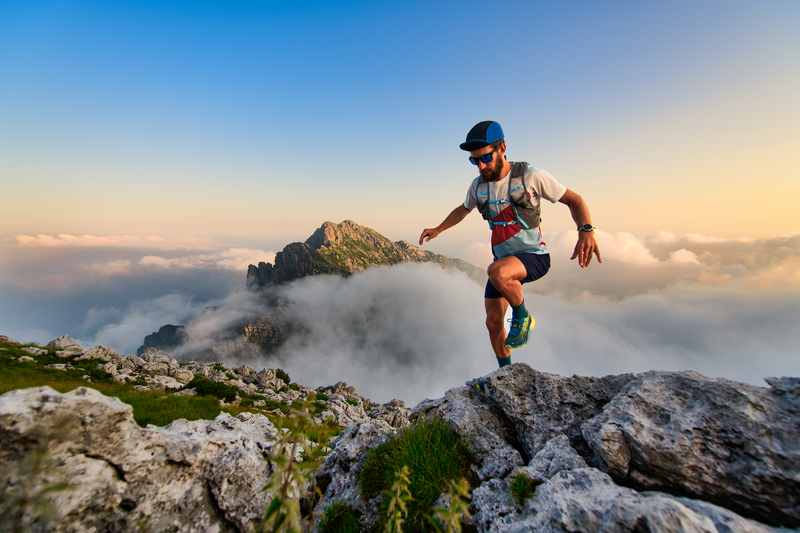
95% of researchers rate our articles as excellent or good
Learn more about the work of our research integrity team to safeguard the quality of each article we publish.
Find out more
ORIGINAL RESEARCH article
Front. Genet. , 05 August 2021
Sec. Genomics of Plants and the Phytoecosystem
Volume 12 - 2021 | https://doi.org/10.3389/fgene.2021.629267
This article is part of the Research Topic Genetics and Genomics to Enhance Crop Production, Towards Food Security View all 36 articles
Pisum sativum (pea) yields in the United States have declined significantly over the last decades, predominantly due to susceptibility to root rot diseases. One of the main causal agents of root rot is the fungus Fusarium solani f. sp. pisi (Fsp), leading to yield losses ranging from 15 to 60%. Determining and subsequently incorporating the genetic basis for resistance in new cultivars offers one of the best solutions to control this pathogen; however, no green-seeded pea cultivars with complete resistance to Fsp have been identified. To date, only partial levels of resistance to Fsp has been identified among pea genotypes. SNPs mined from Fsp-responsive differentially expressed genes (DEGs) identified in a preceding study were utilized to identify QTLs associated with Fsp resistance using composite interval mapping in two recombinant inbred line (RIL) populations segregating for partial root rot resistance. A total of 769 DEGs with single nucleotide polymorphisms (SNPs) were identified, and the putative SNPs were evaluated for being polymorphic across four partially resistant and four susceptible P. sativum genotypes. The SNPs with validated polymorphisms were used to screen two RIL populations using two phenotypic criteria: root disease severity and plant height. One QTL, WB.Fsp-Ps 5.1 that mapped to chromosome 5 explained 14.8% of the variance with a confidence interval of 10.4 cM. The other four QTLs located on chromosomes 2, 3, and 5, explained 5.3–8.1% of the variance. The use of SNPs derived from Fsp-responsive DEGs for QTL mapping proved to be an efficient way to identify molecular markers associated with Fsp resistance in pea. These QTLs are potential candidates for marker-assisted selection and gene pyramiding to obtain high levels of partial resistance in pea cultivars to combat root rot caused by Fsp.
Pea (Pisum sativum L.; Family Fabaceae) is an important cool-season, self-pollinating annual diploid crop. A number of cultivars within the species cater to different consumption markets. Green peas, and dry yellow and green peas are sold as food in the fresh and dry markets, respectively, while purple-seeded lines are used for forage and green manure (Miller et al., 2005). Due to its high protein content (20–30%) and overall high nutritional value, pea has become a major contributor to the plant-based protein market (do Carmo et al., 2016; Peng et al., 2016; Xiong et al., 2018; Wei et al., 2020). The shift to plant-based protein is an environmentally sustainable alternative to animal-based protein because the latter contributes significantly to greenhouse gas emissions (Stehfest et al., 2009). Furthermore, studies have shown that dietary proteins in peas are of great benefit to human health and wellness (Reddy and Yang, 2011; Kudre et al., 2013; Dahiya et al., 2015). Consequently, the pea protein market was projected to reach $313.5 million in 2025 (Grand View Research, 2017; Sim et al., 2019).
The profitable production of pea is threatened by soilborne diseases. These diseases are commonly referred to as the pea root rot complex (PRRC) and are caused by a single or combination of pathogens, including Aphanomyces euteiches, Fusarium spp., Mycosphaerella pinodes, Pythium spp., and Rhizoctonia solani (Xue, 2003; Kumari and Katoch, 2020). One of the predominant causal agents of PRRC is the fungus Fusarium solani f. sp. pisi (Fsp). Fsp occurs in most pea fields throughout the world, and the yields of P. sativum cultivars can be reduced up to 15–62% by this pathogen (Seaman, 1976; Grünwald et al., 2003). Fsp infects pea seeds during germination, with symptoms of root rot beginning at or near the cotyledon-hypocotyl junction and progressing under the soil and upper region of the taproot (JM, Kraft and Pfleger, 2006). Round or irregular light brown lesions that progress to dark black lesions on below-ground stems have also been reported, along with stunting and death (Jung et al., 1999). Fsp can survive in the soil for more than one season and conditions that decrease root growth, such as soil compaction, extreme temperatures, and moisture levels, can increase Fusarium-mediated root damage (Shaykh et al., 1977; JM, Kraft and Pfleger, 2006).
The development of pea cultivars with root rot resistance has been considered the best long-term management option among the many root rot control strategies (Conner et al., 2014; Bodah et al., 2016; Wang et al., 2018). However, breeding for Fsp resistance is challenging, since resistance to Fsp is a quantitative trait (Mukankusi et al., 2011; Román-Avilés et al., 2011; Bodah et al., 2016). Furthermore, routine screening for resistance has proven to be time-consuming, expensive, and highly influenced by the environment (Bodah et al., 2016). Marker-assisted selection (MAS) can help expedite the selection of putative Fsp resistant progeny without the need for expensive phenotyping. Several efforts have been made to develop molecular markers associated with resistance to Fsp root rot in pea (Feng et al., 2011; Coyne et al., 2015, 2019). However, these studies used a limited number of DNA markers and some of the QTLs identified require further fine mapping to provide informative markers due to large confidence intervals (16.8–28.5 cM).
