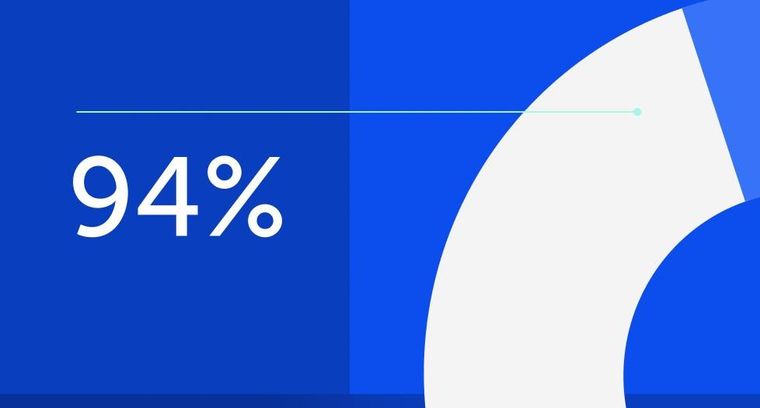
94% of researchers rate our articles as excellent or good
Learn more about the work of our research integrity team to safeguard the quality of each article we publish.
Find out more
MINI REVIEW article
Front. Genet., 13 September 2018
Sec. Genetics of Common and Rare Diseases
Volume 9 - 2018 | https://doi.org/10.3389/fgene.2018.00388
This article is part of the Research TopicModel Organisms: A Precious Resource for Understanding of the Molecular Mechanisms Underlying Human Physiology and DiseaseView all 14 articles
Pyridoxal 5′-phosphate (PLP), the active form of vitamin B6, works as cofactor in numerous enzymatic reactions and it behaves as antioxidant molecule. PLP deficiency has been associated to many human pathologies including cancer and diabetes and the mechanism behind this connection is now becoming clearer. Inadequate intake of this vitamin increases the risk of many cancers; furthermore, PLP deprivation impairs insulin secretion in rats, whereas PLP supplementation prevents diabetic complications and improves gestational diabetes. Growing evidence shows that diabetes and cancer are correlated not only because they share same risk factors but also because diabetic patients have a higher risk of developing tumors, although the underlying mechanisms remain elusive. In this review, we will explore data obtained in Drosophila revealing the existence of a connection between vitamin B6, DNA damage and diabetes, as flies in the past decade turned out to be a promising model also for metabolic diseases including diabetes. We will focus on recent studies that revealed a specific role for PLP in maintaining chromosome integrity and glucose homeostasis, and we will show that these aspects are correlated. In addition, we will discuss recent data identifying PLP as a putative linking factor between diabetes and cancer.
The biologically active form of the vitamin B6, the pyridoxal 5′-phosphate (PLP), acts as coenzyme in about 160 distinct enzymatic activities mainly involved in amino acid, carbohydrate and lipid metabolism, and plays key roles in the synthesis and/or catabolism of certain neurotransmitters (Percudani and Peracchi, 2003; di Salvo et al., 2011). In addition, PLP works as antioxidant molecule by quenching oxygen reactive species (ROS) (Ehrenshaft et al., 1999) and counteracting the formation of Advanced Glycation End products (AGEs), genotoxic compounds associated with senescence and diabetes (Booth et al., 1997). Mammals, differently from microorganisms, are not able to synthesize PLP but they recycle it through a salvage pathway from B6 vitamers as pyridoxal (PL), pyridoxamine (PM), and pyridoxine (PN) contained in food (McCormick and Chen, 1999). In the cytoplasm PL, PM, and PN are converted into the 5′-phosphorylated vitamers by pyridoxal kinase (PDXK), while the FMN-dependent pyridoxine 5′-phosphate oxidase (PNPO) converts PNP and PMP into PLP.
Deficiency of vitamin B6 has been implicated in several clinically relevant diseases including autism, schizophrenia, Alzheimer, Parkinson, epilepsy, Down’s syndrome, diabetes, and cancer. In this review we focus on the role of PLP in diabetes and cancer suggesting more specialist readings for the other pathologies (Hellmann and Mooney, 2010; di Salvo et al., 2012).
