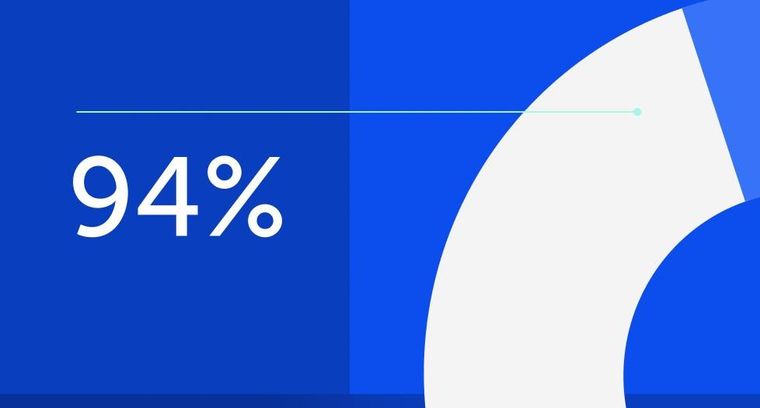
94% of researchers rate our articles as excellent or good
Learn more about the work of our research integrity team to safeguard the quality of each article we publish.
Find out more
REVIEW article
Front. Genet., 28 February 2020
Sec. Epigenomics and Epigenetics
Volume 11 - 2020 | https://doi.org/10.3389/fgene.2020.00095
This article is part of the Research TopicMitochondrial Genetics and EpigeneticsView all 13 articles
A correction has been applied to this article in:
Corrigendum: ncRNAs: New Players in Mitochondrial Health and Disease?
The regulation of mitochondrial proteome is unique in that its components have origins in both mitochondria and nucleus. With the development of OMICS technologies, emerging evidence indicates an interaction between mitochondria and nucleus based not only on the proteins but also on the non-coding RNAs (ncRNAs). It is now accepted that large parts of the non‐coding genome are transcribed into various ncRNA species. Although their characterization has been a hot topic in recent years, the function of the majority remains unknown. Recently, ncRNA species microRNA (miRNA) and long-non coding RNAs (lncRNA) have been gaining attention as direct or indirect modulators of the mitochondrial proteome homeostasis. These ncRNA can impact mitochondria indirectly by affecting transcripts encoding for mitochondrial proteins in the cytoplasm. Furthermore, reports of mitochondria-localized miRNAs, termed mitomiRs, and lncRNAs directly regulating mitochondrial gene expression suggest the import of RNA to mitochondria, but also transcription from the mitochondrial genome. Interestingly, ncRNAs have been also shown to hide small open reading frames (sORFs) encoding for small functional peptides termed micropeptides, with several examples reported with a role in mitochondria. In this review, we provide a literature overview on ncRNAs and micropeptides found to be associated with mitochondrial biology in the context of both health and disease. Although reported, small study overlap and rare replications by other groups make the presence, transport, and role of ncRNA in mitochondria an attractive, but still challenging subject. Finally, we touch the topic of their potential as prognosis markers and therapeutic targets.
Molecular biology has historically described RNA as an intermediate between genetic information stored in DNA and protein synthesis. The estimated number of protein-coding genes is around 20,000 (Pertea et al., 2018). Classical approaches to classify RNAs with protein-coding potential—the messenger RNAs (mRNAs)—were typically based on the existence of open reading frame (ORF) longer than 300 nucleotides (nt), conservation, and/or functional domains (Dinger et al., 2008). Nevertheless, as protein-coding regions encompass only ∼2% of the human genome, the rest has been considered as “dark matter”. Detected RNAs not translated into proteins were named non-coding RNAs (ncRNA) and initially regarded as a transcriptional noise or the byproducts of genetic information flow from DNA to protein. Nevertheless, since the discovery of transfer RNAs (tRNAs) and ribosomal RNAs (rRNAs), the number and understanding of new and putative functional ncRNAs have expanded. Moreover, the boundaries between the coding and non-coding RNAs have become more blurry. Evidence is emerging that some RNAs, initially classified as non-coding, hide small ORFs (sORFs, < 300 nt) encoding for small functional peptides- micropeptides. Currently, we know dozen of different ncRNAs, which can be can be classified as housekeeping or regulatory ncRNS, according to Szymanski et al. (2003).
Housekeeping ncRNAs are constitutively expressed and mostly well functionally characterized classes of rRNAs, tRNAs, small nuclear RNAs (snRNAs), small-nucleolar RNAs (snoRNAs), Ribonuclease P RNA (RNase P), Ribonuclease MRP RNA (MRP RNase, RNRP), and Telomerase RNA component (TERC). rRNAs are the most abundant class of RNAs in most cells, composing around 80% of cellular transcriptome. They serve as the essential binding site for ribosomal proteins within the assembled ribosome and contribute to the binding of extra-ribosomal factors and ribosome-associated proteins, resulting in the protein translation machinery (Noller et al., 2017; Simsek et al., 2017). tRNAs provide the interface between nucleic acids and proteins during translation by carrying an amino acid on its 3′ end and reading the mRNA by base-pairing induced by the ribosome, which uniquely determines the position of amino acids in proteins (Schimmel, 2018). snRNAs participate in the assembly and function of canonical spliceosomes (Wang and Burge, 2008). snoRNAs are localized to the nucleolus and guide the methylation and pseudouridylation of rRNAs, tRNAs, and snRNAs (Maxwell and Fournier, 1995). RNase P has a role in precursor-tRNA cleavage, RMRP in precursor-rRNA cleavage, and TERC in telomere synthesis (discussed later).
Regulatory ncRNAs are mostly produced in a cell- or tissue-specific fashion during certain stages of cell differentiation or organism development, or as a response to changes in the environment. They are still poorly understood and a very heterogeneous group that can act in different ways, from gene expression regulation to modulation of protein and RNA distribution within cells (Szymanski et al., 2003). They are divided based on their length into short (<200 nt) and long (>200 nt, lncRNAs) RNAs. Short ncRNAs consist of microRNAs (miRNAs), small interfering RNAs (siRNAs) and Piwi-associated RNAs (piRNAs). miRNAs are endogenous, single-stranded, 19-23 nt in length RNAs that can bind to a target mRNA with a complementary sequence to induce its cleavage, degradation, or interfere with translation. Similar in size, siRNAs are exogenous RNAs that undergo processing and function in post-transcriptional gene silencing (Carthew and Sontheimer, 2009). piRNAs are single stranded, 26-31 nucleotides long RNAs that form complexes with the piwi family of proteins. These complexes have a role in RNA and epigenetic silencing of transposons (Siomi et al., 2011). Longer than 200 nt, lncRNAs represent the most abundant, yet least understood class of RNAs, with an average length ~ 1000 nt (Ulitsky and Bartel, 2013). They share some features typical for mRNAs, such as transcription by the RNA-polymerase II (Pol II), 5′end cap, 3′end polyadenylation and presence of alternative splicing isoforms (Kopp and Mendell, 2018). However, compared to the mRNAs, they exhibit lower expression levels, more tissue-specific expression, and poor sequence conservation (Derrien et al., 2012; Djebali et al., 2012; Kopp and Mendell, 2018; Fazal et al., 2019). Although often considered as nucleus-enriched, lncRNAs exhibit variety of subcellular localization, which often helps to determine their biological function (Carlevaro-Fita and Johnson, 2019). Finally, circular RNAs (circRNAs) are a special class of RNAs with the 3′ and 5′ ends covalently linked, generally formed by alternative splicing of pre-mRNA (Salzman et al., 2012). They have been proposed to act as miRNAs sponges or even as templates for protein synthesis (Ragan et al., 2019).
Interest in the ncRNAs has been stimulated by the development of high-throughput OMICS technologies. Genome‐, transcriptome‐, translatome- and proteome‐wide measurements by the whole genome sequencing (WGS), RNA-sequencing (RNA-seq), ribosome profiling (Ribo-seq) and mass spectrometry (MS), respectively. In combination, these methods offer the possibility of a systematic analysis of different stages of gene expression (Ori et al., 2015; Wang et al., 2019). RNA-seq data have shown that up to 85% of the genome is transcribed and identified, among others, novel transcript isoforms, transcripts arising from intergenic regions, overlapping transcripts, and transcribed pseudogenes (Consortium, 2012; Djebali et al., 2012; Hangauer et al., 2013). Ribo-seq has shown widespread and pervasive translation on cytosolic RNAs, with surprisingly ~40% lncRNAs being engaged with the ribosome (Ingolia et al., 2009; Kearse and Wilusz, 2017). Reported ribosomal occupancy of RNAs indicated on the one side presence of different protein isoforms and regulatory upstream open reading frames ORFs (uORFs) from the mRNAs, and on the other, more exciting side, new ways of translational regulation and possible micropeptide production from lncRNAs (Morris and Geballe, 2000; Andrews and Rothnagel, 2014). It must be taken into account that the ribosomal occupancy of transcripts need not automatically lead to the production of stable, functional polypeptides, and that further evidence is needed in order to reclassify transcripts as indeed protein-coding (Guttman et al., 2013). MS has proven as a useful tool to inspect the postulated translational event, with developing proteogenomics approaches confirming the presence of some peptides encoded by previously non-coding regions (Slavoff et al., 2013; Fields et al., 2015; Wang et al., 2019). However, in order to omit the possibility of false-positive findings from MS, further functional studies on revealed peptides are needed, and these studies remain sparse.
The complexity of gene expression has in most cases been published on the levels of detection and its functional relevance remains elusive. Still, it has revealed that the distinguishment between mRNAs and ncRNAs is more challenging than initially assumed and that automatic gene annotation systems, although straightforward across large datasets, can sometimes be misleading. Traditional arbitrary ORF cutoff can lead to misclassification of some ncRNAs as mRNAs as they can by chance contain putative ORFs. This is especially true for lncRNAs, such as functionally characterized H19, Xist, Mirg, Gtl2, and KcnqOT1 (Prasanth and Spector, 2007). Some ncRNAs have evolved from the protein-coding genes, and so will keep certain features and homologies to mRNAs (Duret et al., 2006). For example, Xist has evolved into the ncRNA through the process of pseudogenization, during which proto-Xist had lost its protein-coding function and its flanking genes had turned into pseudogenes (Duret et al., 2006). On the contrary, micropeptide-encoding regions may be incorrectly classified as non-coding due to their size (Yeasmin et al., 2018). Next, the absence of ORF conservation does not guarantee an absence of protein-coding potential. Indeed, the majority of micropeptide-encoding regions are not conserved (Ji et al., 2015), suggesting their role in encoding evolutionary young proteins (Ruiz-Orera et al., 2014). Finally, some genes are bifunctional, and its products function independently both as RNAs and proteins. The first report of such a gene was the human Steroid Receptor Activator (SRA) (Lanz et al., 1999; Chooniedass-Kothari et al., 2004). SRA was initially characterized as ncRNA which co-activates steroid hormone receptors (Lanz et al., 1999) and later was revealed to also encode a functional protein (SRAP), which seems to modulate SRA activity (Chooniedass-Kothari et al., 2004).
Emerging discoveries in the ncRNA field have also raised the possibility that some ncRNAs affect mitochondrial biology. Mitochondria are crucial organelles for the integration of several key metabolic processes and the primary powerhouses in the cell (Spinelli and Haigis, 2018). The control of mitochondrial protein homeostasis is unique in that its components have origins in both mitochondria and nucleus (Figure 1). Mitochondria contain their own circular genome (mtDNA). In humans, it is 16,569 bp in length and contains 37 genes- encoding for 2 rRNAs, 22 tRNAs, and 13 proteins of the oxidative phosphorylation (OXPHOS) system (Anderson et al., 1981) (Figure 2). The rRNA coding sequences and all but one protein-coding sequences are separated by tRNAs and deprived of introns. The mtDNA is transcribed entirely from both strands, named heavy (H) or light (L). Transcription is initiated from the two H-strand (HSP1/2) and one L-strand promoter, located in the major non-coding region named “control region”, resulting in long polycistronic transcripts. LSP controls the transcription of eight tRNAs and the ND6 gene. HSP1 transcription produces a transcript containing tRNAPhe, tRNAVal, and the rRNAs, while transcription from HSP2 generates a transcript that spans almost the entire genome (Montoya et al., 1983; Chang and Clayton, 1984). The main proteins controlling the process are the RNA polymerase (POLRMT), two transcription factors (TFAM and TF2BM), transcription elongation factor (TEFM), and transcription termination factor (mTERF1) (Barshad et al., 2018). The “tRNA punctuation” model (Ojala et al., 1981) proposes that individual mRNA, rRNAs, and tRNAs are released from the polycistronic transcripts by the cleavage of tRNAs, which is in humans performed by endonucleases RNase P complex and ELAC2 (Holzmann et al., 2008; Brzezniak et al., 2011). After release, the rRNAs undergo chemical nucleotide modifications before becoming part of mitoribosome, the tRNAs undergo chemical nucleotide modifications, CCA addition at the 3′-end, deadenylation and finally aminoacylation, and the mRNAs get 3′ end polyadenylated (D’Souza and Minczuk, 2018). The half-life of mitochondrial transcripts and the decay of RNA intermediates are mediated by a complex of polynucleotide phosphorylase (PNPase) and SUV3 (Borowski et al., 2013). Finally, the mature mRNAs, tRNAs, and the assembled mitoribosome come together in the translation apparatus, for the synthesis of 13 subunits of OXPHOS system.
Figure 1 Proposed mitochondrial proteome and transcriptome. Mitochondrial homeostasis is depending on its own gene expression, but also on the import of nuclear-encoded proteins from the cytoplasm. In recent years, emerging evidence suggests import, but also mtDNA-transcription of different classes of ncRNAs in mitochondria.
Figure 2 mtDNA map showing heavy (outside circle) and light (inside circle) strand and within them the control region with promoters (HSP1, HSP2, LSP), and genes encoding for 13 mitochondrial proteins, 2 rRNAs, 22 tRNAs, and recently discovered mitochondria-encoded lncRNAs (mtlncRNAs) (highlighted with red star).
As mtDNA’s coding capacity is very limited, mitochondria are heavily dependent on the import of about 1,500 nuclear-encoded proteins. Besides, there have been indications that mitochondrial homeostasis is maintained not just through proteins, but also ncRNAs (Figure 1). The presence of housekeeping mitochondrial nuclear-encoded ncRNAs has been postulated for decades. These ncRNAs include tRNAs (tRNALeuUAA, tRNAGlnUUG, tRNAGlnCUG, tRNALysCUU), 5S rRNA, RMRP, and RNase P (Chang and Clayton, 1987a; Chang and Clayton, 1987b; Kiss et al., 1992; Yoshionari et al., 1994; Magalhaes et al., 1998; Puranam and Attardi, 2001; Holzmann et al., 2008). A systematic analysis of mitochondrial transcriptome further strengthened these claims. RNA-seq from 143B cells mitochondria and mitoplasts revealed the presence of several nuclear- and mitochondrial-encoded small RNAs and antisense transcripts (Mercer et al., 2011). Soon afterward, Rackham et al. (2011) observed by RNA-seq on HeLa cells that ncRNAs, excluding rRNAs and tRNAs, make up 15% of the human mitochondrial transcriptome, and identified three lncRNAs transcribed from the mtDNA. Follow-up studies have also reported the presence of ncRNAs encoded by the nuclear DNA, especially miRNAs and lncRNAs, within mitochondria across various cell types and tissues, suggesting that these ncRNAs may play important roles in the mitochondrial homeostasis (Kim et al., 2017b; Jeandard et al., 2019). The summary of the proposed nuclear-encoded ncRNAs is given in Table 1.
Although detection of ncRNAs in mitochondria paved the way to more extensive research in this field with several examples of ncRNAs functionally described as directly impacting mitochondrial biology, these transcripts are far from being well characterized. It is important to mention that there are (still) many controversies and debates ongoing about the sole existence of ncRNA in mitochondria. The main obstacle presents the technical challenge of truly separating isolated and uncontaminated mitochondria from other membrane vesicles (endoplasmic reticulum (ER), the Golgi apparatus, the endosomes) they are tightly associated within the cell (Vendramin et al., 2017). Therefore, to assess the purity of mitochondria or mitoplasts, ER or other membrane vesicles should be used instead of cytosol or nucleus, which was not always the case. Mitoplasts—rather than mitochondria—should be subjected to RNase treatment before lysis in order to minimize the risk of contamination. Unfortunately, these control steps have not always been performed systematically, so the published data is to date a complicated topic of many debates (Vendramin et al., 2017). Moreover, implementation of high sensitive NGS techniques such as deep sequencing is likely to detect small amounts of contaminants, leading to data misinterpretation. Finally, as this field is still very fresh, many studies miss independent replicates and functional studies are published by one research group.
Despite these controversies, an increasing body of evidence has connected ncRNAs and their machinery with mitochondrial biology. In this review, we focus on classes of ncRNAs described to be functionally related with and/or localized in mitochondria: the housekeeping ncRNAs, miRNAs, and lncRNAs. We also take up the topic of mitochondrial micropeptides, recently discovered to be encoded within regions initially annotated as non-coding. Overall, we summarize knowledge on ncRNAs in mitochondrial biology and discuss their discovery, biosynthesis, import, and function in the context of both health and disease. Finally, we touch their potential as prognosis markers and therapeutic targets.
Several tRNAs, 5S rRNA, RMRP, and RNase P present housekeeping ncRNAs whose mitochondrial localization, transport, and function have been discussed for years. For some of them, their interacting RNA-binding proteins (RBPs) have been proposed and associated with mitochondrial import and function (Figure 3, Table 1). However, the exact import mechanism across mitochondrial membranes and the function of these ncRNAs remain unclear. It is important to note that reports of these ncRNAs have been sparse and therefore questionable, so more evidence is needed to confirm/deny their presence and role in mitochondria.
Figure 3 Proposed import mechanisms of tRNAs (A), 5s rRNA (B), and RMRP (C) into human mitochondria. ncRNAs could be targeted by various nuclear-encoded proteins localized in the nucleus and close or inside the organelle. The mechanism behind translocation across mitochondrial membranes is still unknown, but RMRP and Rnase P seem to require the PNPase (D).OMM, outer mitochondrial membrane; IMS, intermembrane space; IMM, inner mitochondrial membrane.
Nuclear-encoded tRNAs have been observed in mitochondria across many species, as most eukaryotes lack some of the essential tRNAs in their mtDNA and must import them (Tarassov et al., 2007; Schneider, 2011). Even though human mtDNA encodes all the necessary tRNAs, published data indicate that they are able to import some of the cytosolic tRNAs through conserved protein machinery. In vitro experiments have shown that the synthetic transcripts of yeast tRNAs could be internalized by the isolated human mitochondria (Kolesnikova et al., 2000; Entelis et al., 2001). Later, nuclear-encoded tRNAs have been detected in mitochondria (Rubio et al., 2008; Mercer et al., 2011), namely tRNALeuUAA, tRNAGlnUUG, and tRNAGlnCUG. Gowher et al. (2013) successfully targeted yeast tRNALys CUU into human mitochondria in vivo, suggesting similarities in the tRNA import between the two species (Figure 3A). The current proposal by Gowher et al. (2013) is that tRNAs are recruited from the cytosol to the mitochondria with the precursor pre-KARS2 (mitochondrial lysyl-tRNA synthetase), helped by ENO2 (glyolitic enlolase). It is still unclear how the tRNA-pre-KARS2 complex then gets internalized into the mitochondrial matrix (Gowher et al., 2013; Kim et al., 2017b). Possible protein import pathway could consist of the translocase of the outer (TOM) and inner (TIM) mitochondrial membrane, as in yeast (Tarassov and Martin, 1996). Although the import of tRNA is yet to be fully understood, it could present a novel concept for therapy for disorders caused by defects in mtDNA-encoded tRNAs. Successful import of tRNA compensating the mutated mtDNA could rescue defects in mitochondrial translation. Rescue of mtDNA mutations by the import of designed tRNAs to mitochondria has been reported in vitro and in vivo (Salinas et al., 2008; Wang et al., 2012a), but more recent reports are missing.