In a preceding time-course transcriptome study, we utilized a combined genetic and RNAseq approach to identify Fsp-responsive differentially expressed genes among four partially resistant and four susceptible genotypes (Williamson-Benavides et al., 2020). These genotypes were selected for their contrasting root severity index phenotype (Bodah et al., 2016). Genes involved in secretion and exocytosis, anthocyanin biosynthesis pathway genes, and a previously-described pathogenesis-related (PR) gene DRR230 were observed to be overexpressed in partially resistant genotypes (Chiang and Hadwiger, 1991; Hadwiger, 2008, 2015; Williamson-Benavides et al., 2020). Since the use of single nucleotide polymorphisms (SNPs) can help to refine genetic mapping studies due to their high abundance in the genome (Deulvot et al., 2010), SNPs mined from differentially expressed genes (DEGs) were utilized to identify QTLs associated with Fsp resistance using composite interval mapping in two recombinant inbred line (RIL) populations segregating for root rot resistance as observed in greenhouse evaluations.
The parental plant material used in this study was the same as described in a preceding study (Williamson-Benavides et al., 2020). Briefly, four genotypes with partial resistance to Fsp—00-5001, 00-5003, 00-5004, and 00-5007— and four susceptible genotypes— “Aragorn,” “Banner,” “Bolero,” and “DSP”—were selected based on their disease resistance to Fsp (Table 1). These genotypes were previously classified as either partially resistant or susceptible based on phenotyping root disease severity index (RDS), plant height, shoot dry weight, and root dry weight after Fsp challenge (Bodah et al., 2016). The 5,000 series pea breeding lines were found to be the most resistant lines among the white-flowered pea lines. The susceptible genotypes are among the most frequently used commercial pea varieties in the United States (Table 1).
Table 1. Selected green-seeded pea genotypes for SNP genotyping [Adapted from Williamson-Benavides et al. (2020)].
The 00-5001, 00-5003, 00-5004, and 00-5007 pea breeding lines were developed by Porter et al. (2014) via single-seed descent at USDA–ARS, Prosser, WA. The parentage of 00-5001 is PH14-119/M7477// Coquette/3/86-2197/74-410-2 (Kraft, 1989; USDA–ARS NGRP, 2020). The parentage of 00-5003 is 69PH42-691004/Recette//Popet/3/PH14-119/DL-1/3/B563-429-2/PI257593//DSP TAC (USDA–ARS NGRP, 2020). The parentage of 00-5004 is 79-2022/ICI 1203-1//Menlo/3/PI189171/DL-2//75-786 (Kraft and Tuck, 1986; USDA–ARS NGRP, 2020). The parentage of 00-5007 is 00-5005/00-5006.00-5005 parentage is B669-87-0/M7477//Blixt B5119/3/00-5001/74SN5/3/PH14-119/DL-1//74SN3/Recette/5/FR-725 (Kraft and Giles, 1976; USDA–ARS NGRP, 2020). The parentage of 00-5006 is 00-5003/00-5004.
Two F7-derived recombinant inbred line (RIL) populations with 190 individuals each derived from the crosses “Aragorn” × 00-5001 (Population I) and “Banner” × 00-5007 (Population II) were developed by single-seed descent and maintained at ProGene LLC Plant Research, Othello, WA, United States.
For the two populations, a total of 190 individuals with four replicates each were challenged with three Fsp isolates: Fs 02, Fs 07, and Fs 09. These isolates were obtained from infected pea roots collected in the Palouse Region of Washington and Idaho by Dr. Lyndon Porter, USDA-ARS Vegetable and Forage Crops Research Unit, Prosser, WA (United States). The three isolates were single-spored and were identified based on the partial translation elongation factor 1-alpha sequences (Geiser et al., 2004). The pathogenicity of each Fsp isolate to pea was also confirmed (Bodah et al., 2016). The three isolates were grown on pentachloronitrobenzene (PCNB) selective media for six days (Nash and Snyder, 1962). Cultures were transferred to KERR’s media (Kerr, 1963) and incubated on a shaker at 120 rpm under continuous light for six days at 23 to 25°C. The spore concentration of each isolate was determined with a hemocytometer and diluted to 1 × 106 spores/ml of water. A spore suspension inoculum was created with equal parts by volume from each of the three isolates.
RIL seeds were surface sterilized in a 0.6% sodium hypochlorite solution and rinsed in sterile distilled H2O. The seeds were then soaked for 16 h in the Fsp spore suspension as described previously (Bodah et al., 2016). After the challenge with the spore suspension, seeds were planted in a completely randomized design in plastic planter cones (Conetainer, 0.25 L volume, Stuewe and Sons Inc.) filled with a standard perlite medium in a greenhouse at Crites Seed Inc. (Moscow, ID). Plants were irrigated as needed, generally every 24–36 h, and the perlite was watered to saturation at 100% field capacity. A 12-h photoperiod was maintained using 400-watt metal halide lamps for supplemental light. Plants were grown at temperatures ranging between 21–27°C during the day and 12–18°C at night.
Quantitative evaluation of RDS and plant height were recorded 21 days after planting. RDS was evaluated on a visual scale from 0 to 6, in which 0 = no diseases symptoms; 1 = small hypocotyl lesions; 2 = lesions coalescing around epicotyls and hypocotyls; 3 = lesions starting to spread into the root system with some root tips infected; 4 = epicotyl, hypocotyl and root system almost completely infected and limited white, uninfected tissue visible; 5 = completely infected root; and 6 = plant failed to emerge (Bodah et al., 2016). Plant height is a reliable indication of resistance to Fsp and height showed the highest negative correlation among all growth parameters related to RDS (Bodah et al., 2016). RDS and height data across the four replicates were averaged for each RIL for further analyses. Phenotyping was repeated twice. Infected root tissue from three inoculated plants was taken at random to verify the presence of Fsp in infected tissue. The root tissue was surface sterilized and plated onto PCNB. Culture morphology and growth were observed under a microscope and compared with the original cultures to verify the presence of Fsp in the infected tissue.
