Therefore, it is urgent to find novel viral therapeutic targets to control COVID-19. In this regard, evidence shows that cells in which ORF8 is expressed, MHC-I molecules selectively target lysosomal degradation by autophagy and hinder antigen presentation by reducing the recognition and clearance of infected cells. Other pathways of recognition interrupted by the presence of ORF8 are IFN-I signaling, and NF-κB functions. ORF8 also activates the ERK pathway through CD166 signaling (Bouhaddou et al., 2020) and it stimulates growth pathways directly as reported by Gordon et al. (2020). Likewise, ORF8 mimics immune molecules such as IL-1β, activating immunological effector signals from B cells and inhibitory molecules from immune cells such as macrophages, CD8+ T lymphocytes and NK cells (Table 1). These facts pointed out the multi-organizational role of ORF8 inside the host cells. The central question that remains is how all these functions are contributing to ensure viral replication. It seems that all ORF8 interactions are focused on provide an intracellular favorable environment to viral seems that all ORF8 interactions favor a suitable environment for viral replication through activated growth pathways and downregulated immune system, all through the inactivation of macrophages, NK cells, B cells and CD8+ T lymphocytes, making ORF8 a feasible therapeutic target (Supplementary Figure 1). This complex network of interactions contributes to worsen the immune deregulation observed in severe cases of COVID-19 (Pasrija and Naime, 2021).
Consequently, ORF8 is a feasible therapeutic target to simultaneously shut-down viral replication and host immune downregulation. However, ORF8 is a highly mutating region of the SARS-CoV-2 genome, which decreases the feasibility of ORF8 as a good therapeutic target (Zinzula, 2021), hindering the search for inhibitory drugs. A valid approach to overcome this obstacle is either targeting ORF8 immunological functions or its growth-promoting functions. In general, several RNA viruses such as hepatitis C virus, influenza A virus, Zika virus, and MERS-CoV require a specific metabolic environment and have their own activator mechanisms that ensure the intracellular proliferation of the virus (Karam et al., 2021), In the particular case of SARS-CoV-2, ORF8 can activate the mTOR-PI3K-AKT signaling pathway with a MAPK-dependent process to ensure a proliferative environment. This favorable environment can be blocked with inhibitors of mTORC1 like Rapamycin. It has been reported for the case of MERS-CoV replication that Rapamycin was able to reduce 60% of this virus (Kindrachuk et al., 2015). Thus, the blockage of growth pathways to prevent ORF8 biding interactions can be a better option than the targeting of several immune cells to stop viral infection.
It is possible that Rapamycin, as a co-adjuvant treatment, can improve clinical outcome because it is able to block viral interactions that promotes cell growth, and viral replication. Moreover, Rapamycin can reduce pro-inflammatory cytokines decreasing cell damage in patients with severe COVID-19 (Bischof et al., 2021). The metabolic changes conferred by SARS-CoV-2 infection in renal epithelial cells and lung air-fluid interface (ALI) cultures, showed that SARS-CoV-2 infection reduces the oxidative metabolism of glutamine while maintaining reductive carboxylation, increasing the activity of mTORC1. The work of Mullen et al. (2021) provide evidence of mTORC1 activation in lung tissue from COVID-19 patients, and that mTORC1 inhibitors reduce viral replication in renal epithelial cells and lung ALI cultures. These results suggest that targeting mTORC1 can be a feasible treatment strategy for COVID-19 patients, although more studies are required to determine the mechanism of inhibition and potential efficacy in patients. Rapamycin (Sirolimus) was chosen as it can interact through its methoxy group with the immunophilin binding protein FK506 (FKBP12) forming the rapamycin-FKBP12 complex that is highly specific to the mTOR protein, inhibiting effector processes such as antigen-induced T cell proliferation and cytokine-induced proliferative responses. From the family of polyketide macrolide drugs, Rapamycin (Sirolimus) it is the most studied and unlike Tacrolimus, it does not inhibit calcineurin (PP2B). Despite the fact that the effectiveness of Rapamycin has already been proven as a promising anti-covid drug, the interaction effects with another anti-inflammatory compounds are still to be discovered and open the possibility to have better therapeutic results with lower doses, avoiding toxic effects during the treatment.