Several studies have suggested that 5S rRNA is imported to the mammalian mitochondria (Yoshionari et al., 1994; Magalhaes et al., 1998). Entelis et al. (2001) suggested that mitochondrial 5S rRNA might substitute for its lost counterpart and be part of mitoribosome large subunit. Smirnov et al. (2008) proposed a model of mitochondrial 5S rRNA import (Figure 3B), starting with the recognition and transport of 5S rRNA from the nucleus to the cytoplasm by TFIIIA (Ciganda and Williams, 2011). In the cytosol, 5S rRNA was proposed to interact with pre-MRPL18 (precursor of mitochondrial ribosomal protein L18). This interaction might induce a conformational change in 5S rRNA that makes it recognized and bound by the mitochondrial enzyme Rhodanese, which helps it possibly translocate into mitochondria through a yet unknown mechanism. In the matrix 5S rRNA was proposed to associate with the mature MRPL18 and with mitoribosomes, affecting mitochondrial translation efficiency (Smirnov et al., 2010; Smirnov et al., 2011). However, as cryo-electron microscopy did not detect 5S rRNA within the mammalian mitoribosome 5S rRNA (Greber et al., 2015), its possible function in mitochondria remains enigmatic.
RMRP is a part of the RNase MRP, a ribonucleoprotein complex whose function has been discussed for decades. In the nucleus, it is involved in the pre-rRNA processing (Schmitt and Clayton, 1993; Chu et al., 1994; Goldfarb and Cech, 2017). In mitochondria, it was postulated to cleave RNA complementary to the light chain near the D-loop sites that mark the transition from RNA to DNA synthesis (Chang and Clayton, 1987b; Lee and Clayton, 1997). Three RNA-binding proteins (RBPs- HuR, PNPase, and GRSF1) have been implicated in the RMRP transport and role in mitochondria (Figure 3C). In the nucleus, RMRP is bound to HuR, which promotes its export to the cytosol in a CRM1-dependent manner (Noh et al., 2016). The exported RMRP might be then targeted into the mitochondrial intermembrane space through yet unknown mechanisms where PNPase was suggested to enable its import into the matrix (Wang et al., 2012b), after which its abundance in the matrix was reported to be increased through the interaction with GRSF1 (Noh et al., 2016). However, recent studies cast a shadow on the role of RMRP complex in mitochondria. Agaronyan et al. (2015) have shown that the RNA primer formation is a result of a premature arrest of the mitochondrial RNA polymerase after a G-quadruplex. Moreover, only the 3′ half (~130 nt) of RMRP could be found in mitochondria, indicating a processing that would result in a loss of catalytic activity (Esakova and Krasilnikov, 2010). These reports indicate that RMRP unlikely acts as an endonuclease in mitochondria. However, its interaction with GRSF1, an important component of the RNA granules (Antonicka et al., 2013; Jourdain et al., 2013), might still make it involved in the RNA metabolism.
RNase P processes the 5′ leader of precursor tRNA, which is a critical step of processing mitochondrial polycistronic transcripts (Ojala et al., 1981; Rackham et al., 2016). Two types of RNase P are known: ribonucleoproteins RNases P containing RNase P and protein-only RNases P (PRORP) (Lechner et al., 2015; Klemm et al., 2016). In the majority of species, including humans, it is assumed that the ribonucleoprotein RNase P acts in the nucleus and PRORP in mitochondria (Holzmann et al., 2008; Lechner et al., 2015). Strengthening this assumption, studies have reported that mammalian mitochondrial RNAse P does not require the catalytic RNA component for catalysis (Rossmanith et al., 1995; Holzmann et al., 2008). Nevertheless, RNase P was partially purified from HeLa cells mitochondria. Detected “mtRNase P”, together with the observed sensitivity of RNAse P to the nuclease treatment, suggested that RNAse P acts as a ribonucleoprotein also in mitochondria (Doersen et al., 1985). In addition, several groups indicated that mtRNase P is imported into the mitochondrial matrix through interaction with PNPase (Wang et al., 2010; Mercer et al., 2011; Noh et al., 2016) (Figure 3D). However, as so far functional RNase P ribonucleoprotein has not been reported in mitochondria, the existence of mtRNase P remains controversial (Jeandard et al., 2019).
hTERC is the RNA component of the human telomerase, where it serves as a sequence template for the telomere replication (Gall, 1990). As its sequence contains a region similar to an RMRP and RNase P short stem-loop that was proposed to enable their entry into mitochondria (Wang et al., 2010), hTERC was also proposed to be mitochondria-localized (Cheng et al., 2018). It was detected by the RT-PCR in purified mitoplasts, but as as a shorter, 195 nt-long transcript, which was termed TERC-53. Zheng et al. (2019) demonstrated that TERC-53 is mostly localized in the cytosol, where it regulates cellular senescence and is involved in cognition decline in mice hippocampus without affecting telomerase activity or mitochondrial functions. Having this in mind, the authors hypothesized that TERC-53 is exported from the mitochondria back to the cytosol (Cheng et al., 2018; Zheng et al., 2019). However, this hypothesis indicates hTERC processing occurring within the mitochondria, which has so far not been reported.
Vertebrate genomes contain thousands of miRNAs: according to MiRBase catalog, with the human genome containing 2,654 mature sequences (Kozomara et al., 2019). The biogenesis and biological functions of miRNAs have been widely studied in eukaryotic cells (Bartel, 2009) (Figure 4). In short, miRNAs are transcribed from the intergenic regions or in antisense orientation to coding regions as the primary miRNA transcript (pri-miRNA). pri-miRNA is processed in the nucleus by Drosha and/or DiGeorge syndrome chromosomal region 8 (DGCR8). This results in premature miRNA (pre-miRNA) which is then bound by exportin 5 (XPO5). XPO5, along with RanGTP, enables the export of the pre-miRNA through the nuclear pore into the cytosol. There RNase Dicer (DICER1 in humans) cleaves it, producing mature double-stranded miRNA. From two strands, the “passenger strand” undergoes RNA degradation while the remaining “guide strand” associates with argonaute 2 (AGO2) and becomes part of a multiprotein RNA-induced silencing complex (RISC) (Han et al., 2006). The main function of miRNA within RISC is post-transcriptional gene regulation by promoting mRNA degradation or translational repression by sequence-specific binding to the target mRNA. mRNA degradation is achieved via AGO2 (Carthew and Sontheimer, 2009; Chekulaeva and Filipowicz, 2009). Translational control is mediated by GW182 (Czech and Hannon, 2011; Iwakawa and Tomari, 2015). Moreover, miRNAs have also been implicated in some non-canonical functions, such as direct transcription and chromatin state regulation in the nucleus, and even translational promotion (Vasudevan, 2012; Yao et al., 2019). Each miRNA can target multiple genes, enabling them to regulate the expression of over 60% of the human genes and therefore moderate any part of cellular biology (Bartel, 2009; Friedman et al., 2009). Focusing on mitochondria, based on their localization and genetic origin, three different classes of mitochondria-related miRNAs can be distinguished (1) cytoplasmic, nuclear-encoded miRNAs targeting mitochondria-related transcripts; (2) mitochondrial, nuclear-encoded miRNAs; and (3) mitochondrial, mtDNA-encoded miRNAs (Bandiera et al., 2013) (Figure 4). The two latter classes, termed mitomiRs, are yet to be functionally deciphered.
Figure 4 miRNA biogenesis, function in the cytoplasm within RISC, and proposed transport/presence in mitochondria. RISC, RNA-induced silencing complex.
As about 1,500 nuclear-encoded proteins are imported into mitochondria and involved in diverse mitochondrial functions, many miRNAs have been described as directly targeting their mRNAs in the cytoplasm. By downregulating transcripts encoding for proteins involved in a variety of mitochondrial processes, reported miRNAs can indirectly influence mitochondrial biology and homeostasis. A summary of miRNAs reported to target nuclear-encoded mitochondrial transcripts is given in Table 2.
The tricarboxylic acid (TCA) cycle is a central pathway in the metabolism of sugars, lipids, and amino acids. Several miRNAs have been described to directly target transcripts of enzymes involved in its chemical reactions (Figure 5, Table 2A). For example, miR-26a targets subunit X of pyruvate dehydrogenase (PDH). As PDH catalyzes a crucial reaction before acetyl-coA enters the TCA cycle, its repression is leading to the decreased levels of acetyl-coA and the accumulation of pyruvate (Chen et al., 2014). In cancer research, miRNAs have been discovered to have a role in developing drug tolerance. Altered miR-147b initiates a reversible state of tolerance to osimertinib in lung cancer cells by binding SDHD (Zhang et al., 2019). Pretreatment with a miR-147b inhibitor delayed osimertinib-associated drug tolerance, providing a promising target for preventing tumor relapse (Zhang et al., 2019).
Figure 5 miRNAs targeting transcripts encoding proteins involved in the TCA cycle. Red arrows present the repressing effect of miRNA on its target mRNA.
OXPHOS system is composed of five protein complexes in the inner mitochondrial membrane that through oxidoreductase reactions generate a proton gradient, ultimately driving ATP synthesis. Several miRNAs have been described as directly targeting the OXPHOS subunits or assembly factors (Figure 6, Table 2B). It was shown that miR-663 positively regulates OXPHOS subunit and assembly factor protein levels by direct stabilization of complex III assembly factor UQCC2 (Carden et al., 2017). In breast cancer cell lines, mitochondrial dysfunction downregulates miR-663 through hypermethylation of its promoter, which leads to decreasing OXPHOS proteins levels and enzymatic activity and stability of supercomplexes, which promotes tumorigenesis (Carden et al., 2017).
Figure 6 miRNAs targeting transcripts encoding proteins involved in the OXPHOS. Red arrows present the repressing effect of miRNA on its target mRNA.
Fatty acid metabolism includes catabolic and anabolic processes that involve triglycerides, phospholipids, steroid hormones, and ketone bodies. Several miRNAs have been described as regulators of these processes (Table 2C). As fatty acid oxidation defects have been linked to the obesity and the development of insulin resistance (Kusunoki et al., 2006), these miRNAs could serve as potential therapeutic targets. As an example, PPARGC1B encodes for PGC-1β, a transcriptional coactivator that promotes mitochondrial biogenesis. Interestingly, this locus can also encode for miR-378 and miR-378*, which counterbalance the effect of PGC1-β by targeting carnitine-O-acetyltransferase (CRAT) (Carrer et al., 2012). miR-378/378* knockout (KO) mice showed significantly greater mitochondrial function and oxidative capacity.
The main steps of breakdown and synthesis of amino acids occur in mitochondria. Several miRNAs have been connected to amino acid metabolism (Table 2D). Most of the published work is focused on the regulation of glutaminase (GLS), which catalyzes the conversion of glutamine to glutamate. miR-23a and miR-23b participate in targeting glutaminase and thereby contribute to the mitochondrial amino acid metabolism (Gao et al., 2009).
Parts of the nucleotide and one-carbon metabolism are occurring in mitochondria. Various miRNAs can influence these processes (Desler et al., 2010) (Table 2E). For example, miR-149, miR-125, and miR-22 have been found to target MTHFR (Stone et al., 2011; Wu C. et al., 2013).
Many mitochondrial transporter and carrier proteins enable the import and export of molecules across the mitochondrial membranes. By targeting the transcripts encoding for these proteins, miRNAs are able to influence mitochondrial biology (Table 2F). It has been shown that the miR-15/16 cluster, composed of miR-15b, miR-16, miR-195, and miR-424, target Arl2 (Nishi et al., 2010).
Mitochondria are constantly changing their size, shape, and number to maximize the capacity for OXPHOS and answer the cell needs. This is achieved through the coordinated processes of biogenesis, fission, and fusion (Tilokani et al., 2018). Several miRNAs have been shown to be involved in the regulation of mitochondrial dynamics by directly or indirectly targeting these key factors (Figure 7, Table 2G). miR-149 indirectly promotes mitochondrial biogenesis by inhibiting PARP-2, which increases the NAD+ levels and SIRT-1 activity, finally leading to the increased activity of PGC-1α, the master regulator of mitochondrial biogenesis. Skeletal muscles from a high fat diet-fed obese mice have low levels of miR-149 and present with mitochondrial dysfunction, which might be due to miR-149-induced SIRT-1/PGC-1α pathway dysregulation. Noteworthy, miRNAs have been implicated in the mitochondria-mediated transition of skeletal muscle fiber types. miR-499 directly targets Fnip1, a negative regulator of AMPK, a known activator of PGC-1 α, and thereby triggers a muscle mitochondrial oxidative metabolism program (Liu L. et al., 2016). The miR-30 family, highly expressed in heart, was reported to regulate mitochondria fission and apoptosis by directly targeting p53, a transcriptional activator of Drp1 (Li et al., 2010). In addition, Drp1 is indirectly regulated by miR-499, which targets Drp1 activator dephosphatase calcinurin (van Rooij et al., 2009; Wang et al., 2011). Finally, miR-499 transcription is regulated by p53 on the transcript level (Wang et al., 2011).
Figure 7 miRNA targeting transcripts encoding proteins involved in the mitochondrial dynamics, autophagy, mitophagy and ROS production. Red arrows present inhibitory effect of miRNA on its target mRNA or repressive effect of protein on its interaction partners, and green arrows present the activating effect of protein on its interaction partner.
MELAS syndrome is caused by mutations in mtDNA affecting tRNALeuUUR. One of the phenotypes of MELAS patients is the increased oxidative stress. In addition, mutant tRNAsLeuUUR have reduced levels of the taurine-containing chemical modification at the wobble uridine (U34). Meseguer et al. (2015) reported that elevated oxidative stress in mutant cells leads to induction of miRNA-9/9*, which then act as post-transcriptional repressors of the tRNA-modification enzymes GTPBP3, MTO1, and TRMU. Downregulation of these enzymes disrupts the chemical modification at U34 of non-mutant tRNAs and contributes to mitochondrial dysfunction (Meseguer et al., 2015).
Autophagy is a catabolic process which prevents cell damage and promotes the cell survival by degrading and/or recycling dysfunctional components during cellular stress (Dikic and Elazar, 2018). Mitophagy is a form of autophagy that removes faulty or superfluous mitochondria, regulating their number to match the cellular needs (Pickles et al., 2018). miRNAs are also involved in the mitochondria-mediated apoptosis (Figure 7, Table 2H). Moreover, they are frequently dysregulated in human cancers, where they may function as potent oncogenes or tumor suppressors (Peng and Croce, 2016). Since mitochondrial dysfunction is one of the hallmarks of cancer (Wallace, 2012), miRNAs targeting apoptosis-related transcripts could be important in the development of cancer therapies. miR-101 (Frankel et al., 2011), miR-30a (Zhu et al., 2009), miR-15a, and miR-16 (Cimmino et al., 2005) have been reported to target oncogenic Bcl-2 and Mcl-1, and are frequently deleted or decreased in chronic lymphocytic leukemia. miR-21 levels have been shown to be significantly increased, leading to reduced expression of PTEN in human lung and hepatocellular carcinomas (Meng et al., 2007; Zhang et al., 2010).
MitomiRs are defined as miRNAs with mitochondrial localization (Bandiera et al., 2011). The majority of mitomiRs were suggested to originate from the nuclear genome, but also there were reports of mtDNA-encoded miRNAs. Different experimental approaches across mammalian tissues and cell lines indicated the mitochondrial presence of miRNAs, but also proteins involved in miRNAs biogenesis and function, suggesting miRNAs import, transcription, and/or processing and function within mitochondria themselves. Intriguingly, mitomiRs have some unique features which distinguish them from conventional cytosolic miRNAs (Bandiera et al., 2011; Barrey et al., 2011). Most of the nuclear-encoded mitomiRs loci are located within mitochondrial gene clusters or close to mitochondrial genes, and their transcriptions are often coregulated (Baskerville and Bartel, 2005; Bandiera et al., 2011). Their size slightly differs (between 17 and 25 nt instead of the average 22 nt), and they contain short 3′ overhangs, stem-loop secondary structures, and unique thermodynamic features (Vendramin et al., 2017). They lack 5′cap and most were predicted in silico to target multiple mtDNA sites. It has thus been speculated that at least some of these features could present a signal for entry into mitochondria (Bandiera et al., 2011; Barrey et al., 2011).
mitomiRs have been found via different approaches (from miRNA microarray and RT-qPCR to deep sRNA-sequencing) and across various tissues and organisms. To begin with, sequence analysis of cDNA libraries from mice mitochondrial RNA identified clones mapping to four nuclear-encoded miRNAs and three regions within the D-loop (Lung et al., 2006). Other reports on miRNAs localized in mammalian mitochondria have expanded in the past decade (Kren et al., 2009; Bandiera et al., 2011; Barrey et al., 2011; Mercer et al., 2011; Sripada et al., 2012; Jagannathan et al., 2015), as summarized in Table 3. For example, Kren et al. (2009) reported by miRNA microarray 15 nuclear-encoded miRNAs from highly purified rat liver mitochondria and further strengthened their findings with Northern blot and stem-loop RT-qPCR analyses. Barrey et al. (2011) in silico predicted 33 pre-miRNAs and 25 miRNAs targeting mtDNA and experimentally confirmed localization of pre-mir302a, let-7b, and mir-365 to isolated mitochondria from the human myotubes. Mercer et al. (2011) detected 31 mitochondria-encoded small RNAs in human 143B mitoplasts by sRNA-seq, the majority (84%) derived from mt-tRNA genes.
The presence of miRNA-associated proteins in the mitochondria was only recently recognized (summarized in Table 4). Wang et al. (2015a) and Vargas et al. (2016) reported Dicer in the rat brain, but it was reported as absent in the mitochondria isolated from the heart (Chen et al., 2010; Das et al., 2012; Jagannathan et al., 2015). So far, only one colocalization of pre-miR-338 and Dicer in rat brain mitochondria has been published (Vargas et al., 2016). If indeed true, the presence of Dicer could indicate that mature miRNA are formed from the precursors in mitochondria, from where they could directly affect the mitochondrial transcripts or even be exported to act in the cytosol (Bienertova-Vasku et al., 2013). However, mitochondrial localization of Dicer, Drosha, and DGCR8 has not yet been validated by other groups. Several studies have documented the presence of RNA-interference components, most notably AGO2, in the mitochondria, implying the functional importance of mitomiRs. As an example, Ago2 immunoprecipitated with miRNA from mitochondria in rat cardiac myocytes (Das et al., 2012). In addition, FXR1, a postulated RISC subunit, has been found together with Ago2 in the mitochondrial matrix of mouse cardiomyocytes (Jagannathan et al., 2015). However, an important factor for miRNA-mediated translational repression- GW182 has not been detected in any studies (Ro et al., 2013; Zhang et al., 2014). Finally, the presence of Dicer and AGO2 in mitochondria need not necessarily imply processing and function of mitomiRs, as these enzymes are involved also in other, miRNA-independent, processes (Janowski et al., 2006; Song and Rossi, 2017).