Broad-sense heritability was estimated with this equation Va/(Va + Ve), where Va represented the genetic variance, Ve the environmental variance.
Leaf tissue was freeze-dried in a lyophilizer. Leaf tissue samples included the eight white-flowered parental genotypes —00-5001, 00-5003, 00-5004, and 00-5007 “Aragorn,” “Banner,” “Bolero,” and “DSP,” as well as the 380 RILs from Population I and Population II. DNA was extracted with the BioSprint 96 DNA Plant kit (Qiagen, Mainz, Germany). A Nanodrop ND-8000 Spectrophotometer (ThermoFisher, MA, United States) was used to quantify the extracted DNA.
A time-course RNAseq analysis, performed on sets of partially resistant and susceptible genotypes after Fsp challenge resulted in the identification of 42,905 differentially expressed contigs (DECs) (Williamson-Benavides et al., 2020). SeqMan Pro (DNASTAR, WI, United States) and custom scripts were utilized to identify single nucleotide polymorphisms (SNPs) within the set of 42,905 DECs. The Assay Design Suite software (Agena Bioscience, CA, United States) and the SNP report generated by SeqMan Pro were used to generate two sets of primers for amplifying SNP containing regions (Supplementary Table 1). The high-throughput MassARRAY Technology was used to validate the SNPs. Genotype calling was done from the samples deposited on the chips with the MassARRAY RT v 3.0.0.4 software (Agena Bioscience, CA, United States). Results were analyzed with the MassARRAY Typer v 3.4 software (Agena Bioscience, CA, United States). SNPs were validated across eight pea genotypes “Aragorn,” “Banner,” “DSP,” “Bolero,” 00-5001, 00-5003, 00-5004, 00-5007, which included the four parents of the two segregating populations. Each SNP was screened twice for each individual. SNPs confirmed to be polymorphic between “Aragorn” × 00-5001, and “Banner” × 00-5007 were used -for genotyping of 190 RILs each from Population I and Population II.
The physical location of the SNPs used in this study was determined using the pea genome (Kreplak et al., 2019). The SNP marker sequence was aligned via BLAST against the complete P. sativum genome in URGI BLAST1. RDS and height averages for each RIL were used to map the QTLs associated with resistance to Fsp. QTLs were detected with the composite interval mapping (CIM) function of the R statistical software version 3.0.2 (R core team, Vienna, Austria). CIM default settings were used. The Kosambi map function was applied to impute missing marker genotype data. QTLs were considered significant above the threshold LOD score 3.0. QTLs were named with the prefix WB.Fsp-Ps followed by the chromosome number and the QTL number within the chromosome.
The QTLs WB.Fsp-Ps 5.1, WB.Fsp-Ps 5.2, WB.Fsp-Ps 5.3, WB.Fsp-Ps 2.1, and WB.Fsp-Ps 3.1 were annotated using the functional annotation and gene ontology (GO) data generated in a preceding study (Williamson-Benavides et al., 2020). QTL annotation provided the identity of genes and DECs located within the selected genomic regions. The confidence intervals for each of the QTLs were taken into account to identify the genomic sequence of each QTL from the pea genome (Kreplak et al., 2019). The transcriptome data were aligned via BLAST against the QTL sequence regions in CLC Bio Genomics Workbench 6.0.1 (CLC Bio, Aarhus, Denmark).
The quantitative evaluation of RDS and plant height was averaged per RIL across the four replicates as there was no significant difference between the replicates (p < 0.05). Frequency histograms for both traits per population are presented in Figure 1. The phenotypic means for the parents for Population I were Aragorn-RDS = 4.5; Aragorn-Height = 7.0; 00-5001-RDS = 2.5; and 00-5001-Height = 10.5. The phenotypic means for the parents for Population II were Banner-RDS = 3.3; Banner-Height = 13.0; 00-5007-RDS = 2.3; and 00-5007-Height = 10.0. The two RIL populations displayed transgressive segregation for both increased susceptibility and resistance over the two parental lines as measured by RDS and height traits (Figure 1).
Figure 1. Frequency histograms of root disease severity (RDS) and plant height of recombinant lines (RILs) after challenge with Fusarium solani f. sp. pisi. RILs were derived from crosses “Aragorn” × 00-5001 (A,B) and “Banner” × 00-5007 (C,D).
Based on the Shapiro–Wilk test, in Population I, data were not normally distributed for RDS [W(189) = 0.95, p < 0.01] or for height [W(189) = 0.91, p < 0.01]. Similarly in Population II, data were not normally distributed for RDS [W(189) = 0.90, p < 0.01] or for height [W(189) = 0.97, p < 0.01]. A significant negative correlation was found between the RDS and height values for Population I [r(188) = −2.76, p < 0.01] and Population II [r(188) = −4.38, p < 0.01]. For Population I, broad sense heritability was 49.8 and 70.5% for RDS and height, respectively. For Population II, broad sense heritability was 43.1 and 83.4% for RDS and height, respectively.
SeqMan Pro (DNASTAR, WI, United States) identified a total of 769 SNPs across DECs (Figure 2A and Supplementary Table 2). The predicted SNPs were validated in the “Aragorn,” “Banner,” “DSP,” “Bolero,” 00-5001, 00-5003, 00-5004, and 00-5007 pea genotypes (Supplementary Table 3). A total of 118 SNPs were confirmed for cultivars DSP and Bolero while 256 SNPs were confirmed for 5007 and Banner (Table 2). SNPs confirmed to be polymorphic between “Aragorn” × 00-5001 (219 SNPs) (Figure 2B) and “Banner” × 00-5007 (256 SNPs) (Figure 2C) were used to screen 190 individuals each of RIL Populations I and II, respectively. The screening results of 190 individuals each for both Population I and II are summarized in Supplementary Tables 4, 5, respectively.
