- 1Laboratory of Fish Genetics, Institute of Animal Physiology and Genetics, Czech Academy of Sciences, Liběchov, Czechia
- 2Departamento de Genética e Evolução, Universidade Federal de São Carlos, São Carlos, Brazil
- 3Secretaria de Estado de Educação de Mato Grosso (SEDUC-MT), Cuiabá, Brazil
The Erythrinidae family (Teleostei: Characiformes) is a small Neotropical fish group with a wide distribution throughout South America, where Hoplias malabaricus corresponds to the most widespread and cytogenetically studied taxon. This species possesses significant genetic variation, as well as huge karyotype diversity among populations, as reflected by its seven major karyotype forms (i.e., karyomorphs A-G) identified up to now. Although morphological differences in their bodies are not outstanding, H. malabaricus karyomorphs are easily identified by differences in 2n, morphology and size of chromosomes, as well as by distinct evolutionary steps of sex chromosomes development. Here, we performed comparative genomic hybridization (CGH) to analyse both the intra- and inter-genomic status in terms of repetitive DNA divergence among all but one (E) H. malabaricus karyomorphs. Our results indicated that they have close relationships, but with evolutionary divergences among their genomes, yielding a range of non-overlapping karyomorph-specific signals. Besides, male-specific regions were uncovered on the sex chromosomes, confirming their differential evolutionary trajectories. In conclusion, the hypothesis that H. malabaricus karyomorphs are result of speciation events was strengthened.
Introduction
The Erythrinidae family (Teleostei: Characiformes) is a small group of Neotropical fishes with a wide distribution throughout South America. This family currently consists of three well-recognized genera—Erythrinus (Scopoli, 1777), Hoplias (Gill, 1903), and Hoplerythrinus (Gill, 1895) with at least 15 until now recognized species (Oyakawa, 2003; Oyakawa and Mattox, 2009). Erythrinids live in diverse habitats, from small lakes and lagoons to large rivers (Oyakawa, 2003). However, unlike the large migratory Neotropical fishes, they are usually not able to overcome obstacles such as waterfalls and large rapids, due to their sedentary lifestyle (Oyakawa, 2003). This situation may have contributed to reduced gene flow between sub-populations in the same hydrographic basin. Consequently, great genome diversity has been documented within the Erythrinidae family, reflected in the noticeable diversity of karyotypes—particularly in the diploid number (2n), karyotype structure and sex chromosome systems (reviewed in Bertollo, 2007).
Hoplias malabaricus is the most widespread as well as cytogenetically investigated taxon, with analyzed populations from north to south of Brazil, Uruguay, Argentina, and Suriname. Despite its wide distribution, this taxon is characterized by low vagility, tending to constitute small populations, being able to survive under low oxygen conditions and to adapt to new environments (Rantin et al., 1992, 1993; Rios et al., 2002). Such characteristics are probably associated with the significant genetic variation and enormous karyotype diversity evidenced by the seven major karyotype forms (i.e., karyomorphs A-G Bertollo et al., 2000; Cioffi et al., 2012). However, despite rather morphological uniformity of body plan, H. malabaricus karyomorphs are easily distinguished by 2n, morphology and size of chromosomes, as well as by different evolutionary stages of distinct sex chromosome systems, indicating the occurrence of an unrecognized species diversity (Bertollo et al., 2000; Bertollo, 2007). Among the seven karyomorphs examined to date, only those of A and E do not show heteromorphic sex chromosomes. Indeed, a well-differentiated XY sex chromosome system occurs in the karyomorph B, while karyomorphs C and F possess such system in an early state of differentiation and finally, karyomorphs D and G harbor a X1X2Y and XY1Y2 multiple sex system, respectively (reviewed in Freitas et al., 2017). These findings indicate independent origins of sex chromosome systems as evidenced by whole chromosome painting (WCP) data (Cioffi et al., 2013). Altogether, H. malabaricus provides a suited model for evolutionary, cytotaxonomic and biodiversity analyses (for review see Cioffi et al., 2012).
The development of recent molecular methologies has allowed a qualitative improvement on chromosome researches of different biological taxa. Among them, the genomic in situ hybridization (GISH) and comparative genomic hybridization (CGH), originally developed for clinical studies (Kallioniemi et al., 1992), are now successfully applied for several other purposes, such as the identification of parental genomes in hybrids/allopolyploids (Bi and Bogart, 2006; Knytl et al., 2013; Symonová et al., 2013a, 2015; Doležálková et al., 2016; Majtánová et al., 2016), the detection of sex-specific content on homomorphic sex chromosomes (Ezaz et al., 2006; Altmanová et al., 2016; Rovatsos et al., 2016; Freitas et al., 2017) and the genome comparisons among related species (Valente et al., 2009; Symonová et al., 2013b; Majka et al., 2016; Carvalho et al., 2017; Moraes et al., 2017). All these (and many other) studies proved that GISH and CGH technologies, despite representing rather “rough” molecular tools, may be successful in providing clues about the genome evolution, with their resolution being based on the differential distribution of already divergent genome-specific repetitive DNA classes (Kato et al., 2005; Chester et al., 2010), as these sequences are generally highly abundant in eukaryotic genomes and display faster evolutionary rates than the single-copy ones (Charlesworth et al., 1994; Cioffi and Bertollo, 2012; López-Flores and Garrido-Ramos, 2012). Hence, in the present study, we performed analyses including CGH procedures to explore both inter- and intra-genomic divergences (within the range defined above) among H. malabaricus karyomorphs. Our results provided new insigths for better understanding of the ongoing processes of the karyomorph differentiations, as well as their sex chromosome systems.
Materials and Methods
Individuals and Mitotic Chromosome Preparations
Analyzed representatives of H. malabaricus karyomorphs are given in Table 1. The individuals were collected under appropriate authorization of the Brazilian environmental agency ICMBIO/SISBIO (License numbers 48628-2 and 10538-1) and deposited in the fish collection of the Cytogenetic Laboratory, Departamento de Genética e Evolução, Universidade Federal de São Carlos. Mitotic chromosomes were obtained by protocols described in Bertollo et al. (2015). The experiments followed ethical and anesthesia conducts, in accordance with the Ethics Committee on Animal Experimentation of the Universidade Federal de São Carlos (Process number CEUA 1853260315).
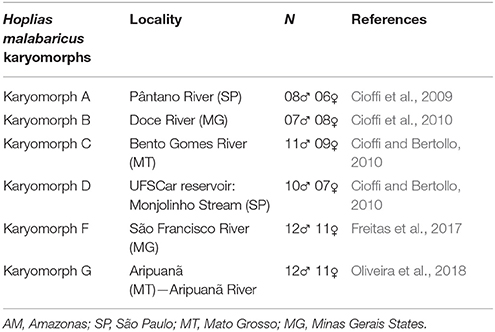
Table 1. Collection sites of Hoplias malabaricus karyomorphs, with the respective localities and sample sizes.