Epidemiological studies and meta-analysis indicate an inverse correlation between vitamin B6 and cancer development. For example, high expression levels of PDXK have been positively correlated with survival of non-small cell lung cancer (NSCLC) patients (Galluzzi et al., 2012). Furthermore, vitamin B6 intake and blood PLP levels were inversely correlated with the colorectal cancer risk (Gylling et al., 2017). PLP has been proposed to influence carcinogenesis through different pathways including those involved in DNA metabolism, suggesting that antitumor properties of vitamin B6 may be in part due to its protective role against DNA damage (Ames and Wakimoto, 2002). Vitamin B6 has also been associated to diabetes. However, it is not clear whether low PLP levels represent a cause or an effect of diabetes or both. Some studies report that low PLP levels can contribute to cause diabetes (Toyota et al., 1981; Rubi, 2012), whereas others show that diabetes decreases PLP levels (Bennink and Schreurs, 1975; Spellacy et al., 1977; Okada et al., 1999). Several groups reported that B6 administration produces beneficial effects on diabetic pathology and its complications (Cohen et al., 1984; Solomon and Cohen, 1989; Ellis et al., 1991; Hayakawa and Shibata, 1991; Jain, 2007), although underlying cellular and molecular mechanisms are not completely understood.
Pyridoxal 5′-phosphate deficiency might impact on diabetes in different ways. For example, it could act on the pathway that converts tryptophan into nicotinic acid as PLP is a cofactor of some enzymes that work in this pathway (Bennink and Schreurs, 1975; Spellacy et al., 1977; Oxenkrug, 2013). It has been shown that metabolites produced when this pathway does not work properly can interfere with biological insulin activity (Kotake et al., 1975) causing insulin resistance, a hallmark of type 2 diabetes. Moreover, it has also been proposed that PLP may impact on insulin resistance by controlling the expression of genes involved in adipogenesis (Moreno-Navarrete et al., 2016). Another hypothesis is that PLP deficiency might cause insulin resistance through an increase of homocysteine due to impairment of enzymes such as cystathionine-β-synthase (CBS) and cystathionine-γ-lyase (CGL), which require PLP as a coenzyme (Liu et al., 2016).
Cancer and diabetes are correlated as they share some risk factors. Growing evidence shows that diabetic patients have an increased risk to develop some malignance throughout multiple, not fully elucidate mechanisms, including DNA damage (Noto et al., 2011; Dankner et al., 2016). Interpret the cause-effect relationships in humans is difficult for unavailability of controls and high costs of human research. Researches in the field thus rely on model organisms as well as human 3D cultures and stem cell based systems (Riminucci et al., 2006; Simao et al., 2016). In this review we show how Drosophila has turned out to be a useful model not only to investigate the role of vitamin B6 in cancer and in diabetes but also to connect these two pathologies. Furthermore, we present evidence from flies suggesting that incorrect PLP intake could represent a cancer risk factor for diabetic patients, as it enhances DNA damage.
Using Drosophila as a model system we demonstrated that PLP plays a crucial role in genome integrity maintenance (Marzio et al., 2014). Drosophila dPdxk gene encodes the ortholog of the PDXK enzyme, which is required for vitamin B6 biosynthesis. Mutations in dPdxk gene produce, in larval neuroblasts, chromosome aberrations (CABs) (∼6 vs. 0.5% in controls), which are fully rescued by PLP. Dividing larval neuroblasts represent a suitable system to study CABs in Drosophila as they exhibit morphologically well defined chromosomes that can be stained by a variety of procedures (Gatti and Goldberg, 1991). CABs have been previously observed after X-ray treatment (Gatti et al., 1974) and as a consequence of mutations in genes which control chromatin structure (Mengoli et al., 2014) or different steps of DNA repair (Bianchi et al., 2017; Merigliano et al., 2017). Vitamin B6 antagonists, namely 4-deoxypyridoxine hydrochloride (4-DP), penicillamine, cycloserine, or isoniazid, produce high CAB frequencies (ranging from 3 to 19%) in wild type cells, further confirming that PLP plays an essential role in genome integrity maintenance. The aforementioned function is evolutionarily conserved in humans as the depletion of the human PDXK counterpart induces CABs and a copy of the human PDXK gene inserted in a Drosophila dPdxk1 background is capable to rescue CABs (Marzio et al., 2014). Also in Saccharomyces cerevisiae mutations in BUD16 gene, encoding PDXK, result in gross chromosomal rearrangements. Altogether these data support the hypothesis that low PLP levels may promote cancer initiation and progression throughout the formation of CABs, which represent a cancer prerequisite (Mitelman et al., 2007; Aguilera and Gomez-Gonzalez, 2008; Bunting and Nussenzweig, 2013).