Figure 2. Physical maps of SNPs and QTLs identified in the seven Pisum sativum chromosomes. Location of 769 SNPs, mined from Fsp-responsive differentially expressed genes (DEGs), in pea genome (A). Total of 100 and 154 SNPs with validated polymorphisms for population I (“Aragorn” × 00-5001) (B) and population II (“Banner” × 00-5007) (C), respectively. Five QTLs were identified in association with disease resistance (D). Major QTL Fsp-Ps 2.1 (Coyne et al., 2019) was added as a reference (D). Physical distances, represented in base pairs (bp), are shown on the left side of the graphs.
The physical genomic location of all the SNPs used in this study was determined using the pea genome (Kreplak et al., 2019; Figure 2 and Supplementary Table 6). Chromosome 1, 2, 3, 4, 5, 6, and 7 registered a total of 92, 86, 84, 122, 118, 78, 139 SNPs, respectively (Figure 2 and Supplementary Table 6). A total of 47 SNP markers were identified in 42 scaffolds that had not been assigned to any of the seven chromosomes of pea (Supplementary Table 6). Three of the predicted SNP markers were not localized on the pea genome.
Prior to QTL mapping for Population I and II, a quality assessment of the genotypic data was performed. Individuals and markers with more than 80% of missing data were omitted in each database. Markers with distorted segregation patterns were also removed from the data for QTL analysis. A total of 190 RILs and 100 markers were used for QTL analysis of Population I (Figure 2B and Supplementary Table 7). A total of 182 individuals and 154 markers were used for QTL analysis of Population II (Figure 2C and Supplementary Table 8). Means per RIL for RDS and height were used to map the QTLs associated with resistance to Fsp. Five different QTLs were identified in the two RIL populations for RDS and height (Figure 2D and Table 3). These QTLs explained 5.3 to 14.8% of the phenotypic variance (Table 3).
Table 3. Quantitative trait loci detected for resistance to Fusarium solani f. sp. pisi root rot in two RIL populations using root disease severity (RDS) and height.
The transcriptome data, generated previously (Williamson-Benavides et al., 2020) were aligned via BLAST with the 5 QTLs: WB-Fsp-Ps 5.1 (Supplementary Table 9), WB-Fsp-Ps 5.2 (Supplementary Table 10), WB-Fsp-Ps 5.3 (Supplementary Table 11), WB-Fsp-Ps 2.1 (Supplementary Table 12), and WB-Fsp-Ps 3.1 (Supplementary Table 13). From the total set of aligned genes, 119–133 genes per QTL had previously been classified as differentially expressed (Supplementary Tables 9–13). A total of 3–17 DEGs and 6–11 non-DEGs were also identified, predicted as having unknown function or annotated as hypothetical proteins in QTLs WB-Fsp-Ps 5.1, 5.2, 5.3, 2.1, and 3.1 (Supplementary Tables 9–13).
A total of 7 DEGs associated with disease response were found in WB-Fsp-Ps 5.1. These genes are involved in the synthesis of lipids (acetyl-CoA carboxylase); cell signaling (C-type lectin receptor-like tyrosine-protein kinase and MAPK); nodulation (nodulation-signaling pathway 2 protein), and protein degradation (F-box/kelch-repeat). Another ten genes in WB-Fsp-Ps 5.1 were associated with disease resistance; however, these genes did not exhibit differential expression (Williamson-Benavides et al., 2020). These set of genes is associated with synthesis of lipids (1 gene); auxin signaling (2), ethylene synthesis (1), pectin synthesis (1), and regulation of transcription (5).
Seventeen genes found in WB-Fsp-Ps 5.2 were associated with disease response and also showed differential expression after Fsp challenge. This list included three PR (pathogenesis-related) genes (universal stress protein PHOS32-like, endochitinase PR4, protein enhanced disease resistance 2); an anthocyanin 5-aromatic acyltransferase; four receptor-like kinases; and seven TFs of the GATA, NLP8, C2H2, and scarecrow types. Another set of seventeen contigs were identified as candidate genes but did not show any differential expression. The genes on the latter list are associated with synthesis of lipids (ketoacyl-CoA synthase); three transcription factors (GLABRA and PosF21 type); an endochitinase PR4; four receptor like-kinases; and two universal stress proteins PHOS32.
Twenty-two DEGs in WB-Fsp-Ps 5.3 were associated with disease response mechanism. This list included drug transporters (ABC transporters); a cluster of seven F-box proteins; genes involved in cell wall biosynthesis and modification (pectinesterase/pectinesterase inhibitor and polygalacturonase); the TFIIS TF; and two PR proteins—protein enhanced disease resistance 4-like and pathogenic type III effector avirulence factor. Three more ABC transporters were found in the WB-Fsp-Ps 5.3, but they did not show any differential expression. Other candidate genes found in WB-Fsp-Ps 5.3 that did not show differential expression included an autophagy-related protein; a brassinosteroid receptor; another F-box gene; five more receptor kinases; a protein enhanced disease resistance 4-like and pathogenic type III effector avirulence factor; two TFs [CCHC(Zn) family and ERF110]; and a UDP-glucuronate:xylan alpha-glucuronosyltransferase 1.
Fourteen DEGs involved in disease resistance mechanism were associated with WB-Fsp-Ps 2.1. These genes are known to participate in cell membrane synthesis and modification (CSC1 protein and sphingolipid transporter); PR gene response (disease resistance protein RPM1 and disease resistance protein RGA3); regulation of transcription (Myb/SANT and ninja-family protein AFP3); and cell signaling (receptor-like protein kinase 2). Three genes involved in cell wall and membrane synthesis/modification (glycerol-3-phosphate acyltransferase, sphingolipid transporter, and 3UDP-arabinopyranose mutase); and nine TFs (Myb/SANT, ninja-family protein AFP3, PLATZ transcription factor family protein) were also identified as potential candidates that contribute to the effect of WB-Fsp-Ps 2.1. However, this set of genes did not show differential expression after Fsp challenge.
Eleven DEGs associated with disease response were found within WB-Fsp-Ps 3.1. These genes were annotated as ethylene response sensors; polygalacturonase inhibitors; phopholipases; receptor kinases; and NDR1/HIN1-like protein 10. A set of fourteen genes were characterized as associated with disease response, however they did not show differential expression. This list contains cathepsin B-like protease 2; ethylene-insensitive protein 2; F-box protein PP2-A15 isoform X2; mannan synthase 1-like isoform X1; polygalacturonase inhibitor; nuclear transcription factor Y subunit B-10; and serine/threonine protein receptor genes.