Preparation of Probes for Comparative Genomic Hybridization (CGH)
The total genomic DNAs (gDNAs) from male and female specimens of all karyomorphs listed in Table 1 were extracted from liver tissue by the standard phenol-chloroform-isoamylalkohol method (Sambrook and Russell, 2001). Two different experimental designs were used for this study, as outlined in Figure 1. In the first set of experiments (inter-karyomorph genomic comparisons), the gDNA of karyomorph B male specimens was chosen as a reference due to its well-differentiated XY sex chromosomes and used for hybridization against metaphase chromosomes of the other karyomorphs (Figure 1A). For this purpose, male-derived gDNAs of each karyomorph A, C, D, F and G were labeled with digoxigenin-11-dUTP using DIG-nick-translation Mix (Roche, Mannheim, Germany), while male-derived gDNA of karyomorph B was labeled with biotin-16-dUTP using BIO-nick-translation Mix (Roche). For blocking the repetitive sequences in all experiments, we used unlabeled C0t-1 DNA (i.e., fraction of genomic DNA enriched for highly and moderately repetitive sequences), prepared according to Zwick et al. (1997). Hence, the final probe cocktail for each slide was composed of 500 ng of male-derived gDNA of karyomorph B, 500 ng of male-derived DNA corresponding to one of the comparative karyomorphs, 15 μg of female-derived C0t-1 DNA of karyomorph B and 15 μg of female-derived C0t-1 DNA from the respective comparative karyomorph. The probe was ethanol-precipitated and the dry pellets were resuspended in hybridization buffer containing 50% formamide, 2 × SSC, 10% SDS, 10% dextran sulfate and Denhardt's buffer, pH 7.0. In the second set of experiments (Figure 1B) we focused on intra-karyomorph comparisons, with special emphasis on molecular composition of putative, nascent or well-differentiated sex chromosomes. In this case, male-derived gDNAs of all karyomorphs were labeled with biotin-16-dUTP and female gDNAs with digoxigenin-11-dUTP by means of nick translation as described above. The final hybridization mixture for each slide (20 μl) was composed of male- and female-derived gDNAs (500 ng each), 25 μg of female-derived C0t-1 DNA from the respective karyomorph and the hybridization buffer described above. The chosen ratio of probe vs. C0t-1 DNA amount was based on the experiments performed in our previous studies in fishes including erythrinids (Symonová et al., 2013a,b, 2015; Carvalho et al., 2017; Freitas et al., 2017; Moraes et al., 2017; Yano et al., 2017; Oliveira et al., 2018) and corroborated the ratio used in other related fish studies (e.g., Valente et al., 2009). According to our experiences, this ratio reflects high stringency towards repetitive DNA blocking and yet avoids the probability of improper probe dissolution in the hybridization buffer, which would otherwise cause artifacts.
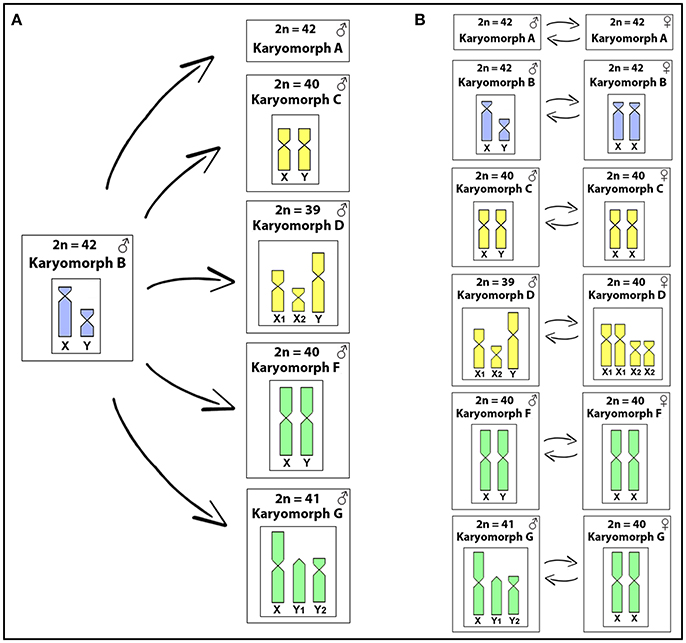
Figure 1. The experimental designs used in this study. In the first one (A), gDNA of karyomorph B male specimens was used for hybridization against chromosomal background of the other karyomorphs (except for karyomorph E), focusing on inter-karyomorph comparisons. In the second set of experiments (B) male- and female-derived gDNAs of each karyomorph under study were hybridized together, focusing on the intra-karyomorph comparisons, with special emphasis on the molecular composition of putative, nascent, or well-differentiated sex chromosomes.
Fish used for CGH
The CGH experiments followed the methodology described in Symonová et al. (2015), with modifications. Briefly, prior to hybridization, slides were aged at 37°C for 2h, followed by an RNAse A (90 min, 37°C) and then pepsin (50 μg/ml in 10 mM HCl, 3 min, 37°C) treatments. Chromosomes were subsequently denatured in 75% formamide (pH 7.0) in 2 × SSC (74°C, 3 min), and then immediately cooled and dehydrated through 70% (cold), 85%, and 100% (RT) ethanol series. The hybridization mixture was denatured at 86°C for 6 min, cooled at 4°C (10 min) and then applied on the slides. The hybridization was performed at 37°C for 72 h. Post-hybridization washes were carried out once in 50% formamide in 2 × SSC (pH 7.0) (44°C, 10 min each) and three times in 1 × SSC (44°C, 7 min each). Prior to probe detection, the slides were incubated with 3% non-fat dried milk (NFDM) in order to avoid the non-specific binding of antibodies. The hybridization signal was detected using Anti-Digoxigenin-Rhodamin (Roche) and Avidin-FITC (Sigma). Chromosomes were counterstained and mounted in antifade containing 1.5 μg/ml DAPI (Vector, Burlingame, CA, USA).
Microscopic Analyses and Image Processing
At least 30 metaphase spreads per individual were analyzed to confirm the 2n, karyotype structure and CGH results. Images were captured using an Olympus BX50 microscope (Olympus Corporation, Ishikawa, Japan), with CoolSNAP and the images were processed using Image Pro Plus 4.1 software (Media Cybernetics, Silver Spring, MD, USA). Chromosome morphology was classified according to Levan et al. (1964).
Results
Inter-Karyomorph Genomic Relationships
In each experiment, both genome-derived probes showed rather equal binding to all chromosomes, with preferential localization in the centromeric and pericentromeric regions of most chromosomes and in terminal parts of some of them (yellow signals, i.e., combination of green and red), indicating the shared repetitive DNA content in such regions. The hybridization pattern in karyomorph A displayed stronger binding of the A-derived probe to the centomeric or telomeric regions of several chromosomes, while the B-derived probe that co-hybridized to these regions, produced signals of less intensity. Moreover, several exclusive A-specific markings appeared mostly in distal chromosomal regions (Figures 2A–D). Similar situation was observed also in karyomorph C (Figures 2E–H), where the majority of the accumulated blocks was shared by both probes, including those in the pericentromeric regions of the XY chromosomes, but several signals and especially those located in the terminal region of the long arms of the largest m pair were found to be accumulated with C-specific probe only. In karyomorph D, stronger binding of the D-derived probe was highlighted in many centromeres, in addition to some telomeric segments (Figures 2I–L). Remarkably, both genomic probes equally stained the heterochromatic block displayed by the neo-Y chromosome. In the karyomorph F, besides the shared binding pattern to majority of heterochromatic blocks, the F-derived probe yielded specific signals on the chromosomal pair bearing NOR-like regions (Figures 2M–P). In addition, the male-specific region on the nascent Y chromosome of this karyomorph displayed some affinity to B-derived male probe, despite being preferentially labeled with the F-male specific one (Figures 2N,O). In comparison to that, the male-specific region of karyomorph G, covering the entire short arms of the Y1 chromosome, was stained almost exclusively by the G-derived probe, while the B-derived probe produced only faint and dispersed signals in this region (Figures 2Q–T). In a similar way, the G-specific probe showed predominant binding to terminally located repetitive blocks on a few other chromosomes.