We obtained evidence that in Drosophila PLP is involved in glucose metabolism as dPdxk1 mutants display, in their hemolymph, higher glucose concentrations compared to wild type individuals. dPdxk1 mutants have normal insulin levels, but a weakened ability to respond to insulin signaling (Marzio et al., 2014). Remarkably, in dPdxk1 mutants, high glucose levels and CABs are correlated. In dPdxk1 mutant brains, indeed, 1% glucose in vitro treatment strongly increases CAB frequency (from 6 to 20%); in contrast sugar treatment of wild type larvae and brains did not result in detectable effects on chromosome integrity. The relationship between glucose and CABs, in PLP depleted cells, is evolutionarily conserved as glucose supplementation enhances chromosome damage also in PDXK depleted HeLa cells (Marzio et al., 2014). In addition, the wild type PDXK human gene, inserted in dPdxk1 flies, is able to reduce hyperglycemia. Hyperglycemia triggers the formation of AGEs that in turn produces ROS, which are harmful for DNA. It has been shown that ROS, even at low levels, can cause DNA damage that further leads to DNA double strands breaks (DSBs) (Sharma et al., 2016) and that CABs are mainly generated by unrepaired or improperly repaired DSBs. Repairing complex DSBs may result in genomic instability that can be involved in the etiology of a wide variety of human diseases including cancer (Khanna and Jackson, 2001; Kasparek and Humphrey, 2011).
dPdxk1 mutant cells accumulate AGEs and treatment of dPdxk1 mutants with alpha lipoic acid (ALA), a known AGE inhibitor, rescues not only AGEs but also CABs, suggesting that PLP protects from DNA damage Drosophila cells by counteracting AGE formation (Marzio et al., 2014). To the best of our knowledge only our work (Marzio et al., 2014) showed the cause effect relationship between AGEs and CABs in flies. However, Drosophila represents a good model to study AGEs as flies accumulate significant AGEs over their lifespan (Oudes et al., 1998) and, in addition, an AGE-rich diet results in ROS accumulation (Tsakiri et al., 2013). AGE formation is at the basis of many diabetic complications (Thorpe and Baynes, 1996; Brownlee, 2001; Vlassara and Palace, 2002) and it also can contribute to diabetes onset (Vlassara and Uribarri, 2014). Our data are consistent with studies indicating that vitamin B6 is beneficial for diabetes complication as, for example, nephropathy (Hayakawa and Shibata, 1991) and retinopathy (Ellis et al., 1991) and with in vivo studies showing that PLP is able to reduce AGE accumulation and protein glycation (Cohen et al., 1984; Solomon and Cohen, 1989). How PLP counteracts AGEs is not completely understood but it has been proposed that it may trap 3 deoxyglucosone (3-DG), an AGE’s metabolism intermediate (Nakamura et al., 2007), although other mechanisms are possible. Besides to its antioxidant role, PLP also works as cofactor for serine hydroxymethyltransferase enzyme, which takes part to the thymidylate synthase cycle by converting dUMP in dTMP (Florio et al., 2011). However, whereas PLP depletion in yeast compromises DNA synthesis (Kanellis et al., 2007), in Drosophila it does not seems to have the same effect. Although in dPdxk1 mutants there is an altered dTMP/dUTP ratio DNA syntesis is not the main cause of CABs as dPdxk1 mutant are only slighty sensitive to Hydroxyurea, a drug that interferes with replication (Marzio et al., 2014). However, considering the wide range of enzymatic reactions regulated by vitamin B6, we cannot exclude that in addition to block AGE formation PLP may prevent CABs also through other mechanisms.
Drosophila represents a good model to study diabetes as flies and humans largely share mechanisms involved in glucose homeostasis maintenance (Graham and Pick, 2017). In addition, fly genome possess well characterized orthologs of most genes working in the insulin signaling pathway that controls the glucose uptake and storage (Garofalo, 2002).
In humans and mice mutations in insulin pathway genes cause severe insulin resistance syndromes and type 2 diabetes (reviewed in Boucher et al., 2014). In Drosophila type 2 diabetes models can be generated by two different strategies: by downregulating conserved genes working on insulin pathways as for example the insulin receptor InR, the insulin substrate receptor chico/IRS1, Akt1, PI3K, and by feeding flies with a high sugar rich diet (Alfa and Kim, 2016). In both cases resulting flies exhibit diabetic hallmarks as hyperglycemia and insulin resistance allowing the study in flies of various aspects of diabetes and related human disorders. In addition, a diabetic fly model also enhances the ability to identify genes and discover functional interactions that can be exploited for disease treatment.