Here, we have reported the identification of five QTLs that are associated with Fsp resistance in pea (Figure 2D and Table 3). Each of these QTLs explains 5.3–14.8% of the total phenotypic variation, and together they add up to 33.21% of the variation. These five QTLs were identified by using polymorphisms embedded in Fsp-responsive DEGs. These polymorphisms and DEGs were originally identified via RNAseq. The identification of DEGs that respond to or are associated with specific biotic or abiotic stimulus, as well as the development of markers embedded in these DEGs is an efficient alternative to genotyping by sequencing for fine mapping.
To the best of our knowledge, this is the only study to report the presence of QTLs associated with Fsp resistance on chromosomes 2 and 3 of pea (Figure 2D and Table 3). A total of three QTLs were identified on chromosome 5. Previously, three QTLs associated with Fsp resistance, Fsp-Ps 3.1, 3.2 and 3.3, had been reported on chromosome 5 (Coyne et al., 2015, 2019). QTLs Fsp-Ps 3.2 and Fsp-Ps 3.3 are located close to each other and adjacent to the newly identified WB-Fsp-Ps 5.3. Further fine mapping should be able to determine if these three QTLs are in fact three, two, or only one QTL. The situation is similar in the case of QTLs WB-Fsp-Ps 5.1 (Figure 2D and Table 3) and Fsp-Ps 3.1 (Coyne et al., 2015). LOD intervals from these two QTLs do not overlap either, although they are located close to one another on chromosome 5. This proximity can mean that they represent one QTL.
Interestingly, this study did not find any QTLs on chromosome 6. Fsp-Ps 2.1 (Coyne et al., 2019; Figure 2B) and a QTL identified by Feng et al. (2011) located on chromosome 6 explained 44.4–53.4 and 39% of the phenotypic variance, respectively. These two remain the major QTLs identified so far for Fsp resistance in pea. The absence of a major QTL on chromosome 6 in this study could be due to the diversity of the parental source of resistance used in this study (005001 and 00-5007), versus what was used in previous studies (PI 180693, PI 557501, “Carman”) (Feng et al., 2011; Coyne et al., 2015, 2019). The large effect shown by Fsp-Ps 2.1, seen in previous reports, may explain the bimodal distribution for traits such as the root severity index, plant height, and plant weight (Coyne et al., 2019). However, data presented in this study did not show a bimodal distribution but showed a trend toward normal distribution, which might explain the absence of QTLs with large effects.
The establishment of associations between disease-related genes and resistance, or susceptibility can facilitate the understanding of the possible mechanism(s) involved in the pathogenicity of Fsp in pea. WB-Fsp-Ps 5.1 was the major QTL identified in this study. Among the DEGs identified in this QTL, an F-box/kelch-repeat protein (DN2516_c0_g1_i1) demonstrated reduced expression at 12 h (FC = −24.4) after Fsp challenge in the partially resistant, but not in the susceptible genotypes. Nine F-box protein–coding genes have been found in the region of a highly dominant QTL that provides resistance to A. euteiches, a root rot pathogen in pea (Djébali et al., 2009; Pilet-Nayel et al., 2009). F-box proteins are known to be involved in hormone regulation and in plant immunity (Guo and Ecker, 2003; Lechner et al., 2006). A nodulation signaling gene found in WB-Fsp-Ps 5.1 was also found to be upregulated at 0 h (FC = 2.4) in susceptible genotypes when the expression levels were compared against the expression levels in partially resistant genotypes. It has been reported that a central regulator of symbiotic nodule development is determinant of susceptibility toward A. euteiches in Medicago truncatula (Rey et al., 2013).
Several genes associated with disease resistance were located in WB-Fsp-Ps 5.2 QTL region (Supplementary Table 10). Contigs DN19556_c0_g1_i1, DN2007_c0_g1_i4, DN77_c0_g1_i6 were identified as anthocyanin 5-aromatic acyltransferase, endochitinase PR4, and protein enhanced disease resistance 2. These genes showed differential expression; their expression was significantly higher in the susceptible genotypes compared to the partially resistant genotypes (Williamson-Benavides et al., 2020). The same pattern was observed for membrane receptors in all five QTLs; TFs present in WB-Fsp-Ps 5.2; NDR1/HIN1-like protein 10 and polygalacturonase inhibitors found in WB-Fsp-Ps 3.1; the RPM1-like and putative disease resistance protein RGA3 identified in WB-Fsp-Ps 2.1; as well as for a cluster of F-box proteins, a pathogenic type III effector avirulence factor, a pectinesterase/pectinesterase inhibitor, and a protein enhanced disease resistance 4-like gene found in WB-Fsp-Ps 5.3 (Supplementary Tables 9, 10,12). The high expression of any of these genes might be associated with disease susceptibility. However, further reverse genetics analyses will need to be performed to determine the dominant or recessive nature of these genes and QTL(s).
Cell death in the pea-Fsp interaction can help in the progression of Fsp infection due to the necrotrophic nature of the Fsp pathogen (Williamson-Benavides et al., 2020). The contig DN352_c0_g1_i17 located within WB-Fsp-Ps 5.3 was identified as CPR-5 protein which is known to negatively regulate the senescence and chlorotic lesions induced by pathogens when controlling programmed cell death (Bowling et al., 1997; Yoshida et al., 2002). This gene is highly suppressed in expression at 12 h (FC = −3.03) after Fsp challenge in the susceptible genotype, which might trigger cell death.
Gene DN813_c0_g1_i4, located in WB-Fsp-Ps 2.1, was highly overexpressed in the partially resistant genotype when compared against the expression values in the susceptible genotypes under controlled conditions at 6 (FC = −4.76) and 12 h (FC = −2.71) and under Fsp-inoculation at 0 (FC = −3.76) and 12 h (FC = −2.29). BLAST search of contig TRINITY_DN813_c0_g1_i4 identified it as Medicago truncatula 1-aminocyclopropane-1-carboxylate oxidase homolog 1 (XM_003627980) (e-value: 5e-165, percentage identity: 84.5%). The 1-aminocyclopropane-1-carboxylate oxidase enzyme is involved in the production of ethylene. Jasmonate-induced defense responses, the expected response to counter the presence of necrotrophic pathogens such as Fsp, are known to be associated with elevation of 1-aminocyclopropane-1-carboxylate oxidase and also to increase the activity of defense-related enzymes and subsequent control of disease incidence (Yu et al., 2011; Dixit et al., 2016). Another R gene located in WB-Fsp-Ps 2.1 is the putative disease resistance protein RGA3; however, differential expression was not observed for this gene (Williamson-Benavides et al., 2020).