The actual evidence shows that the variations observed in the most unstable region of the SARS-CoV-2 genome result in changes in the structure and functions of a set of proteins that counteract the immune response of the host (Supplementary Figure 1). However, SARS-CoV-2 seems not to have a mechanism that allows viral replication under non-permissive conditions (Figure 1). In consequence, the blockage of the activation of cell growth pathway through the inhibition of mTORC1 activity can be a therapeutic strategy that the virus possibly cannot counteract. Nonetheless, more research is necessary to explore the therapeutic use of Rapamycin against the SARS-CoV-2 infection.
Conclusion
ORF8 is the most linked protein in the virus-host hybrid molecular network formed during the SARS-CoV-2 infection. The structural properties of ORF8 suggest functional mimicry with several immunological molecules such as the IL-1β receptor, resulting in immune system evasion that helps the virus to adapt to new hosts. Additionally, ORF8 restructures the vesicular trafficking in the host cell, and enhances the activity of the growth pathway through the mitogen-activated protein kinases (MAPKs). However, the high mutation rate of ORF8 decreases its feasibility as a good therapeutic target. In consequence, the blockage of the activation of cell growth pathway through the inhibition of mTORC1 activity with Rapamycin can be a therapeutic strategy that the virus possibly cannot counteract.
Author Contributions
AV and AB wrote the manuscript and contributed equally to this work. AV, AB, EÁ-B, and JD conceived the study and discussed the content of the review. JD coordinated the study. All authors contributed to the article and approved the submitted version.
Funding
This work was supported by UNAM-PAPIIT IN211721. AV and AB were supported by CONACYT postdoctoral grants Modality 3 of ProNacEs.
Conflict of Interest
The authors declare that the research was conducted in the absence of any commercial or financial relationships that could be construed as a potential conflict of interest.
Publisher’s Note
All claims expressed in this article are solely those of the authors and do not necessarily represent those of their affiliated organizations, or those of the publisher, the editors and the reviewers. Any product that may be evaluated in this article, or claim that may be made by its manufacturer, is not guaranteed or endorsed by the publisher.
Acknowledgments
We thank PRODEP-UAEM and CONACYT for the financial support for this work. JD thanks to Erika Juárez Luna for her logistic support.
Supplementary Material
The Supplementary Material for this article can be found online at: https://www.frontiersin.org/articles/10.3389/fgene.2021.693227/full#supplementary-material
References
Abdelrahman, Z., Mengyuan, L., and Xiaosheng, W. (2020). Comparative review of SARS-CoV-2, SARS-CoV, MERS-CoV, and influenza a respiratory viruses. Front. Immunol. 11:552909. doi: 10.3389/fimmu.2020.552909
Alkhansa, A., Ghayas, L., and Loubna, E. Z. (2021). Mutational analysis of SARS-CoV-2 ORF8 during six months of COVID-19 pandemic. Gene Rep. 23:101024. doi: 10.1016/j.genrep.2021.101024
Bischof, E., Siow, R. C., Zhavoronkov, A., and Kaeberlein, M. (2021). The potential of rapalogs to enhance resilience against SARS-CoV-2 infection and reduce the severity of COVID-19. Lancet Healthy Longev. 2, e105–e111. doi: 10.1016/s2666-7568(20)30068-4
