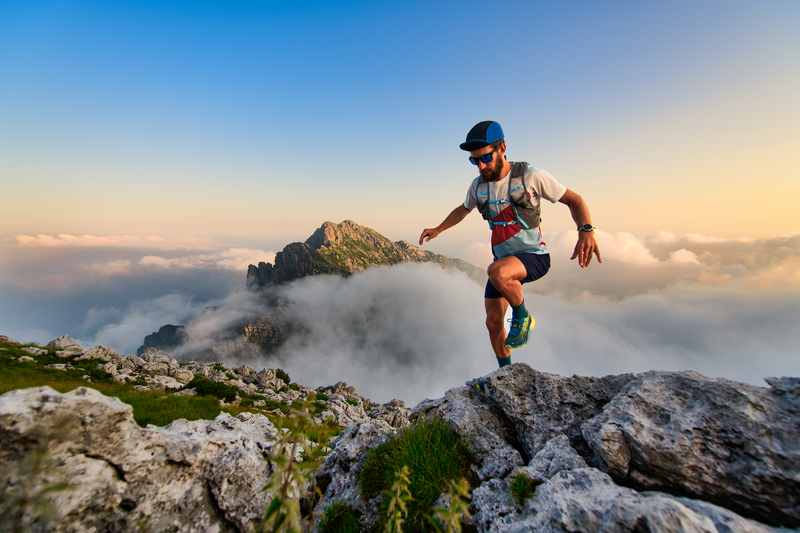
94% of researchers rate our articles as excellent or good
Learn more about the work of our research integrity team to safeguard the quality of each article we publish.
Find out more
ORIGINAL RESEARCH article
Front. Genet. , 16 April 2021
Sec. Genetics of Common and Rare Diseases
Volume 12 - 2021 | https://doi.org/10.3389/fgene.2021.640859
The etiology of hypertriglyceridemia (HTG) and acute pancreatitis (AP) is complex. Herein, we dissected the underlying etiology in a patient with HTG and AP. The patient had a 20-year history of heavy alcohol consumption and an 8-year history of mild HTG. He was hospitalized for alcohol-triggered AP, with a plasma triglyceride (TG) level up to 21.4 mmol/L. A temporary rise in post-heparin LPL concentration (1.5–2.5 times of controls) was noted during the early days of AP whilst LPL activity was consistently low (50∼70% of controls). His TG level rapidly decreased to normal in response to treatment, and remained normal to borderline high during a ∼3-year follow-up period during which he had abstained completely from alcohol. Sequencing of the five primary HTG genes (i.e., LPL, APOC2, APOA5, GPIHBP1 and LMF1) identified two heterozygous variants. One was the common APOA5 c.553G > T (p.Gly185Cys) variant, which has been previously associated with altered TG levels as well as HTG-induced acute pancreatitis (HTG-AP). The other was a rare variant in the LPL gene, c.756T > G (p.Ile252Met), which was predicted to be likely pathogenic and found experimentally to cause a 40% loss of LPL activity without affecting either protein synthesis or secretion. We provide evidence that both a gene-gene interaction (between the common APOA5 variant and the rare LPL variant) and a gene-environment interaction (between alcohol and digenic inheritance) might have contributed to the development of mild HTG and alcohol-triggered AP in the patient, thereby improving our understanding of the complex etiology of HTG and HTG-AP.
Acute pancreatitis (AP) is a common critical disease, has poor outcomes and a high mortality rate, and requires complex clinical management (Hazra and Gulliford, 2014; Lee and Papachristou, 2019). The etiology of AP is diverse (AGA, 2007; IAP/APA, 2013). In Western countries, gallstones and alcohol consumption represent the most common causes (Sekimoto et al., 2006; Yadav and Lowenfels, 2013) whereas in China, hypertriglyceridemia (HTG) rather than alcohol consumption is the second leading cause (Yin et al., 2015; Jin et al., 2019). Hypertriglyceridemia-induced acute pancreatitis (HTG-AP) has been defined as pancreatitis with a triglyceride (TG) level of up to 1000 mg/dL (11.3 mmol/L) alone, or 500 mg/dL (5.65 mmol/L) accompanied by lipemic or lactescent (milky in appearance) blood, after excluding other etiologies (Zafrir et al., 2018). By comparison with biliary acute pancreatitis, HTG-AP occurs earlier, is more severe, has a higher recurrence rate, and affects more males than females (Yin et al., 2015; Li et al., 2018; Jin et al., 2019).
HTG can be broadly divided into primary and secondary categories in terms of etiology (Yuan et al., 2007; Rygiel, 2018). Secondary HTG is primarily related to lifestyle factors such as heavy alcohol consumption and smoking, physiological factors such as pregnancy, or comorbid pathological conditions such as obesity, diabetes mellitus, hypothyroidism, and renal dysfunction (Black and Sprecher, 1993; Merkel et al., 2002). By contrast, primary HTG has been found to be mainly due to genetic defects in five lipid metabolism-related genes (Nawawi et al., 2020) namely LPL (lipoprotein lipase, OMIM #609708), LMF1 (lipase maturation factor 1, OMIM #611761), GPIHBP1 (glycosylphosphatidylinositol-anchored high density lipoprotein-binding protein 1, OMIM #612757), APOA5 (apolipoprotein A-V, OMIM #606368), and APOC2 (apolipoprotein C-II, OMIM #608083). LPL is the key enzyme catabolizing triglyceride (Olivecrona, 2016). LMF1 functions in the maturation of LPL (Peterfy et al., 2007). GPIHBP1 mediates the transmembrane transport and binding of LPL (Beigneux et al., 2007). APOA5 and APOC2 act as basic activators of LPL activity (Breckenridge et al., 1978; Pennacchio et al., 2001).
The abovementioned dichotomous classification of HTG provides a useful framework for understanding the etiology of HTG. However, in most cases, the etiology of HTG is complex, and often involves primary-primary (i.e., gene-gene) and primary-secondary (i.e., gene-environment) interactions (Wang et al., 2008; Hegele et al., 2014; Cole et al., 2015; Serveaux Dancer et al., 2018; Chen et al., 2019; Dron et al., 2019; Pu et al., 2020). Herein, we describe the clinical, genetic and functional analysis of a patient with alcohol-triggered HTG-AP, which serves to provide us with several novel insights into the complex etiology of HTG.