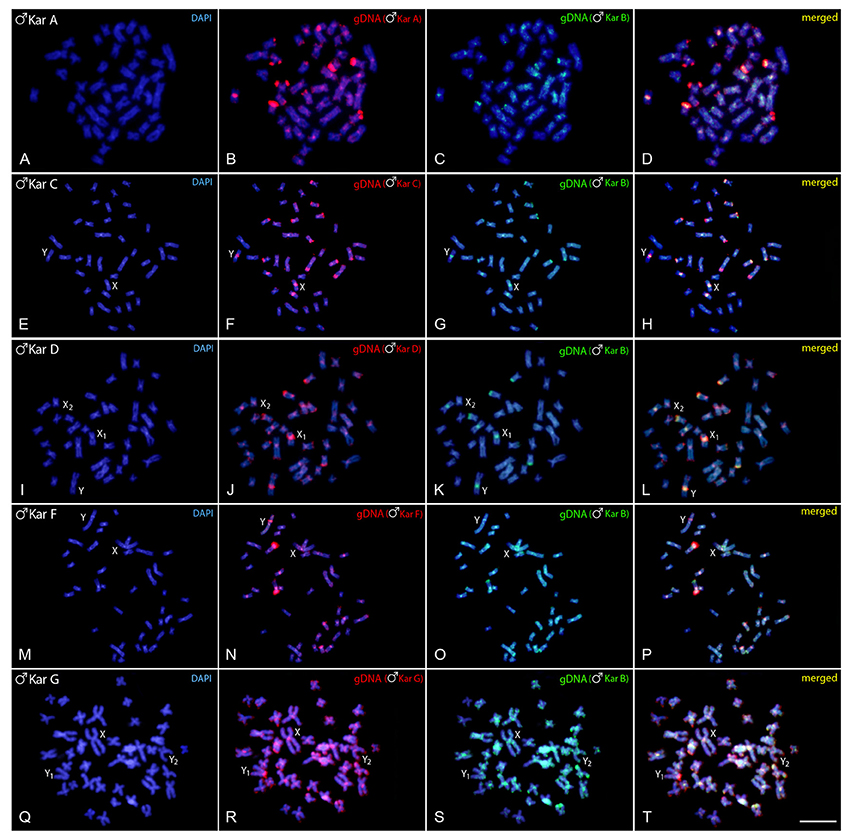
Figure 2. Mitotic chromosome spreads of Hoplias malabaricus males after CGH—interkaryomorph comparison. Male-derived genomic probe from karyomorph B mapped against male chromosomes of karyomorph A (A–D), karyomorph C (E–H), karyomorph D (I–L), karyomorph F (M–P), and karyomorph G (Q–T). First column (A,E,I,M,Q,I): DAPI images (blue); Second column (B,F,J,N,R): hybridization pattern using the male-derived probe (red) of each analyzed karyomorph; Third column (C,G,K,O,S): hybridization pattern using the male-derived probe of karyomorph B (green). Fourth column (D,H,L,P,T): merged images of both genomic probes and DAPI staining. The common genomic regions of both compared karyomorphs are depicted in yellow. Bar = 10 μm.
Intra-Karyomorph Genomic Relationships: Detecting Male-Specific Regions
Experiments performed on female chromosome spreads of karyomorphs B, C, D, F, and G showed the absence of identifiable sex-specific segments.
Regarding karyomorph A, no exclusive male-specific regions were identified on male chromosome complement (Figures 3A–D). In male chromosome spreads of karyomorph B, CGH enabled to recognize male-specific region located terminally on the long arms of the Y chromosome (Figures 3E–H). In karyomorph C, unlike the biased accumulation of repetitive DNAs in the X pericentromeric region (Cioffi and Bertollo, 2010), a slight binding preference for the male-derived probe to the pericentromeric region of Y chromosome was evidenced (Figures 3I–L). Female-derived probe produced only a faint hybridization signal in such region, while both probes matched equally the large heterochromatic segment located in the pericentromeric part of the X chromosome. Accordingly, CGH procedure failed to detect any sex-specific region on male chromosomes of karyomorph D (Figures 3M–P). In karyomorph F, a prominent interstitial band on the metacentric Y chromosome was also enriched with male-specific sequences, although a concurrent faint hybridization signal produced by the female-derived probe was also apparent (Figures 3Q–T). CGH on male preparations from karyomorph G unmasked a clear male-specific region covering the short arms of Y1 chromosome (Figures 3U–X). The summary of observed intra-karyomorph CGH patterns is given in Figure 4.
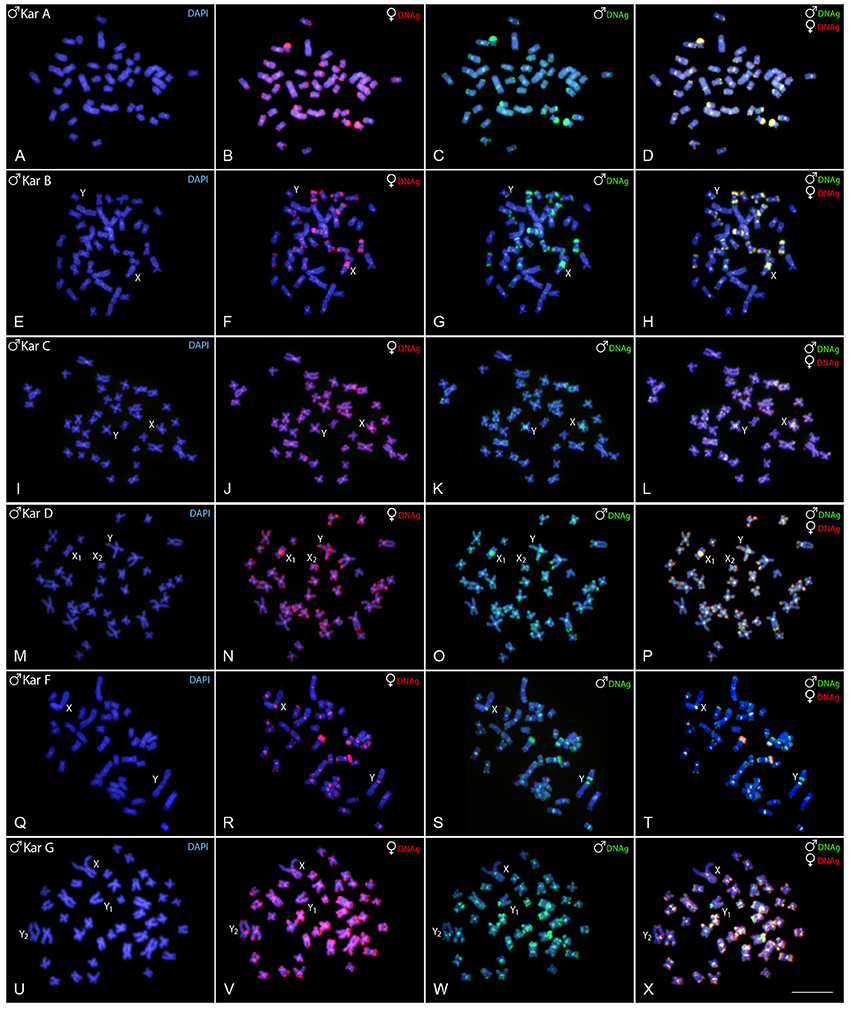
Figure 3. Mitotic chromosome spreads of Hoplias malabaricus males after CGH—intra-karyomorph hybridizations. Male- and female-derived genomic probes hybridized together for each karyomorph. First column (A,E,I,M,Q,U): DAPI images (blue); Second column (B,F,J,N,R,V): hybridization pattern of the female-derived probe (red) of each analyzed karyomorph; Third column (C,G,K,O,S,W): hybridization pattern of the male-derived probe (green) of the respective karyomorph. Fourth column (D,H,L,P,T,X): merged images of both genomic probes and DAPI staining. The common genomic regions for male and female are depicted in yellow. Bar = 10 μm.