Although protein transport across mitochondrial membranes is well described, the translocases for RNA transport across mitochondrial membranes remain speculative. Several mechanisms of miRNAs transport into the mitochondria have been proposed. As shown in Figure 8, the potential players are AGO2, processing bodies (P-bodies), polynucleotide phosphorylase (PNPase) and voltage-gated ion channels (VDAC). AGO2 has been proposed as an important factor in the subcellular localization of miRNAs. Zhang et al. (2014) have shown an association of miR-1 with Ago2 in mitochondria and proposed their mechanism of action. At the baseline, miR-1 is found in the cytoplasm within RISC with 3′UTR of HDAC4. However, during myogenesis, GW182 detaches and HDAC4 loses 5′cap and poly(A) tail, suggesting that loss of GW182 alone or in combination with changes in HDAC4 facilitates the transport of Ago2:miR-1 into mitochondria (Figure 8A). Still, it remains unclear if AGO2 and miRNA translocate together as a complex (Figure 8B) or separately (Figure 8C) into the mitochondria and by which mechanism. Another hypothesis involves P-bodies, as they interact with mitochondria and can regulate mRNA decay, mRNA storage, and possibly miRNA import into different cellular compartments (Huang et al., 2011; Bandiera et al., 2013; Luo et al., 2018). Activation of several pathways and phosphorylation at the Ago2 Ser387 site has been shown to separate the Ago2/miRNA complex from the RISC and activate its intake into the P-body (Huang et al., 2011; McKenzie et al., 2016) (Figure 8D). As GW182 is also a P-body subunit (Liu et al., 2005), it might still have significance for the Ago2-miRNA import. PNPase is another candidate, as it has already been postulated to recognize specific structures of the housekeeping ncRNAs and help RNA fold properly to migrate through the mitochondrial membranes and return to its original conformation when they arrive in the mitochondrial matrix (Wang et al., 2010; Wang et al., 2012a) (Figure 8E). Several pre-miRNAs share the specific stem-loop structure that PNPase could recognize and enable import (Wang et al., 2010; Barrey et al., 2011; Lin et al., 2012). PNPase levels were reported to affect mitomiR-378 mitochondrial localization and co-immunoprecipitation showed Ago2 association with PNPase, suggesting that PNPase can bind to the miRNA within the complex with Ago2 (Shepherd et al., 2017). Transport across mitochondrial membranes could occur via TOM/TIM complexes (Figure 8F). Still, additional studies are needed to prove whether and how Ago2 can go through such small pores, even if facilitated by PNPase. Finally, it has been demonstrated that VDAC, the most abundant outer mitochondrial membrane protein in plants, could help transport of tRNAs across the outer mitochondrial membrane in plant cells (Salinas et al., 2006) (Figure 8G). This mechanism is yet to be tested in the animal systems.
Figure 8 Proposed import mechanisms of miRNAs to mammalian mitochondria. A detachment of AGO2 and miRNA from RISC or just GW182 due to AGO2 phosphorylation or some other signal activation (A) could promote their translocation together (B) or separately (C) into the mitochondria. This process could be stimulated by P-bodies (D). Translocation across mitochondrial membranes is unknown but suggested to be promoted by PNPase (E) and occur within TOM/TIM complexes (F). Alternatively, miRNAs could rely on VDAC (G) at the OMM, as proposed for tRNAs in plants. OMM, outer mitochondrial membrane; IMS, intermembrane space; IMM, inner mitochondrial membrane; TOM/TIM, translocases of OMM/IMM; VDAC, voltage-gated ion channels.
Although many have been detected, very few mitomiRs were functionally described to impact mitochondria (Baradan et al., 2017). Das et al. (2012) found miR-181c, Ago2, and COX1 in mitochondrial co-immunoprecipitate, suggesting that mature miR-181c could translocate to mitochondria and together with Ago2 repress the translation of this mitochondrial transcript. Overexpression of miR-181c seems to lead to a loss of COX1 and an increase COX2 and COX3, resulting in complex IV remodeling. miR-378 has been proposed to bind ATP6 in mitochondria in the presence of Ago2 and FXR1, leading to a decrease of ATP6 in mouse type 1 diabetic heart (Jagannathan et al., 2015). miR-1, specifically induced during myogenesis, is able to promote translation of COX1 and ND1 within Ago2-miRNA complex in mitochondria, while, on the contrary, suppressing its target transcripts in the cytosol (Zhang et al., 2014). However, the binding of miR-1 to mitochondrial transcripts has been suggested only by Ago2 CLIP experiments, and to date, miR‐1 is the only example of this non-canonical mitomiR function. Nevertheless, as many mitochondrial diseases are caused by defects in mitochondrial translation (Pearce et al., 2013), the upregulation of mitochondrial translation via miRNAs may be a new therapeutic route for these diseases which currently have no cure and few treatment options. Finally, a recent report reveals the role of mitomiRs in mitochondrial transcriptional regulation. mitomiR-2392, together with Ago2, was reported to recognize target sequences in the H-strand and partially inhibit polycistronic mtDNA transcription in a tongue squamous cell carcinoma (TSCC) cells, leading to downregulation of oxidative phosphorylation and upregulation of glycolysis (Fan et al., 2019).
To summarize, the identification of a miRNA inside mitochondria has, without a doubt, raised the interest in studying mitomiRs. However, mitomiRs are far from being well recognized. It is initially crucial to prevent any contamination during mitochondrial/mitoplast isolation to certain their mitochondrial localization. Furthermore, the mechanisms of their import, including interaction factors and important sequence features, and functions in mitochondria are yet to be elucidated. One should be aware that mitomiRs reported across various cell types and species show a very poor overlap. This could reflect species and cell type-specific expression of mitomiRs (Geiger and Dalgaard, 2017). On the other hand, such low reproducibility raises urgent questions regarding the techniques used in the published studies (Vendramin et al., 2017). Although several hypotheses concerning miRNA import into mitochondria have been proposed, it remains without convincing experimental validation. Finally, mitomiRs mode of action in mitochondria is largely enigmatic. On the one hand, only AGO2 from RISC has been proposed to reside in the mitochondria and on the other hand, mitochondrial mRNAs contain no or very small 3′ UTRs, questioning if they can function as canonical miRNAs.
The number of lncRNA genes in mammals varies broadly between different sources, from less than 20,000 to more than 100,000 in humans (Zhao et al., 2016; Kopp and Mendell, 2018). According to noncode.org, they are encompassing ∼144 000 loci in humans (Zhao et al., 2016). Intriguingly, although nucleus-enriched, lncRNAs have been observed in different cell compartments, including mitochondria (Dong et al., 2017). Their biological activities are highly influenced by their localization in the cell (Mercer and Mattick, 2013; Fatica and Bozzoni, 2014). lncRNAs have been suggested to regulate cellular biology via transcriptional regulation, organization of nuclear domains, and bindings to proteins or other RNAs (Ulitsky and Bartel, 2013; Kopp and Mendell, 2018). It is therefore not surprising that their disruption has been associated with different diseases (Briggs et al., 2015; Huarte, 2015; Uchida and Dimmeler, 2015).
lncRNAs can be functionally classified into those that act in cis, and those that act in trans (Kopp and Mendell, 2018). In cis, the lncRNA locus can regulate chromatin or gene expression of nearbye genes in at least three potential mechanisms: (1) DNA elements within the lncRNA promoter or locus carry the regulatory function, which is not related to the lncRNA or its production; (2) the act of transcription and/or splicing of the lncRNA affects nearby genes, irrespective of the transcribed lncRNA sequence; and (3) the lncRNA transcript alone affects the nearby genes, most commonly leading to the establishment of repressive or activating chromatin states. Some lncRNAs function in trans throughout the cell in, again, at least three potential mechanisms: (1) lncRNAs affect chromatin states and gene expression of distant genetic regions, (2) lncRNAs take part in the nuclear structure and organization (for example, as parts of speckles and paraspeckles), and (3) lncRNAs interact with proteins and/or other RNA molecules and modulate their expression and function (Lee, 2012; Rinn and Chang, 2012). Moreover, some transcripts initially annotated as lncRNAs are not non-coding, but actually encoding for biologically active micropeptides (Anderson et al., 2015; Matsumoto et al., 2017; Kopp and Mendell, 2018).
Over twenty lncRNAs have been described so far to affect the mitochondrial biology directly or indirectly. Some act in the cytosol, by regulating mitochondria-associated genes, often in interaction with miRNA, thus creating a complex mRNA-ncRNA regulation network. Other nuclear-encoded lncRNAs have been described to localize and act in mitochondria. As their transport mechanism into mitochondria is unknown their presence remains questionable. Finally, several lncRNAs have been discovered to be transcribed from mtDNA. These two latter mitochondria-localized, but origin-different lncRNAs could be refered to as nuclear-transported mitochondria-associated lncRNAs (ntmtlncRNAs) and mitochondria-encoded lncRNAs (mtlncRNAs) (Zhao et al., 2018).
Several lncRNAs, some previously well described in the non-mitochondrial function, have been associated with mitochondrial metabolism. As in the case of miRNAs, these lncRNAs were proposed to impact a variety of mitochondrial functions by directly targeting or indirectly influencing mitochondrial-related genes/transcripts/proteins. It should be noted that most of these studies report an indirect effect of lncRNAs perturbations on mitochondria function. Besides, most of these lncRNAs were reported in the context of complex systems such as cancer. Nevertheless, they could present possible treatment strategies (De Paepe et al., 2018). A summary of these findings is given in Table 5, with several examples given below.
Cerox1 (cytoplasmic endogenous regulator of oxidative phosphorylation 1) has been described as the first direct lncRNA modulator of OXPHOS. It has been reported to positively regulate the levels of at least 12 complex I transcripts in miRNA-dependent fashion, by binding miR-488-3p and blocking its post-transcriptional repression of these transcripts and enabling translation. Cerox1 knockdown was shown to decrease the enzymatic activities of complex I and IV. Accordingly, its overexpression was shown to increase their enzymatic activities and halve the cellular oxidative stress (Sirey et al., 2019).
Long et al. (2016) have described Tug1 as a regulator of PGC-1α transcription in diabetic nephropathy (DN). Tug1-binding site was identified upstream of the Ppargc1a promoter region. Tug1 interaction with this region recruited PGC-1α to promote its own gene transcription. Tug1 expression was significantly repressed in the podocytes of diabetic mice and its overexpression lead to improved mitochondrial bioenergetics (Long et al., 2016).
Li et al. (2017) proposed the pro-oncogenic role of lncRNA UCA1 in bladder tumors. UCA1 is supposed to regulate mitochondrial function through upregulating ARL2, a direct target of miR-195. In this way, it inhibits the miR-195 signaling pathway, leading to a tumor growth (Li et al., 2017).
Several nuclear-encoded lncRNAs have been reported in mitochondria and proposed to regulate their biology (Vendramin et al., 2017; Zhao et al., 2018). However, due to a very limited number of publications and unresolved import mechanism, the presence and role of these lncRNAs are yet to be confirmed.
SAMMSON is predominantly expressed in aggressive melanomas, where it was described as a promoter of cell growth (Leucci et al., 2016; Vendramin et al., 2018). It has been proposed to bind to CARF and promote its binding to p32 in the cytosol (Vendramin et al., 2018). p32 is a mitochondrial and cytosolic protein that is required for the maturation of mitochondrial rRNAs (Wu H. et al., 2013), but also described as an important player in tumor metabolism (Fogal et al., 2010). Its interaction with CARF via SAMMSON promotes its mitochondrial targeting, where it increases protein synthesis, leading to an increased tumor cell growth (Vendramin et al., 2018). Knockdown of SAMMSON was shown to impair the p32 targeting to the mitochondria, resulting in mitochondrial protein synthesis defects and increased apoptosis, which could be of therapeutical potential (Leucci et al., 2016). As a fraction of SAMMSON was found to co-localize and co-purify with mitochondria, Leucci et al. (2016) proposed that it is accompanying p32 to the mitochondria.
The steroid receptor RNA activator (SRA) is an important coactivator of nuclear hormone receptors and a target for several RBPs, namely SHARP and SLIRP (Colley et al., 2008). By interaction with SRA, SHARP represses SRA-augmented estrogen-induced transactivation (Shi et al., 2001). SLIRP binds to the complex of SRA and SHARP and interferes with the repressing activity of SHARP. However, SLIRP is predominantly localized to the mitochondria (Colley et al., 2008; Pagliarini et al., 2008), where it regulates the expression, processing, and stability of mRNAs (Baughman et al., 2009; Dong et al., 2017). SRA and SLIRP were found in mitochondria, but their import and roles are yet to be explained (Dong et al., 2017).
Metastasis-associated lung adenocarcinoma transcript 1 (MALAT1) is one of the most-studied lncRNAs, mostly associated with cancer and metastasis (Wu et al., 2015; Sun and Li, 2019). Recently, Zhao et al. (2019) discovered that MALAT1, although normally enriched in the nucleus, to be also enriched in the mitochondria collected from HepG2 cells. MALAT1-deficient HepG2 cells produced less ATP and had impaired cell invasion, suggesting a role of this lncRNA in the mitochondrial metabolism (Zhao et al., 2019).
Sets of lncRNAs have been reported to be transcribed from the mtDNA (Figure 2). Surprisingly, it has been noted that some of these lncRNAs seem to operate in the nucleus. However, their trafficking raises questions far beyond the current knowledge (Dietrich et al., 2015; Vendramin et al., 2017). Up to this date, the existence and functional relevance of these lncRNAs are still debatable. Mitochondria-encoded lncRNAs are divided into three categories:
1. Simple antisense mitochondrial DNA-encoded lncRNAs
Antisense transcripts arising from the ND4 and ND6 loci were initially detected in cDNA libraries of mice mitochondria, but Northern blot failed to confirm their presence (Lung et al., 2006). Later, strand-specific RNA-seq of purified mitochondria identified lncND5, lncND6, and lncCytb as antisense transcripts (Mercer et al., 2011). Rackham et al. (2011) confirmed existence of these transcripts by RNA-seq and RT-qPCR, additionally revealing that they are 58%, 34% and 14% as abundant as their mRNA counterparts, respectively. These antisense RNAs create RNA-RNA duplexes with their complementary mRNAs, suggesting their role in mRNAs expression and stability (Rackham et al., 2011). Interestingly, Zhao et al. (2019) discovered that lncCytB is aberrantly transported to the nucleus in hepatoma HepG2 cells as compared with normal hepatic HL7702 cells, suggesting a new function of this lncRNA as a mitochondria-nuclear communicator in cancer. Furthermore, Gao et al. (2018) discovered within the PacBio full-length transcriptome dataset the lncRNA MDL1, which covers the tRNAPro antisense gene and the entire D-loop region, and its antisense transcript MDL1AS.
2. Chimeric mitochondrial DNA-encoded lncRNAs
The first member of this class was discovered in mouse cells, comprised of the 16S rRNA linked to a 121 nucleotide 5′-leader sequence deriving from its complementary strand (Villegas et al., 2000). Afterward, similar transcript, called sense mitochondrial ncRNA (SncmtRNA), was identified in humans, and in this case, the mitochondrial 16S rRNA is linked to an 815 nucleotide 5′-leader sequence from its complementary strand (Villegas et al., 2007). SncmtRNA forms an 820 bp, double-stranded structure with a 40 nucleotide loop (Dietrich et al., 2015). Interestingly, SncmtRNA was only detected in the proliferating tumor but not in resting cells, suggesting that it might serve as a marker of cell proliferation (Villegas et al., 2007). Later, two antisense lncRNAs, called ASncmtRNA-1 and ASncmtRNA-2 were discovered. Here, the antisense mitochondrial 16S rRNA is linked to a 310 or 545 nucleotide 5′-leader sequence deriving from the complementary sense strand (Burzio et al., 2009). These two transcripts also form distinct double-stranded structures with a nucleotide loop. In contrast to SncmtRNA, they were detected mainly in normal cells and were much less expressed in proliferating tumor cells, suggesting their role as tumor suppressors (Burzio et al., 2009). Later, they were reported to be present in the nucleus associated with heterochromatin (Landerer et al., 2011). However, more data is needed to support this claim. It has been postulated that ASncmtRNA-2 gets transported into the nucleus, where it presents a precursor of two miRNAs (hsamiR-4485 and hsa-miR-1973), which could potentially regulate survivin, an inhibitor of apoptosis (Vidaurre et al., 2014; Bianchessi et al., 2015). Indeed, knockdown of ASncmtRNAs promoted apoptotic cell death due to the survivin downregulation at the translational level (Vidaurre et al., 2014).
3. Putative mitochondrial DNA-encoded lncRNAs
These lncRNAs have been identified in the heart disease studies (Kumarswamy et al., 2014; Yang et al., 2014; Dietrich et al., 2015). RNA-seq revealed a high relative abundance (over 70%) of these transcripts in the total lncRNA population from patients with a severe heart failure (Yang et al., 2014). The most significant lncRNA has been named long intergenic noncoding RNA predicting CARdiac remodeling (LIPCAR). Aligning the LIPCAR sequence to the human mtDNA revealed that the 5′ half aligns to the lncCytb, while the 3′ half aligns to the antisense region of COX2 (Dorn, 2014). As its circulating levels were increased in the late stages of left ventricular remodeling and patients with chronic heart failure, LIPCAR could be used as a prognostic biomarker (Kumarswamy et al., 2014; Dietrich et al., 2015).
To conclude, lncRNAs are slowly but surely drawing attention with their complex mechanisms behind gene regulation. However, the physiological relevance of lncRNAs in mitochondria is still enigmatic. The crucial issue is the investigation of transport of the nuclear- or mtDNA-encoded lncRNAs to mitochondria and even to the nucleus. Unfortunately, there is no published data on the topic so far. Finally, the questions of specific lncRNAs mechanisms of gene regulation remain to be solved.
Micropeptides are a class of small peptides encoded by a sORFs, without N-terminal signaling sequence and as such are released into cytoplasm immediately after translation. Due to their sORF that escapes automatic gene annotation, they tend to be overlooked and therefore misannotated as non-coding. Indeed, lncRNAs and TUFs (transcripts of unknown function) represent the greatest source for sORFs (Yeasmin et al., 2018). Although numerous ribosome profiling studies have reported substantial ribosome occupancy of the lncRNA transcripts, the MS and the proteogenomic approaches have confirmed only a small portion of them, numbers ranging from less than 100 to up to 1600 (van Heesch et al., 2019). With a lack of consensus in the datasets, the true coding potential of lncRNAs currently remains open to speculation. Several in-depth investigations have characterized lncRNA-derived micropeptides with important roles in the ion channel modulation (Anderson et al., 2015), cell signaling (Matsumoto et al., 2017) and RNA regulation (D’Lima et al., 2017). It is important to state that the mammalian mitochondrial proteome is surprisingly enriched in micropeptides, accounting for 5% of its proteins (Calvo et al., 2016). In recent years, several micropeptides within lncRNA were discovered and characterized with a role in mitochondria, some even encoded by the mtDNA (Kim et al., 2017a). Termed mitochondrial-derived peptides (MDPs) (Kim et al., 2017a), these mtDNA-encoded peptides- humanin, MOTS-c, and SHLPs were described as potential mitochondrial bioenergetics and metabolism regulators.
Mitoregulin (MOXI, MPM) has been discovered by four different groups recently as a muscle- and heart-enriched 56-amino acids inner mitochondrial membrane micropeptide encoded within LINC00116. It has a role in mitochondrial respiratory chain supercomplexes support, fatty acids oxidation, and Ca2+ dynamics (Makarewich et al., 2018; Stein et al., 2018; Chugunova et al., 2019; Lin et al., 2019). Lin et al. (2019) highlighted its importance in the muscle tissue, finding it upregulated during myogenic differentiation and knockout mice exhibiting smaller skeletal muscle fibers, worse muscle performance, and slower regeneration.
Humanin is a 24-amino acids micropeptide whose sORF is embeded within the 16S rRNA of mtDNA (Yen et al., 2013). It was initially discovered in the surviving cells of Alzheimer’s disease brain (Hashimoto et al., 2001), suggesting its neuroprotective and cytoprotective role that has later been investigated and acknowledged across various diseases (Hashimoto et al., 2001; Muzumdar et al., 2009; Bachar et al., 2010; Oh et al., 2011; Gong et al., 2014; Kim et al., 2018). It was shown to block apoptosis, improve insulin sensitivity, decrease inflammation, and reduce oxidative stress during aging (Guo et al., 2003; Muzumdar et al., 2009; Zhao et al., 2013; Sreekumar et al., 2016). Its effects are yet to be assessed for therapeutic purposes, especially in the treatments of diabetes and neurodegenerative disorders.