This study was approved by the Ethics Committee of Jinling Hospital. Informed consent for publication was obtained from all participants.
The male Chinese patient experienced sudden upper abdominal pain after consuming ∼150 g alcohol on October 29, 2017. He was initially admitted to a local hospital: physical examination revealed epigastric tenderness, without Gray Turner’s or Cullen’s sign; amylase increased from 305 U/L to 1,294 U/L over one night; a computed tomography (CT) scan indicated AP. He was transferred to our severe acute pancreatitis treatment center in Jinling Hospital on October 31, 2017. Diagnosis of AP and classification of the disease in terms of clinical severity were made in accordance with the modified Atlanta classification (Banks et al., 2013).
Fasting blood samples were collected for this analysis. Plasma lipid profiles including TG, total cholesterol (TC), high-density lipoprotein cholesterol (HDL-C) and low-density lipoprotein cholesterol (LDL-C), as well as plasma glucose were measured enzymatically on an automatic analyzer (Hitachi High-Tech, 7600–120, Japan).
Blood samples were collected after overnight fasting and 10 min after intravenous heparin injection (60 IU/kg body weight). LPL mass was determined using a human LPL Elisa kit (TSZ Biological Trade Co., Ltd., San Francisco, CA, United States) in accordance with the manufacturer’s instructions. To measure LPL activity, plasma total lipase activity and hepatic lipase activity were first determined by a LPL-mediated lipolysis reaction; this was performed by means of a free fatty acid (FFA) release assay kit [Wako kit# NEFA-HR(2), Japan], using TG-rich plasma from GPIHBP1-deficient (GPIHBP1–/–) mice as the lipolytic substrate (Li et al., 2020; Shi et al., 2020). It should be noted that, for the hepatic lipase activity assay, the sample was pretreated with 1 M NaCl and incubated for 60 min at 4°C in order to inactivate LPL. Subtraction of the hepatic lipase activity from the total lipase activity then yielded a measure of the plasma LPL activity. All assays were performed in five replicates. Eight healthy volunteers from our center (four males and four females with a mean age of 28 years) were used as controls.
Genomic DNA was extracted from a blood sample using the TIANamp Blood DNA kit (TIANGEN Biotech, Beijing, China) according to the manufacturer’s instructions. All coding and proximal intronic regions of the LPL, APOC2, APOA5, GPIHBP1, and LMF1 genes were analyzed by Sanger sequencing as previously described (Chen et al., 2019; Pu et al., 2020). All primer sequences are available upon email request.
NM_000237, NM_000483, NM_001371904, NM_178172, and NM_022773 were used as the mRNA reference sequences for the LPL, APOC2, APOA5, GPIHBP1, and LMF1 genes, respectively. Variant nomenclature followed the Human Genome Variation Society (HGVS) recommendations (den Dunnen et al., 2016). Variant population allele frequencies were in accordance with the Genome Aggregation Database (gnomAD)1 (Karczewski et al., 2020). The Human Gene Mutation Database (HGMD)2 (Stenson et al., 2020), ClinVar3, the LPL locus-specific mutation database4 and PubMed5 were used to establish whether or not a given variant had been previously described. LPL amino acid sequences from diverse vertebrate species were taken from https://www.ncbi.nlm.nih.gov/home/proteins/to evaluate the evolutionary conservation status of the p.Ile252 position. As previously described (Guéguen et al., 2020), we used the PP3 rule established by VarSome (Kopanos et al., 2019) to predict the pathogenicity of missense variants; the PP3 verdict was based upon computational evidence derived from 13 in silico algorithms (i.e., BayesDel_addAF, DANN, DEOGEN2, EIGEN, FATHMM-MKL, M-CAP, MutationAssessor, MutationTaster, MVP, PrimateAI, REVEL, and SIFT).
The wild-type LPL cDNA expression vector has been previously described (Li et al., 2020; Shi et al., 2020) and was used here to generate the mutant [c.756T > G (p.Ile252Met)] expression vector by site-directed mutagenesis. The mutated site was verified by Sanger sequencing. Human embryonic kidney 293T (HEK293T) cells (ATCC, CRL-3216) were cultured in Dulbecco’s Modified Eagle’s Medium (DMEM, Lonza, C11995500BT) with 10% Fetal Bovine serum (FBS) and 1% penicillin-streptomycin at 37°C with 5% CO2. Transfections were performed using Lipofectamine 3000 (Thermo, L3000015) in 6-well plates, essentially as previously described (Yu et al., 2006; Han et al., 2020). It should, however, be noted that in addition to the wild-type expression vector, mutant expression vector and empty vector transfection groups, a new group mimicking the heterozygous state of the variant was included here. More specifically, whereas the former three groups each used 3 μg of the respective plasmid for transfection, the new group used a mix of 1.5 μg wild-type plasmid and 1.5 μg mutant plasmid for transfection. Moreover, whereas the transfection time (i.e., from addition of the plasmid into the culture media to the change to fresh media) was invariably maintained for 6 h, downstream treatments were different in accordance with the subsequent Western blot analysis. Thus, for analysis of LPL expressed in the transfected cells without heparin treatment, the cells were cultured for 48 h with the fresh media before cell lysate preparation. For analysis of LPL expressed in the transfected cells and secreted into the media, the fresh medium was further changed to a 500 μL heparin-DMEM mixture (the ratio of DMEM and heparin was 500:8, whereas heparin was 20 units/mL) per well; the cells were cultured for an additional 30 min. Three independent transfections were performed for each experiment.
Transfected cell medium was centrifuged at 12,000 r/min for 5 min to remove cells and cell debris and stored at –80°C for later analysis. Transfected cells were treated with 80 μL RIPA Lysis Buffer (Beyotime, P0013B, China) and 8 μL protease inhibitor (PI, Roche, 4693116001) and centrifuged at 12,000 r/min for 5 min; the supernatant was stored at −80°C for subsequent analysis. Protein concentration was determined by BCA kit.