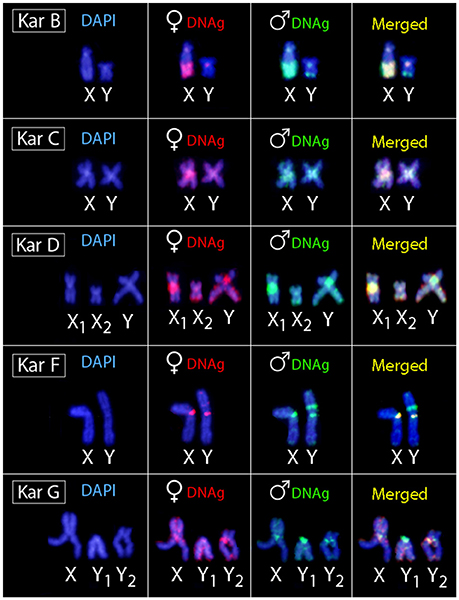
Figure 4. Sex chromosomes of H. malabaricus karyomorphs B, C, D, F and G after CGH procedures. First column: DAPI images; Second column: hybridization pattern using the female-derived probe (red); Third column: hybridization pattern using the male-derived probe (green); Fourth column: merged images of both genomic probes and DAPI staining. Common genomic regions for male and female are depicted in yellow.
Discussion
Genomic Diversity Among Karyomorphs
Sex chromosome systems in H. malabaricus display only male heterogamety and therefore inter-karyomorph genomic comparisons between males were supposed to be informative in indicating their interrelationships. Hence, the male-derived gDNA of reference karyomorph B was probed on chromosomes of karyomorphs A, C, D, F, and G. Even though the B-derived probe showed lower affinity to chromosomes of other karyomorphs, the overall pattern of these experiments was relatively similar. In all experiments, both genome-derived probes showed preferred accumulation to chromosome regions previously identified as C-bands and C0t-1 DNA hybridization sites (Born and Bertollo, 2000; Cioffi and Bertollo, 2010; Cioffi et al., 2010, 2011a), documenting their repetitive DNA content. However, despite less intense, hybridization signals were also apparent along the rest of chromosomal material. Our findings are in line with the general patterns observed in previous GISH/CGH-based reports (e.g., Traut and Winking, 2001; Valente et al., 2009; Koubová et al., 2014; Altmanová et al., 2016) in the sense of biased hybridization in heterochromatic regions and point to the fact that even high amount of C0t-1 DNA is insufficient to outcompete highly repetitive (heterochromatic) regions. Given that the resolution of the CGH procedure predominantly relies on the presence of species-specific (or sex-specific) repetitive sequences, together with the evolutionary distance of the compared genomes (Kato et al., 2005; Chester et al., 2010), our overall results indicate that karyomorphs of H. malabaricus are closely related, but with divergences among their genomes, yielding a range of non-overlapping karyomorph-specific signals. Remarkably, the B-derived probe displayed the lowest degree of hybridization correspondence with karyomorphs D and F, suggesting the ongoing processes of sequence divergence. These findings are indicative of ongoing evolutionary processes driving the divergence and possibly also speciation within H. malabaricus populations, facilitated by the sedentary lifestyle of these fishes (as discussed in detail further in the text).
An array of molecular and cytogenetic methods, including DNA barcoding and phylogeographic approaches, have already led to the hypothesis that H. malabaricus likely represents a “species complex,” with several undescribed species (Bertollo et al., 2000; Santos et al., 2009; Cioffi et al., 2012; Marques et al., 2013). Furthermore, different karyomorphs with a sympatric or syntopic occurrence were found to lack any hybrid forms, as proven by cytogenetic and RAPD (random amplified polymorphic DNA) analyses (Dergam et al., 1998, 2002; Bertollo et al., 2000), yet a sporadic case of hybridization followed by an elevation of the ploidy level was already reported (Utsunomia et al., 2014).
When compared to other members of the Erythrinidae family, similar degree of cytotaxonomic diversity can be found in Erythrinus erythrinus and Hoplerythrinus unitaeniatus groups (Bertollo, 2007; Cioffi et al., 2012; Rosa et al., 2012; Martinez et al., 2015, 2016), while—in stark contrast—the Hoplias lacerdae species complex exhibits highly conserved karyotype structure (Blanco et al., 2011; de Oliveira et al., 2015). It is likely that the chromosomal diversity inside some Erythrinidae species might be associated with their species-specific lifestyles. In this sense, because H. malabaricus, E. erythrinus, and H. unitaeniatus appear to constitute small and restricted populations, with low vagility (Blanco et al., 2011), they experience a higher rate of stochastic fixation of chromosome rearrangements and, consequently, an elevated evolutionary genome dynamism that might contribute to speciation and/or local adaptation processes (see King, 1993; Faria and Navarro, 2010 for an exhaustive discussion). It is therefore remarkable that the three H. malabaricus karyomorphs with more restricted geographic distribution, i.e., the B, D and G ones, possess morphogically recognizable sex chromosomes (Bertollo et al., 2000; Cioffi et al., 2012).
Intra-Karyomorph Genomic Diversity and Male-Specific Sequences
Based on data available from the last comprehensive fish karyotype overview (Arai, 2011), so far only 5% of karyologically analyzed actinopterygian fish species possess heteromorphic sex chromosomes. However, this is very likely an underestimation, with many other well-differentiated or even nascent sex chromosome systems still awaiting their discovery, especially when taking into account that homomorphic (i.e., cytologically indistinguishable or hardly detectable) sex chromosomes are thought to be frequent in fishes (Mank and Avise, 2009; Schartl et al., 2016). Within the Erythrinidae family, three different simple or multiple sex chromosome systems in advanced and/or nascent evolutionary stages have been reported among both E. erythrinus and H. malabaricus karyomorphs (reviewed in Cioffi et al., 2012), where males possess always the heterogametic sex.
Within H. malabaricus group, karyomorph A is characterized by 2n = 42 for both males and females, without an apparent sex chromosome system, while karyomorph B, though also with 2n = 42 for both sexes, exhibits a well-differentiated ♀XX/♂XY sex chromosome system. In this case, the subtelocentric large X chromosome is clearly distinguished from the small-sized metacentric Y, in addition to presence of a conspicuous heterochromatic block distally located on its long arms (Born and Bertollo, 2000; Cioffi et al., 2010). Previous repetitive DNA mapping and WCP data indicate that such sex chromosome system is likely derived from a proto-sex chromosome (the 21st pair of karyomorph A) due enrichment in several types of DNA repeats confined to only one of the homologs, namely the X chromosome in karyomorph B (Cioffi et al., 2010, 2011a,c, 2013). However, CGH procedures did not reveal any sex-specific region in karyomorph A, probably due to low level of sex-specific repetitive DNA divergence or due the small size of the sex-determining region, remaining below the detection limit of the CGH method (that ranges approximately between 2 and 3 Mbp; Schoumans et al., 2004). Theoretically, alternate mechanisms of sex determining region creation such as, e.g., epimutation-coupled recombination suppression (as eloquently discussed in Ezaz and Deakin, 2014) cannot be entirely ruled out and these, again, may have gone undetected through CGH. Finally, the possibility that the sex-determining region in this karyomorph is completely absent seems equally likely. However, the frequent changes of master sex determining genes repeatedly observed in fishes, especially in XX/XY systems (Heule et al., 2014; Martínez et al., 2014), favor the former view as the new non-recombining region is needed to be established again. In support of this view, in several another fish species, the sex determining regions might be very tiny (reviewed in Schartl et al., 2016), with the extreme case of fugu genome, Takifugu rubripes, where the Y-specific sex-determining gene differs from the homologous region on the X chromosome by a single non-synonymous substitution (Kamiya et al., 2012). Moreover, even in the platyfish, Xiphophorus maculatus, with genetically defined sex chromosomes, no visible differences between X and Y were evidenced after CGH (Traut and Winking, 2001), similarly to what had been occasionally observed in some other animals (Koubová et al., 2014; Altmanová et al., 2016; Green et al., 2016; Gazoni et al., 2018). In yet another case, however, CGH proved to be resolute even in sex chromosomes of a very young age (Montiel et al., 2017). Finally, we cannot entirely exclude the possibility that bright fluorescence from major rDNA loci located on several chromosomes of the karyomorph A complement, most probably including the pair no. 21, could disable the detection of the hypothetical sex-determining region in its vicinity. Maybe a finer-scale approach such as BAC FISH mapping of specific candidate genes identified based on utilization of recent genome sequencing approaches and corresponding bioinformatic tools, togehter with other related “state-of-the-art” technologies may shed more light on this issue (for examples in fishes, see: Reichwald et al., 2015; Portela-Bens et al., 2017; Sutherland et al., 2017; Liu et al., 2018).