MOTS-c (mitochondrial open reading frame of the 12S rRNA type-c) is a 16-amino acids micropeptide with an sORF within the 12S rRNA mtDNA and reported to act in the cytoplasm (Lee et al., 2015). The micropeptide was found to target the methionine-folate cycle and de novo purine biosynthesis pathway, increase AICAR levels, and activate AMPK, by which it increases glucose utilization, fatty acid oxidation, and changes nucleotide metabolism. MOTS-c has been proposed as a biomarker for metabolic function, as it correlates with markers of insulin resistance and obesity (Du et al., 2018). In high fat diet-induced obese mice, it prevented obesity, fat accumulation, and hyperinsulinemia, making it a possible therapeutic target (Lee et al., 2015).
SHLPs (small humanin-like peptides) are a group of 6 peptides discovered by an in silico approach to be encoded in the 16S rRNA region of mtDNA in mice (Cobb et al., 2016). Each peptide is 20-38 amino acids long, and their names were given due to similar biological effects as Humanin. Each SHLP showed a unique expression pattern across different tissues. Incubation of each synthetic SHLP with cells affected cell viability, proliferation, and apoptosis differentially, suggesting a specific role of each. Moreover, SHLP2 and SHLP3 induced oxygen consumption rate (OCR) and increased cellular ATP levels, which indicated them as mitochondrial modulators (Cobb et al., 2016). Indeed, the administration of SHLP2 to a cellular model of macular degeneration rescued its defects in the OXPHOS and the mtDNA copy number, and induced anti-apoptotic effects, indicating its therapeutic potential (Nashine et al., 2018). In addition, an intracerebral infusion of SHLP2 increased glucose uptake and suppressed hepatic glucose production (Cobb et al., 2016). Further supporting their role as insulin sensitizers, both SHLPs promoted pre-adipocyte differentiation (Cobb et al., 2016). Similarly to humanin, the circulating levels of MOTS-c and SHLP2 declined with age, indicating that they are potential regulators of aging (Lee et al., Lee et al., 2015; Cobb et al., 2016).
Development of high-throughput OMICS techniques, especially the next-generation sequencing, has shed new light on the non-coding fraction of the genome. Transcription of the majority of the eukaryotic genome generates not only mRNAs but a much bigger fraction of different ncRNA species that show complex structure, patterns of expression and regulation. It is now becoming apparent that RNAs are not important for cell only in the context of mRNAs as intermediates between DNA and protein, but also as powerful players themselves by affecting basically any stage of gene expression. The now expanding RNA field highlights the importance of bioinformatics analysis in order to predict and examine existence, evolution, structure, and function of non-coding regions and transcripts. Focusing on mitochondria, dozens of ncRNAs acting in the cytosol have been described to indirectly influence mitochondrial biology, usually by targeting mitochondria-related, nuclear-encoded transcripts. More surprisingly, recent research indicated that the mitochondrial transcriptome could represent a mixture of the intrinsic transcriptome and complemented by some extrinsic RNA, implying RNA import (Figure 1). Although dozens of papers reported ncRNAs in mitochondria, their existence is still under a question mark. Further research will need to identify their interacting partners and elucidate the molecular mechanisms behind their synthesis, transport, and function. Housekeeping ncRNAs have been proposed to have a mitochondrial localization even for decades, however, recent deeper insights into the mitochondrial biology have cast a shadow on their hypothesized role. It is clear that the re-evaluation of their presence and especially function in mitochondria is needed. Focusing on miRNA, they are well-described fine-modulators of gene regulation in the cytosol. It is not surprising that they can impact mitochondria by targeting its transcripts in the cytosol. Additionally, recent discoveries of mitomiRs suggest an attractive, even closer interplay of miRNAs and mitochondria occurring in mitochondria themselves. Yet, these findings are still a topic of many debates and therefore should be handled with caution. On the one side, the discovery of mitomiRs across different tissues and cell types by different techniques promises they are more than a false-positive finding. However, on the other side is the poor overlap between datasets that raises doubts concerning methods used. Focusing on lncRNAs, although they are among the least well-understood of these transcript species, they are slowly but surely emerging as important components of gene regulatory networks. Although the field of lncRNAs has just started to expand, published reports indicate that they influence mitochondria in different ways. Moreover, mtDNA seems to encode some lncRNAs itself. However, this field is still very fresh and further confirmation is needed, especially in the case of mitochondria-imported lncRNAs. Of clinical relevance, ncRNAs dysregulation has been noted in various mitochondria-related diseases, mostly cancer. Their association with tumorigenesis has been increasingly demonstrated. As ncRNAs often exhibit cancer-type-specific expression patterns (Iyer et al., 2015), targeting them could prove as a very selective and specific approach. Notably, they can be targeted by the antagomiRs or antisense oligonucleotides (ASOs) (reviewed by Matsui and Corey, 2017). Indeed, several pre-clinical studies have already demonstrated the therapeutic benefits of ncRNA inhibition. For example, inhibition of SAMMSON in melanoma xenografts suppressed the tumor growth (Leucci et al., 2016). ASOs targeting ASncmtRNA reduced the progression of renal adenocarcinoma and melanoma metastases in mice (Lobos-Gonzalez et al., 2016; Borgna et al., 2017). Finally, ncRNA-derived micropeptides, although biologically active as peptides, are especially interesting in terms of their discovery. As many ribosomal-profiling studies report significant ribosomal occupancy of non-coding transcripts, it is evident that further confirmation of these findings by mass spectrometry is needed in order to recognize the importance of these reported translational activities. Discoveries of mitochondrial-derived peptides and enrichment of the mammalian mitochondrial proteome in micropeptides suggest the organelle as an evolutionary playground for small proteins, either due to still unknown localization signals or import system or simply driven by the size or amino acid (positive charge) composition (van Heesch et al., 2019). This also promises that there could be many micropeptides hidden in the non-coding region, awaiting discovery and characterization. Of clinical interest, discovered mitochondria-derived micropeptides have exhibited a variety of cyto- and neuroprotective effects, and promising results of both in vitro and in vivo studies further strengthen their therapeutic potential. Overall, ncRNAs in mitochondria present a thought-provoking, but unfortunately still neglected field of study. It raises many interesting, but also challenging questions whose answers might be of clinical importance. It may reveal some enigmatic biological mechanisms (such as the RNA import in mitochondria) and eventually lead to the development of new therapeutic strategies for mitochondria-related diseases. However, before the field of ncRNA truly expands, there are still a lot of experimental approaches to be optimized and biological mechanisms to be deciphered to conclude their importance for mitochondria.
MG: conceived the topic for the review, wrote the manuscript and created the tables and figures. HP: helped shape the review, supervised the writing process, provided the critical feedback, contributed to the final version of the manuscript.
The authors declare that the research was conducted in the absence of any commercial or financial relationships that could be construed as a potential conflict of interest.
Authors acknowledge the support by the DZHG (German Centre for Cardiovascular Research) and the BMBF (German Federal Ministry for Education and Research).
Adlakha, Y. K., Khanna, S., Singh, R., Singh, V. P., Agrawal, A., Saini, N. (2013). Pro-apoptotic miRNA-128-2 modulates ABCA1, ABCG1 and RXRalpha expression and cholesterol homeostasis. Cell Death Dis. 4, e780. doi: 10.1038/cddis.2013.301
Agaronyan, K., Morozov, Y. I., Anikin, M., Temiakov, D. (2015). Mitochondrial biology. Replication-transcription switch in human mitochondria. Science 347 (6221), 548–551. doi: 10.1126/science.aaa0986
Alessio, E., Buson, L., Chemello, F., Peggion, C., Grespi, F., Martini, P., et al. (2019). Single cell analysis reveals the involvement of the long non-coding RNA Pvt1 in the modulation of muscle atrophy and mitochondrial network. Nucleic Acids Res. 47 (4), 1653–1670. doi: 10.1093/nar/gkz007
Alvarez-Dominguez, J. R., Bai, Z., Xu, D., Yuan, B., Lo, K. A., Yoon, M. J., et al. (2015). De Novo reconstruction of adipose tissue transcriptomes reveals long Non-coding RNA regulators of brown adipocyte development. Cell Metab. 21 (5), 764–776. doi: 10.1016/j.cmet.2015.04.003
Anderson, S., Bankier, A. T., Barrell, B. G., de Bruijn, M. H., Coulson, A. R., Drouin, J., et al. (1981). Sequence and organization of the human mitochondrial genome. Nature 290 (5806), 457–465. doi: 10.1038/290457a0
Anderson, D. M., Anderson, K. M., Chang, C. L., Makarewich, C. A., Nelson, B. R., McAnally, J. R., et al. (2015). A Micropeptide encoded by a putative long noncoding RNA regulates muscle performance. Cell 160 (4), 595–606. doi: 10.1016/j.cell.2015.01.009
Andrews, S. J., Rothnagel, J. A. (2014). Emerging evidence for functional peptides encoded by short open reading frames. Nat. Rev. Genet. 15 (3), 193–204. doi: 10.1038/nrg3520
Antonicka, H., Sasarman, F., Nishimura, T., Paupe, V., Shoubridge, E. A. (2013). The mitochondrial RNA-Binding protein GRSF1 localizes to RNA granules and is required for posttranscriptional mitochondrial gene expression. Cell Metab. 17 (3), 386–398. doi: 10.1016/j.cmet.2013.02.006
Aoi, W., Naito, Y., Mizushima, K., Takanami, Y., Kawai, Y., Ichikawa, H., et al. (2010). The microRNA miR-696 regulates PGC-1{alpha} in mouse skeletal muscle in response to physical activity. Am. J. Physiol. Endocrinol. Metab. 298 (4), E799–E806. doi: 10.1152/ajpendo.00448.2009
Aschrafi, A., Schwechter, A. D., Mameza, M. G., Natera-Naranjo, O., Gioio, A. E., Kaplan, B. B. (2008). MicroRNA-338 regulates local cytochrome c oxidase IV mRNA levels and oxidative phosphorylation in the axons of sympathetic neurons. J. Neurosci. 28 (47), 12581–12590. doi: 10.1523/JNEUROSCI.3338-08.2008
Aschrafi, A., Kar, A. N., Natera-Naranjo, O., MacGibeny, M. A., Gioio, A. E., Kaplan, B. B. (2012). MicroRNA-338 regulates the axonal expression of multiple nuclear-encoded mitochondrial mRNAs encoding subunits of the oxidative phosphorylation machinery. Cell Mol. Life Sci. 69 (23), 4017–4027. doi: 10.1007/s00018-012-1064-8
Autour, A., Jeng, S. C. Y., Cawte, A. D., Abdolahzadeh, A., Galli, A., Panchapakesan, S. S. S., et al. (2018). Fluorogenic RNA Mango aptamers for imaging small non-coding RNAs in mammalian cells. Nat. Commun. 9 (1), 656. doi: 10.1038/s41467-018-02993-8
Bachar, A. R., Scheffer, L., Schroeder, A. S., Nakamura, H. K., Cobb, L. J., Oh, Y. K., et al. (2010). Humanin is expressed in human vascular walls and has a cytoprotective effect against oxidized LDL-induced oxidative stress. Cardiovasc. Res. 88 (2), 360–366. doi: 10.1093/cvr/cvq191
Bai, X. Y., Ma, Y., Ding, R., Fu, B., Shi, S., Chen, X. M. (2011). miR-335 and miR-34a Promote renal senescence by suppressing mitochondrial antioxidative enzymes. J. Am. Soc. Nephrol. 22 (7), 1252–1261. doi: 10.1681/ASN.2010040367
Bandiera, S., Ruberg, S., Girard, M., Cagnard, N., Hanein, S., Chretien, D., et al. (2011). Nuclear outsourcing of RNA interference components to human mitochondria. PloS One 6 (6), e20746. doi: 10.1371/journal.pone.0020746
Bandiera, S., Mategot, R., Girard, M., Demongeot, J., Henrion-Caude, A. (2013). MitomiRs delineating the intracellular localization of microRNAs at mitochondria. Free Radic. Biol. Med. 64, 12–19. doi: 10.1016/j.freeradbiomed.2013.06.013
Baradan, R., Hollander, J. M., Das, S. (2017). Mitochondrial miRNAs in diabetes: just the tip of the iceberg. Can. J. Physiol. Pharmacol. 95 (10), 1156–1162. doi: 10.1139/cjpp-2016-0580
Barrey, E., Saint-Auret, G., Bonnamy, B., Damas, D., Boyer, O., Gidrol, X. (2011). Pre-microRNA and mature microRNA in human mitochondria. PloS One 6 (5), e20220. doi: 10.1371/journal.pone.0020220
Barshad, G., Marom, S., Cohen, T., Mishmar, D. (2018). Mitochondrial DNA transcription and its regulation: an evolutionary perspective. Trends Genet. 34 (9), 682–692. doi: 10.1016/j.tig.2018.05.009
Bartel, D. P. (2009). MicroRNAs: target recognition and regulatory functions. Cell 136 (2), 215–233. doi: 10.1016/j.cell.2009.01.002
Bartkiewicz, M., Gold, H., Altman, S. (1989). Identification and characterization of an RNA molecule that copurifies with RNase P activity from HeLa cells. Genes Dev. 3 (4), 488–499. doi: 10.1101/gad.3.4.488
Baseler, W. A., Thapa, D., Jagannathan, R., Dabkowski, E. R., Croston, T. L., Hollander, J. M. (2012). miR-141 as a regulator of the mitochondrial phosphate carrier (Slc25a3) in the type 1 diabetic heart. Am. J. Physiology-Cell Physiol. 303 (12), C1244–C1251. doi: 10.1152/ajpcell.00137.2012
Baskerville, S., Bartel, D. P. (2005). Microarray profiling of microRNAs reveals frequent coexpression with neighboring miRNAs and host genes. RNA 11 (3), 241–247. doi: 10.1261/rna.7240905
Baughman, J. M., Nilsson, R., Gohil, V. M., Arlow, D. H., Gauhar, Z., Mootha, V. K. (2009). A computational screen for regulators of oxidative phosphorylation implicates SLIRP in mitochondrial RNA homeostasis. PloS Genet. 5 (8), e1000590. doi: 10.1371/journal.pgen.1000590
Bian, Z., Li, L. M., Tang, R., Hou, D. X., Chen, X., Zhang, C. Y., et al. (2010). Identification of mouse liver mitochondria-associated miRNAs and their potential biological functions. Cell Res. 20 (9), 1076–1078. doi: 10.1038/cr.2010.119
Bianchessi, V., Badi, I., Bertolotti, M., Nigro, P., D’Alessandra, Y., Capogrossi, M. C., et al. (2015). The mitochondrial lncRNA ASncmtRNA-2 is induced in aging and replicative senescence in Endothelial Cells. J. Mol. Cell. Cardiol. 81, 62–70. doi: 10.1016/j.yjmcc.2015.01.012
Bienertova-Vasku, J., Sana, J., Slaby, O. (2013). The role of microRNAs in mitochondria in cancer. Cancer Lett. 336 (1), 1–7. doi: 10.1016/j.canlet.2013.05.001
Borgna, V., Villegas, J., Burzio, V. A., Belmar, S., Araya, M., Jeldes, E., et al. (2017). Mitochondrial ASncmtRNA-1 and ASncmtRNA-2 as potent targets to inhibit tumor growth and metastasis in the RenCa murine renal adenocarcinoma model. Oncotarget 8 (27), 43692–43708. doi: 10.18632/oncotarget.18460
Borowski, L. S., Dziembowski, A., Hejnowicz, M. S., Stepien, P. P., Szczesny, R. J. (2013). Human mitochondrial RNA decay mediated by PNPase-hSuv3 complex takes place in distinct foci. Nucleic Acids Res. 41 (2), 1223–1240. doi: 10.1093/nar/gks1130
Briggs, J. A., Wolvetang, E. J., Mattick, J. S., Rinn, J. L., Barry, G. (2015). Mechanisms of long Non-coding RNAs in Mammalian nervous system development, plasticity, disease, and evolution. Neuron 88 (5), 861–877. doi: 10.1016/j.neuron.2015.09.045
Brzezniak, L. K., Bijata, M., Szczesny, R. J., Stepien, P. P. (2011). Involvement of human ELAC2 gene product in 3′ end processing of mitochondrial tRNAs. RNA Biol. 8 (4), 616–626. doi: 10.4161/rna.8.4.15393
Bucha, S., Mukhopadhyay, D., Bhattacharyya, N. P. (2015). Regulation of mitochondrial morphology and cell cycle by microRNA-214 targeting Mitofusin2. Biochem. Biophys. Res. Commun. 465 (4), 797–802. doi: 10.1016/j.bbrc.2015.08.090