30 μg cell lysates or 200 μL cell media were mixed with SDS-PAGE Protein Loading Buffer (reducing) and incubated at 95°C for 10 min. It should be noted that the cell media were firstly concentrated in the upper layer of the polyacrylamide gel (concentration of 12%) by repeated sample loading (40 μL × 5 times; each time run at 90 V for 15 min). Proteins were separated by SDS-PAGE (10% acrylamide gel, 130 V, 90 min), transferred onto a nitrocellulose membrane (220 mA, 120 min), and then blocked with 5% BSA (room temperature, 60 min). After washing with 0.2% Tris-Buffered Saline with Tween 20 (TBST), membranes were incubated overnight with mouse anti-LPL antibody (Santa, sc-73646; 1:200 dilution) at 4°C. After washing with 0.2% TBST, membranes were incubated for 1 h with goat anti-mouse (Abcam, ab6728; 1:2000 dilution) IgG H&L (HRP) antibody, and again washed three times. After 5 min incubation with chemiluminescent HRP substrate (Thermo Fisher Scientific), bands were visualized and analyzed by the Chemidoc XRS System, Image Lab Software (Clinx Science Instruments, Shanghai, China). The protein bands were quantified by densitometry and normalized to GAPDH (Santa, sc-69778; 1:2000 dilution).
This was performed in the same way as for the analysis of LPL activity in post-heparin plasma, but without the treatment with 1M NaCl, as there was no hepatic lipase activity in the cell medium or lysates. Each assay was performed in five replicates.
Results from Western blot and LPL activity analysis in transfected cell medium and lysates are shown as mean ± standard deviation (SD) from at least three independent experiments. The differences between groups were evaluated by means of a Student’s t-test using SPSS 24.0, with p-values less than 0.05 being considered indicative of statistical significance.
The patient suffered from an attack of AP that appears to have been triggered by the consumption of ∼150 g alcohol on October 29, 2017. On day 3 (October 31, 2017) of the disease onset, he was transferred from the local hospital to our therapy center at Jinling Hospital, one of the biggest referral centers for severe AP treatment in China. In our center, the patient was more precisely diagnosed as having HTG-AP on the basis that his TG level was as high as 17.8 mmol/L (Figure 1). Figure 2 shows computed tomography images of the affected pancreas from two time points during the early days of the disease. Continuous renal replacement therapy (CRRT) and pulmonary ventilation were performed to rescue renal and respiratory function, respectively. After a 64-day hospitalization in our intensive care unit, and 40 days on a normal ward, he was discharged on February 11, 2018.
Figure 1. Dynamic changes of the fasting plasma TG levels, post-heparin LPL mass and LPL activity during hospitalization and follow-up periods of the patient with alcohol-triggered HTG-AP. Day 3 (D3), numbered by reference to the time of disease onset, corresponds to the date when the patient was transferred to our service. See Table 1 for the precise values of the three parameters. HTG-AP, hypertriglyceridemia-induced acute pancreatitis; LPL, lipoprotein lipase; ICU, intensive care unit.
Figure 2. Abdominal computed tomography images of the patient showing the affected pancreas. The left image was taken on the third day of onset of acute pancreatitis, showing peripancreatic exudation (arrow) and the enlarged pancreas with adjacent water density shadow. The right image was taken on the eighteenth day of disease onset, showing the occurrence of infected pancreatic necrosis. The box indicates a partial necrotic pancreas whilst the arrows indicate infective necrosis.
The patient had an 8-year history of mild HTG (a fasting plasma TG level of ∼5 mmol/L) and a 20-year history of continuous consumption of ∼100 g alcohol per day. He had no prior attacks of AP. He had had no cholelithiasis, diabetes or other diseases that are known to cause HTG. He also had no history of drug abuse. There was no family history of AP. His elder brother was, however, reported to have drunk >80 g alcohol per day for >20 years and had a borderline high TG (2.08 mmol/L).
Plasma lipid profiles and post-heparin LPL mass and activity in the patient were determined regularly during his hospital stay and follow-up period (Table 1 and Figure 1). The highest LPL mass (429.0 U/L; ∼2.3 times higher than normal controls) was observed on day 5 whereas the lowest LPL activity (0.8841 mEq/L/h; ∼50% of normal controls) was observed on day 6 of AP onset. It should be noted that during the follow-up period, the patient abstained from both drinking and smoking as advised; his TG levels remained at normal or at most borderline high levels. The normal post-heparin LPL mass and activity values were averages derived from eight healthy controls (Table 1). Additionally, post-heparin LPL mass and activity in the elder brother (187.6 U/L and 1.7112 mEq/L/h, respectively) and son (274.3 U/L and 1.7338 mEq/L/h, respectively) were found to be within the normal range (184.4 ± 56.1 U/L and 1.7242 ± 0.4358 mEq/L/h, respectively).
Sequencing of the LPL, APOA5, APOC2, LMF1, and GPIHBP1 genes in the patient led to the identification of two heterozygous variants (Figure 3A). One was the common APOA5 c.553G > T (p.Gly185Cys; rs2075291) variant, which has previously been associated with altered TG levels (Kao et al., 2003; Tang et al., 2006) as well as HTG-AP (Pu et al., 2020). The other is a rare variant in the LPL gene, c.756T > G (p.Ile252Met). This latter variant has an allele frequency of 0.000003978 (1/251358) in the gnomAD dataset (see text footnote 1). It does not, however, appear in the literature nor has it been registered in HGMD (see text footnote 2), ClinVar (see text footnote 3) or the LPL locus-specific mutation database (see text footnote 4) (as of 22 September 2020). The LPL p.Ile252 position is strictly conserved during mammalian and avian evolution (Figure 3C) and the p.Ile252 variant was predicted to be “pathogenic” in accordance with the PP3 rule established by VarSome (Kopanos et al., 2019). This pathogenicity assessment was based on nine independent predictions from BayesDel_addAF, DANN, DEOGEN2, FATHMM-MKL, M-CAP, MutationAssessor, MutationTaster, REVEL, and SIFT against 3 benign predictions from EIGEN, MVP, and PrimateAI.
Figure 3. Genetic variants detected in the patient and the family tree. (A) Sanger sequencing electropherogram showing the novel LPL c.756T > G (p.Ile252Met) variant and the known common APOA5 c.553G > T (p.Glu185Cys) variant identified in the patient. The variants are indicated by arrows. (B) Arrow denotes the patient. Genotype status with respect to the APOA5 c.553G > T and LPL c.756T > G variants, age, BMI and TG levels are provided for each subject. (C) Alignment of partial LPL amino acid sequences spanning the p.252 site. LPL, lipoprotein lipase; APOA5, apolipoprotein A5; BMI, body mass index; het, heterozygous; wt, wild-type; TG, triglyceride; NA, not analyzed.