A male-specific region confined to a distal part of the long arms of Y chromosome was identified in the karyomorph B corresponding to the location of a constitutive heterochromatin block previously described (Born and Bertollo, 2000). The position of this region is noteworthy since a large NOR is found in the corresponding homologous region on the X chromosome, leading to the considerable size difference between both sex chromosomes (Born and Bertollo, 2000). Therefore, we are dealing here with an unusual situation, resembling the findings in weakly electric fish Eigenmannia virescens (de Almeida-Toledo and Foresti, 2001; Henning et al., 2008) or in the snake eel Ophisurus serpens (Salvadori et al., 2018), where the accumulation of rDNA and other repetitive DNAs occurs also on the X instead of Y chromosome. In our specific case, the sex-specific region is present on the corresponding C-positive but NOR-negative (Born and Bertollo, 2000; Cioffi et al., 2010) region on the Y chromosome. It is likely that the differential accumulation of repetitive DNA sequences might have decreased the recombination rate between the sex pair due to their delayed pairing during meiosis (Griffin et al., 2002). Alternatively, the co-amplification of the NOR region with other repetitive DNA sequences on the X chromosome can be viewed as a consequence of the whole differentiation process of the sex pair, helping to buffer the absence of functional rDNA copies on the Y chromosome. In fact, it is noteworthy that the NOR on the X chromosome is always genetically active (Born and Bertollo, 2000). The growing number of reports pointing to sex chromosome-specific NORs (see Kawai et al., 2007; Badenhorst et al., 2013; Yano et al., 2017 for references) possibly indicates that such regions might have played a more relevant role in nascent sex chromosome evolution than currently known.
In karyomorph C (2n = 40, for both sexes), the nascent and morphologically undifferentiated XY sex chromosomes were formerly evidenced by a small accumulation of repetitive DNAs occurring exclusively on the X chromosome (Cioffi and Bertollo, 2010). Here, it is likely that these newly emerging sex-related elements have not had the necessary evolutionary time to evolve and hence accumulated low proportion of tentatively Y-specific sequences Despite that, we cannot rule out that some differentiation in the hybridization pattern of both genomic probes in the pericentromeric region of the nascent Y chromosome is caused by a copy number variation of interspersed repetitive sequences between the sex chromosomes. Finally, it is worth mentioning that B-derived gDNA probe displayed strong binding to this region (on both X and Y) in the inter-karyomorph experiment (see Figures 2F–H), suggesting certain degree of shared sequences, but the extent of overlap with C-derived probe was not absolute.
Karyomorph D (2n = 40 in females/39 in males) is characterized by a X1X2Y multiple sex chromosomes system, where the neo-Y originated via a tandem fusion between the nascent Y chromosome and one autosomal homolog corresponding to the pair No. 20 of karyomorph C (Bertollo et al., 1997; Cioffi and Bertollo, 2010). Indeed, such origin was also confirmed by additional data from inter-karyomorph chromosome painting and mapping of several repetitive DNA classes (Cioffi et al., 2009, 2011b,c). Previous studies on male meiosis showed stabilized pachytene sex trivalents, as well as asynapsis in the region of presumed sequence divergences (Bertollo and Mestriner, 1998), thus pointing to a putative sex-specific region. In favor of this scenario, Rosa et al. (2009) reported noticeable alterations in location of constitutive heterochromatin and 18S rDNA sites on the neo-Y chromosome, indicating that pericentric inversions probably have also taken place in the early process of the sex-specific chromosome differentiation. However, although in karyomorph C, a slight binding preference for the male-derived probe to the pericentromeric region of Y chromosome was observed, our CGH data did not reveal any conspicuous Y-specific region in the neo-sex chromosome system of karyomorph D. In this sense, while in karyomorph D the recombination arrest and the establishment of the stable multiple sex chromosomes was most likely achieved by chromosomal rearrangements, in karyomorph C the accumulation of repetitive DNA sequences seems to have a central role in triggering the differentiation of the nascent XY sex system (Bertollo et al., 1997; Cioffi and Bertollo, 2010).
Karyomorphs E, F, and G were supposed to be closely related (Bertollo et al., 2000). Although the karyomorph E (2n = 42) was not sampled in this study, our results confirmed previous findings in karyomorphs F (Freitas et al., 2017) and G (Oliveira et al., 2018). More specifically, karyomorph F (2n = 40, for both sexes) was found to exhibit a nascent XY sex chromosome system, where the male-specific content was highlighted as a prominent interstitial heterochromatic block on the large metacentric Y chromosome, coincident with several microsatellite motifs and retrotransposons (RTEs) (Freitas et al., 2017, present study). Importantly, as the faint hybridization signal produced by the female-specific probe was allocated also within this region, we witness here a bit similar situation to that found in karyomorph C. If we admit that slightly preferred accumulation of male-exclusive sequences in pericentromeric region of the Y chromosome in males of karyomorph C might be related to the early stage of sex-determining region formation, the observed pattern in karyomorph F may reflect a later phase of similar process. At this stage, the accumulation of repetitive DNA in the Y-specific region in karyomorph F probably involves also the portion of sequences that are common for both sexes.
In contrast to karyomorph F, the sex chromosome system found in karyomorph G (2n = 40 in females/41 in males) is characterized by presence of XY1Y2 chromosomes, where the unusual acrocentric Y1 element carries the male-specific region, enriched with several different types of repetitive DNAs including 5S rDNA (Oliveira et al., 2018), hence strengthening the view discussed above. As initially proposed by Bertollo et al. (2000) and confirmed by the recent findings (Oliveira et al., 2018), the emergence of sex chromosomes in karyomorph G proceeded through a tandem fusion involving chromosomes from two different pairs that might be tentatively assigned to specific pairs in karyomorph E—a hypothetical ancestral karyotype to both F and G karyomorphs. Importantly, while the tandem fusion was fixed in heterozygous condition in karyomorph G as only one homolog from each pair underwent this rearrangement (hence resulting in unpaired large-sized metacentric X chromosome complemented with the remaining unfused Y1 and Y2 chromosomes in males), in karyomorph F both homologs from the mentioned pairs gave rise to two large-sized metacentric chromosomes, the X and Y ones (Freitas et al., 2017; Oliveira et al., 2018, this study). Noteworthy, the XY1Y2 system of the karyomorph G differs from the X1X2Y neo-sex chromosomes of karyomorph D in the way that a sex-determining region is clearly detectable by CGH only in the former case, indicating a different evolutionary stage between such sex systems. All these findings in karyomorphs F and G, i.e., (i) shared homology between their sex chromosomes, pointing to a common origin through tandem fusion and (ii) lack of homology between multiple sex chromosomes found in karyomorphs D and G, are supported also by recent Zoo-FISH experiments (Oliveira et al., 2018).