Bouhaddou, M., Memon, D., Meyer, B., White, K. M., Rezelj, V. V., Correa Marrero, M., et al. (2020). The global phosphorylation landscape of SARS-CoV-2 infection. Cell 182, 685–712.e19. doi: 10.1016/j.cell.2020.06.034
Chan, A. P., Choi, Y., and Schork, N. J. (2020). Conserved genomic terminals of SARS-COV-2 as co-evolving functional elements and potential therapeutic targets. BioRxiv [Preprint]. doi: 10.1101/2020.07.06.190207
Chappell, P. E., Garner, L. I., Yan, J., Metcalfe, C., Hatherley, D., Johnson, S., et al. (2015). Structures of CD6 and its ligand CD166 give insight into their interaction. Structure 23, 1426–1436. doi: 10.1016/j.str.2015.05.019
de Sousa, E., Ligeiro, D., Lérias, J. R., Zhang, C., Agrati, C., Osman, M., et al. (2020). Mortality in COVID-19 disease patients: correlating the association of major histocompatibility complex (MHC) with severe acute respiratory syndrome 2 (SARS-CoV-2) variants. Int. J. Infect. Dis. 98, 454–459. doi: 10.1016/j.ijid.2020.07.016
Díaz, J. (2020). SARS-CoV-2 molecular network structure. Front. Physiol. 11:870. doi: 10.3389/fphys.2020.00870
Flower, T. G., Buffalo, C. Z., Hooy, R. M., Allaire, M., Ren, X., and Hurley, J. H. (2020). Structure of SARS-CoV-2 ORF8, a rapidly evolving coronavirus protein implicated in immune evasion. BioRxiv [Preprint]. doi: 10.1101/2020.08.27.270637
Gilfillan, S., Chan, C. J., Cella, M., Haynes, N. M., Rapaport, A. S., Boles, K. S., et al. (2008). DNAM-1 promotes activation of cytotoxic lymphocytes by nonprofessional antigen-presenting cells and tumors. J. Exp. Med. 205, 2965–2973. doi: 10.1084/jem.20081752
Gordon, D. E., Jang, G. M., Bouhaddou, M., Xu, J., Obernier, K., White, K. M., et al. (2020). A SARS-CoV-2 protein interaction map reveals targets for drug repurposing. Nature 583, 459–468. doi: 10.1038/s41586-020-2286-9
Hatherley, D., Lea, S. M., Johnson, S., and Barclay, A. N. (2013). Structures of CD200/CD200 receptor family and implications for topology, regulation, and evolution. Structure 21, 820–832. doi: 10.1016/j.str.2013.03.008
Hill, A., Niles, B., Cuyegkeng, A., and Powers, T. (2018). Redesigning TOR kinase to explore the structural basis for TORC 1 and TORC 2 assembly. Biomolecules 8:36. doi: 10.3390/biom8020036
Holm, L. (2020). DALI and the persistence of protein shape. Prot. Sci. 29, 128–140. doi: 10.1002/pro.3749
Ibáñez, A., Sarrias, M., Farnós, M., Gimferrer, I., Serra-Pagès, C., Vives, J., et al. (2006). Mitogen-activated protein kinase pathway activation by the CD6 lymphocyte surface receptor. J. Immunol. 177, 1152–1159. doi: 10.4049/jimmunol.177.2.1152
Ikemizu, S., Robert, J. C., Gilbert, R. J. C., Fennelly, J. A., Collins, A. V., Harlos, K., et al. (2000). Structure and dimerization of a soluble form of B7-1. Immunity 12, 51–60. doi: 10.1016/S1074-7613(00)80158-2
Islam, M. R., Hoque, M. N., Rahman, M. S., Rubayet Ul Alam, A. S. M., Akther, M., Akter Puspo, J., et al. (2020). Genome-wide analysis of SARS-CoV-2 virus strains circulating worldwide implicates heterogeneity. Sci. Rep. 10, 1–9. doi: 10.1038/s41598-020-70812-6
Karam, B. S., Morris, R. S., Bramante, C. T., Puskarich, M., Zolfaghari, E. J., Lotfi-Emran, S., et al. (2021). MTOR inhibition in COVID-19: a commentary and review of efficacy in RNA viruses. J. Med. Virol. 93, 1843–1846. doi: 10.1002/jmv.26728
Kindrachuk, J., Ork, B., Hart, B. J., Mazur, S., Holbrook, M. R., Frieman, M. B., et al. (2015). Antiviral potential of ERK/MAPK and PI3K/AKT/MTOR signaling modulation for middle east respiratory syndrome coronavirus infection as identified by temporal kinome analysis. Antimicrob. Agents Chemother. 59, 1088–1099. doi: 10.1128/AAC.03659-14