The patient’s older brother, wife and son were also available for genetic analysis. The brother and son were found to carry the common APOA5 p.Gly185Cys variant (Figure 3B).
We firstly performed Western blot analysis of LPL expressed in the transfected cells without heparin treatment. No difference was found between the wild-type, wild-type/mutant, and mutant expression vector groups (Figure 4). We then performed western blot analysis of LPL present in the transfected cell lysates and those secreted into the culture medium after heparin treatment (exogenous heparin promotes the release of cell surface-bound LPL into the medium). No difference was found between the three groups in either case (Figure 5). However, the mutant expression vector yielded reduced LPL activity in both cell lysates and medium. More specifically, the reduction from the “homozygous” mutant expression vector was approximately double that from the “heterozygous” mutant expression vector and, if expressed in the context of alleles, we estimate that the mutation caused a 40% loss of enzyme activity as compared to the wild-type (Figure 6). Taken together, the LPL p.Ile252Met variant served to reduce the enzymatic activity of the LPL protein but did not exert a dominant-negative effect.
Figure 4. Western blot analysis of LPL expression in transfected HEK293T cells without heparin treatment. (A) Results were the average taken from three independent experiments. (B) LPL, lipoprotein lipase; WT, wild-type; EV, empty vector.
Figure 5. Western blot analysis of post-heparin LPL expression in cell lysates (A) and media (B) of transfected HEK293T cells. Results were the average taken from three independent experiments. LPL, lipoprotein lipase; WT, wild-type; EV, empty vector.
Figure 6. Post-heparin LPL activity in transfected HER293T cell lysates (A) and medium (B). Results were expressed as mean ± SD from three independent transfections, with each transfection being performed in five replicates. LPL, lipoprotein lipase; WT, wild-type; EV, empty vector. *p < 0.05; **p < 0.01; ***p < 0.001.
In the present study, we report the detailed clinical, biochemical and family analysis of a patient with alcohol-triggered HTG-AP as well as the in vitro functional analysis of the rare LPL p.Ile252Met variant. We obtained long-term data on TG levels and post-heparin LPL mass and activity for the patient and performed in vitro functional analysis of the missense variant of interest in both the “heterozygous” and “homozygous” state and under different experimental conditions (with and without heparin treatment). Integration of these findings provides several novel insights into the complex etiology of HTG and HTG-AP.
First, the patient was found to carry a known common risk single nucleotide polymorphism (SNP) (i.e., APOA5 c.553G > T (p.Gly185Cys); rs2075291) and a rare variant [i.e., LPL c.756T > G (p.Ile252Met)]. APOA5 c.553G > T has an allele frequency of 0.06765 in East Asians (according to gnomAD); and in the Asian population, c.553T carriers displayed an increased risk of HTG as compared with c.553 GG carriers, with the overall random effects odds ratio (OR) being 3.55 [95% confidence interval (CI) 2.46 to 5.13] in the context of a dominant genetic model (He et al., 2016). By reference to the definitions employed by Manolio et al. (2009), the APOA5 c.553G > T variant may be best described as a common genetic variant with moderate genetic effect (on HTG) in the Asian population. By contrast, the LPL c.756T > G (p.Ile252Met) variant is extremely rare in normal populations (allele frequency of 0.000003978 according to gnomAD) and has not previously been reported in HTG patients. The p. Ile252 site is conserved across the classes Mammalia and Aves (Figure 3C), suggesting its functional importance for protein structure and function. Consistent with the conservation of Ile252 over 300 million years of evolution, p.Ile252Met was predicted to be pathogenic by 9 of the 13 in silico algorithms employed by VarSome (Kopanos et al., 2019). Further, post-heparin LPL activity in the patient is approximately 70% of normal (Table 1 and Figure 1). It should be noted here that the post-heparin LPL activity in the patient was, in fact, a reflection of the net result of the complex interaction between the LPL gene and its regulating genes (such as APOC2, APOA5, GPIHBP1, and LMF1) as well as environmental and lifestyle factors. Thus, to decipher the direct effect of the p.Ile252Met variant on LPL protein and function, we further performed an in vitro transfection study, which demonstrated that the mutant p.Ile252Met allele caused a 40% loss of enzyme activity as compared to the wild-type allele (Figure 6). Based upon these observations, we believe that it is reasonable to define the LPL p.Ile252Met variant as a rare genetic variant that confers a rather moderate effect on LPL function (and presumably on HTG). Taken together, the patient was determined to have a digenic basis for his previous mild HTG and the current severe HTG-AP. To the best of our knowledge, this is the first time that trans heterozygosity for a common variant and a rare variant, both of which have a moderate effect on HTG, has been described in this disease. In this regard, it is pertinent to mention that in the context of TG-related genes, common variants are generally thought to have a small genetic effect whereas rare variants are thought to have a strong genetic effect (Johansen and Hegele, 2011; Dron and Hegele, 2020). Additionally, it should also be appreciated that the APOA5 p.Gly185Cys variant appeared to amplify the effect of the LPL p.Ile252Met variant on reducing LPL activity, as the reduction of the plasma LPL activity in the patient (30% lower than normal) was 10% higher than the reduction of the LPL activity conferred by the “heterozygous” mutant LPL variant in vitro (20% lower than wild-type). Although care should always be exercised when comparing in vivo data directly with in vitro data, such a difference has a strong biological basis. The APOA5 p.Gly185Cys impairs the function of APOA5; since APOA5 is an activator of LPL, this loss-of-function leads in turn to less activated LPL (Dorfmeister et al., 2008; Huang et al., 2012; Sharma et al., 2014; Chang et al., 2018).