In summary, our findings support trends in teleost fishes concerning the independent and repeated evolution of sex chromosomes regardless their phylogenetic relationships (Devlin and Nagahama, 2002; Woram et al., 2003; Schartl, 2004; Mank et al., 2006; Mank and Avise, 2009; Cioffi et al., 2013). It has been shown that the sex chromosome systems and/or the stage of their differentiation may differ evidently not only among closely-related species, but also among different populations of the same species (Takehana et al., 2007; Ross et al., 2009; Zhou et al., 2010; Cioffi et al., 2012; Cnaani, 2013). Such an exceptional sex chromosome variability could be possibly associated with the high plasticity and dynamics of teleost genomes (Ravi and Venkatesh, 2008), a feature usually assigned to a specific whole-genome duplication (TSGD) that occurred at the base of teleostean radiation (Hurley et al., 2007). As a consequence, duplicated redundant copies of different genes might have evolved into master sex-determining genes (Matsuda et al., 2002; Nanda et al., 2002), thus leading to emergence of distinct sex chromosomes in different evolutionary lineages (Schartl, 2004; Mank and Avise, 2009). The outstanding pace of sex chromosome turnover is, however, commonly observable also in other cold-blooded vertebrates such as amphibians and reptiles, hence a number of alternative hypotheses about the evolutionary forces standing behind this phenomenon have already been proposed (for recent reviews and in depth discussion, see Mank and Avise, 2009; Kitano and Peichel, 2012; Kikuchi and Hamaguchi, 2013; Bachtrog et al., 2014; Brykov, 2014; Pokorná et al., 2014; Pennell et al., 2015; Schartl et al., 2016). In a broader context, handful of studies have provided direct evidence that the emergence of sex chromosomes, or even the sex chromosome turnover itself, might play a major role in reproductive isolation promoting evolutionary divergences and eventually speciation (e.g., Kitano et al., 2009; Nguyen et al., 2013), which is evidently the case for H. malabaricus.
Conclusion
Our data provided additional layer of evidence about the status of the taxon H. malabaricus and corroborated previous studies in the conclusion that it includes taxonomically distinct species. The CGH procedures proved to be very useful in detecting the hidden biodiversity in this fish group, as they have opened novel views and widen our understanding of the ongoing processes of inter-karyomorph genome differentiation, as well as the amazing variety of sex chromosome systems inside this fish group. Besides, our approach not only uncovered the male-specific regions on the sex chromosomes, but also confirmed different trajectories of the sex chromosome evolution. Future studies using high throughput sequencing will be applied in microdissected sex chromosomes for furthering our understanding of sex determination in this species complex and its possible link with the speciation process.
Author Contributions
AS: Designed the study, performed the experiments, and drafted the manuscript. CY, TH, and EdO: Performed the experiments and drafted the manuscript; PR and LB: Drafted and revised the manuscript; MC: Designed the study drafted and revised the manuscript. All authors read and approved the final version of the manuscript.
Conflict of Interest Statement
The authors declare that the research was conducted in the absence of any commercial or financial relationships that could be construed as a potential conflict of interest.
Acknowledgments
This study was supported by Conselho Nacional de Desenvolvimento Científico e Tecnológico—CNPq (Proc. nos 304992/2015-1, 401575/2016-0 and 152105/2016-6), Fundação de Amparo à Pesquisa do Estado de São Paulo- FAPESP (Proc. No 2016/21411-7) and the project EXCELLENCE CZ.02.1.01/0.0/0.0/15_003/0000460 OP RDE and RVO: 67985904.
Abbreviations
2n, iploid chromosome number; CGH, comparative genomic hybridization; DAPI, 4′,6-diamidino-2-phenylindole; dUTP, 2′-Deoxyuridine-5′-Triphosphate; FISH, fluorescence in situ hybridization; gDNA, total genomic DNA; m, metacentric chromosome; NFDM, non-fat dried milk; NOR, nucleolar organizer region, PCR, polymerase chain reaction; rDNA, ribosomal DNA; SD, sex determination; sm, submetacentric chromosome; TEs, transposable elements; WCP, whole chromosomal painting.
References
de Almeida-Toledo, L. F., and Foresti, F. (2001). Morphologically differentiated sex chromosomes in neotropical freshwater fish. Genetica 111, 91–100. doi: 10.1023/A:1013768104422
Altmanová, M., Rovatsos, M., Kratochvíl, L., and Johnson Pokorná, M. (2016). Minute Y chromosomes and karyotype evolution in Madagascan iguanas (Squamata: Iguania: Opluridae). Biol. J. Linn. Soc. 118, 618–633. doi: 10.1111/bij.12751
Bachtrog, D., Mank, J. E., Peichel, C. L., Kirkpatrick, M., Otto, S. P., Ashman, T. L., et al. (2014). Sex determination: why so many ways of doing it? PLoS Biol. 12:e1001899. doi: 10.1371/journal.pbio.1001899
Badenhorst, D., Stanyon, R., Engstrom, T., and Valenzuela, N. (2013). A ZZ/ZW microchromosome system in the spiny softshell turtle, Apalone spinifera, reveals an intriguing sex chromosome conservation in Trionychidae. Chromosome Res. 21, 137–147. doi: 10.1007/s10577-013-9343-2
Bertollo, L. A. C. (2007). “Chromosome evolution in the Neotropical Erythrinidae fish family: an overview,” in Fish Cytogenetics, eds E. Pisano, C. Ozouf-Costaz, F. Foresti, and B. G. Kapoor (Enfield, NH: Science Publishers), 195–211.
Bertollo, L. A. C., and Mestriner, C. A. (1998). The X1X2Y sex chromosome system in the fish Hoplias malabaricus II Meiotic analyses. Chromosome Res. 6, 141–147. doi: 10.1023/A:1009243114124
Bertollo, L. A., Born, G. G., Dergam, J. A., Fenocchio, A. S., and Moreira-Filho, O. (2000). A biodiversity approach in the neotropical Erythrinidae fish, Hoplias malabaricus. Karyotypic survey, geographic distribution of cytotypes and cytotaxonomic considerations. Chromosome Res. 8, 603–613. doi: 10.1023/A:1009233907558
Bertollo, L. A. C., Fontes, M. S., Fenocchio, A. S., and Cano, J. (1997). The X1X2Y sex chromosome system in the fish Hoplias malabaricus. I. G-, C- and chromosome replication banding. Chromosome Res. 5, 493–499. doi: 10.1023/A:1018477232354
Bertollo, L. A. C., Moreira-Filho, O., and Cioffi, M. B. (2015). “Direct chromosome preparations from freshwater teleost fishes,” in Fish Techniques, Ray-Fin Fishes and Chondrichthyans, eds C. Ozouf-Costaz, E. Pisano, F. Foresti, and L. F. de Almeida Toledo (Boca Ranton, FL: CRC Press), 21–26.