Meta-analysis and epidemiological studies indicate that diabetic patients have an increased risk to develop several solid and hematologic malignancies (including liver, pancreas, colorectal, kidney, bladder, endometrial, and breast cancers, and non-Hodgkin’s lymphoma) although the molecular mechanisms are not completely clarified (Vigneri, 2009; Noto et al., 2011; Dankner et al., 2016). However, some risk factors have been identified including hyperinsulinemia and hyperglycemia that might rise cancer risk in diabetic patients by promoting cell growth (Shikata et al., 2013). Besides triggering cell division hyperglycemia also causes oxidative stress as glucose in excess promotes, through different pathways, ROS formation which in turn induces DNA and cellular damage (Rains and Jain, 2011). In addition, in cells from diabetic patients an impaired DNA repair, combined to a weakened antioxidant defense, contributes to enhance DNA damage (Blasiak et al., 2004). Consistently, oxidative damage and DNA strand breaks have been found in both type 1 and type 2 diabetic patients (Goodarzi et al., 2010; Tatsch et al., 2012; Anand et al., 2014). We have recently shown in Drosophila that PLP deficiency can further increase DNA damage in cells from diabetic individuals (Merigliano et al., 2018). Using two different type 2 diabetes models, the first obtained by downregulating genes involved in insulin signaling such as InR, chico (IRS1), and Akt1, and the second by feeding wild type flies with a high sugar diet (Musselman et al., 2011), we showed that the treatment of larval neuroblasts with the strong PLP inhibitor 4-DP produced a very high CAB frequency ranging from 60 to 80% (vs. 25% in wild type cells). Accordingly, genetic analysis revealed a synergistic interaction between Akt1 and dPdkx1 mutations in CAB formation (Merigliano et al., 2018). AGEs are in part responsible for CABs in Drosophila diabetic PLP depleted cells as they accumulate in these cells and, more strikingly, ALA rescues either AGEs and CABs (Merigliano et al., 2018). These findings indicate that, in diabetic cells, low PLP levels heavily impact on genome integrity. Thus, if translated to humans, these data suggest that low PLP levels may contribute to increase cancer risk in diabetic patients. Although PLP deficiency is a rare condition caused by excessive alcohol consumption, unwanted effects of some drugs (i.e., isonyazide, cycloserine penicillamine), or celiac disease and renal dialysis (Clayton, 2006), it has been demonstrated, in murine models and by epidemiological studies, that it can also be associated to diabetes (Leklem and Hollenbeck, 1990; Okada et al., 1999; Ahn et al., 2011; Nix et al., 2015). All evidence suggests the importance to maintain under strict control PLP levels in diabetic patients to avoid the chance to increase DNA damage, which could in turn contribute to cancer initiation and progression.
Several studies have shown that insufficient intake of vitamin B6 is associated with increased cancer risk and growing evidence indicates that diabetes patients have a higher risk of developing various types of cancer. The findings reviewed here, obtained in Drosophila, provide a mechanistic link between aforementioned studies by suggesting that PLP deficiency accompanied by hyperglycemia can lead to DNA damage and may contribute to cancerogenesis. Thus, Drosophila has proved to be a useful model system to shed light on a novel and important role of vitamin B6 deficiency in the pathogenesis of cancer and diabetes. In addition, this model organism allowed identifying PLP deficiency as one of the risk factors that contribute to correlating diabetes to cancer.
CM contributed to planning and writing the manuscript. EM and RB contributed to planning the study. IS contributed to writing the manuscript. FV planned the study and wrote the manuscript. All authors performed a critical revision of the manuscript.
The work was supported by MIUR Ateneo to FV and MIUR Avvio alla ricerca to CM.
The authors declare that the research was conducted in the absence of any commercial or financial relationships that could be construed as a potential conflict of interest.
The reviewer RC declared a shared affiliation, with no collaboration, with the authors to the handling Editor at time of review.
Aguilera, A., and Gomez-Gonzalez, B. (2008). Genome instability: a mechanistic view of its causes and consequences. Nat. Rev. Genet. 9, 204–217. doi: 10.1038/nrg2268
Ahn, H. J., Min, K. W., and Cho, Y. O. (2011). Assessment of vitamin B(6) status in Korean patients with newly diagnosed type 2 diabetes. Nutr. Res. Pract. 5, 34–39. doi: 10.4162/nrp.2011.5.1.34
Alfa, R. W., and Kim, S. K. (2016). Using drosophila to discover mechanisms underlying type 2 diabetes. Dis. Model. Mech. 9, 365–376. doi: 10.1242/dmm.023887
Ames, B. N., and Wakimoto, P. (2002). Are vitamin and mineral deficiencies a major cancer risk? Nat. Rev. Cancer 2, 694–704. doi: 10.1038/nrc886
Anand, S., Nath, B., and Saraswathy, R. (2014). Diabetes–increased risk for cancers through chromosomal aberrations? Asian Pac. J. Cancer Prev. 15, 4571–4573.