Second, alcohol abuse is the most important environmental factor causing HTG owing to its impact on very-low-density lipoprotein secretion, lipolysis and free fatty acid fluxes from adipose tissue to the liver (Van de Wiel, 2012; Klop et al., 2013; Zemánková et al., 2015). Herein, having performed long-term follow-up of the TG levels in the patient, we were able to report a previously undescribed scenario: whereby the consumption of 150 g alcohol apparently triggered the attack of severe HTG-AP, the daily consumption of ∼100 g alcohol over 20 y is likely to have contributed to his pre-existing mild-HTG. This latter postulate was based on the observation that the TG levels in the patient remained normal, or at most borderline high, during the nearly 3-year follow-up period, during which the patient had completely abstained from drinking alcohol. Further follow-up studies are, however, needed to draw a firm conclusion.
Third, most of the HTG-associated rare LPL missense variants reported to date result in a complete or >90% functional loss of the affected allele, with this functional loss often being linked to upstream effects on protein synthesis, transport and secretion rather than to an isolated effect on enzyme activity (Rouis et al., 1996; Murano et al., 2005; Yu et al., 2006; Liu et al., 2016; Shi et al., 2020). To date, we are aware of only one rare LPL missense variant in the literature (i.e., a homozygous p.Ala203Thr variant found in a LPL-deficient patient) that specifically disrupted enzyme activity (Beg et al., 1990). However, both in vivo and in vitro findings (Beg et al., 1990) pointed to the p.Ala203Thr variant experiencing a complete loss of enzymatic activity. By contrast, the LPL p.Ile252Met mutant currently described here retained 60% enzyme activity as compared to its wild-type counterpart. Interestingly, p.Ile252Met occurred within a region of LPL, encompassing amino acid positions 243 to 266, that plays a crucial role in determining lipase substrate specificity (Dugi et al., 1995).
Lastly, we observed that whereas LPL mass was significantly increased in the patient during the acute phase of HTG-AP, LPL activity remained essentially unchanged at 70% normal (Figure 1). We surmised that this may be due to a dominant-negative effect of the mutant LPL p.Ile252Met allele, given that LPL is active mainly as a homodimer (Griffon et al., 2009; Arora et al., 2019; Beigneux et al., 2019). To explore this postulate, we mimicked the heterozygous state of the variant by mixing an equal amount of wild-type and mutant expression vectors in the transfection experiments. As shown in Figure 6, the impact of the “homozygous” variant was approximately double that of the “heterozygous” variant in terms of reducing LPL activity, thereby excluding such a postulate. It might also be that under stress of severe HTG, a positive feedback cycle stimulated LPL synthesis. However, a significant fraction of rapidly synthesized LPL proteins may not fold correctly and hence might not function normally. In this regard, it did not escape our attention that in the aforementioned LPL-deficient patient carrying the LPL p.Ala203Thr variant, higher than normal LPL mass concentrations were present in both pre- and post-heparin samples (Beg et al., 1990). It should, however, be noted that no information was available as to whether or not the patient had AP when the analysis was performed. Moreover, unlike our patient who had retained 70% normal LPL activity, the homozygous p.Ala203Thr carrier had no LPL activity at all. Consequently, we surmise that in this latter patient, the increased LPL mass may more likely be due to a feedback stimulus resulting from the absence of LPL activity. Irrespective of the underlying reasons, the increased LPL mass in both patients is consistent with the in vitro evidence that the respective LPL missense variants did not affect either protein synthesis or secretion.
The above notwithstanding, it should be emphasized that this study focused on a single case with HTG-AP. Further studies of more cases are required for us to obtain a better understanding of the genotype-phenotype relationships underlying this rare entity. Moreover, although loss-of-function APOA5 variants have previously been shown to lead to less activated LPL by several studies (Dorfmeister et al., 2008; Huang et al., 2012; Sharma et al., 2014; Chang et al., 2018), co-transfection experiments of APOA5 p.Gly185Cys and LPL p.Ile252Met expression vectors would be expected to yield confirmatory evidence for the gene-gene interaction between the two variants.
We provide concrete evidence that gene-gene and gene-environment interactions are responsible for causing mild HTG and HTG-AP in this patient. The novel insights generated from this study not only improve our understanding of the complex etiology of HTG, but also have important implications for variant interpretation as well as disease prevention. For example, the study of the novel LPL p.Ile252Met variant clearly demonstrates that rare variants can confer a small or modest effect in the same way as common variants. Moreover, whereas the evaluation of evolutionary conservation and in silico pathogenicity prediction are both helpful in assessing the pathogenic relevance of missense variants, the precise underlying biological mechanisms can only be addressed by in vitro functional analysis. Finally, subjects found to carry any genetic risk factors for HTG should be well advised to be abstemious with regard to their alcohol consumption.
The original contributions presented in the study are included in the article/supplementary material, further inquiries can be directed to the corresponding author/s.
The studies involving human participants were reviewed and approved by the Ethics Committee of Jinling Hospital. The patients/participants provided their written informed consent to participate in this study. Written informed consent was obtained from the individual(s) for the publication of any potentially identifiable images or data included in this article.
QY: conceptualization, methodology, project administration, and writing-original draft. NP: data curation, investigation, methodology, and writing-original draft. X-YL: investigation, methodology, and software. X-LS and W-WC: validation and visualization. G-FZ: software and visualization. Y-PH: investigation and software. JZ: data curation and funding acquisition. F-XC: project administration and validation. B-QL: project administration and resources. Z-HT: formal analysis and funding acquisition. CF: supervision and writing – review and editing. DC: formal analysis and writing – review and editing. J-MC: conceptualization, formal analysis, supervision, writing-original draft, and writing – review and editing. W-QL: funding acquisition, project administration, and resources. All authors contributed to revision of the manuscript and approved the final manuscript.
This study was supported by the National Natural Science Foundation of China (Nos. 81670588, 81870441, and 81900592). The funding sources did not play any role in designing this study, sample collection, analyses, interpretation of the data, or in writing the manuscript. We have not been paid to write this article by any pharmaceutical companies or other agencies. The corresponding authors had full access to all data in the study and had final responsibility for the decision to submit for publication.
The authors declare that the research was conducted in the absence of any commercial or financial relationships that could be construed as a potential conflict of interest.