Bukeirat, M., Sarkar, S. N., Hu, H., Quintana, D. D., Simpkins, J. W., Ren, X. (2016). MiR-34a regulates blood-brain barrier permeability and mitochondrial function by targeting cytochrome c. J. Cereb. Blood Flow Metab. 36 (2), 387–392. doi: 10.1177/0271678X15606147
Burzio, V. A., Villota, C., Villegas, J., Landerer, E., Boccardo, E., Villa, L. L., et al. (2009). Expression of a family of noncoding mitochondrial RNAs distinguishes normal from cancer cells. Proc. Natl. Acad. Sci. U. S. A. 106 (23), 9430–9434. doi: 10.1073/pnas.0903086106
Calvo, S. E., Clauser, K. R., Mootha, V. K. (2016). MitoCarta2.0: an updated inventory of mammalian mitochondrial proteins. Nucleic Acids Res. 44 (D1), D1251–D1257. doi: 10.1093/nar/gkv1003
Carden, T., Singh, B., Mooga, V., Bajpai, P., Singh, K. K. (2017). Epigenetic modification of miR-663 controls mitochondria-to-nucleus retrograde signaling and tumor progression. J. Biol. Chem. 292 (50), 20694–20706. doi: 10.1074/jbc.M117.797001
Carlevaro-Fita, J., Johnson, R. (2019). Global positioning system: understanding long noncoding RNAs through subcellular localization. Mol. Cell 73 (5), 869–883. doi: 10.1016/j.molcel.2019.02.008
Carrer, M., Liu, N., Grueter, C. E., Williams, A. H., Frisard, M. I., Hulver, M. W., et al. (2012). Control of mitochondrial metabolism and systemic energy homeostasis by microRNAs 378 and 378*. Proc. Natl. Acad. Sci. U. S. A. 109 (38), 15330–15335. doi: 10.1073/pnas.1207605109
Carthew, R. W., Sontheimer, E. J. (2009). Origins and mechanisms of miRNAs and siRNAs. Cell 136 (4), 642–655. doi: 10.1016/j.cell.2009.01.035
Chan, S. Y., Zhang, Y. Y., Hemann, C., Mahoney, C. E., Zweier, J. L., Loscalzo, J. (2009). MicroRNA-210 controls mitochondrial metabolism during hypoxia by repressing the iron-sulfur cluster assembly proteins ISCU1/2. Cell Metab. 10 (4), 273–284. doi: 10.1016/j.cmet.2009.08.015
Chang, D. D., Clayton, D. A. (1984). Precise identification of individual promoters for transcription of each strand of human mitochondrial DNA. Cell 36 (3), 635–664. doi: 10.1016/0092-8674(84)90343-x
Chang, D. D., Clayton, D. A. (1987a). A mammalian mitochondrial RNA processing activity contains nucleus-encoded RNA. Science 235 (4793), 1178–1184. doi: 10.1126/science.2434997
Chang, D. D., Clayton, D. A. (1987b). A novel endoribonuclease cleaves at a priming site of mouse mitochondrial DNA replication. EMBO J. 6 (2), 409–417. doi: 10.1002/j.1460-2075.1987.tb04770.x
Chaudhuri, A. D., Choi, D. C., Kabaria, S., Tran, A., Junn, E. (2016). MicroRNA-7 regulates the function of mitochondrial permeability transition pore by targeting VDAC1 expression. J. Biol. Chem. 291 (12), 6483–6493. doi: 10.1074/jbc.M115.691352
Chekulaeva, M., Filipowicz, W. (2009). Mechanisms of miRNA-mediated post-transcriptional regulation in animal cells. Curr. Opin. Cell Biol. 21 (3), 452–460. doi: 10.1016/j.ceb.2009.04.009
Chen, X., Wang, K. H., Chen, J. N., Guo, J. G., Yin, Y., Cai, X., et al. (2009). In vitro evidence suggests that miR-133a-mediated regulation of Uncoupling Protein 2 (UCP2) is an indispensable step in myogenic differentiation. J. Biol. Chem. 284 (8), 5362–5369. doi: 10.1074/jbc.M807523200
Chen, Z., Li, Y., Zhang, H., Huang, P., Luthra, R. (2010). Hypoxia-regulated microRNA-210 modulates mitochondrial function and decreases ISCU and COX10 expression. Oncogene 29 (30), 4362–4368. doi: 10.1038/onc.2010.193
Chen, B., Liu, Y., Jin, X., Lu, W., Liu, J., Xia, Z., et al. (2014). MicroRNA-26a regulates glucose metabolism by direct targeting PDHX in colorectal cancer cells. BMC Cancer 14, 443. doi: 10.1186/1471-2407-14-443
Chen, G. Y., Guo, H., Song, Y., Chang, H. Y., Wang, S. J., Zhang, M. M., et al. (2016). Long non-coding RNA AK055347 is upregulated in patients with atrial fibrillation and regulates mitochondrial energy production in myocardiocytes. Mol. Med. Rep. 14 (6), 5311–5317. doi: 10.3892/mmr.2016.5893
Chen, X., Zhou, X., Lu, D., Yang, X., Zhou, Z., Chen, X., et al. (2016). Aberrantly expressed long noncoding RNAs are involved in sevoflurane-induced developing hippocampal neuronal apoptosis: a microarray related study. Metab. Brain Dis. 31 (5), 1031–1040. doi: 10.1007/s11011-016-9838-6
Cheng, Y., Liu, P. P., Zheng, Q., Gao, G., Yuan, J. P., Wang, P. F., et al. (2018). Mitochondrial trafficking and processing of Telomerase RNA TERC. Cell Rep. 24 (10), 2589–2595. doi: 10.1016/j.celrep.2018.08.003
Chooniedass-Kothari, S., Emberley, E., Hamedani, M. K., Troup, S., Wang, X., Czosnek, A., et al. (2004). The steroid receptor RNA activator is the first functional RNA encoding a protein. FEBS Lett. 566 (1-3), 43–47. doi: 10.1016/j.febslet.2004.03.104
Chu, S., Archer, R. H., Zengel, J. M., Lindahl, L. (1994). The RNA of RNase MRP is required for normal processing of ribosomal RNA. Proc. Natl. Acad. Sci. U. S. A. 91 (2), 659–663. doi: 10.1073/pnas.91.2.659
Chugunova, A., Loseva, E., Mazin, P., Mitina, A., Navalayeu, T., Bilan, D., et al. (2019). LINC00116 codes for a mitochondrial peptide linking respiration and lipid metabolism. Proc. Natl. Acad. Sci. U. S. A. 116 (11), 4940–4945. doi: 10.1073/pnas.1809105116
Ciganda, M., Williams, N. (2011). Eukaryotic 5S rRNA biogenesis. Wiley Interdiscip. Rev. RNA 2 (4), 523–533. doi: 10.1002/wrna.74
Cimmino, A., Calin, G. A., Fabbri, M., Iorio, M. V., Ferracin, M., Shimizu, M., et al. (2005). miR-15 and miR-16 induce apoptosis by targeting BCL2. Proc. Natl. Acad. Sci. U. S. A. 102 (39), 13944–13949. doi: 10.1073/pnas.0506654102
Civelek, M., Hagopian, R., Pan, C., Che, N., Yang, W. P., Kayne, P. S., et al. (2013). Genetic regulation of human adipose microRNA expression and its consequences for metabolic traits. Hum. Mol. Genet. 22 (15), 3023–3037. doi: 10.1093/hmg/ddt159
Cobb, L. J., Lee, C. H., Xiao, J. L., Yen, K., Wong, R. G., Nakamura, H. K., et al. (2016). Naturally occurring mitochondrial-derived peptides are age-dependent regulators of apoptosis, insulin sensitivity, and inflammatory markers. Aging-Us 8 (4), 796–809. doi: 10.18632/aging.100943
Colley, S. M., Iyer, K. R., Leedman, P. J. (2008). The RNA coregulator SRA, its binding proteins and nuclear receptor signaling activity. IUBMB Life 60 (3), 159–164. doi: 10.1002/iub.22
Consortium, E. P. (2012). An integrated encyclopedia of DNA elements in the human genome. Nature 489 (7414), 57–74. doi: 10.1038/nature11247
Czech, B., Hannon, G. J. (2011). Small RNA sorting: matchmaking for Argonautes. Nat. Rev. Genet. 12 (1), 19–31. doi: 10.1038/nrg2916
D’Lima, N. G., Ma, J., Winkler, L., Chu, Q., Loh, K. H., Corpuz, E. O., et al. (2017). A human microprotein that interacts with the mRNA decapping complex. Nat. Chem. Biol. 13 (2), 174–180. doi: 10.1038/Nchembio.2249
D’Souza, A. R., Minczuk, M. (2018). Mitochondrial transcription and translation: overview. Mitochondrial Dis. 62 (3), 309–320. doi: 10.1042/Ebc20170102
Dahlmans, D., Houzelle, A., Andreux, P., Wang, X., Jorgensen, J. A., Moullan, N., et al. (2019). MicroRNA-382 silencing induces a mitonuclear protein imbalance and activates the mitochondrial unfolded protein response in muscle cells. J. Cell Physiol. 234 (5), 6601–6610. doi: 10.1002/jcp.27401
Das, S., Ferlito, M., Kent, O. A., Fox-Talbot, K., Wang, R., Liu, D. L., et al. (2012). Nuclear miRNA regulates the mitochondrial genome in the heart. Circ. Res. 110 (12), 1596–1603. doi: 10.1161/Circresaha.112.267732
Das, S., Bedja, D., Campbell, N., Dunkerly, B., Chenna, V., Maitra, A., et al. (2014). miR-181c regulates the mitochondrial genome, bioenergetics, and propensity for heart failure in vivo. PloS One 9 (5), e96820. doi: 10.1371/journal.pone.0096820
Dasgupta, N., Peng, Y., Tan, Z., Ciraolo, G., Wang, D., Li, R. (2015). miRNAs in mtDNA-less cell mitochondria. Cell Death Discovery 1, 15004. doi: 10.1038/cddiscovery.2015.4
De Paepe, B., Lefever, S., Mestdagh, P. (2018). How long noncoding RNAs enforce their will on mitochondrial activity: regulation of mitochondrial respiration, reactive oxygen species production, apoptosis, and metabolic reprogramming in cancer. Curr. Genet. 64 (1), 163–172. doi: 10.1007/s00294-017-0744-1
Derrien, T., Johnson, R., Bussotti, G., Tanzer, A., Djebali, S., Tilgner, H., et al. (2012). The GENCODE v7 catalog of human long noncoding RNAs: analysis of their gene structure, evolution, and expression. Genome Res. 22 (9), 1775–1789. doi: 10.1101/gr.132159.111
Desler, C., Lykke, A., Rasmussen, L. J. (2010). The effect of mitochondrial dysfunction on cytosolic nucleotide metabolism. J. Nucleic Acids. pii: 701518. doi: 10.4061/2010/701518. 2010.
Dietrich, A., Wallet, C., Iqbal, R. K., Gualberto, J. M., Lotfi, F. (2015). Organellar non-coding RNAs: emerging regulation mechanisms. Biochimie 117, 48–62. doi: 10.1016/j.biochi.2015.06.027
Dikic, I., Elazar, Z. (2018). Mechanism and medical implications of mammalian autophagy. Nat. Rev. Mol. Cell Biol. 19 (6), 349–364. doi: 10.1038/s41580-018-0003-4
Dinger, M. E., Pang, K. C., Mercer, T. R., Mattick, J. S. (2008). Differentiating protein-coding and noncoding RNA: challenges and ambiguities. PloS Comput. Biol. 4 (11), e1000176. doi: 10.1371/journal.pcbi.1000176
Djebali, S., Davis, C. A., Merkel, A., Dobin, A., Lassmann, T., Mortazavi, A., et al. (2012). Landscape of transcription in human cells. Nature 489 (7414), 101–108. doi: 10.1038/nature11233
Doersen, C. J., Guerrier-Takada, C., Altman, S., Attardi, G. (1985). Characterization of an RNase P activity from HeLa cell mitochondria. Comparison with the cytosol RNase P activity. J. Biol. Chem. 260 (10), 5942–5949.
Dong, Y. R., Yoshitomi, T., Hu, J. F., Cui, J. Z. (2017). Long noncoding RNAs coordinate functions between mitochondria and the nucleus. Epigenet. Chromatin 10 (1), 41. doi: 10.1186/s13072-017-0149-x
Dorn, G. W., 2nd (2014). LIPCAR: a mitochondrial lnc in the noncoding RNA chain? Circ. Res. 114 (10), 1548–1550. doi: 10.1161/CIRCRESAHA.114.304028
Du, Y., Li, J., Xu, T., Zhou, D. D., Zhang, L., Wang, X. (2017). MicroRNA-145 induces apoptosis of glioma cells by targeting BNIP3 and Notch signaling. Oncotarget 8 (37), 61510–61527. doi: 10.18632/oncotarget.18604
Du, C., Zhang, C., Wu, W., Liang, Y., Wang, A., Wu, S., et al. (2018). Circulating MOTS-c levels are decreased in obese male children and adolescents and associated with insulin resistance. Pediatr. Diabetes 19, 1058–1064. doi: 10.1111/pedi.12685
Duret, L., Chureau, C., Samain, S., Weissenbach, J., Avner, P. (2006). The Xist RNA gene evolved in eutherians by pseudogenization of a protein-coding gene. Science 312 (5780), 1653–1655. doi: 10.1126/science.1126316
el Azzouzi, H., Leptidis, S., Dirkx, E., Hoeks, J., van Bree, B., Brand, K., et al. (2013). The hypoxia-inducible microRNA cluster miR-199a approximately 214 targets myocardial PPARdelta and impairs mitochondrial fatty acid oxidation. Cell Metab. 18 (3), 341–354. doi: 10.1016/j.cmet.2013.08.009
Entelis, N. S., Kolesnikova, O. A., Dogan, S., Martin, R. P., Tarassov, I. A. (2001). 5 S rRNA and tRNA import into human mitochondria - Comparison of in vitro requirements. J. Biol. Chem. 276 (49), 45642–45653. doi: 10.1074/jbc.M103906200
Esakova, O., Krasilnikov, A. S. (2010). Of proteins and RNA: the RNase P/MRP family. RNA 16 (9), 1725–1747. doi: 10.1261/rna.2214510
Esau, C., Davis, S., Murray, S. F., Yu, X. X., Pandey, S. K., Pear, M., et al. (2006). miR-122 regulation of lipid metabolism revealed by in vivo antisense targeting. Cell Metab. 3 (2), 87–98. doi: 10.1016/j.cmet.2006.01.005
Fan, S., Chen, W. X., Lv, X. B., Tang, Q. L., Sun, L. J., Liu, B. D., et al. (2015). miR-483-5p determines mitochondrial fission and cisplatin sensitivity in tongue squamous cell carcinoma by targeting FIS1. Cancer Lett. 362 (2), 183–191. doi: 10.1016/j.canlet.2015.03.045
Fan, S., Tian, T., Chen, W., Lv, X., Lei, X., Zhang, H., et al. (2019). Mitochondrial miRNA determines chemoresistance by reprogramming metabolism and regulating Mitochondrial transcription. Cancer Res. 79 (6), 1069–1084. doi: 10.1158/0008-5472.CAN-18-2505
Fatica, A., Bozzoni, I. (2014). Long non-coding RNAs: new players in cell differentiation and development. Nat. Rev. Genet. 15 (1), 7–21. doi: 10.1038/nrg3606
Fazal, F. M., Han, S., Parker, K. R., Kaewsapsak, P., Xu, J., Boettiger, A. N., et al. (2019). Atlas of subcellular RNA localization revealed by APEX-Seq. Cell 178 (2), 473–490 e426. doi: 10.1016/j.cell.2019.05.027
Fields, A. P., Rodriguez, E. H., Jovanovic, M., Stern-Ginossar, N., Haas, B. J., Mertins, P., et al. (2015). A regression-based analysis of ribosome-profiling data reveals a conserved complexity to mammalian translation. Mol. Cell 60 (5), 816–827. doi: 10.1016/j.molcel.2015.11.013
Fogal, V., Richardson, A. D., Karmali, P. P., Scheffler, I. E., Smith, J. W., Ruoslahti, E. (2010). Mitochondrial p32 protein is a critical regulator of tumor metabolism via maintenance of oxidative phosphorylation. Mol. Cell Biol. 30 (6), 1303–1318. doi: 10.1128/MCB.01101-09
Frankel, L. B., Wen, J. Y., Lees, M., Hoyer-Hansen, M., Farkas, T., Krogh, A., et al. (2011). microRNA-101 is a potent inhibitor of autophagy. EMBO J. 30 (22), 4628–4641. doi: 10.1038/emboj.2011.331
Friedman, R. C., Farh, K. K., Burge, C. B., Bartel, D. P. (2009). Most mammalian mRNAs are conserved targets of microRNAs. Genome Res. 19 (1), 92–105. doi: 10.1101/gr.082701.108
Gall, J. G. (1990). Telomerase RNA: tying up the loose ends. Nature 344 (6262), 108–109. doi: 10.1038/344108a0
Gao, P., Tchernyshyov, I., Chang, T. C., Lee, Y. S., Kita, K., Ochi, T., et al. (2009). c-Myc suppression of miR-23a/b enhances mitochondrial glutaminase expression and glutamine metabolism. Nature 458 (7239), 762–765. doi: 10.1038/nature07823
Gao, J., Liu, M., Zou, Y., Mao, M., Shen, T., Zhang, C., et al. (2015). Long non-coding RNA growth arrest-specific transcript 5 is involved in ovarian cancer cell apoptosis through the mitochondria-mediated apoptosis pathway. Oncol. Rep. 34 (6), 3212–3221. doi: 10.3892/or.2015.4318
Gao, S., Tian, X. X., Chang, H., Sun, Y., Wu, Z. F., Cheng, Z., et al. (2018). Two novel lncRNAs discovered in human mitochondrial DNA using PacBio full-length transcriptome data. Mitochondrion 38, 41–47. doi: 10.1016/j.mito.2017.08.002
Ge, Y. X., Yan, X. D., Jin, Y. G., Yang, X. Y., Yu, X., Zhou, L. Q., et al. (2015). fMiRNA-192 and miRNA-204 directly suppress lncRNA HOTTIP and interrupt GLS1-mediated Glutaminolysis in Hepatocellular Carcinoma. PloS Genet. 12 (1), e1005825. doi: 10.1371/journal.pgen.1005726
Geiger, J., Dalgaard, L. T. (2017). Interplay of mitochondrial metabolism and microRNAs. Cell Mol. Life Sci. 74 (4), 631–646. doi: 10.1007/s00018-016-2342-7
Gerin, I., Clerbaux, L. A., Haumont, O., Lanthier, N., Das, A. K., Burant, C. F., et al. (2010). Expression of miR-33 from an SREBP2 intron inhibits cholesterol export and fatty acid oxidation. J. Biol. Chem. 285 (44), 33652–33661. doi: 10.1074/jbc.M110.152090
Goldfarb, K. C., Cech, T. R. (2017). Targeted CRISPR disruption reveals a role for RNase MRP RNA in human preribosomal RNA processing. Genes Dev. 31 (1), 59–71. doi: 10.1101/gad.286963.116
Gong, Z., Tas, E., Muzumdar, R. (2014). Humanin and age-related diseases: a new link? Front. Endocrinol. (Lausanne) 5, 210. doi: 10.3389/fendo.2014.00210
Gowher, A., Smirnov, A., Tarassov, I., Entelis, N. (2013). Induced tRNA import into human Mitochondria: implication of a Host Aminoacyl-tRNA-Synthetase. PloS One 8 (6), e66228. doi: 10.1371/journal.pone.0066228
Greber, B. J., Bieri, P., Leibundgut, M., Leitner, A., Aebersold, R., Boehringer, D., et al. (2015). Ribosome. The complete structure of the 55S mammalian mitochondrial ribosome. Science 348 (6232), 303–308. doi: 10.1126/science.aaa3872
Guan, X. X., Wang, L., Liu, Z. H., Guo, X. F., Jiang, Y., Lu, Y., et al. (2016). miR-106a promotes cardiac hypertrophy by targeting mitofusin 2. J. Mol. Cell. Cardiol. 99, 207–217. doi: 10.1016/j.yjmcc.2016.08.016
Guo, B., Zhai, D. Y., Cabezas, E., Welsh, K., Nouraini, S., Satterthwait, A. C., et al. (2003). Humanin peptide suppresses apoptosis by interfering with Bax activation. Nature 423 (6938), 456–461. doi: 10.1038/nature01627