Liu, W., and Li, H. (2020). COVID-19:attacks the 1-beta chain of hemoglobin and captures the porphyrin to inhibit human heme metabolism. ChemRxiv [Preprint]. doi: 10.26434/chemrxiv.11938173.v9
McKee, D. L., Sternberg, A., Stange, U., Laufer, S., and Naujokat, C. (2020). Candidate drugs against SARS-CoV-2 and COVID-19. Pharmacol. Res. 157:104859. doi: 10.1016/j.phrs.2020.104859
Mullen, P. J., Garcia, G., Purkayastha, A., Matulionis, N., Schmid, E. W., Momcilovic, M., et al. (2021). SARS-CoV-2 infection rewires host cell metabolism and is potentially susceptible to MTORC1 inhibition. Nat. Commun. 12:1876. doi: 10.1038/s41467-021-22166-4
Muth, D., Corman, V. M., Roth, H., Binger, T., Dijkman, R., and Theresa Gottula, l, et al. (2018). Attenuation of replication by a 29 nucleotide deletion in SARS-coronavirus acquired during the early stages of human-to-human transmission. Sci. Rep. 8, 1–11. doi: 10.1038/s41598-018-33487-8
Neches, R. Y., Kyrpides, N. C., and Ouzounis, C. A. (2021). Atypical divergence of SARS-CoV-2 Orf 8 from Orf 7a within the coronavirus lineage suggests potential stealthy viral strategies in immune evasion. mBio 12:e03014–20. doi: 10.1128/mBio.03014-20
Pasrija, R., and Naime, M. (2021). The deregulated immune reaction and cytokines release storm (CRS) in COVID-19 disease. Int. Immunopharmacol. 90:107225. doi: 10.1016/j.intimp.2020.107225
Pereira, F. (2020). Evolutionary dynamics of the SARS-CoV-2 ORF8 accessory gene. Infection, genetics and evolution. J. Mol. Epidemiol. Evol. Genet. Inf. Dis. 85:104525. doi: 10.1016/j.meegid.2020.104525
Pereira, F. (2021). SARS-CoV-2 variants combining spike mutations and the absence of ORF8 may be more transmissible and require close monitoring. Biochem. Biophys. Res. Commun. 550, 8–14. doi: 10.1016/j.bbrc.2021.02.080
Radaev, S., Zou, Z., Tolar, P., Nguyen, K., Nguyen, A., Krueger, P. D., et al. (2010). Structural and functional studies of Igαβ; and its assembly with the B cell antigen receptor. Structure 18, 934–943. doi: 10.1016/j.str.2010.04.019
Rashid, F., Dzakah, E. E., Wang, H., and Tang, S. (2021). The ORF8 protein of SARS-CoV-2 induced endoplasmic reticulum stress and mediated immune evasion by antagonizing production of interferon beta. Virus Res. 296:198350. doi: 10.1016/j.virusres.2021.198350
Rollins, M. R., and Gibbons, J. R. M. (2017). CD80 expressed by CD8 + T cells contributes to PD-L1-induced apoptosis of activated CD8 + T Cells. J. Immunol. Res. 2017:7659462. doi: 10.1155/2017/7659462
Saxton, R. A., and Sabatini, D. M. (2017). MTOR signaling in growth, metabolism, and disease. Cell 169, 361–371. doi: 10.1016/j.cell.2017.03.035
Schreuder, H., Tardif, C., Trump-Kallmeyer, S., Soffientini, A., Sarubbi, E., Akeson, A., et al. (1997). A new cytokine-receptor binding mode revealed by the crystal structure of the IL-1 receptor with an antagonist. Nature 386, 194–200. doi: 10.1038/386194a0
Sharma, H. B., Panigrahi, S., Sarmah, A. K., and Dubey, B. K. (2021). ORF8 contributes to cytokine storm during SARS-CoV-2 infection by activating IL-17 pathway. iScience 24:102293. doi: 10.1016/j.isci.2021.102293
Su, Y. C. F., Anderson, D. E., Young, B. E., Linster, M., Zhu, F., Jayakumar, J., et al. (2020). Discovery and genomic characterization of a 382-nucleotide deletion in ORF7B and Orf8 during the early evolution of SARS-CoV-2. MBio 11, 1–9. doi: 10.1128/mBio.01610-20