AGA (2007). AGA institute medical position statement on acute pancreatitis. Gastroenterology 132, 2019–2021. doi: 10.1053/j.gastro.2007.03.066
Arora, R., Nimonkar, A. V., Baird, D., Wang, C., Chiu, C. H., Horton, P. A., et al. (2019). Structure of lipoprotein lipase in complex with GPIHBP1. Proc. Natl. Acad. Sci. U.S.A. 116, 10360–10365. doi: 10.1073/pnas.1820171116
Banks, P. A., Bollen, T. L., Dervenis, C., Gooszen, H. G., Johnson, C. D., Sarr, M. G., et al. (2013). Classification of acute pancreatitis–2012: revision of the atlanta classification and definitions by international consensus. Gut 62, 102–111. doi: 10.1136/gutjnl-2012-302779
Beg, O. U., Meng, M. S., Skarlatos, S. I., Previato, L., Brunzell, J. D., and Brewer, H. B. Jr., et al. (1990). Lipoprotein lipaseBethesda: a single amino acid substitution (Ala-176>Thr) leads to abnormal heparin binding and loss of enzymic activity. Proc. Natl. Acad. Sci. U.S.A. 87, 3474–3478. doi: 10.1073/pnas.87.9.3474
Beigneux, A. P., Allan, C. M., Sandoval, N. P., Cho, G. W., Heizer, P. J., Jung, R. S., et al. (2019). Lipoprotein lipase is active as a monomer. Proc. Natl. Acad. Sci. U.S.A. 116, 6319–6328. doi: 10.1073/pnas.1900983116
Beigneux, A. P., Davies, B. S., Gin, P., Weinstein, M. M., Farber, E., Qiao, X., et al. (2007). Glycosylphosphatidylinositol-anchored high-density lipoprotein-binding protein 1 plays a critical role in the lipolytic processing of chylomicrons. Cell Metab. 5, 279–291. doi: 10.1016/j.cmet.2007.02.002
Black, D. M., and Sprecher, D. L. (1993). Dietary treatment and growth of hyperchylomicronemic children severely restricted in dietary fat. Am. J. Dis. Child 147, 60–62.
Breckenridge, W. C., Little, J. A., Steiner, G., Chow, A., and Poapst, M. (1978). Hypertriglyceridemia associated with deficiency of apolipoprotein C-II. N. Engl. J. Med. 298, 1265–1273. doi: 10.1056/nejm197806082982301
Chang, C. K., Lin, X. R., Lin, Y. L., Fang, W. H., Lin, S. W., Chang, S. Y., et al. (2018). Magnolol-mediated regulation of plasma triglyceride through affecting lipoprotein lipase activity in apolipoprotein A5 knock-in mice. PLoS One 13:e0192740. doi: 10.1371/journal.pone.0192740
Chen, W. W., Yang, Q., Li, X. Y., Shi, X. L., Pu, N., Lu, G. T., et al. (2019). Identification of a novel and heterozygous LMF1 nonsense mutation in an acute pancreatitis patient with severe hypertriglyceridemia, severe obesity and heavy smoking. Lipids Health Dis. 18, 68–72. doi: 10.1186/s12944-019-1012-9
Cole, C. B., Nikpay, M., and McPherson, R. (2015). Gene-environment interaction in dyslipidemia. Curr. Opin. Lipidol. 26, 133–138. doi: 10.1097/mol.0000000000000160
den Dunnen, J. T., Dalgleish, R., Maglott, D. R., Hart, R. K., Greenblatt, M. S., McGowan-Jordan, J., et al. (2016). HGVS Recommendations for the Description of Sequence Variants: 2016 Update. Hum. Mutat. 37, 564–569. doi: 10.1002/humu.22981
Dorfmeister, B., Zeng, W. W., Dichlberger, A., Nilsson, S. K., Schaap, F. G., Hubacek, J. A., et al. (2008). Effects of six APOA5 variants, identified in patients with severe hypertriglyceridemia, on in vitro lipoprotein lipase activity and receptor binding. Arterioscler Thromb. Vasc. Biol. 28, 1866–1871. doi: 10.1161/atvbaha.108.172866
Dron, J. S., and Hegele, R. A. (2020). Genetics of hypertriglyceridemia. Front. Endocrinol. (Lausanne) 11:455. doi: 10.3389/fendo.2020.00455
Dron, J. S., Wang, J., Cao, H., McIntyre, A. D., Iacocca, M. A., Menard, J. R., et al. (2019). Severe hypertriglyceridemia is primarily polygenic. J. Clin. Lipidol. 13, 80–88. doi: 10.1016/j.jacl.2018.10.006
Dugi, K. A., Dichek, H. L., and Santamarina-Fojo, S. (1995). Human hepatic and lipoprotein lipase: the loop covering the catalytic site mediates lipase substrate specificity. J. Biol. Chem. 270, 25396–25401. doi: 10.1074/jbc.270.43.25396
Griffon, N., Jin, W., Petty, T. J., Millar, J., Badellino, K. O., Saven, J. G., et al. (2009). Identification of the active form of endothelial lipase, a homodimer in a head-to-tail conformation. J. Biol. Chem. 284, 23322–23330. doi: 10.1074/jbc.M109.037002
Guéguen, P., Dupuis, A., Py, J. Y., Desprès, A., Masson, E., Le Marechal, C., et al. (2020). Pathogenic and likely pathogenic variants in at least five genes account for approximately 3% of mild isolated nonsyndromic thrombocytopenia. Transfusion 60, 2419–2431. doi: 10.1111/trf.15992
Han, P., Wei, G., Cai, K., Xiang, X., Deng, W. P., Li, Y. B., et al. (2020). Identification and functional characterization of mutations in LPL gene causing severe hypertriglyceridaemia and acute pancreatitis. J. Cell Mol. Med. 24, 1286–1299. doi: 10.1111/jcmm.14768
Hazra, N., and Gulliford, M. (2014). Evaluating pancreatitis in primary care: a population-based cohort study. Br. J. Gen. Pract. 64, e295–e301. doi: 10.3399/bjgp14X679732
He, H., Lei, L., Chen, E., Dong, J., Zhang, K., and Yang, J. (2016). The c.553G>T genetic variant of the APOA5 gene and altered triglyceride levels in the Asian population: a meta-analysis of case-control studies. Genet. Test Mol. Biomarkers 20, 758–765. doi: 10.1089/gtmb.2016.0047