Guttman, M., Russell, P., Ingolia, N. T., Weissman, J. S., Lander, E. S. (2013). Ribosome profiling provides evidence that large noncoding RNAs do not encode proteins. Cell 154 (1), 240–251. doi: 10.1016/j.cell.2013.06.009
Han, J., Lee, Y., Yeom, K. H., Nam, J. W., Heo, I., Rhee, J. K., et al. (2006). Molecular basis for the recognition of primary microRNAs by the Drosha-DGCR8 complex. Cell 125 (5), 887–901. doi: 10.1016/j.cell.2006.03.043
Hangauer, M. J., Vaughn, I. W., McManus, M. T. (2013). Pervasive transcription of the human genome produces thousands of previously unidentified long intergenic noncoding RNAs. PloS Genet. 9 (6), e1003569. doi: 10.1371/journal.pgen.1003569
Hashimoto, Y., Niikura, T., Tajima, H., Yasukawa, T., Sudo, H., Ito, Y., et al. (2001). A rescue factor abolishing neuronal cell death by a wide spectrum of familial Alzheimer’s disease genes and Abeta. Proc. Natl. Acad. Sci. U. S. A 98 (11), 6336–6341. doi: 10.1073/pnas.101133498
Holzmann, J., Frank, P., Loffler, E., Bennett, K. L., Gerner, C., Rossmanith, W. (2008). RNase P without RNA: identification and functional reconstitution of the human mitochondrial tRNA processing enzyme. Cell 135 (3), 462–474. doi: 10.1016/j.cell.2008.09.013
Hu, W., Chan, C. S., Wu, R., Zhang, C., Sun, Y., Song, J. S., et al. (2010). Negative regulation of tumor suppressor p53 by microRNA miR-504. Mol. Cell 38 (5), 689–699. doi: 10.1016/j.molcel.2010.05.027
Huang, L., Mollet, S., Souquere, S., Le Roy, F., Ernoult-Lange, M., Pierron, G., et al. (2011). Mitochondria associate with P-bodies and modulate MicroRNA-mediated RNA Interference. J. Biol. Chem. 286 (27), 24219–24230. doi: 10.1074/jbc.M111.240259
Huarte, M. (2015). The emerging role of lncRNAs in cancer. Nat. Med. 21 (11), 1253–1261. doi: 10.1038/nm.3981
Iliopoulos, D., Drosatos, K., Hiyama, Y., Goldberg, I. J., Zannis, V. I. (2010). MicroRNA-370 controls the expression of microRNA-122 and Cpt1alpha and affects lipid metabolism. J. Lipid Res. 51 (6), 1513–1523. doi: 10.1194/jlr.M004812
Ingolia, N. T., Ghaemmaghami, S., Newman, J. R. S., Weissman, J. S. (2009). Genome-wide analysis in vivo of translation with nucleotide resolution using ribosome profiling. Science 324 (5924), 218–223. doi: 10.1126/science.1168978
Iwakawa, H. O., Tomari, Y. (2015). The functions of MicroRNAs: mRNA decay and translational repression. Trends Cell Biol. 25 (11), 651–665. doi: 10.1016/j.tcb.2015.07.011
Iyer, M. K., Niknafs, Y. S., Malik, R., Singhal, U., Sahu, A., Hosono, Y., et al. (2015). The landscape of long noncoding RNAs in the human transcriptome. Nat. Genet. 47 (3), 199–208. doi: 10.1038/ng.3192
Jagannathan, R., Thapa, D., Nichols, C. E., Shepherd, D. L., Stricker, J. C., Croston, T. L., et al. (2015). Translational Rregulation of the Mitochondrial genome following redistribution of Mitochondrial MicroRNA in the diabetic heart. Circ. Cardiovasc. Genet. 8 (6), 785–802. doi: 10.1161/CIRCGENETICS.115.001067
Janowski, B. A., Huffman, K. E., Schwartz, J. C., Ram, R., Nordsell, R., Shames, D. S., et al. (2006). Involvement of AGO1 and AGO2 in mammalian transcriptional silencing. Nat. Struct. Mol. Biol. 13 (9), 787–792. doi: 10.1038/nsmb1140
Jeandard, D., Smirnova, A., Tarassov, I., Barrey, E., Smirnov, A., Entelis, N. (2019). Import of non-coding RNAs into human Mitochondria: a critical review and emerging approaches. Cells 8 (3), E286. doi: 10.3390/cells8030286
Ji, Z., Song, R. S., Regev, A., Struhl, K. (2015). Many lncRNAs, 5′ UTRs, and pseudogenes are translated and some are likely to express functional proteins. Elife 4, e08890. doi: 10.7554/eLife.08890
Jiang, J. D., Yang, J. F., Wang, Z. F., Wu, G. Y., Liu, F. (2013). TFAM is directly regulated by miR-23b in glioma. Oncol. Rep. 30 (5), 2105–2110. doi: 10.3892/or.2013.2712
Joshi, S. R., Dhagia, V., Gairhe, S., Edwards, J. G., McMurtry, I. F., Gupte, S. A. (2016). MicroRNA-140 is elevated and mitofusin-1 is downregulated in the right ventricle of the Sugen5416/hypoxia/normoxia model of pulmonary arterial hypertension. Am. J. Physiology-Heart Circulatory Physiol. 311 (3), H689–H698. doi: 10.1152/ajpheart.00264.2016
Jourdain, A. A., Koppen, M., Wydro, M., Rodley, C. D., Lightowlers, R. N., Chrzanowska-Lightowlers, Z. M., et al. (2013). GRSF1 Regulates RNA Processing in Mitochondrial RNA Granules. Cell Metab. 17 (3), 399–410. doi: 10.1016/j.cmet.2013.02.005
Kang, T., Lu, W., Xu, W., Anderson, L., Bacanamwo, M., Thompson, W., et al. (2013). MicroRNA-27 (miR-27) targets prohibitin and impairs adipocyte differentiation and mitochondrial function in human adipose-derived stem cells. J. Biol. Chem. 288 (48), 34394–34402. doi: 10.1074/jbc.M113.514372
Karbiener, M., Pisani, D. F., Frontini, A., Oberreiter, L. M., Lang, E., Vegiopoulos, A., et al. (2014). MicroRNA-26 family is required for human adipogenesis and drives characteristics of brown adipocytes. Stem Cells 32 (6), 1578–1590. doi: 10.1002/stem.1603
Kearse, M. G., Wilusz, J. E. (2017). Non-AUG translation: a new start for protein synthesis in eukaryotes. Genes Dev. 31 (17), 1717–1731. doi: 10.1101/gad.305250.117
Kim, S., Rhee, J. K., Yoo, H. J., Lee, H. J., Lee, E. J., Lee, J. W., et al. (2015). Bioinformatic and metabolomic analysis reveals miR-155 regulates thiamine level in breast cancer. Cancer Lett. 357 (2), 488–497. doi: 10.1016/j.canlet.2014.11.058
Kim, S. J., Xiao, J. L., Wan, J. X., Cohen, P., Yen, K. (2017a). Mitochondrially derived peptides as novel regulators of metabolism. J. Physiology-London 595 (21), 6613–6621. doi: 10.1113/Jp274472
Kim, K. M., Noh, J. H., Abdelmohsen, K., Gorospe, M. (2017b). Mitochondrial noncoding RNA transport. Bmb Rep. 50 (4), 164–174. doi: 10.5483/bmbrep.2017.50.4.013
Kim, S. J., Mehta, H. H., Wan, J., Kuehnemann, C., Chen, J., Cohen, P. (2018). Mitochondrial peptides modulate mitochondrial function during cellular senescence. Aging (Albany NY) 10 (6), 1239–1256. doi: 10.18632/aging.101463
Kiss, T., Marshallsay, C., Filipowicz, W. (1992). 7-2/MRP RNAs in plant and mammalian cells: association with higher order structures in the nucleolus. EMBO J. 11 (10), 3737–3746. doi: 10.1002/j.1460-2075.1992.tb05459.x
Klemm, B. P., Wu, N., Chen, Y., Liu, X., Kaitany, K. J., Howard, M. J., et al. (2016). The diversity of ribonuclease P: protein and RNA catalysts with analogous biological functions. Biomolecules 6 (2), E27. doi: 10.3390/biom6020027
Kolesnikova, O. A., Entelis, N. S., Mireau, H., Fox, T. D., Martin, R. P., Tarassov, I. A. (2000). Suppression of mutations in mitochondrial DNA by tRNAs imported from the cytoplasm. Science 289 (5486), 1931–1933. doi: 10.1126/science.289.5486.1931
Kong, L., Zhou, X., Wu, Y., Wang, Y., Chen, L., Li, P., et al. (2015). Targeting HOTAIR induces mitochondria related apoptosis and inhibits tumor growth in head and neck squamous cell carcinoma in vitro and in vivo. Curr. Mol. Med. 15 (10), 952–960. doi: 10.2174/1566524016666151123112716
Kopp, F., Mendell, J. T. (2018). Functional classification and experimental dissection of long noncoding RNAs. Cell 172 (3), 393–407. doi: 10.1016/j.cell.2018.01.011
Kozomara, A., Birgaoanu, M., Griffiths-Jones, S. (2019). miRBase: from microRNA sequences to function. Nucleic Acids Res. 47 (D1), D155–D162. doi: 10.1093/nar/gky1141
Kren, B. T., Wong, P. Y. P., Sarver, A., Zhang, X. X., Zeng, Y., Steer, C. J. (2009). microRNAs identified in highly purified liver-derived mitochondria may play a role in apoptosis. RNA Biol. 6 (1), 65–72. doi: 10.4161/rna.6.1.7534
Kumarswamy, R., Bauters, C., Volkmann, I., Maury, F., Fetisch, J., Holzmann, A., et al. (2014). Circulating long noncoding RNA, LIPCAR, predicts survival in patients with heart failure. Circ. Res. 114 (10), 1569–1575. doi: 10.1161/Circresaha.114.303915
Kurtz, C. L., Peck, B. C. E., Fannin, E. E., Beysen, C., Miao, J., Landstreet, S. R., et al. (2014). MicroRNA-29 Fine-Tunes the expression of key FOXA2-activated lipid metabolism genes and is dysregulated in animal models of insulin resistance and diabetes. Diabetes 63 (9), 3141–3148. doi: 10.2337/db13-1015
Kusunoki, J., Kanatani, A., Moller, D. E. (2006). Modulation of fatty acid metabolism as a potential approach to the treatment of obesity and the metabolic syndrome. Endocrine 29 (1), 91–100. doi: 10.1385/Endo:29:1:91
Landerer, E., Villegas, J., Burzio, V. A., Oliveira, L., Villota, C., Lopez, C., et al. (2011). Nuclear localization of the mitochondrial ncRNAs in normal and cancer cells. Cell. Oncol. 34 (4), 297–305. doi: 10.1007/s13402-011-0018-8
Lanz, R. B., McKenna, N. J., Onate, S. A., Albrecht, U., Wong, J. M., Tsai, S. Y., et al. (1999). A steroid receptor coactivator, SRA, functions as an RNA and is present in an SRC-1 complex. Cell 97 (1), 17–27. doi: 10.1016/S0092-8674(00)80711-4
Le, M. T. N., Teh, C., Shyh-Chang, N., Xie, H. M., Zhou, B. Y., Korzh, V., et al. (2009). MicroRNA-125b is a novel negative regulator of p53. Genes Dev. 23 (7), 862–876. doi: 10.1101/gad.1767609
Lechner, M., Rossmanith, W., Hartmann, R. K., Tholken, C., Gutmann, B., Giege, P., et al. (2015). Distribution of Ribonucleoprotein and Protein-Only RNase P in Eukarya. Mol. Biol. Evol. 32 (12), 3186–3193. doi: 10.1093/molbev/msv187
Lee, D. Y., Clayton, D. A. (1997). RNase mitochondrial RNA processing correctly cleaves a novel R loop at the mitochondrial DNA leading-strand origin of replication. Genes Dev. 11 (5), 582–592. doi: 10.1101/gad.11.5.582
Lee, C., Zeng, J., Drew, B. G., Sallam, T., Martin-Montalvo, A., Wan, J. X., et al. (2015). The Mitochondrial-derived peptide MOTS-c promotes metabolic Homeostasis and reduces obesity and insulin resistance. Cell Metab. 21 (3), 443–454. doi: 10.1016/j.cmet.2015.02.009
Lee, H., Tak, H., Park, S. J., Jo, Y. K., Cho, D. H., Lee, E. K. (2017). microRNA-200a-3p enhances mitochondrial elongation by targeting mitochondrial fission factor. Bmb Rep. 50 (4), 214–219. doi: 10.5483/BMBRep.2017.50.4.006
Lee, J. T. (2012). Epigenetic regulation by long noncoding RNAs. Science 338 (6113), 1435–1439. doi: 10.1126/science.1231776
Leivonen, S. K., Rokka, A., Ostling, P., Kohonen, P., Corthals, G. L., Kallioniemi, O., et al. (2011). Identification of miR-193b targets in breast cancer cells and systems biological analysis of their functional impact. Mol. Cell. Proteomics 10 (7), M110.005322. doi: 10.1074/mcp.M110.005322
Leucci, E., Vendramin, R., Spinazzi, M., Laurette, P., Fiers, M., Wouters, J., et al. (2016). Melanoma addiction to the long non-coding RNA SAMMSON. Nature 531 (7595), 518–522. doi: 10.1038/nature17161
Li, J. C., Donath, S., Li, Y. R., Qin, D., Prabhakar, B. S., Li, P. F. (2010). miR-30 regulates mitochondrial fission through targeting p53 and the dynamin-related Protein-1 pathway. PloS Genet. 6 (1), e1000795. doi: 10.1371/journal.pgen.1000795
Li, X., Wang, F. S., Wu, Z. Y., Lin, J. L., Lan, W. B., Lin, J. H. (2014). MicroRNA-19b targets Mfn1 to inhibit Mfn1-induced apoptosis in osteosarcoma cells. Neoplasma 61 (3), 265–273. doi: 10.4149/neo_2014_034
Li, Y., Peng, T., Li, L., Wang, X., Duan, R., Gao, H., et al. (2014). MicroRNA-9 regulates neural apoptosis in methylmalonic acidemia via targeting BCL2L11. Int. J. Dev. Neurosci. 36, 19–24. doi: 10.1016/j.ijdevneu.2014.04.005
Li, W., Zhang, X. L., Zhuang, H. X., Chen, H. G., Chen, Y. Q., Tian, W. L., et al. (2014). MicroRNA-137 is a novel hypoxia-responsive MicroRNA that inhibits mitophagy via regulation of two mitophagy receptors FUNDC1 and NIX. J. Biol. Chem. 289 (15), 10691–10701. doi: 10.1074/jbc.M113.537050
Li, H. J., Li, X., Pang, H., Pan, J. J., Xie, X. J., Chen, W. (2015). Long non-coding RNA UCA1 promotes glutamine metabolism by targeting miR-16 in human bladder cancer. Jpn. J. Clin. Oncol. 45 (11), 1055–1063. doi: 10.1093/jjco/hyv132
Li, X., Wang, H., Yao, B., Xu, W., Chen, J., Zhou, X. (2016). lncRNA H19/miR-675 axis regulates cardiomyocyte apoptosis by targeting VDAC1 in diabetic cardiomyopathy. Sci. Rep. 6, 36340. doi: 10.1038/srep36340
Li, H. J., Sun, X. M., Li, Z. K., Yin, Q. W., Pang, H., Pan, J. J., et al. (2017). LncRNA uca1 promotes mitochondrial function of bladder cancer via the MiR-195/ARL2 signaling pathway. Cell Physiol. Biochem. 43 (6), 2548–2561. doi: 10.1159/000484507
Lin, C. L., Wang, Y. T., Yang, W. Z., Hsiao, Y. Y., Yuan, H. S. (2012). Crystal structure of human polynucleotide phosphorylase: insights into its domain function in RNA binding and degradation. Nucleic Acids Res. 40 (9), 4146–4157. doi: 10.1093/nar/gkr1281
Lin, Y. F., Xiao, M. H., Chen, H. X., Meng, Y., Zhao, N., Yang, L., et al. (2019). A novel mitochondrial micropeptide MPM enhances mitochondrial respiratory activity and promotes myogenic differentiation. Cell Death Dis. 10 (7), 528. doi: 10.1038/s41419-019-1767-y
Liu, J., Rivas, F. V., Wohlschlegel, J., Yates, J. R., 3rd, Parker, R., Hannon, G. J. (2005). A role for the P-body component GW182 in microRNA function. Nat. Cell Biol. 7 (12), 1261–1266. doi: 10.1038/ncb1333
Liu, J., Liang, X., Zhou, D., Lai, L., Xiao, L., Liu, L., et al. (2016). Coupling of mitochondrial function and skeletal muscle fiber type by a miR-499/Fnip1/AMPK circuit. EMBO Mol. Med. 8 (10), 1212–1228. doi: 10.15252/emmm.201606372
Liu, B., Shen, E. D., Liao, M. M., Hu, Y. B., Wu, K., Yang, P., et al. (2016). Expression and mechanisms of long non-coding RNA genes MEG3 and ANRIL in gallbladder cancer. Tumour Biol. 37 (7), 9875–9886. doi: 10.1007/s13277-016-4863-y
Liu, T., Wang, Z., Zhou, R., Liang, W. (2019). Focally amplified lncRNA on chromosome 1 regulates apoptosis of esophageal cancer cells via DRP1 and mitochondrial dynamics. IUBMB Life 71 (2), 254–260. doi: 10.1002/iub.1971
Lobos-Gonzalez, L., Silva, V., Araya, M., Restovic, F., Echenique, J., Oliveira-Cruz, L., et al. (2016). Targeting antisense mitochondrial ncRNAs inhibits murine melanoma tumor growth and metastasis through reduction in survival and invasion factors. Oncotarget 7 (36), 58331–58350. doi: 10.18632/oncotarget.11110
Long, B., Wang, K., Li, N., Murtaza, I., Xiao, J. Y., Fan, Y. Y., et al. (2013). miR-761 regulates the mitochondrial network by targeting mitochondrial fission factor. Free Radical Biol. Med. 65, 371–379. doi: 10.1016/j.freeradbiomed.2013.07.009
Long, J. Y., Badal, S. S., Ye, Z. C., Wang, Y., Ayanga, B. A., Galvan, D. L., et al. (2016). Long noncoding RNA Tug1 regulates mitochondrial bioenergetics in diabetic nephropathy. J. Clin. Invest. 126 (11), 4205–4218. doi: 10.1172/Jci87927
Lung, B., Zemann, A., Madej, M. J., Schuelke, M., Techritz, S., Ruf, S., et al. (2006). Identification of small non-coding RNAs from mitochondria and chloroplasts. Nucleic Acids Res. 34 (14), 3842–3852. doi: 10.1093/nar/gkl448
Luo, Y., Na, Z., Slavoff, S. A. (2018). P-Bodies: composition, properties, and functions. Biochemistry 57 (17), 2424–2431. doi: 10.1021/acs.biochem.7b01162
Magalhaes, P. J., Andreu, A. L., Schon, E. A. (1998). Evidence for the presence of 5S rRNA in mammalian mitochondria. Mol. Biol. Cell 9 (9), 2375–2382. doi: 10.1091/mbc.9.9.2375
Makarewich, C. A., Baskin, K. K., Munir, A. Z., Bezprozvannaya, S., Sharma, G., Khemtong, C., et al. (2018). MOXI is a mitochondrial micropeptide that enhances fatty acid beta-oxidation. Cell Rep. 23 (13), 3701–3709. doi: 10.1016/j.celrep.2018.05.058
Marchi, S., Lupini, L., Patergnani, S., Rimessi, A., Missiroli, S., Bonora, M., et al. (2013). Downregulation of the mitochondrial calcium uniporter by cancer-related miR-25. Curr. Biol. 23 (1), 58–63. doi: 10.1016/j.cub.2012.11.026
Matsui, M., Corey, D. R. (2017). Non-coding RNAs as drug targets. Nat. Rev. Drug Discovery 16 (3), 167–179. doi: 10.1038/nrd.2016.117
Matsumoto, A., Pasut, A., Matsumoto, M., Yamashita, R., Fung, J., Monteleone, E., et al. (2017). mTORC1 and muscle regeneration are regulated by the LINC00961-encoded SPAR polypeptide. Nature 541 (7636), 228–232. doi: 10.1038/nature21034
Maxwell, E. S., Fournier, M. J. (1995). The small nucleolar RNAs. Annu. Rev. Biochem. 64, 897–934. doi: 10.1146/annurev.bi.64.070195.004341