We identified transgressive segregation in the two populations in study. Several of the transgressive lines showed enhanced resistance. For instance, a total of 29 and 5 RILs are more resistant than 00-5001 and 00-5007, respectively, based on their RDS scores. Genotypes 00-5001 and 00-5007 were previously characterized as high yielding varieties with important agronomics such as higher resistance to Fsp, resistance to Fusarium wilt, semi-leafless leaf type, and anti-lodging characteristics. Therefore, the transgressive lines with enhanced resistance might serve as potential candidate cultivars with good agronomics and even higher resistance to Fsp. Yields of these transgressive lines can be compared to elite cultivars under controlled and root rot conditions.
The use of polymorphic DEGs for QTL mapping resulted in the identification of a new major QTL WB.Fsp-Ps 5.1 as well as other four minor QTLs. This outcome indicates that a combined gene expression and genetics approach is effective in identifying genomic regions that may otherwise remain undetected especially for quantitative traits. Chromosome 5 is a source of several QTLs associated with Fsp resistance. Some of these QTLs on chromosome 5 are closely located to each other, which is a sign of resistance islands. Clustered resistance genes within the same genetic locus (resistance islands) can be transferred en bloc to new pea varieties through breeding. Selection toward these newly identified QTLs, along with previously identified QTLs, should allow for rapid improvement of resistance to Fsp root rot in the commercial pea genotypes. Furthermore, candidate genes, nested in each QTL will be instrumental in furthering our understanding of Fsp-pea interactions.
The raw sequencing data used for RNAseq analysis and identification of polymorphic regions is publicly available from the NCBI Sequence Read Archive (SRA, https://www.ncbi.nlm.nih.gov/sra) under the accession number PRJNA630497.
AD, EB, RS, and BW-B designed the study. BW-B, GN, and EB performed the experiments and generated the data. BW-B analyzed the data. LP provided the tolerant pea genotypes and Fsp isolates. AD supervised the study. All authors read and approved the final manuscript.
BW-B acknowledges graduate research assistantship support from Washington State University Graduate School. Work in the Dhingra Laboratory was supported in part by Washington State University Agriculture Research Center Hatch Grant WNP00011 and United States Dry Pea and Lentil Commission. The authors acknowledge that this study was in part supported by funding from ProGene Plant Research. The funder was not involved in the study design, collection, analysis, interpretation of data, the writing of this article or the decision to submit it for publication.
The authors declare that the research was conducted in the absence of any commercial or financial relationships that could be construed as a potential conflict of interest.
All claims expressed in this article are solely those of the authors and do not necessarily represent those of their affiliated organizations, or those of the publisher, the editors and the reviewers. Any product that may be evaluated in this article, or claim that may be made by its manufacturer, is not guaranteed or endorsed by the publisher.
The authors would like to thank Kurt Braunwart, CEO, ProGene Plant Research for critical discussions and his support for the project. The authors would also like to thank Nnadozie Oraguzie for critical reading of the manuscript and their valuable suggestions. This manuscript has been submitted to bioRxiv as a preprint— doi: https://doi.org/10.1101/2020.11.13.382077 (Williamson-Benavides et al., 2021).
The Supplementary Material for this article can be found online at: https://www.frontiersin.org/articles/10.3389/fgene.2021.629267/full#supplementary-material
Bodah, E. T., Porter, L. D., Chaves, B., and Dhingra, A. (2016). Evaluation of pea accessions and commercial cultivars for fusarium root rot resistance. Euphytica 208, 63–72. doi: 10.1007/s10681-015-1545-6
Bowling, S. A., Clarke, J. D., Liu, Y., Klessig, D. F., and Dong, X. (1997). The cpr5 mutant of Arabidopsis expresses both NPR1-dependent and NPR1-independent resistance. Plant Cell 9, 1573–1584. doi: 10.2307/3870444
Chiang, C. C., and Hadwiger, L. A. (1991). The Fusarium solani-induced expression of a pea gene family encoding high cysteine content proteins. Mol. Plant Microbe Interact. 4, 324–331. doi: 10.1094/mpmi-4-324
Conner, R. L., Hou, A., Balasubramanian, P., McLaren, D. L., Henriquez, M. A., Chang, K.-F., et al. (2014). Reaction of dry bean cultivars grown in western Canada to root rot inoculation. Can. J. Plant Sci. 94, 1219–1230. doi: 10.4141/cjps2013-416
Coyne, C. J., Pilet-Nayel, M., McGee, R. J., Porter, L. D., Smýkal, P., and Grünwald, N. J. (2015). Identification of QTL controlling high levels of partial resistance to Fusarium solani f. sp. pisi in pea. Plant Breed. 134, 446–453.