Bennink, H. J., and Schreurs, W. H. (1975). Improvement of oral glucose tolerance in gestational diabetes by pyridoxine. Br. Med. J. 3, 13–15. doi: 10.1136/bmj.3.5974.13
Bianchi, F. T., Tocco, C., Pallavicini, G., Liu, Y., Verni, F., Merigliano, C., et al. (2017). Citron kinase deficiency leads to chromosomal instability and TP53-sensitive microcephaly. Cell Rep. 18, 1674–1686. doi: 10.1016/j.celrep.2017.01.054
Blasiak, J., Arabski, M., Krupa, R., Wozniak, K., Zadrozny, M., Kasznicki, J., et al. (2004). DNA damage and repair in type 2 diabetes mellitus. Mutat. Res. 554, 297–304. doi: 10.1016/j.mrfmmm.2004.05.011
Booth, A. A., Khalifah, R. G., Todd, P., and Hudson, B. G. (1997). In vitro kinetic studies of formation of antigenic advanced glycation end products (AGEs). novel inhibition of post-amadori glycation pathways. J. Biol. Chem. 272, 5430–5437. doi: 10.1074/jbc.272.9.5430
Boucher, J., Kleinridders, A., and Kahn, C. R. (2014). Insulin receptor signaling in normal and insulin-resistant states. Cold Spring Harb. Perspect. Biol. 6:a009191. doi: 10.1101/cshperspect.a009191
Brownlee, M. (2001). Biochemistry and molecular cell biology of diabetic complications. Nature 414, 813–820. doi: 10.1038/414813a
Bunting, S. F., and Nussenzweig, A. (2013). End-joining, translocations and cancer. Nat. Rev. Cancer 13, 443–454. doi: 10.1038/nrc3537
Clayton, P. T. (2006). B6-responsive disorders: a model of vitamin dependency. J. Inherit. Metab. Dis. 29, 317–326. doi: 10.1007/s10545-005-0243-2
Cohen, K. L., Gorecki, G. A., Silverstein, S. B., Ebersole, J. S., and Solomon, L. R. (1984). Effect of pyridoxine (vitamin B6) on diabetic patients with peripheral neuropathy. J. Am. Podiatry Assoc. 74, 394–397. doi: 10.7547/87507315-74-8-394
Dankner, R., Boffetta, P., Balicer, R. D., Boker, L. K., Sadeh, M., Berlin, A., et al. (2016). Time-Dependent risk of cancer after a diabetes diagnosis in a cohort of 2.3 Million adults. Am. J. Epidemiol. 183, 1098–1106. doi: 10.1093/aje/kwv290
di Salvo, M. L., Contestabile, R., and Safo, M. K. (2011). Vitamin B(6) salvage enzymes: mechanism, structure and regulation. Biochim. Biophys. Acta 1814, 1597–1608. doi: 10.1016/j.bbapap.2010.12.006
di Salvo, M. L., Safo, M. K., and Contestabile, R. (2012). Biomedical aspects of pyridoxal 5′-phosphate availability. Front. Biosci. 4, 897–913.