McKenzie, A. J., Hoshino, D., Hong, N. H., Cha, D. J., Franklin, J. L., Coffey, R. J., et al. (2016). KRAS-MEK signaling controls ago2 sorting into exosomes. Cell Rep. 15 (5), 978–987. doi: 10.1016/j.celrep.2016.03.085
Meng, F., Henson, R., Wehbe-Janek, H., Ghoshal, K., Jacob, S. T., Patel, T. (2007). MicroRNA-21 regulates expression of the PTEN tumor suppressor gene in human hepatocellular cancer. Gastroenterology 133 (2), 647–658. doi: 10.1053/j.gastro.2007.05.022
Mercer, T. R., Mattick, J. S. (2013). Structure and function of long noncoding RNAs in epigenetic regulation. Nat. Struct. Mol. Biol. 20 (3), 300–307. doi: 10.1038/nsmb.2480
Mercer, T. R., Neph, S., Dinger, M. E., Crawford, J., Smith, M. A., Shearwood, A. M. J., et al. (2011). The human Mitochondrial transcriptome. Cell 146 (4), 645–658. doi: 10.1016/j.cell.2011.06.051
Mersey, B. D., Jin, P., Danner, D. J. (2005). Human microRNA (miR29b) expression controls the amount of branched chain alpha-ketoacid dehydrogenase complex in a cell. Hum. Mol. Genet. 14 (22), 3371–3377. doi: 10.1093/hmg/ddi368
Meseguer, S., Martinez-Zamora, A., Garcia-Arumi, E., Andreu, A. L., Armengod, M. E. (2015). The ROS-sensitive microRNA-9/9* controls the expression of mitochondrial tRNA-modifying enzymes and is involved in the molecular mechanism of MELAS syndrome. Hum. Mol. Gen. 24 (1), 167–184. doi: 10.1093/hmg/ddu427
Mohamed, J. S., Hajira, A., Pardo, P. S., Boriek, A. M. (2014). MicroRNA-149 inhibits PARP-2 and promotes mitochondrial biogenesis via SIRT-1/PGC-1alpha network in skeletal muscle. Diabetes 63 (5), 1546–1559. doi: 10.2337/db13-1364
Molitoris, J. K., McColl, K. S., Distelhorst, C. W. (2011). Glucocorticoid-mediated repression of the oncogenic microRNA cluster miR-17~92 contributes to the induction of Bim and initiation of apoptosis. Mol. Endocrinol. 25 (3), 409–420. doi: 10.1210/me.2010-0402
Montoya, J., Gaines, G. L., Attardi, G. (1983). The pattern of transcription of the human mitochondrial rRNA genes reveals two overlapping transcription units. Cell 34 (1), 151–159. doi: 10.1016/0092-8674(83)90145-9
Morita, S., Horii, T., Kimura, M., Hatada, I. (2013). MiR-184 regulates insulin secretion through repression of Slc25a22. Peerj 1, e162. doi: 10.7717/peerj.162
Morris, D. R., Geballe, A. P. (2000). Upstream open reading frames as regulators of mRNA translation. Mol. Cell. Biol. 20 (23), 8635–8642. doi: 10.1128/Mcb.20.23.8635-8642.2000
Mott, J. L., Kobayashi, S., Bronk, S. F., Gores, G. J. (2007). mir-29 regulates Mcl-1 protein expression and apoptosis. Oncogene 26 (42), 6133–6140. doi: 10.1038/sj.onc.1210436
Muzumdar, R. H., Huffman, D. M., Atzmon, G., Buettner, C., Cobb, L. J., Fishman, S., et al. (2009). Humanin: a novel central regulator of peripheral insulin action. PloS One 4 (7), e6334. doi: 10.1371/journal.pone.0006334
Nashine, S., Cohen, P., Nesburn, A. B., Kuppermann, B. D., Kenney, M. C. (2018). Characterizing the protective effects of SHLP2, a mitochondrial-derived peptide, in macular degeneration. Sci. Rep. 8, 15175. doi: 10.1038/s41598-018-33290-5
Nishi, H., Ono, K., Iwanaga, Y., Horie, T., Nagao, K., Takemura, G., et al. (2010). MicroRNA-15b modulates cellular ATP levels and degenerates mitochondria via Arl2 in neonatal rat cardiac myocytes. J. Biol. Chem. 285 (7), 4920–4930. doi: 10.1074/jbc.M109.082610
Noh, J. H., Kim, K. M., Abdelmohsen, K., Yoon, J. H., Panda, A. C., Munk, R., et al. (2016). HuR and GRSF1 modulate the nuclear export and mitochondrial localization of the lncRNA RMRP. Genes Dev. 30 (10), 1224–1239. doi: 10.1101/gad.276022.115
Noller, H. F., Lancaster, L., Mohan, S., Zhou, J. (2017). Ribosome structural dynamics in translocation: yet another functional role for ribosomal RNA. Quarterly Reviews of Biophysics. 50, e12. doi: 10.1017/S0033583517000117
Oh, Y. K., Bachar, A. R., Zacharias, D. G., Kim, S. G., Wan, J. X., Cobb, L. J., et al. (2011). Humanin preserves endothelial function and prevents atherosclerotic plaque progression in hypercholesterolemic ApoE deficient mice. Atherosclerosis 219 (1), 65–73. doi: 10.1016/j.atherosclerosis.2011.06.038
Ojala, D., Montoya, J., Attardi, G. (1981). tRNA punctuation model of RNA processing in human mitochondria. Nature 290 (5806), 470–474. doi: 10.1038/290470a0
Ori, A., Toyama, B. H., Harris, M. S., Bock, T., Iskar, M., Bork, P., et al. (2015). Integrated transcriptome and proteome analyses reveal organ-specific proteome deterioration in old rats. Cell Syst. 1 (3), 224–237. doi: 10.1016/j.cels.2015.08.012
Ouyang, Y. B., Lu, Y., Yue, S., Giffard, R. G. (2012). miR-181 targets multiple Bcl-2 family members and influences apoptosis and mitochondrial function in astrocytes. Mitochondrion 12 (2), 213–219. doi: 10.1016/j.mito.2011.09.001
Pagliarini, D. J., Calvo, S. E., Chang, B., Sheth, S. A., Vafai, S. B., Ong, S. E., et al. (2008). A mitochondrial protein compendium elucidates complex I disease biology. Cell 134 (1), 112–123. doi: 10.1016/j.cell.2008.06.016
Pearce, S., Nezich, C. L., Spinazzola, A. (2013). Mitochondrial diseases: translation matters. Mol. Cell Neurosci. 55, 1–12. doi: 10.1016/j.mcn.2012.08.013
Peng, Y., Croce, C. M. (2016). The role of MicroRNAs in human cancer. Signal Transduct. Target Ther. 1, 15004. doi: 10.1038/sigtrans.2015.4
Peng, Y., Xiang, H., Chen, C., Zheng, R., Chai, J., Peng, J., et al. (2013). MiR-224 impairs adipocyte early differentiation and regulates fatty acid metabolism. Int. J. Biochem. Cell Biol. 45 (8), 1585–1593. doi: 10.1016/j.biocel.2013.04.029
Pertea, M., Shumate, A., Pertea, G., Varabyou, A., Breitwieser, F. P., Chang, Y. C., et al. (2018). CHESS: a new human gene catalog curated from thousands of large-scale RNA sequencing experiments reveals extensive transcriptional noise. Genome Biol. 19 (1), 208. doi: 10.1186/s13059-018-1590-2
Pickles, S., Vigie, P., Youle, R. J. (2018). Mitophagy and quality control mechanisms in mitochondrial maintenance. Curr. Biol. 28 (4), R170–R185. doi: 10.1016/j.cub.2018.01.004
Prasanth, K. V., Spector, D. L. (2007). Eukaryotic regulatory RNAs: an answer to the ‘genome complexity’ conundrum. Genes Dev. 21 (1), 11–42. doi: 10.1101/gad.1484207
Puissegur, M. P., Mazure, N. M., Bertero, T., Pradelli, L., Grosso, S., Robbe-Sermesant, K., et al. (2011). miR-210 is overexpressed in late stages of lung cancer and mediates mitochondrial alterations associated with modulation of HIF-1 activity. Cell Death Differ. 18 (3), 465–478. doi: 10.1038/cdd.2010.119
Puranam, R. S., Attardi, G. (2001). The RNase P associated with HeLa cell mitochondria contains an essential RNA component identical in sequence to that of the nuclear RNase P. Mol. Cell. Biol. 21 (2), 548–561. doi: 10.1128/Mcb.21.2.548-561.2001
Quinones-Lombrana, A., Blanco, J. G. (2015). Chromosome 21-derived hsa-miR-155-5p regulates mitochondrial biogenesis by targeting Mitochondrial transcription factor A (TFAM). Biochim. Biophys. Acta 1852 (7), 1420–1427. doi: 10.1016/j.bbadis.2015.04.004
Rackham, O., Shearwood, A. M., Mercer, T. R., Davies, S. M., Mattick, J. S., Filipovska, A. (2011). Long noncoding RNAs are generated from the mitochondrial genome and regulated by nuclear-encoded proteins. RNA 17 (12), 2085–2093. doi: 10.1261/rna.029405.111
Rackham, O., Busch, J. D., Matic, S., Siira, S. J., Kuznetsova, I., Atanassov, I., et al. (2016). Hierarchical RNA processing is required for mitochondrial ribosome assembly. Cell Rep. 16 (7), 1874–1890. doi: 10.1016/j.celrep.2016.07.031
Ragan, C., Goodall, G. J., Shirokikh, N. E., Preiss, T. (2019). Insights into the biogenesis and potential functions of exonic circular RNA. Sci. Rep. 9 (1), 2048. doi: 10.1038/s41598-018-37037-0
Redis, R. S., Vela, L. E., Lu, W. Q., de Oliveira, J. F., Ivan, C., Rodriguez-Aguayo, C., et al. (2016). Allele-Specific reprogramming of cancer metabolism by the long non-coding RNA CCAT2. Mol. Cell 61 (4), 640–640. doi: 10.1016/j.molcel.2016.02.006
Rinn, J. L., Chang, H. Y. (2012). Genome regulation by long noncoding RNAs. Annu. Rev. Biochem. 81, 145–166. doi: 10.1146/annurev-biochem-051410-092902. Vol 81.
Rippo, M. R., Olivieri, F., Monsurro, V., Prattichizzo, F., Albertini, M. C., Procopio, A. D. (2014). MitomiRs in human inflamm-aging: a hypothesis involving miR-181a, miR-34a and miR-146a. Exp. Gerontol. 56, 154–163. doi: 10.1016/j.exger.2014.03.002
Ro, S., Ma, H. Y., Park, C., Ortogero, N., Song, R., Hennig, G. W., et al. (2013). The mitochondrial genome encodes abundant small noncoding RNAs. Cell Res. 23 (6), 759–774. doi: 10.1038/cr.2013.37
Rossmanith, W., Tullo, A., Potuschak, T., Karwan, R., Sbisa, E. (1995). Human mitochondrial tRNA processing. J. Biol. Chem. 270 (21), 12885–12891. doi: 10.1074/jbc.270.21.12885
Rottiers, V., Naar, A. M. (2012). MicroRNAs in metabolism and metabolic disorders. Nat. Rev. Mol. Cell Biol. 13 (4), 239–250. doi: 10.1038/nrm3313
Rubio, M. A. T., Rinehart, J. J., Krett, B., Duvezin-Caubet, S., Reichert, A. S., Soll, D., et al. (2008). Mammalian mitochondria have the innate ability to import tRNAs by a mechanism distinct from protein import. Proc. Natl. Acad. Sci. U. S. A. 105 (27), 9186–9191. doi: 10.1073/pnas.0804283105
Ruiz-Orera, J., Messeguer, X., Subirana, J. A., Alba, M. M. (2014). Long non-coding RNAs as a source of new peptides. Elife 3, e03523. doi: 10.7554/eLife.03523
Russell, A. P., Wada, S., Vergani, L., Hock, M. B., Lamon, S., Leger, B., et al. (2013). Disruption of skeletal muscle mitochondrial network genes and miRNAs in amyotrophic lateral sclerosis. Neurobiol. Dis. 49, 107–117. doi: 10.1016/j.nbd.2012.08.015
Salinas, T., Duchene, A. M., Delage, L., Nilsson, S., Glaser, E., Zaepfel, M., et al. (2006). The voltage-dependent anion channel, a major component of the tRNA import machinery in plant mitochondria. Proc. Natl. Acad. Sci. United States America 103 (48), 18362–18367. doi: 10.1073/pnas.0606449103
Salinas, T., Duchene, A. M., Marechal-Drouard, L. (2008). Recent advances in tRNA mitochondrial import. Trends In Biochem. Sci. 33 (7), 320–329. doi: 10.1016/j.tibs.2008.04.010
Salzman, J., Gawad, C., Wang, P. L., Lacayo, N., Brown, P. O. (2012). Circular RNAs are the predominant transcript isoform from hundreds of human genes in diverse cell types. PloS One 7 (2), e30733. doi: 10.1371/journal.pone.0030733
Schimmel, P. (2018). The emerging complexity of the tRNA world: mammalian tRNAs beyond protein synthesis. Nat. Rev. Mol. Cell Biol. 19 (1), 45–58. doi: 10.1038/nrm.2017.77
Schmitt, M. E., Clayton, D. A. (1993). Nuclear RNase MRP is required for correct processing of pre-5.8S rRNA in Saccharomyces cerevisiae. Mol. Cell Biol. 13 (12), 7935–7941. doi: 10.1128/mcb.13.12.7935
Schneider, A. (2011). Mitochondrial tRNA import and its consequences for mitochondrial translation. Annu. Rev. Biochem. 80, 1033–1053. doi: 10.1146/annurev-biochem-060109-092838
Shepherd, D. L., Hathaway, Q. A., Pinti, M. V., Nichols, C. E., Durr, A. J., Sreekumar, S., et al. (2017). Exploring the mitochondrial microRNA import pathway through Polynucleotide Phosphorylase (PNPase). J. Mol. Cell. Cardiol. 110, 15–25. doi: 10.1016/j.yjmcc.2017.06.012
Shi, Q. L., Gibson, G. E. (2011). Up-regulation of the mitochondrial malate dehydrogenase by oxidative stress is mediated by miR-743a. J. Neurochem. 118 (3), 440–448. doi: 10.1111/j.1471-4159.2011.07333.x
Shi, Y. H., Downes, M., Xie, W., Kao, H. Y., Ordentlich, P., Tsai, C. C., et al. (2001). Sharp, an inducible cofactor that integrates nuclear receptor repression and activation. Genes Dev. 15 (9), 1140–1151. doi: 10.1101/gad.871201
Shinde, S., Bhadra, U. (2015). A complex genome-microRNA interplay in human mitochondria. BioMed. Res. Int. 2015, 206382. doi: 10.1155/2015/206382
Simsek, D., Tiu, G. C., Flynn, R. A., Byeon, G. W., Leppek, K., Xu, A. F., et al. (2017). The Mammalian Ribo-interactome reveals ribosome functional diversity and heterogeneity. Cell 169 (6), 1051–1065 e1018. doi: 10.1016/j.cell.2017.05.022
Singh, R., Saini, N. (2012). Downregulation of BCL2 by miRNAs augments drug-induced apoptosis–a combined computational and experimental approach. J. Cell Sci. 125 (Pt 6), 1568–1578. doi: 10.1242/jcs.095976
Siomi, M. C., Sato, K., Pezic, D., Aravin, A. A. (2011). PIWI-interacting small RNAs: the vanguard of genome defence. Nat. Rev. Mol. Cell Biol. 12 (4), 246–258. doi: 10.1038/nrm3089
Sirey, T. M., Roberts, K., Haerty, W., Bedoya-Reina, O., Rogatti-Granados, S., Tan, J. Y., et al. (2019). The long non-coding RNA Cerox1 is a post transcriptional regulator of mitochondrial complex I catalytic activity. Elife 8, e45051. doi: 10.7554/eLife.45051
Slavoff, S. A., Mitchell, A. J., Schwaid, A. G., Cabili, M. N., Ma, J., Levin, J. Z., et al. (2013). Peptidomic discovery of short open reading frame-encoded peptides in human cells. Nat. Chem. Biol. 9 (1), 59–64. doi: 10.1038/nchembio.1120
Smirnov, A., Tarassov, I., Mager-Heckel, A. M., Letzelter, M., Martin, R. P., Krasheninnikov, I. A., et al. (2008). Two distinct structural elements of 5S rRNA are needed for its import into human mitochondria. Rna-a Publ. RNA Soc. 14 (4), 749–759. doi: 10.1261/rna.952208
Smirnov, A., Comte, C., Mager-Heckel, A. M., Addis, V., Krasheninnikov, I. A., Martin, R. P., et al. (2010). Mitochondrial Enzyme Rhodanese is essential for 5 S Ribosomal RNA import into human Mitochondria. J. Biol. Chem. 285 (40), 30792–30803. doi: 10.1074/jbc.M110.151183
Smirnov, A., Entelis, N., Martin, R. P., Tarassov, I. (2011). Biological significance of 5S rRNA import into human mitochondria: role of ribosomal protein MRP-L18. Genes Dev. 25 (12), 1289–1305. doi: 10.1101/gad.624711
Song, M., Rossi, J. J. (2017). Molecular mechanisms of Dicer: endonuclease and enzymatic activity. Biochem. J. 474 (10), 1603–1618. doi: 10.1042/BCJ20160759
Soni, M. S., Rabaglia, M. E., Bhatnagar, S., Shang, J., Ilkayeva, O., Mynatt, R., et al. (2014). Downregulation of carnitine acyl-carnitine translocase by miRNAs 132 and 212 amplifies glucose-stimulated insulin secretion. Diabetes 63 (11), 3805–3814. doi: 10.2337/db13-1677
Spinelli, J. B., Haigis, M. C. (2018). The multifaceted contributions of mitochondria to cellular metabolism. Nat. Cell Biol. 20 (7), 745–754. doi: 10.10138/s41556-018-0124-1
Sreekumar, P. G., Ishikawa, K., Spee, C., Mehta, H. H., Wan, J. X., Yen, K., et al. (2016). The Mitochondrial-derived peptide humanin protects RPE cells from Oxidative stress, senescence, and Mitochondrial Dysfunction. Invest. Ophthalmol. Visual Sci. 57 (3), 1238–1253. doi: 10.1167/iovs.15-17053
Sripada, L., Tomar, D., Prajapati, P., Singh, R., Singh, A. K., Singh, R. (2012). Systematic analysis of small RNAs associated with human mitochondria by deep sequencing: detailed analysis of mitochondrial associated miRNA. PloS One 7 (9), e44873. doi: 10.1371/journal.pone.0044873
Stein, C. S., Jadiya, P., Zhang, X. M., McLendon, J. M., Abouassaly, G. M., Witmer, N. H., et al. (2018). Mitoregulin: a lncRNA-Encoded microprotein that supports mitochondrial supercomplexes and respiratory efficiency. Cell Rep. 23 (13), 3710–3720. doi: 10.1016/j.celrep.2018.06.002
Stone, N., Pangilinan, F., Molloy, A. M., Shane, B., Scott, J. M., Ueland, P. M., et al. (2011). Bioinformatic and genetic association analysis of MicroRNA target sites in one-carbon metabolism genes. PloS One 6 (7), e21851. doi: 10.1371/journal.pone.0021851
Sun, Y., Li, M. (2019). New insights into long non-coding RNA MALAT1 in cancer and metastasis. Cancers (Basel). 11 (2), 216. doi: 10.3390/cancers11020216
Sun, L. L., Jiang, B. G., Li, W. T., Zou, J. J., Shi, Y. Q., Liu, Z. M. (2011). MicroRNA-15a positively regulates insulin synthesis by inhibiting uncoupling protein-2 expression. Diabetes Res. Clin. Pract. 91 (1), 94–100. doi: 10.1016/j.diabres.2010.11.006
Sun, Y. M., Lin, K. Y., Chen, Y. Q. (2013). Diverse functions of miR-125 family in different cell contexts. J. Hematol. Oncol. 6, 6. doi: 10.1186/1756-8722-6-6
Szymanski, M., Barciszewska, M. Z., Zywicki, M., Barciszewski, J. (2003). Noncoding RNA transcripts. J. Appl. Gen. 44 (1), 1–19.