Bi, K., and Bogart, J. P. (2006). Identification of intergenomic recombinations in unisexual salamanders of the genus Ambystoma by genomic in situ hybridization (GISH). Cytogenet. Genome Res. 112, 307–312. doi: 10.1159/000089885
Blanco, D. R., Lui, R. L., Vicari, M. R., Bertollo, L. A., and Moreira-Filho, O. (2011). Comparative cytogenetics of giant trahiras Hoplias aimara and H. intermedius (Characiformes, Erythrinidae): chromosomal characteristics of minor and major ribosomal DNA and cross-species repetitive centromeric sequences mapping differ among morphologically identical karyotypes. Cytogenet. Genome Res. 132, 71–78. doi: 10.1159/000320923
Born, G. G., and Bertollo, L. A. (2000). An XX/XY sex chromosome system in a fish species, Hoplias malabaricus, with a polymorphic NOR-bearing X chromosome. Chromosome Res. 8, 111–118. doi: 10.1023/A:1009238402051
Brykov, V. A. (2014). Mechanisms of sex determination in fish: evolutionary and practical aspects. Russ. J. Mar. Biol. 40, 407–417. doi: 10.1134/S1063074014060145
Carvalho, P. C., de Oliveira, E. A., Bertollo, L. A. C., Yano, C. F., Oliveira, C., Decru, E., et al. (2017). First chromosomal analysis in Hepsetidae (Actinopterygii, Characiformes): insights into relationship between African and Neotropical fish groups. Front. Genet. 8:203. doi: 10.3389/fgene.2017.00203
Charlesworth, B., Sniegowski, P., and Stephan, W. (1994). The evolutionary dynamics of repetitive DNA in eukaryotes. Nature 371, 215–220. doi: 10.1038/371215a0
Chester, M., Leitch, A. R., Soltis, P. S., and Soltis, D. E. (2010). Review of the application of modern cytogenetic methods (FISH/GISH) to the study of reticulation (polyploidy/hybridisation). Genes 1, 166–192. doi: 10.3390/genes1020166
Cioffi, M. B., and Bertollo, L. A. (2010). Initial steps in XY chromosome differentiation in Hoplias malabaricus and the origin of an X1X2Y sex chromosome system in this fish group. Heredity 105, 554–561. doi: 10.1038/hdy.2010.18
Cioffi, M. B., and Bertollo, L. A. (2012). Chromosomal distribution and evolution of repetitive DNAs in fish. Genome Dyn. 7, 197–221. doi: 10.1159/000337950
Cioffi, M. B., Liehr, T., Trifonov, V., Molina, W. F., and Bertollo, L. A. C. (2013). Independent sex chromosome evolution in lower vertebrates: a molecular cytogenetic overview in the erythrinidae fish family. Cytogenet. Genome Res. 141, 186–194. doi: 10.1159/000354039
Cioffi, M. B., Martins, C., and Bertollo, L. A. C. (2009). Comparative chromosome mapping of repetitive sequences. Implications for genomic evolution in the fish, Hoplias malabaricus. BMC Genet. 10:34. doi: 10.1186/1471-2156-10-34
Cioffi, M. B., Martins, C., Vicari, M. R., Rebordinos, L., and Bertollo, L. A. (2010). Differentiation of the XY sex chromosomes in the fish Hoplias malabaricus (Characiformes, Erythrinidae). Unusual accumulation of repetitive sequences on the X chromosome. Sex. Dev. 4, 176–185. doi: 10.1159/000309726
Cioffi, M. B., Molina, W. F., Artoni, R. F., and Bertollo, L. A. (2012). “Chromosomes as tools for discovering biodiversity – the case of Erythrinidae fish family,” in Recent Trends in Cytogenetic Studies – Methodologies and Applications, ed P. Tirunilai (Rijeka: InTech Publisher), 125–146.
Cioffi, M. B., Molina, W. F., Moreira-Filho, O., and Bertollo, L. A. (2011a). Chromosomal distribution of repetitive DNA sequences highlights the independent differentiation of multiple sex chromosomes in two closely related fish species. Cytogenet. Genome Res. 134, 295–302. doi: 10.1159/000329481
Cioffi, M. B., Sánchez, A., Marchal, J. A., Kosyakova, N., Liehr, T., Trifonov, V., et al. (2011b). Cross-species chromosome painting tracks the independent origin of multiple sex chromosomes in two cofamiliar Erythrinidae fishes. BMC Evol. Biol. 11:186. doi: 10.1186/1471-2148-11-186
Cioffi, M. B., Sánchez, A., Marchal, J. A., Kosyakova, N., Liehr, T., Trifonov, V., et al. (2011c). Whole chromosome painting reveals independent origin of sex chromosomes in closely related forms of a fish species. Genetica 139, 1065–1072. doi: 10.1007/s10709-011-9610-0
Cnaani, A. (2013). The tilapias' chromosomes influencing sex determination. Cytogenet. Genome Res. 141, 195–205. doi: 10.1159/000355304
Dergam, J. A., Paiva, S. R., Schaefer, C. E., Godinho, A. L., and Vieira, F. (2002). Phylogeography and RAPD-PCR variation in Hoplias malabaricus (Bloch, 1974) (Pisces, Teleostei) in southeastern Brazil. Genet. Mol. Biol. 25, 379–387. doi: 10.1590/S1415-47572002000400005
Dergam, J. A., Suzuki, H. I., Shibatta, O. A., Duboc, L. F., Júlio, H. F. Jr., Giuliano-Caetano, L., et al. (1998). Molecular biogeography of the neotropical fish Hoplias malabaricus (Erythrinidae, Characiformes) in the Iguaçu, Tibagi, and Paraná rivers. Genet. Mol. Biol. 21, 493–496. doi: 10.1590/S1415-47571998000400015
Devlin, R. H., and Nagahama, Y. (2002). Sex determination and sex differentiation in fish: an overview of genetic, physiological, and environmental influences. Aquaculture 208, 191–364. doi: 10.1016/S0044-8486(02)00057-1
Doležálková, M., Sember, A., Marec, F., Ráb, P., Plötner, J., and Choleva, L. (2016). Is premeiotic genome elimination an exclusive mechanism for hemiclonal reproduction in hybrid males of the genus Pelophylax? BMC Genet. 17:100. doi: 10.1186/s12863-016-0408-z
Ezaz, T., and Deakin, J. E. (2014). Repetitive sequence and sex chromosome evolution in vertebrates. Adv. Evol. Biol. 2014, 1–9. doi: 10.1155/2014/104683
Ezaz, T., Valenzuela, N., Grützner, F., Miura, I., Georges, A., Burke, R. L., et al. (2006). An XX/XY sex microchromosome system in a freshwater turtle, Chelodina longicollis (Testudines: Chelidae) with genetic sex determination. Chromosome Res. 14, 139–150. doi: 10.1007/s10577-006-1029-6
Faria, R., and Navarro, A. (2010). Chromosomal speciation revisited: rearranging theory with pieces of evidence. Trends Ecol. Evol. 25, 660–669. doi: 10.1016/j.tree.2010.07.008
Freitas, N. L., Al-Rikabi, A. B. H., Bertollo, L. A. C., Ezaz, T., Yano, C. F., Oliveira, E. A., et al. (2017). Early stages of XY sex chromosomes differentiation in the fish Hoplias malabaricus (Characiformes, Erythrinidae) revealed by DNA repeats accumulation. Curr. Genomics 19, 216–226. doi: 10.2174/1389202918666170711160528
Gazoni, T., Haddad, C. F. B., Narimatsu, H., Cabral-de-Mello, D. C., Lyra, M. L., and Parise-Maltempi, P. P. (2018). More sex chromosomes than autosomes in the Amazonian frog Leptodactylus pentadactylus. Chromosoma. doi: 10.1007/s00412-018-0663-z. [Epub ahead of print].