Coyne, C. J., Porter, L. D., Boutet, G., Ma, Y., McGee, R. J., Lesné, A., et al. (2019). Confirmation of Fusarium root rot resistance QTL Fsp-Ps 2.1 of pea under controlled conditions. BMC Plant Biol. 19:98. doi: 10.1186/s12870-019-1699-9
Dahiya, P. K., Linnemann, A. R., Van Boekel, M., Khetarpaul, N., Grewal, R. B., and Nout, M. J. R. (2015). Mung bean: technological and nutritional potential. Crit. Rev. Food Sci. Nutr. 55, 670–688. doi: 10.1080/10408398.2012.671202
Deulvot, C., Charrel, H., Marty, A., Jacquin, F., Donnadieu, C., Lejeune-Hénaut, I., et al. (2010). Highly-multiplexed SNP genotyping for genetic mapping and germplasm diversity studies in pea. BMC Genomics 11:468. doi: 10.1186/1471-2164-11-468
Dixit, R., Agrawal, L., Gupta, S., Kumar, M., Yadav, S., Chauhan, P. S., et al. (2016). Southern blight disease of tomato control by 1-aminocyclopropane-1-carboxylate (ACC) deaminase producing Paenibacillus lentimorbus B-30488. Plant Signal. Behav. 11:e1113363. doi: 10.1080/15592324.2015.1113363
Djébali, N., Jauneau, A., Ameline-Torregrosa, C., Chardon, F., Jaulneau, V., Mathé, C., et al. (2009). Partial resistance of Medicago truncatula to Aphanomyces euteiches is associated with protection of the root stele and is controlled by a major QTL rich in proteasome-related genes. Mol. Plant Microbe Interact. 22, 1043–1055. doi: 10.1094/mpmi-22-9-1043
do Carmo, C. S., Nunes, A. N., Silva, I., Maia, C., Poejo, J., Ferreira-Dias, S., et al. (2016). Formulation of pea protein for increased satiety and improved foaming properties. RSC Adv. 6, 6048–6057. doi: 10.1039/c5ra22452g
Feng, J., Hwang, R., Chang, K. F., Conner, R. L., Hwang, S. F., Strelkov, S. E., et al. (2011). Identification of microsatellite markers linked to quantitative trait loci controlling resistance to Fusarium root rot in field pea. Can. J. plant Sci. 91, 199–204. doi: 10.4141/cjps09176
Geiser, D. M., del Mar Jiménez-Gasco, M., Kang, S., Makalowska, I., Veeraraghavan, N., Ward, T. J., et al. (2004). FUSARIUM-ID v. 1.0: a DNA sequence database for identifying Fusarium. Eur. J. plant Pathol. 110, 473–479. doi: 10.1007/978-1-4020-2285-2_2
Grand View Research (2017). Pea Protein Mark. Size Worth $313.5 Million By 2025. Available online at: www.grandviewresearch.%0Acom/press-release/global-pea-proteins-market (accessed August 15, 2020).
Grünwald, N. J., Coffman, V. A., and Kraft, J. M. (2003). Sources of partial resistance to Fusarium root rot in the Pisum core collection. Plant Dis. 87, 1197–1200. doi: 10.1094/pdis.2003.87.10.1197
Guo, H., and Ecker, J. R. (2003). Plant responses to ethylene gas are mediated by SCFEBF1/EBF2-dependent proteolysis of EIN3 transcription factor. Cell 115, 667–677. doi: 10.1016/s0092-8674(03)00969-3
Hadwiger, L. A. (2008). Pea–Fusarium solani interactions contributions of a system toward understanding disease resistance. Phytopathology 98, 372–379.
Hadwiger, L. A. (2015). Anatomy of a nonhost disease resistance response of pea to Fusarium solani: PR gene elicitation via DNase, chitosan and chromatin alterations. Front. Plant Sci. 6:373. doi: 10.3389/fpls.2015.00373
Jung, Y.-S., Kim, Y.-T., Yoo, S.-J., and Kim, H.-G. (1999). Mycological characteristics of Fusarium solani f. sp. pisi isolated from pea, ginseng and soybean in Korea. Plant Pathol. J. 15, 44–47.
Kerr, A. (1963). The root rot-Fusarium wilt complex of peas. Aust. J. Biol. Sci. 16, 55–69. doi: 10.1071/bi9630055
Kraft, J. M. (1989). Registration of 86-638, 86-2197, 86-2231, and 86-2236 PEA Germplasms. Crop Sci. 29, 494–495. doi: 10.2135/cropsci1989.0011183x002900020068x
Kraft, J. M., and Giles, R. A. (1976). Registration of 74SN3, 74SN4, and 74SN5 PEA Germplasm1 (Reg. No. GP 15 to GP 17). Crop Sci. 16:126. doi: 10.2135/cropsci1976.0011183x001600010042x
Kraft, J. M., and Pfleger, F. L. (2006). Compendium of Pea Disease and Pests, 2nd Edn. Saint Paul, MN: APS Press.
Kraft, J. M., and Tuck, J. A. (1986). Registration of 75-786, 84-1638, and 84-1930 Pea Germplasms. Crop Sci. 26, 1262–1263. doi: 10.2135/cropsci1986.0011183x002600060053x
Kreplak, J., Madoui, M. A., Cápal, P., Novák, P., Labadie, K., Aubert, G., et al. (2019). A reference genome for pea provides insight into legume genome evolution. Nat. Genet. 51, 1411–1422. doi: 10.1038/s41588-019-0480-1
Kudre, T. G., Benjakul, S., and Kishimura, H. (2013). Comparative study on chemical compositions and properties of protein isolates from mung bean, black bean and bambara groundnut. J. Sci. Food Agric. 93, 2429–2436. doi: 10.1002/jsfa.6052
Kumari, N., and Katoch, S. (2020). “Wilt and root rot complex of important pulse crops: their detection and integrated management,” in Management of Fungal Pathogens in Pulses, eds B. Singh, G. Singh, K. Kumar, S. Nayak, and N. Srinivasa (Cham: Springer), 93–119. doi: 10.1007/978-3-030-35947-8_6
Lechner, E., Achard, P., Vansiri, A., Potuschak, T., and Genschik, P. (2006). F-box proteins everywhere. Curr. Opin. Plant Biol. 9, 631–638. doi: 10.1016/j.pbi.2006.09.003
Miller, P., McKay, K., Jones, C., Blodgett, S., Menalled, F., Riesselman, J., et al. (2005). Growing Dry Pea in Montana. Bozeman, MT: Montana State University Extension Service.
Mukankusi, C., Derera, J., Melis, R., Gibson, P. T., and Buruchara, R. (2011). Genetic analysis of resistance to Fusarium root rot in common bean. Euphytica 182:11. doi: 10.1007/s10681-011-0413-2
Nash, S. M., and Snyder, W. C. (1962). Quantitative estimations by plate counts of propagules of the bean root rot Fusarium in field soils. Phytopathology 52, 567–572.