Trzupek, D., Dunstan, M., Cutler, A. J., Lee, M., Godfrey, L., Jarvis, L., et al. (2019). Discovery of CD80 and CD86 as recent activation markers on regulatory T cells by protein-RNA single-cell analysis. BioRxiv [Preprint]. doi: 10.1101/706275
Vasile, S., Coligan, J. E., Yoshida, M., and Seon, B. K. (1994). Isolation and chemical characterization of the human B29 and Mb-1 proteins of the B cell antigen receptor complex. Mol. Immunol. 31, 419–427. doi: 10.1016/0161-5890(94)90061-2
Velazquez-Salinas, L., Zarate, S., Eberl, S., Gladue, D. P., Novella, I., and Borca, M. V. (2020). Positive selection of ORF3a and ORF8 genes drives the evolution of SARS-CoV-2 during the 2020 COVID-19 pandemic. BioRxiv [Preprint]. doi: 10.1101/2020.04.10.035964
Vilar, S., and Isom, D. G. (2021). One Year of SARS-CoV-2: how much has the virus changed? Biology 10:91. doi: 10.3390/biology10020091
Wang, D., Zhang, S., Li, L., Liu, X., Mei, K., and Wang, X. (2010). Structural insights into the assembly and activation of IL-1β with its receptors. Nat. Immunol. 11, 905–911. doi: 10.1038/ni.1925
Wang, H., Qi, J., Zhang, S., Li, Y., Tan, S., and Gao, G. F. (2019). Binding mode of the side-by-side two-IgV molecule CD226/DNAM-1 to its ligand CD155/Necl-5. Proc. Natl. Acad. Sci. 116, 988–996. doi: 10.1073/pnas.1815716116
WHO (2021). Repurposed antiviral drugs for Covid-19 — interim WHO solidarity trial results. N. Engl. J. Med. 384, 497–511. doi: 10.1056/nejmoa2023184
Xia, H., Cao, Z., Xie, X., Zhang, X., Chen, J. Y., Wang, H., et al. (2020). Evasion of type I interferon by SARS-CoV-2. Cell Rep. 33:108234. doi: 10.1016/j.celrep.2020.108234
Young, B. E., Fong, S. W., Chan, Y. H., Mak, T. M., Ang, L. W., Anderson, D. E., et al. (2020). Effects of a major deletion in the SARS-CoV-2 genome on the severity of infection and the inflammatory response: an observational cohort study. Lancet 396, 603–611. doi: 10.1016/S0140-6736(20)31757-8
Zhang, Y., Zhang, J., Chen, Y., Luo, B., Yuan, Y., Huang, F., et al. (2020). The ORF8 protein of SARS-CoV-2 mediates immune evasion through potently downregulating MHC-I. BioRxiv [Preprint]. doi: 10.1101/2020.05.24.111823
Zhang, Z., Wu, N., Lu, Y., Davidson, D., Colonna, M., and Veillette, A. (2015). DNAM-1 controls NK cell activation via an ITT-like Motif. J. Exp. Med. 212, 2165–2182. doi: 10.1084/jem.20150792
Keywords: SARS-CoV-2, COVID-19, ORF8, structural biology, COVID-19 therapeutics
Citation: Valcarcel A, Bensussen A, Álvarez-Buylla ER and Díaz J (2021) Structural Analysis of SARS-CoV-2 ORF8 Protein: Pathogenic and Therapeutic Implications. Front. Genet. 12:693227. doi: 10.3389/fgene.2021.693227
Received: 10 April 2021; Accepted: 29 July 2021;
Published: 06 September 2021.
Edited by:
Dariusz Plewczynski, Warsaw University of Technology, PolandReviewed by:
Sandra Paulina Smieszek, Vanda Pharmaceuticals Inc., United StatesPragati Agnihotri, Advanced BioScience Laboratories, Inc. (ABL), United States
Copyright © 2021 Valcarcel, Bensussen, Álvarez-Buylla and Díaz. This is an open-access article distributed under the terms of the Creative Commons Attribution License (CC BY). The use, distribution or reproduction in other forums is permitted, provided the original author(s) and the copyright owner(s) are credited and that the original publication in this journal is cited, in accordance with accepted academic practice. No use, distribution or reproduction is permitted which does not comply with these terms.
*Correspondence: José Díaz, YmlvZmlzaWNhQHlhaG9vLmNvbQ==; Elena R. Álvarez-Buylla, ZWxlbmFidXlsbGFAcHJvdG9ubWFpbC5jb20=