Ehrenshaft, M., Bilski, P., Li, M. Y., Chignell, C. F., and Daub, M. E. (1999). A highly conserved sequence is a novel gene involved in de novo vitamin B6 biosynthesis. Proc. Natl. Acad. Sci. U.S.A. 96, 9374–9378. doi: 10.1073/pnas.96.16.9374
Ellis, J. M., Folkers, K., Minadeo, M., VanBuskirk, R., Xia, L. J., and Tamagawa, H. (1991). A deficiency of vitamin B6 is a plausible molecular basis of the retinopathy of patients with diabetes mellitus. Biochem. Biophys. Res. Commun. 179, 615–619. doi: 10.1016/0006-291X(91)91416-A
Florio, R., di Salvo, M. L., Vivoli, M., and Contestabile, R. (2011). Serine hydroxymethyltransferase: a model enzyme for mechanistic, structural, and evolutionary studies. Biochim. Biophys. Acta 1814, 1489–1496. doi: 10.1016/j.bbapap.2010.10.010
Galluzzi, L., Vitale, I., Senovilla, L., Olaussen, K. A., Pinna, G., Eisenberg, T., et al. (2012). Prognostic impact of vitamin B6 metabolism in lung cancer. Cell Rep. 2, 257–269. doi: 10.1016/j.celrep.2012.06.017
Garofalo, R. S. (2002). Genetic analysis of insulin signaling in Drosophila. Trends Endocrinol. Metab. 13, 156–162. doi: 10.1016/S1043-2760(01)00548-3
Gatti, M., and Goldberg, M. L. (1991). Mutations affecting cell division in Drosophila. Methods Cell Biol. 35, 543–586. doi: 10.1016/S0091-679X(08)60587-7
Gatti, M., Tanzarella, C., and Olivieri, G. (1974). Variation with sex of irradiation-induced chromosome damage in somatic cells of Drosophila melanogaster. Nature 247, 151–152. doi: 10.1038/247151a0
Goodarzi, M. T., Navidi, A. A., Rezaei, M., and Babahmadi-Rezaei, H. (2010). Oxidative damage to DNA and lipids: correlation with protein glycation in patients with type 1 diabetes. J. Clin. Lab. Anal. 24, 72–76. doi: 10.1002/jcla.20328
Graham, P., and Pick, L. (2017). Drosophila as a Model for diabetes and diseases of insulin resistance. Curr. Top. Dev. Biol 121, 397–419. doi: 10.1016/bs.ctdb.2016.07.011
Gylling, B., Myte, R., Schneede, J., Hallmans, G., Haggstrom, J., Johansson, I., et al. (2017). Vitamin B-6 and colorectal cancer risk: a prospective population-based study using 3 distinct plasma markers of vitamin B-6 status. Am. J. Clin. Nutr. 105, 897–904. doi: 10.3945/ajcn.116.139337
Hayakawa, M., and Shibata, M. (1991). The in vitro and in vivo inhibition of protein glycosylation and diabetic vascular basement membrane thickening by pyridoxal-5′-phosphate. J. Nutr. Sci. Vitaminol. 37, 149–159. doi: 10.3177/jnsv.37.149
Hellmann, H., and Mooney, S. (2010). Vitamin B6: a molecule for human health? Molecules 15, 442–459. doi: 10.3390/molecules15010442
Jain, S. K. (2007). Vitamin B6 (pyridoxamine) supplementation and complications of diabetes. Metabolism 56, 168–171. doi: 10.1016/j.metabol.2006.09.002
Kanellis, P., Gagliardi, M., Banath, J. P., Szilard, R. K., Nakada, S., Galicia, S., et al. (2007). A screen for suppressors of gross chromosomal rearrangements identifies a conserved role for PLP in preventing DNA lesions. PLoS Genet. 3:e134. doi: 10.1371/journal.pgen.0030134
Kasparek, T. R., and Humphrey, T. C. (2011). DNA double-strand break repair pathways, chromosomal rearrangements and cancer. Semin. Cell Dev. Biol. 22, 886–897. doi: 10.1016/j.semcdb.2011.10.007
Khanna, K. K., and Jackson, S. P. (2001). DNA double-strand breaks: signaling, repair and the cancer connection. Nat. Genet. 27, 247–254. doi: 10.1038/85798
Kotake, Y., Ueda, T., Mori, T., Igaki, S., and Hattori, M. (1975). Abnormal tryptophan metabolism and experimental diabetes by xanthurenic acid (XA). Acta Vitaminol. Enzymol. 29, 236–239.