Hegele, R. A., Ginsberg, H. N., Chapman, M. J., Nordestgaard, B. G., Kuivenhoven, J. A., Averna, M., et al. (2014). The polygenic nature of hypertriglyceridaemia: implications for definition, diagnosis, and management. Lancet Diabetes Endocrinol. 2, 655–666. doi: 10.1016/s2213-8587(13)70191-8
Huang, Y. J., Lin, Y. L., Chiang, C. I., Yen, C. T., Lin, S. W., and Kao, J. T. (2012). Functional importance of apolipoprotein A5 185G in the activation of lipoprotein lipase. Clin. Chim. Acta 413, 246–250. doi: 10.1016/j.cca.2011.09.045
IAP/APA (2013). IAP/APA evidence-based guidelines for the management of acute pancreatitis. Pancreatology 13, e1–e15. doi: 10.1016/j.pan.2013.07.063
Jin, M., Bai, X., Chen, X., Zhang, H., Lu, B., Li, Y., et al. (2019). A 16-year trend of etiology in acute pancreatitis: the increasing proportion of hypertriglyceridemia-associated acute pancreatitis and its adverse effect on prognosis. J. Clin. Lipidol. 13, 947e1–953e1. doi: 10.1016/j.jacl.2019.09.005
Johansen, C. T., and Hegele, R. A. (2011). Genetic bases of hypertriglyceridemic phenotypes. Curr. Opin. Lipidol. 22, 247–253. doi: 10.1097/MOL.0b013e3283471972
Kao, J. T., Wen, H. C., Chien, K. L., Hsu, H. C., and Lin, S. W. (2003). A novel genetic variant in the apolipoprotein A5 gene is associated with hypertriglyceridemia. Hum. Mol. Genet. 12, 2533–2539. doi: 10.1093/hmg/ddg255
Karczewski, K. J., Francioli, L. C., Tiao, G., Cummings, B. B., Alföldi, J., Wang, Q., et al. (2020). The mutational constraint spectrum quantified from variation in 141,456 humans. Nature 581, 434–443. doi: 10.1038/s41586-020-2308-7
Klop, B., do Rego, A. T., and Cabezas, M. C. (2013). Alcohol and plasma triglycerides. Curr. Opin. Lipidol. 24, 321–326. doi: 10.1097/MOL.0b013e3283606845
Kopanos, C., Tsiolkas, V., Kouris, A., Chapple, C. E., Albarca Aguilera, M., Meyer, R., et al. (2019). VarSome: the human genomic variant search engine. Bioinformatics 35, 1978–1980. doi: 10.1093/bioinformatics/bty897
Lee, P. J., and Papachristou, G. I. (2019). New insights into acute pancreatitis. Nat. Rev. Gastroenterol. Hepatol. 16, 479–496. doi: 10.1038/s41575-019-0158-2
Li, X., Ke, L., Dong, J., Ye, B., Meng, L., Mao, W., et al. (2018). Significantly different clinical features between hypertriglyceridemia and biliary acute pancreatitis: a retrospective study of 730 patients from a tertiary center. BMC Gastroenterol. 18:89. doi: 10.1186/s12876-018-0821-z
Li, X. Y., Pu, N., Chen, W. W., Shi, X. L., Zhang, G. F., Ke, L., et al. (2020). Identification of a novel LPL nonsense variant and further insights into the complex etiology and expression of hypertriglyceridemia-induced acute pancreatitis. Lipids Health Dis. 19, 63–70. doi: 10.1186/s12944-020-01249-z
Liu, Y., Lun, Y., Lv, W., Hou, X., and Wang, Y. (2016). A Chinese patient with recurrent pancreatitis during pregnancy induced by hypertriglyceridemia associated with compound heterozygosity (Glu242Lys and Leu252VaL) in the lipoprotein lipase gene. J. Clin. Lipidol. 10, 199.e–203.e. doi: 10.1016/j.jacl.2015.09.010
Manolio, T. A., Collins, F. S., Cox, N. J., Goldstein, D. B., Hindorff, L. A., Hunter, D. J., et al. (2009). Finding the missing heritability of complex diseases. Nature 461, 747–753. doi: 10.1038/nature08494
Merkel, M., Eckel, R. H., and Goldberg, I. J. (2002). Lipoprotein lipase: genetics, lipid uptake, and regulation. J. Lipid Res. 43, 1997–2006.
Murano, T., Sako, T., Oikawa, S., and Shirai, K. (2005). The recovery of dysfunctional lipoprotein lipase (Asp204-Glu) activity by modification of substrate. Atherosclerosis 183, 101–107. doi: 10.1016/j.atherosclerosis.2005.02.025
Nawawi, H. M., Chua, Y. A., and Watts, G. F. (2020). The brave new world of genetic testing in the management of the dyslipidaemias. Curr. Opin. Cardiol. 35, 226–233. doi: 10.1097/HCO.0000000000000721
Olivecrona, G. (2016). Role of lipoprotein lipase in lipid metabolism. Curr. Opin. Lipidol. 27, 233–241. doi: 10.1097/mol.0000000000000297
Pennacchio, L. A., Olivier, M., Hubacek, J. A., Cohen, J. C., Cox, D. R., Fruchart, J. C., et al. (2001). An apolipoprotein influencing triglycerides in humans and mice revealed by comparative sequencing. Science 294, 169–173. doi: 10.1126/science.1064852
Peterfy, M., Ben-Zeev, O., Mao, H. Z., Weissglas-Volkov, D., Aouizerat, B. E., Pullinger, C. R., et al. (2007). Mutations in LMF1 cause combined lipase deficiency and severe hypertriglyceridemia. Nat. Genet. 39, 1483–1487. doi: 10.1038/ng.2007.24
Pu, N., Yang, Q., Shi, X. L., Chen, W. W., Li, X. Y., Zhang, G. F., et al. (2020). Gene-environment interaction between APOA5 c.553G>T and pregnancy in hypertriglyceridemia-induced acute pancreatitis. J. Clin. Lipidol. 14, 498–506. doi: 10.1016/j.jacl.2020.05.003
Rouis, M., Lohse, P., Dugi, K. A., Lohse, P., Beg, O. U., Ronan, R., et al. (1996). Homozygosity for two point mutations in the lipoprotein lipase (LPL) gene in a patient with familial LPL deficiency: LPL(Asp9–>Asn. Tyr262–>His). J. Lipid Res. 37, 651–661.