Tak, H., Kim, J., Jayabalan, A. K., Lee, H., Kang, H., Cho, D. H., et al. (2014). miR-27 regulates mitochondrial networks by directly targeting the mitochondrial fission factor. Exp. Mol. Med. 46, e123. doi: 10.1038/emm.2014.73
Tarassov, I. A., Martin, R. P. (1996). Mechanisms of tRNA import into yeast mitochondria: an overview. Biochimie 78 (6), 502–510. doi: 10.1016/0300-9084(96)84756-0
Tarassov, I., Kamenski, P., Kolesnikova, O., Karicheva, O., Martin, R. P., Krasheninnikov, I. A., et al. (2007). Import of nuclear DNA-encoded RNAs into mitochondria and mitochondrial translation. Cell Cycle 6 (20), 2473–2477. doi: 10.4161/cc.6.20.4783
Tian, T., Lv, X., Pan, G., Lu, Y., Chen, W., He, W., et al. (2019). Long Noncoding RNA MPRL promotes mitochondrial fission and cisplatin chemosensitivity via disruption of pre-miRNA processing. Clin. Cancer Res. 25 (12), 3673–3688. doi: 10.1158/1078-0432.CCR-18-2739
Tibiche, C., Wang, E. (2008). MicroRNA regulatory patterns on the human metabolic network. Open Syst. Biol. J. 1, 1–8. doi: 10.2174/1876392800801010001
Tilokani, L., Nagashima, S., Paupe, V., Prudent, J. (2018). Mitochondrial dynamics: overview of molecular mechanisms. Mitochondrial Dis. 62 (3), 341–360. doi: 10.1042/Ebc20170104
Tsuchida, A., Ohno, S., Wu, W. H., Borjigin, N., Fujita, K., Aoki, T., et al. (2011). miR-92 is a key oncogenic component of the miR-17-92 cluster in colon cancer. Cancer Sci. 102 (12), 2264–2271. doi: 10.1111/j.1349-7006.2011.02081.x
Uchida, S., Dimmeler, S. (2015). Long Noncoding RNAs in cardiovascular diseases. Circ. Res. 116 (4), 737–750. doi: 10.1161/Circresaha.116.302521
Ulitsky, I., Bartel, D. P. (2013). lincRNAs: genomics, evolution, and mechanisms. Cell 154 (1), 26–46. doi: 10.1016/j.cell.2013.06.020
van Heesch, S., Witte, F., Schneider-Lunitz, V., Schulz, J. F., Adami, E., Faber, A. B., et al. (2019). The translational landscape of the human heart. Cell 178 (1), 242–260. doi: 10.1016/j.cell.2019.05.010
van Rooij, E., Quiat, D., Johnson, B. A., Sutherland, L. B., Qi, X. X., Richardson, J. A., et al. (2009). A family of microRNAs encoded by myosin genes governs myosin expression and muscle performance. Dev. Cell 17 (5), 662–673. doi: 10.1016/j.devcel.2009.10.013
Vargas, J. N. S., Kar, A. N., Kowalak, J. A., Gale, J. R., Aschrafi, A., Chen, C. Y., et al. (2016). Axonal localization and mitochondrial association of precursor microRNA 338. Cell. Mol. Life Sci. 73 (22), 4327–4340. doi: 10.1007/s00018-016-2270-6
Vasudevan, S. (2012). Posttranscriptional upregulation by microRNAs. Wiley Interdiscip. Rev. RNA 3 (3), 311–330. doi: 10.1002/wrna.121
Vendramin, R., Marine, J. C., Leucci, E. (2017). Non-coding RNAs: the dark side of nuclear-mitochondrial communication. EMBO J. 36 (9), 1123–1133. doi: 10.15252/embj.201695546
Vendramin, R., Verheyden, Y., Ishikawa, H., Goedert, L., Nicolas, E., Saraf, K., et al. (2018). SAMMSON fosters cancer cell fitness by concertedly enhancing mitochondrial and cytosolic translation. Nat. Struct. Mol. Biol. 25 (11), 1035–103+. doi: 10.1038/s41594-018-0143-4
Vidaurre, S., Fitzpatrick, C., Burzio, V. A., Briones, M., Villota, C., Villegas, J., et al. (2014). Down-regulation of the Antisense Mitochondrial Non-coding RNAs (ncRNAs) is a unique vulnerability of cancer cells and a potential target for cancer therapy. J. Biol. Chem. 289 (39), 27182–27198. doi: 10.1074/jbc.M114.558841
Villegas, J., Zarraga, A. M., Muller, I., Montecinos, L., Werner, E., Brito, M., et al. (2000). A novel chimeric mitochondrial RNA localized in the nucleus of mouse sperm. DNA Cell Biol. 19 (9), 579–588. doi: 10.1089/104454900439809
Villegas, J., Burzio, V., Villota, C., Landerer, E., Martinez, R., Santander, M., et al. (2007). Expression of a novel non-coding mitochondrial RNA in human proliferating cells. Nucleic Acids Res. 35 (21), 7336–7347. doi: 10.1093/nar/gkm863
Vohwinkel, C. U., Lecuona, E., Sun, H., Sommer, N., Vadasz, I., Chandel, N. S., et al. (2011). Elevated CO(2) levels cause mitochondrial dysfunction and impair cell proliferation. J. Biol. Chem. 286 (43), 37067–37076. doi: 10.1074/jbc.M111.290056
Wallace, D. C. (2012). Mitochondria and cancer. Nat. Rev. Cancer 12 (10), 685–698. doi: 10.1038/nrc3365
Wang, Z., Burge, C. B. (2008). Splicing regulation: from a parts list of regulatory elements to an integrated splicing code. RNA 14 (5), 802–813. doi: 10.1261/rna.876308
Wang, X., Wang, X. (2006). Systematic identification of microRNA functions by combining target prediction and expression profiling. Nucleic Acids Res. 34 (5), 1646–1652. doi: 10.1093/nar/gkl068
Wang, G., Chen, H. W., Oktay, Y., Zhang, J., Allen, E. L., Smith, G. M., et al. (2010). PNPASE regulates RNA import into Mitochondria. Cell 142 (3), 456–467. doi: 10.1016/j.cell.2010.06.035
Wang, J. X., Jiao, J. Q., Li, Q. A., Long, B., Wang, K., Liu, J. P., et al. (2011). miR-499 regulates mitochondrial dynamics by targeting calcineurin and dynamin-related protein-1. Nat. Med. 17 (1), 71–U243. doi: 10.1038/nm.2282
Wang, G., Shimada, E., Zhang, J., Hong, J. S., Smith, G. M., Teitell, M. A., et al. (2012a). Correcting human mitochondrial mutations with targeted RNA import. Proc. Natl. Acad. Sci. U. S. A. 109 (13), 4840–4845. doi: 10.1073/pnas.1116792109
Wang, G., Shimada, E., Koehler, C. M., Teitell, M. A. (2012b). PNPASE and RNA trafficking into mitochondria. Biochim. Biophys. Acta 1819 (9-10), 998–1007. doi: 10.1016/j.bbagrm.2011.10.001
Wang, K., Long, B., Jiao, J. Q., Wang, J. X., Liu, J. P., Li, Q., et al. (2012). miR-484 regulates mitochondrial network through targeting Fis1. Nat. Commun. 3, 781. doi: 10.1038/ncomms1770
Wang, K., Long, B., Zhou, L. Y., Liu, F., Zhou, Q. Y., Liu, C. Y., et al. (2014). CARL lncRNA inhibits anoxia-induced mitochondrial fission and apoptosis in cardiomyocytes by impairing miR-539-dependent PHB2 downregulation. Nat. Commun. 5, 3596. doi: 10.1038/ncomms4596
Wang, W. X., Visavadiya, N. P., Pandya, J. D., Nelson, P. T., Sullivan, P. G., Springer, J. E. (2015a). Mitochondria-associated microRNAs in rat hippocampus following traumatic brain injury. Exp. Neurol. 265, 84–93. doi: 10.1016/j.expneurol.2014.12.018
Wang, M., Huang, T., Luo, G., Huang, C., Xiao, X. Y., Wang, L., et al. (2015b). Long non-coding RNA MEG3 induces renal cell carcinoma cells apoptosis by activating the mitochondrial pathway. J. Huazhong Univ. Sci. Technol. Med. Sci. 35 (4), 541–545. doi: 10.1007/s11596-015-1467-5
Wang, D., Eraslan, B., Wieland, T., Hallstrom, B., Hopf, T., Zolg, D. P., et al. (2019). A deep proteome and transcriptome abundance atlas of 29 healthy human tissues. Mol. Syst. Biol. 15 (2), e8503. doi: 10.15252/msb.20188503
Wilfred, B. R., Wang, W. X., Nelson, P. T. (2007). Energizing miRNA research: a review of the role of miRNAs in lipid metabolism, with a prediction that miR-103/107 regulates human metabolic pathways. Mol. Genet. Metab. 91 (3), 209–217. doi: 10.1016/j.ymgme.2007.03.011
Willers, I. M., Martinez-Reyes, I., Martinez-Diez, M., Cuezva, J. M. (2012). miR-127-5p targets the 3′UTR of human beta-F1-ATPase mRNA and inhibits its translation. Biochim. Biophys. Acta 1817 (5), 838–848. doi: 10.1016/j.bbabio.2012.03.005
Wu, C., Gong, Y., Sun, A., Zhang, Y., Zhang, C., Zhang, W., et al. (2013). The human MTHFR rs4846049 polymorphism increases coronary heart disease risk through modifying miRNA binding. Nutr. Metab. Cardiovasc. Dis. 23 (7), 693–698. doi: 10.1016/j.numecd.2012.02.009
Wu, H., Sun, H., Liang, X., Lima, W. F., Crooke, S. T. (2013). Human RNase H1 is associated with protein p32 and is involved in mitochondrial pre-rRNA processing. PloS One 8 (8), e71006. doi: 10.1371/journal.pone.0071006
Wu, Y. T., Huang, C., Meng, X. M., Li, J. (2015). Long Noncoding RNA MALAT1: Insights into its Biogenesis and Implications in Human Disease. Curr. Pharm. Design 21 (34), 5017–5028. doi: 10.2174/1381612821666150724115625
Wu, K. M., Zhao, Z. X., Xiao, Y. L., Peng, J. J., Chen, J. H., He, Y. L. (2016). Roles of mitochondrial transcription factor A and microRNA-590-3p in the development of colon cancer. Mol. Med. Rep. 14 (6), 5475–5480. doi: 10.3892/mmr.2016.5955
Xu, Y., Fang, F., Zhang, J., Josson, S., St Clair, W. H., St Clair, D. K. (2010). miR-17* suppresses tumorigenicity of prostate cancer by inhibiting mitochondrial antioxidant enzymes. PloS One 5 (12), e14356. doi: 10.1371/journal.pone.0014356
Xu, T., Zhang, K., Shi, J., Huang, B., Wang, X., Qian, K., et al. (2019). MicroRNA-940 inhibits glioma progression by blocking mitochondrial folate metabolism through targeting of MTHFD2. Am. J. Cancer Res. 9 (2), 250–269.
Yadav, S., Pandey, A., Shukla, A., Talwelkar, S. S., Kumar, A., Pant, A. B., et al. (2011). miR-497 and miR-302b regulate ethanol-induced neuronal cell death through BCL2 protein and cyclin D2. J. Biol. Chem. 286 (43), 37347–37357. doi: 10.1074/jbc.M111.235531
Yamakuchi, M., Ferlito, M., Lowenstein, C. J. (2008). miR-34a repression of SIRT1 regulates apoptosis. Proc. Natl. Acad. Sci. U. S. A 105 (36), 13421–13426. doi: 10.1073/pnas.0801613105
Yamamoto, H., Morino, K., Nishio, Y., Ugi, S., Yoshizaki, T., Kashiwagi, A., et al. (2012). MicroRNA-494 regulates mitochondrial biogenesis in skeletal muscle through mitochondrial transcription factor A and Forkhead box j3. Am. J. Physiol. Endocrinol. Metab. 303 (12), E1419–E1427. doi: 10.1152/ajpendo.00097.2012
Yang, K. C., Yamada, K. A., Patel, A. Y., Topkara, V. K., George, I., Cheema, F. H., et al. (2014). Deep RNA sequencing reveals dynamic regulation of Myocardial noncoding RNAs in failing human heart and remodeling with mechanical circulatory support. Circulation 129 (9), 1009–1021. doi: 10.1161/Circulationaha.113.003863
Yao, J., Zhou, E. X., Wang, Y. C., Xu, F., Zhang, D. H., Zhong, D. W. (2014). microRNA-200a inhibits cell proliferation by targeting mitochondrial transcription factor A in breast cancer. DNA and Cell Biol. 33 (5), 291–300. doi: 10.1089/dna.2013.2132
Yao, Q., Chen, Y. Q., Zhou, X. (2019). The roles of microRNAs in epigenetic regulation. Curr. Opin. In Chem. Biol. 51, 11–17. doi: 10.1016/j.cbpa.2019.01.024
Yeasmin, F., Yada, T., Akimitsu, N. (2018). Micropeptides encoded in transcripts previously identified as long noncoding RNAs: a new chapter in transcriptomics and proteomics. Front. In Genet. 9, 144. doi: 10.3389/fgene.2018.00144
Yen, K., Lee, C., Mehta, H., Cohen, P. (2013). The emerging role of the mitochondrial-derived peptide humanin in stress resistance. J. Mol. Endocrinol. 50 (1), R11–R19. doi: 10.1530/Jme-12-0203
Yoshionari, S., Koike, T., Yokogawa, T., Nishikawa, K., Ueda, T., Miura, K., et al. (1994). Existence of nuclear-encoded 5S-rRNA in bovine mitochondria. FEBS Lett. 338 (2), 137–142. doi: 10.1016/0014-5793(94)80351-x
Zelenka, J., Alan, L., Jezek, P. (2012). Import of fluorescent RNA into mitochondria of living cells. Biochim. Et Biophys. Acta-Bioenerg. 1817, S143–S143. doi: 10.1016/j.bbabio.2012.06.377
Zhai, H., Song, B., Xu, X., Zhu, W., Ju, J. (2013). Inhibition of autophagy and tumor growth in colon cancer by miR-502. Oncogene 32 (12), 1570–1579. doi: 10.1038/onc.2012.167
Zhang, J. G., Wang, J. J., Zhao, F., Liu, Q., Jiang, K., Yang, G. H. (2010). MicroRNA-21 (miR-21) represses tumor suppressor PTEN and promotes growth and invasion in non-small cell lung cancer (NSCLC). Clin. Chim. Acta 411 (11-12), 846–852. doi: 10.1016/j.cca.2010.02.074
Zhang, X., Zuo, X., Yang, B., Li, Z., Xue, Y., Zhou, Y., et al. (2014). MicroRNA directly enhances mitochondrial translation during muscle differentiation. Cell 158 (3), 607–619. doi: 10.1016/j.cell.2014.05.047
Zhang, R., Zhou, H. M., Jiang, L., Mao, Y. R., Cui, X. M., Xie, B., et al. (2016). MiR-195 dependent roles of mitofusin2 in the mitochondrial dysfunction of hippocampal neurons in SAMP8 mice. Brain Res. 1652, 135–143. doi: 10.1016/j.brainres.2016.09.047
Zhang, B., Yi, J., Zhang, C. L., Zhang, Q. H., Xu, J. F., Shen, H. Q., et al. (2017). MiR-146a inhibits proliferation and induces apoptosis in murine osteoblastic MC3T3-E1 by regulating Bcl2. Eur. Rev. Med. Pharmacol. Sci. 21 (17), 3754–3762.
Zhang, J., Yu, L. M., Xu, Y. L., Liu, Y., Li, Z., Xue, X. D., et al. (2018). Long noncoding RNA upregulated in hypothermia treated cardiomyocytes protects against myocardial infarction through improving mitochondrial function. Int. J. Cardiol. 266, 213–217. doi: 10.1016/j.ijcard.2017.12.097
Zhang, W. C., Wells, J. M., Chow, K. H., Huang, H., Yuan, M., Saxena, T., et al. (2019). miR-147b-mediated TCA cycle dysfunction and pseudohypoxia initiate drug tolerance to EGFR inhibitors in lung adenocarcinoma. Nat. Metab. 1 (4), 460–474. doi: 10.1038/s42255-019-0052-9
Zhao, S. T., Zhao, L., Li, J. H. (2013). Neuroprotective peptide humanin inhibits inflammatory response in Astrocytes induced by Lipopolysaccharide. Neurochem. Res. 38 (3), 581–588. doi: 10.1007/s11064-012-0951-6
Zhao, Y., Li, H., Fang, S., Kang, Y., Wu, W., Hao, Y., et al. (2016). NONCODE 2016: an informative and valuable data source of long non-coding RNAs. Nucleic Acids Res. 44 (D1), D203–D208. doi: 10.1093/nar/gkv1252
Zhao, Y., Sun, L., Wang, R. R., Hu, J. F., Cui, J. (2018). The effects of mitochondria-associated long noncoding RNAs in cancer mitochondria: new players in an old arena. Crit. Rev. Oncol. Hematol. 131, 76–82. doi: 10.1016/j.critrevonc.2018.08.005
Zhao, Y. J., Liu, S. S., Zhou, L., Li, X. L., Meng, Y., Li, Y., et al. (2019). Aberrant shuttling of long noncoding RNAs during the mitochondria-nuclear crosstalk in hepatocellular carcinoma cells. Am. J. Cancer Res. 9 (5), 999–1008.
Zheng, S. Q., Li, Y. X., Zhang, Y., Li, X., Tang, H. (2011). MiR-101 regulates HSV-1 replication by targeting ATP5B. Antiviral Res. 89 (3), 219–226. doi: 10.1016/j.antiviral.2011.01.008
Zheng, P., Xiong, Q., Wu, Y., Chen, Y., Chen, Z., Fleming, J., et al. (2015). Quantitative proteomics analysis reveals novel insights into mechanisms of action of long noncoding RNA Hox transcript antisense intergenic RNA (HOTAIR) in HeLa cells. Mol. Cell Proteomics 14 (6), 1447–1463. doi: 10.1074/mcp.M114.043984
Zheng, Q., Liu, P. P., Gao, G., Yuan, J. P., Wang, P. F., Huang, J. L., et al. (2019). Mitochondrion-processed TERC regulates senescence without affecting telomerase activities. Protein Cell 10 (9), 631–648. doi: 10.1007/s13238-019-0612-5
Zhou, X. H., Zhang, L. S., Zheng, B. C., Yan, Y. C., Zhang, Y., Xie, H. Y., et al. (2016). MicroRNA-761 is upregulated in hepatocellular carcinoma and regulates tumorigenesis by targeting Mitofusin-2. Cancer Sci. 107 (4), 424–432. doi: 10.1111/cas.12904
Zhu, H., Wu, H., Liu, X. P., Li, B. A., Chen, Y., Ren, X. C., et al. (2009). Regulation of autophagy by a beclin 1-targeted microRNA, miR-30a, in cancer cells. Autophagy 5 (6), 816–823. doi: 10.4161/auto.9064
Keywords: mitochondria, ncRNA, lncRNA, miRNA, mtDNA, micropeptide
Citation: Gusic M and Prokisch H (2020) ncRNAs: New Players in Mitochondrial Health and Disease? Front. Genet. 11:95. doi: 10.3389/fgene.2020.00095
Received: 05 November 2019; Accepted: 28 January 2020;
Published: 28 February 2020.
Edited by:
Nejat Dalay, Istanbul University, TurkeyReviewed by:
Girdhari Lal, National Centre for Cell Science, IndiaCopyright © 2020 Gusic and Prokisch. This is an open-access article distributed under the terms of the Creative Commons Attribution License (CC BY). The use, distribution or reproduction in other forums is permitted, provided the original author(s) and the copyright owner(s) are credited and that the original publication in this journal is cited, in accordance with accepted academic practice. No use, distribution or reproduction is permitted which does not comply with these terms.
*Correspondence: Holger Prokisch, cHJva2lzY2hAaGVsbWhvbHR6LW11ZW5jaGVuLmRl
Disclaimer: All claims expressed in this article are solely those of the authors and do not necessarily represent those of their affiliated organizations, or those of the publisher, the editors and the reviewers. Any product that may be evaluated in this article or claim that may be made by its manufacturer is not guaranteed or endorsed by the publisher.
Research integrity at Frontiers
Learn more about the work of our research integrity team to safeguard the quality of each article we publish.