Leklem, J. E., and Hollenbeck, C. B. (1990). Acute ingestion of glucose decreases plasma pyridoxal 5′-phosphate and total vitamin B-6 concentration. Am. J. Clin. Nutr. 51, 832–836. doi: 10.1093/ajcn/51.5.832
Liu, Z., Li, P., Zhao, Z. H., Zhang, Y., Ma, Z. M., and Wang, S. X. (2016). Vitamin B6 prevents endothelial dysfunction, insulin resistance, and hepatic lipid accumulation in apoe (-/-) mice fed with high-fat diet. J. Diabetes Res. 2016:1748065. doi: 10.1155/2016/1748065
Marzio, A., Merigliano, C., Gatti, M., and Verni, F. (2014). Sugar and chromosome stability: clastogenic effects of sugars in vitamin B6-deficient cells. PLoS Genet. 10:e1004199. doi: 10.1371/journal.pgen.1004199
McCormick, D. B., and Chen, H. (1999). Update on interconversions of vitamin B-6 with its coenzyme. J. Nutr. 129, 325–327. doi: 10.1093/jn/129.2.325
Mengoli, V., Bucciarelli, E., Lattao, R., Piergentili, R., Gatti, M., and Bonaccorsi, S. (2014). The analysis of mutant alleles of different strength reveals multiple functions of topoisomerase 2 in regulation of Drosophila chromosome structure. PLoS Genet. 10:e1004739. doi: 10.1371/journal.pgen.1004739
Merigliano, C., Marzio, A., Renda, F., Somma, M. P., Gatti, M., and Verni, F. (2017). A role for the twins protein phosphatase (PP2A-B55) in the maintenance of drosophila genome integrity. Genetics 205, 1151–1167. doi: 10.1534/genetics.116.192781
Merigliano, C., Mascolo, E., La Torre, M., Saggio, I., and Verni, F. (2018). Protective role of vitamin B6 (PLP) against DNA damage in Drosophila models of type 2 diabetes. Sci. Rep. 8:11432. doi: 10.1038/s41598-018-29801-z
Mitelman, F., Johansson, B., and Mertens, F. (2007). The impact of translocations and gene fusions on cancer causation. Nat. Rev. Cancer 7, 233–245. doi: 10.1038/nrc2091
Moreno-Navarrete, J. M., Jove, M., Ortega, F., Xifra, G., Ricart, W., Obis, E., et al. (2016). Metabolomics uncovers the role of adipose tissue PDXK in adipogenesis and systemic insulin sensitivity. Diabetologia 59, 822–832. doi: 10.1007/s00125-016-3863-1
Musselman, L. P., Fink, J. L., Narzinski, K., Ramachandran, P. V., Hathiramani, S. S., Cagan, R. L., et al. (2011). A high-sugar diet produces obesity and insulin resistance in wild-type Drosophila. Dis. Model. Mech. 4, 842–849. doi: 10.1242/dmm.007948
Nakamura, S., Li, H., Adijiang, A., Pischetsrieder, M., and Niwa, T. (2007). Pyridoxal phosphate prevents progression of diabetic nephropathy. Nephrol. Dial. Transplant. 22, 2165–2174. doi: 10.1093/ndt/gfm166
Nix, W. A., Zirwes, R., Bangert, V., Kaiser, R. P., Schilling, M., Hostalek, U., et al. (2015). Vitamin B status in patients with type 2 diabetes mellitus with and without incipient nephropathy. Diabetes. Res. Clin. Pract. 107, 157–165. doi: 10.1016/j.diabres.2014.09.058
Noto, H., Tsujimoto, T., Sasazuki, T., and Noda, M. (2011). Significantly increased risk of cancer in patients with diabetes mellitus: a systematic review and meta-analysis. Endocr. Pract. 17, 616–628. doi: 10.4158/EP10357.RA
Okada, M., Shibuya, M., Yamamoto, E., and Murakami, Y. (1999). Effect of diabetes on vitamin B6 requirement in experimental animals. Diabetes Obes. Metab. 1, 221–225. doi: 10.1046/j.1463-1326.1999.00028.x
Oudes, A. J., Herr, C. M., Olsen, Y., and Fleming, J. E. (1998). Age-dependent accumulation of advanced glycation end-products in adult Drosophila melanogaster. Mech. Ageing Dev. 100, 221–229. doi: 10.1016/S0047-6374(97)00146-2
Oxenkrug, G. (2013). Insulin resistance and dysregulation of tryptophan-kynurenine and kynurenine-nicotinamide adenine dinucleotide metabolic pathways. Mol. Neurobiol. 48, 294–301. doi: 10.1007/s12035-013-8497-4
Percudani, R., and Peracchi, A. (2003). A genomic overview of pyridoxal-phosphate-dependent enzymes. EMBO Rep. 4, 850–854. doi: 10.1038/sj.embor.embor914
Rains, J. L., and Jain, S. K. (2011). Oxidative stress, insulin signaling, and diabetes. Free Radic. Biol. Med. 50, 567–575. doi: 10.1016/j.freeradbiomed.2010.12.006
Riminucci, M., Saggio, I., Robey, P. G., and Bianco, P. (2006). Fibrous dysplasia as a stem cell disease. J. Bone Miner. Res. 21(Suppl. 2), 125–131. doi: 10.1359/jbmr.06s224
Rubi, B. (2012). Pyridoxal 5’-phosphate (PLP) deficiency might contribute to the onset of type I diabetes. Med. Hypotheses 78, 179–182. doi: 10.1016/j.mehy.2011.10.021
Sharma, V., Collins, L. B., Chen, T., Herr, N., Takeda, S., Sun, W., et al. (2016). Oxidative stress at low levels can induce clustered DNA lesions leading to NHEJ mediated mutations. Oncotarget 7, 25377–25390. doi: 10.18632/oncotarget.8298
Shikata, K., Ninomiya, T., and Kiyohara, Y. (2013). Diabetes mellitus and cancer risk: review of the epidemiological evidence. Cancer Sci. 104, 9–14. doi: 10.1111/cas.12043
Simao, D., Pinto, C., Fernandes, P., Peddie, C. J., Piersanti, S., Collinson, L. M., et al. (2016). Evaluation of helper-dependent canine adenovirus vectors in a 3D human CNS model. Gene Ther. 23, 86–94. doi: 10.1038/gt.2015.75
Solomon, L. R., and Cohen, K. (1989). Erythrocyte O2 transport and metabolism and effects of vitamin B6 therapy in type II diabetes mellitus. Diabetes Metab. Res. Rev. 38, 881–886.