Rygiel, K. (2018). Hypertriglyceridemia - common causes, prevention and treatment strategies. Curr. Cardiol. Rev. 14, 67–76. doi: 10.2174/1573403x14666180123165542
Sekimoto, M., Takada, T., Kawarada, Y., Hirata, K., Mayumi, T., Yoshida, M., et al. (2006). JPN Guidelines for the management of acute pancreatitis: epidemiology, etiology, natural history, and outcome predictors in acute pancreatitis. J. Hepatobiliary Pancreat Surg. 13, 10–24. doi: 10.1007/s00534-005-1047-3
Serveaux Dancer, M., Di Filippo, M., Marmontel, O., Valéro, R., Piombo Rivarola, M. D. C., Peretti, N., et al. (2018). New rare genetic variants of LMF1 gene identified in severe hypertriglyceridemia. J. Clin. Lipidol. 12, 1244–1252. doi: 10.1016/j.jacl.2018.06.018
Sharma, V., Witkowski, A., Witkowska, H. E., Dykstra, A., Simonsen, J. B., Nelbach, L., et al. (2014). Aberrant hetero-disulfide bond formation by the hypertriglyceridemia-associated p.Gly185Cys APOA5 variant (rs2075291). Arterioscler Thromb Vasc. Biol. 34, 2254–2260. doi: 10.1161/atvbaha.114.304027
Shi, X. L., Yang, Q., Pu, N., Li, X. Y., Chen, W. W., Zhou, J., et al. (2020). Identification and functional characterization of a novel heterozygous missense variant in the LPL associated with recurrent hypertriglyceridemia-induced acute pancreatitis in pregnancy. Mol. Genet. Genomic Med. 8:e1048. doi: 10.1002/mgg3.1048
Stenson, P. D., Mort, M., Ball, E. V., Chapman, M., Evans, K., Azevedo, L., et al. (2020). The Human Gene Mutation Database (HGMD(®)): optimizing its use in a clinical diagnostic or research setting. Hum. Genet. 139, 1197–1207. doi: 10.1007/s00439-020-02199-3
Tang, Y., Sun, P., Guo, D., Ferro, A., Ji, Y., Chen, Q., et al. (2006). A genetic variant c.553G > T in the apolipoprotein A5 gene is associated with an increased risk of coronary artery disease and altered triglyceride levels in a Chinese population. Atherosclerosis 185, 433–437. doi: 10.1016/j.atherosclerosis.2005.06.026
Van de Wiel, A. (2012). The effect of alcohol on postprandial and fasting triglycerides. Int. J. Vasc. Med. 2012:862504. doi: 10.1155/2012/862504
Wang, J., Ban, M. R., Zou, G. Y., Cao, H., Lin, T., Kennedy, B. A., et al. (2008). Polygenic determinants of severe hypertriglyceridemia. Hum. Mol. Genet. 17, 2894–2899. doi: 10.1093/hmg/ddn188
Yadav, D., and Lowenfels, A. B. (2013). The epidemiology of pancreatitis and pancreatic cancer. Gastroenterology 144, 1252–1261. doi: 10.1053/j.gastro.2013.01.068
Yin, G., Cang, X., Yu, G., Hu, G., Ni, J., Xiong, J., et al. (2015). Different clinical presentations of hyperlipidemic acute pancreatitis: a retrospective study. Pancreas 44, 1105–1110. doi: 10.1097/mpa.0000000000000403
Yu, X. H., Zhao, T. Q., Wang, L., Liu, Z. P., Zhang, C. M., Chen, R., et al. (2006). A novel substitution at the translation initiator codon (ATG–>ATC) of the lipoprotein lipase gene is mainly responsible for lipoprotein lipase deficiency in a patient with severe hypertriglyceridemia and recurrent pancreatitis. Biochem. Biophys. Res. Commun. 341, 82–87. doi: 10.1016/j.bbrc.2005.12.165
Yuan, G., Al-Shali, K. Z., and Hegele, R. A. (2007). Hypertriglyceridemia: its etiology, effects and treatment. Cmaj 176, 1113–1120. doi: 10.1503/cmaj.060963
Zafrir, B., Jubran, A., Hijazi, R., and Shapira, C. (2018). Clinical features and outcomes of severe, very severe, and extreme hypertriglyceridemia in a regional health service. J. Clin. Lipidol. 12, 928–936. doi: 10.1016/j.jacl.2018.03.086
Keywords: acute pancreatitis, alcohol-induced hypertriglyceridemia, LPL gene, APOA5 gene, gene-environment interaction
Citation: Yang Q, Pu N, Li X-Y, Shi X-L, Chen W-W, Zhang G-F, Hu Y-P, Zhou J, Chen F-X, Li B-Q, Tong Z-H, Férec C, Cooper DN, Chen J-M and Li W-Q (2021) Digenic Inheritance and Gene-Environment Interaction in a Patient With Hypertriglyceridemia and Acute Pancreatitis. Front. Genet. 12:640859. doi: 10.3389/fgene.2021.640859
Received: 06 January 2021; Accepted: 30 March 2021;
Published: 16 April 2021.
Edited by:
Fan Jin, Zhejiang University, ChinaReviewed by:
Ruizhi Liu, Jilin University, ChinaCopyright © 2021 Yang, Pu, Li, Shi, Chen, Zhang, Hu, Zhou, Chen, Li, Tong, Férec, Cooper, Chen and Li. This is an open-access article distributed under the terms of the Creative Commons Attribution License (CC BY). The use, distribution or reproduction in other forums is permitted, provided the original author(s) and the copyright owner(s) are credited and that the original publication in this journal is cited, in accordance with accepted academic practice. No use, distribution or reproduction is permitted which does not comply with these terms.
*Correspondence: Qi Yang, eWFuZ3FpX25qQDE2My5jb20=; Wei-Qin Li, bmp6eV9wYW5jcmVhQDE2My5jb20=
†These authors have contributed equally to this work
Disclaimer: All claims expressed in this article are solely those of the authors and do not necessarily represent those of their affiliated organizations, or those of the publisher, the editors and the reviewers. Any product that may be evaluated in this article or claim that may be made by its manufacturer is not guaranteed or endorsed by the publisher.
Research integrity at Frontiers
Learn more about the work of our research integrity team to safeguard the quality of each article we publish.