Peng, W., Kong, X., Chen, Y., Zhang, C., Yang, Y., and Hua, Y. (2016). Effects of heat treatment on the emulsifying properties of pea proteins. Food Hydrocoll. 52, 301–310. doi: 10.1016/j.foodhyd.2015.06.025
Pilet-Nayel, M.-L., Prospéri, J.-M., Hamon, C., Lesne, A., Lecointe, R., Le Goff, I., et al. (2009). AER1, a major gene conferring resistance to Aphanomyces euteiches in Medicago truncatula. Phytopathology 99, 203–208. doi: 10.1094/phyto-99-2-0203
Porter, L. D., Kraft, J. M., and Grünwald, N. J. (2014). Release of pea germplasm with Fusarium resistance combined with desirable yield and anti-lodging traits. J. Plant Regist. 8, 191–194. doi: 10.3198/jpr2013.07.0041crg
Reddy, N., and Yang, Y. (2011). Potential of plant proteins for medical applications. Trends Biotechnol. 29, 490–498. doi: 10.1016/j.tibtech.2011.05.003
Rey, T., Nars, A., Bonhomme, M., Bottin, A., Huguet, S., Balzergue, S., et al. (2013). NFP, a L ys M protein controlling N od f actor perception, also intervenes in Medicago truncatula resistance to pathogens. New Phytol. 198, 875–886. doi: 10.1111/nph.12198
Román-Avilés, B., Lewis, J. M., Kelly, J. D., Alves-Santos, F. M., and Diez, J. J. (2011). “Fusarium genetic control: a long term strategy,” in Control Fusarium Diseases, eds F. Alves-Santos and J. Díez (Thiruvananthapuram: Research Signpost), 65–108.
Seaman, W. L. (1976). Yield loss conversion factors for fusarium root rot of peal. Can. Plant Dis. Surv. 56, 25–32.
Shaykh, M., Soliday, C., and Kolattukudy, P. E. (1977). Proof for the production of cutinase by Fusarium solani f. pisi during penetration into its host, Pisum sativum. Plant Physiol. 60, 170–172. doi: 10.1104/pp.60.1.170
Sim, S. Y. J., Karwe, M. V., and Moraru, C. I. (2019). High pressure structuring of pea protein concentrates. J. Food Process Eng. 42, e13261.
Stehfest, E., Bouwman, L., Van Vuuren, D. P., Den Elzen, M. G. J., Eickhout, B., and Kabat, P. (2009). Climate benefits of changing diet. Clim. Change 95, 83–102. doi: 10.1007/s10584-008-9534-6
USDA–ARS NGRP (2020). Germplasm Resources Information Network- (GRIN). Available online at: https://npgsweb.ars-grin.gov/gringlobal/search.aspx (accessed February 7, 2020).
Wang, W., Jacobs, J. L., Chilvers, M. I., Mukankusi, C. M., Kelly, J. D., and Cichy, K. A. (2018). QTL analysis of Fusarium root rot resistance in an Andean× middle American common bean RIL population. Crop Sci. 58, 1166–1180. doi: 10.2135/cropsci2017.10.0608
Wei, Y., Cai, Z., Wu, M., Guo, Y., Tao, R., Li, R., et al. (2020). Comparative studies on the stabilization of pea protein dispersions by using various polysaccharides. Food Hydrocoll. 98:105233. doi: 10.1016/j.foodhyd.2019.105233
Williamson-Benavides, B., Sharpe, R., Nelson, G., Bodah, E. T., Porter, L. D., and Dhingra, A. (2021). Identification of root rot tolerance QTLs in pea using Fusarium solani f. sp. pisi-responsive differentially expressed genes. bioRxiv [Preprint]. doi: 10.1101/2020.11.13.382077
Williamson-Benavides, B. A., Sharpe, R. M., Nelson, G., Bodah, E. T., Porter, L. D., and Dhingra, A. (2020). Identification of Fusarium solani f. sp. pisi (Fsp) responsive genes in Pisum sativum. Front. Genet. 11:950. doi: 10.3389/fgene.2020.00950
Xiong, T., Xiong, W., Ge, M., Xia, J., Li, B., and Chen, Y. (2018). Effect of high intensity ultrasound on structure and foaming properties of pea protein isolate. Food Res. Int. 109, 260–267. doi: 10.1016/j.foodres.2018.04.044
Xue, A. G. (2003). Biological control of pathogens causing root rot complex in field pea using Clonostachys rosea strain ACM941. Phytopathology 93, 329–335. doi: 10.1094/phyto.2003.93.3.329
Yoshida, S., Ito, M., Nishida, I., and Watanabe, A. (2002). Identification of a novel gene HYS1/CPR5 that has a repressive role in the induction of leaf senescence and pathogen-defence responses in Arabidopsis thaliana. Plant J. 29, 427–437. doi: 10.1046/j.0960-7412.2001.01228.x
Keywords: root rot, quantitative trait loci, SNP, molecular marker, RNAseq, Pisum sativum L., disease resistance
Citation: Williamson-Benavides BA, Sharpe RM, Nelson G, Bodah ET, Porter LD and Dhingra A (2021) Identification of Root Rot Resistance QTLs in Pea Using Fusarium solani f. sp. pisi-Responsive Differentially Expressed Genes. Front. Genet. 12:629267. doi: 10.3389/fgene.2021.629267
Received: 14 November 2020; Accepted: 06 July 2021;
Published: 05 August 2021.
Edited by:
Pinky Agarwal, National Institute of Plant Genome Research (NIPGR), IndiaReviewed by:
Ethan Andersen, Francis Marion University, United StatesCopyright © 2021 Williamson-Benavides, Sharpe, Nelson, Bodah, Porter and Dhingra. This is an open-access article distributed under the terms of the Creative Commons Attribution License (CC BY). The use, distribution or reproduction in other forums is permitted, provided the original author(s) and the copyright owner(s) are credited and that the original publication in this journal is cited, in accordance with accepted academic practice. No use, distribution or reproduction is permitted which does not comply with these terms.
*Correspondence: Amit Dhingra, YWRoaW5ncmFAd3N1LmVkdQ==
†Present address: Eliane T. Bodah, Pure Line Seeds, Inc., Warden, WA, United States
Disclaimer: All claims expressed in this article are solely those of the authors and do not necessarily represent those of their affiliated organizations, or those of the publisher, the editors and the reviewers. Any product that may be evaluated in this article or claim that may be made by its manufacturer is not guaranteed or endorsed by the publisher.
Research integrity at Frontiers
Learn more about the work of our research integrity team to safeguard the quality of each article we publish.