Spellacy, W. N., Buhi, W. C., and Birk, S. A. (1977). Vitamin B6 treatment of gestational diabetes mellitus: studies of blood glucose and plasma insulin. Am. J. Obstet. Gynecol. 127, 599–602. doi: 10.1016/0002-9378(77)90356-8
Tatsch, E., Bochi, G. V., Piva, S. J., De Carvalho, J. A., Kober, H., Torbitz, V. D., et al. (2012). Association between DNA strand breakage and oxidative, inflammatory and endothelial biomarkers in type 2 diabetes. Mutat. Res. 732, 16–20. doi: 10.1016/j.mrfmmm.2012.01.004
Thorpe, S. R., and Baynes, J. W. (1996). Role of the Maillard reaction in diabetes mellitus and diseases of aging. Drugs Aging 9, 69–77. doi: 10.2165/00002512-199609020-00001
Toyota, T., Kai, Y., Kakizaki, M., Ohtsuka, H., Shibata, Y., and Goto, Y. (1981). The endocrine pancreas in pyridoxine deficient rats. Tohoku J. Exp. Med. 134, 331–336. doi: 10.1620/tjem.134.331
Tsakiri, E. N., Iliaki, K. K., Hohn, A., Grimm, S., Papassideri, I. S., Grune, T., et al. (2013). Diet-derived advanced glycation end products or lipofuscin disrupts proteostasis and reduces life span in Drosophila melanogaster. Free Radic. Biol. Med. 65, 1155–1163. doi: 10.1016/j.freeradbiomed.2013.08.186
Vigneri, R. (2009). Diabetes: diabetes therapy and cancer risk. Nat. Rev. Endocrinol. 5, 651–652. doi: 10.1038/nrendo.2009.219
Vlassara, H., and Palace, M. R. (2002). Diabetes and advanced glycation endproducts. J. Intern. Med. 251, 87–101. doi: 10.1046/j.1365-2796.2002.00932.x
Keywords: vitamin B6, pyridoxal 5′- phosphate, diabetes, chromosome aberrations, Drosophila, AGEs
Citation: Merigliano C, Mascolo E, Burla R, Saggio I and Vernì F (2018) The Relationship Between Vitamin B6, Diabetes and Cancer. Front. Genet. 9:388. doi: 10.3389/fgene.2018.00388
Received: 12 July 2018; Accepted: 29 August 2018;
Published: 13 September 2018.
Edited by:
Maria Grazia Giansanti, Istituto di Biologia e Patologia Molecolari (IBPM), Consiglio Nazionale Delle Ricerche (CNR), ItalyReviewed by:
Roberto Contestabile, Università degli Studi di Roma La Sapienza, ItalyCopyright © 2018 Merigliano, Mascolo, Burla, Saggio and Vernì. This is an open-access article distributed under the terms of the Creative Commons Attribution License (CC BY). The use, distribution or reproduction in other forums is permitted, provided the original author(s) and the copyright owner(s) are credited and that the original publication in this journal is cited, in accordance with accepted academic practice. No use, distribution or reproduction is permitted which does not comply with these terms.
*Correspondence: Isabella Saggio, SXNhYmVsbGEuc2FnZ2lvQFVuaXJvbWExLml0 Fiammetta Vernì, RmlhbW1ldHRhLnZlcm5pQFVuaXJvbWExLml0
Disclaimer: All claims expressed in this article are solely those of the authors and do not necessarily represent those of their affiliated organizations, or those of the publisher, the editors and the reviewers. Any product that may be evaluated in this article or claim that may be made by its manufacturer is not guaranteed or endorsed by the publisher.
Research integrity at Frontiers
Learn more about the work of our research integrity team to safeguard the quality of each article we publish.