Green, J. E., Dalíková, M., Sahara, K., Marec, F., and Akam, M. (2016). XX/XY system of sex determination in the geophilomorph centipede Strigamia maritima. PLoS ONE 11:e0150292. doi: 10.1371/journal.pone.0150292
Griffin, D. K., Harvey, S. C., Campos-Ramos, R., Ayling, L.-J., Bromage, N. R., Masabanda, J. S., et al. (2002). Early origins of the X and Y chromosome: lessons from tilapia. Cytogenet. Genome Res. 99, 157–163. doi: 10.1159/000071588
Henning, F., Trifonov, V., Ferguson-Smith, M. A., and de Almeida-Toledo, L. F. (2008). Non-homologous sex chromosomes in two species of the genus Eigenmannia (Teleostei: Gymnotiformes). Cytogenet Genome Res. 121, 55–58. doi: 10.1159/000124382
Heule, C., Salzburger, W., and Böhne, A. (2014). Genetics of sexual development: an evolutionary playground for fish. Genetics 196, 579–591. doi: 10.1534/genetics.114.161158
Hurley, I. A., Mueller, R. L., Dunn, K. A., Schmidt, E. J., Friedman, M., Ho, R. K., et al. (2007). A new time-scale for ray-finned fish evolution. Proc. R. Soc. B Biol. Sci. 274, 489–498. doi: 10.1098/rspb.2006.3749
Kallioniemi, A., Kallioniemi, O. P., Sudar, D., Rutovitz, D., Gray, J. W., Waldman, F., et al. (1992). Comparative genomic hybridization for molecular cytogenetic analysis of solid tumors. Science 258, 818–821. doi: 10.1126/science.1359641
Kamiya, T., Kai, W., Tasumi, S., Oka, A., Matsunaga, T., Mizuno, N., et al. (2012). A trans-species missense SNP in Amhr2 is associated with sex determination in the tiger pufferfish, Takifugu rubripes (Fugu). PLoS Genet. 8:e1002798. doi: 10.1371/journal.pgen.1002798
Kato, A., Vega, J. M., Han, F., Lamb, J. C., and Bircher, J. A. (2005). Advances in plant chromosome identification and cytogenetic techniques. Curr. Opin. Plant. Biol. 8, 148–154. doi: 10.1016/j.pbi.2005.01.014
Kawai, A., Nishida-Umehara, C., Ishijima, J., Tsuda, Y., Ota, H., and Matsuda, Y. (2007). Different origins of bird and reptile sex chromosomes inferred from comparative mapping of chicken Z-linked genes. Cytogenet. Genome Res. 117, 92–102. doi: 10.1159/000103169
Kikuchi, K., and Hamaguchi, S. (2013). Novel sex-determining genes in fish and sex chromosome evolution. Dev. Dyn. 242, 339–353. doi: 10.1002/dvdy.23927
Kitano, J., and Peichel, C. L. (2012). Turnover of sex chromosomes and speciation in fishes. Environ. Biol. Fishes. 94, 549–558. doi: 10.1007/s10641-011-9853-8
Kitano, J., Ross, J. A., Mori, S., Kume, M., Jones, F. C., Chan, Y. F., et al. (2009). A role for a neo-sex chromosome in stickleback speciation. Nature 461, 1079–1083. doi: 10.1038/nature08441
Knytl, M., Kalous, L., Symonová, R., Rylková, K., and Ráb, P. (2013). Chromosome studies of European cyprinid fishes: cross-species painting reveals natural allotetraploid origin of a Carassius female with 206 chromosomes. Cytogenet. Genome Res. 139, 276–283. doi: 10.1159/000350689
Koubová, M., Pokorná, M. J., Rovatsos, M., Farkacová, K., Altmanová, M., and Kratochvíl, L. (2014). Sex determination in Madagascar geckos of the genus Paroedura (Squamata: Gekkonidae): are differentiated sex chromosomes indeed so evolutionary stable? Chromosome Res. 22, 441–452. doi: 10.1007/s10577-014-9430-z
Levan, A., Fredga, K., and Sandberg, A. A. (1964). Nomenclature for centromeric position on chromosomes. Hereditas 52, 201–220. doi: 10.1111/j.1601-5223.1964.tb01953.x
Liu, H., Pang, M., Yu, X., Zhou, Y., Tong, J., and Fu, B. (2018). Sex-specific markers developed by next-generation sequencing confirmed an XX/XY sex determination system in bighead carp (Hypophthalmichehys nobilis) and silver carp (Hypophthalmichthys molitrix). DNA Res. doi: 10.1093/dnares/dsx054. [Epub ahead of print].
López-Flores, I., and Garrido-Ramos, M. A. (2012). The repetitive DNA content of eukaryotic genomes. Genome Dyn. 7, 1–28. doi: 10.1159/000337118
Majka, J., Majka, M., Kwiatek, M., and Wiśniewska, H. (2016). Similarities and differences in the nuclear genome organization within Pooideae species revealed by comparative genomic in situ hybridization (GISH). J. Appl. Genet. 58, 151–161. doi: 10.1007/s13353-016-0369-y
Majtánová, Z., Choleva, L., Symonová, R., Ráb, P., Kotusz, J., Pekárik, L., et al. (2016). Asexual reproduction does not apparently increase the rate of chromosomal evolution: karyotype stability in diploid and triploid clonal hybrid fish (Cobitis, Cypriniformes, Teleostei). PLoS ONE 11:e0146872. doi: 10.1371/journal.pone.0146872
Mank, J. E., and Avise, J. C. (2009). Evolutionary diversity and turn-over of sex determination in teleost fishes. Sex Dev. 3, 60–67. doi: 10.1159/000223071
Mank, J. E., Promislow, D. E. L., and Avise, J. C. (2006). Evolution of alternative sex-determining mechanisms in teleost fishes. Biol. J. Linn. Soc. 87, 83–93. doi: 10.1111/j.1095-8312.2006.00558.x
Marques, D. F., Santos, F. A., da Silva, S. S., Sampaio, I., and Rodrigues, L. R. R. (2013). Cytogenetic and DNA barcoding reveals high divergence within the trahira, Hoplias malabaricus (Characiformes: Erythrinidae) from the lower Amazon River. Neotrop. Ichthyol. 11, 459–466. doi: 10.1590/S1679-62252013000200015
Martinez, J. F., Lui, R. L., Traldi, J. B., Blanco, D. R., and Moreira-Filho, O. (2016). Comparative cytogenetics of Hoplerythrinus unitaeniatus (Agassiz, 1829) (Characiformes, Erythrinidae) species complex from different brazilian hydrographic basins. Cytogenet. Genome Res. 149, 191–200. doi: 10.1159/000448153
Martinez, J. F., Lui, R. L., Traldi, J. B., Blanco, D. R., and Moreira-Filho, O. (2015). Occurrence of natural hybrids among sympatric karyomorphs in Hoplerythrinus unitaeniatus (Characiformes, Erythrinidae). Zebrafish 12, 281–287. doi: 10.1089/zeb.2015.1083
Martínez, P., Viñas, A. M., Sánchez, L., Díaz, N., Ribas, L., and Piferrer, F. (2014). Genetic architecture of sex determination in fish: applications to sex ratio control in aquaculture. Front. Genet. 5:340. doi: 10.3389/fgene.2014.00340
Matsuda, M., Nagahama, Y., Shinomiya, A., Sato, T., Matsuda, C., Kobayashi, T., et al. (2002). DMY is a Y specific DM-domain gene required for male development in the medaka fish. Nature 417, 559–563. doi: 10.1038/nature751
Montiel, E. E., Badenhorst, D., Tamplin, J., Burke, R. L., and Velanzuela, N. (2017). Discovery of the youngest sex chromosomes reveals first case of convergent co-option of ancestral autosomes in turtles. Chromosoma 126, 105–113. doi: 10.1007/s00412-016-0576-7
Moraes, R. L. R., Bertollo, L. A. C., Marinho, M. M. F., Yano, C. F., Hatanaka, T., Barby, F. F., et al. (2017). Evolutionary relationships and cytotaxonomy considerations in the genus Pyrrhulina (Characiformes, Lebiasinidae). Zebrafish 14, 536–546. doi: 10.1089/zeb.2017.1465
Nanda, I., Kondo, M., Hornung, U., Asakawa, S., Winkler, C., Shimizu, A., et al. (2002). A duplicated copy of DMRT1 in the sex-determining region of the Y chromosome of the medaka, Oryzias latipes. Proc. Natl. Acad. Sci. U.S.A. 99, 11778–11783. doi: 10.1073/pnas.182314699
Nguyen, P., Sýkorová, M., Šíchová, J., Kuta, V., Dalíková, M., Capková Frydrychová, R., et al. (2013). Neo-sex chromosomes and adaptive potential in tortricid pests. Proc. Natl. Acad. Sci. U.S.A. 110, 6931–6936. doi: 10.1073/pnas.1220372110
de Oliveira, E. A., Bertollo, L. A. C., Yano, C. F., Liehr, T., and Cioffi, M. B. (2015). Comparative cytogenetics in the genus Hoplias (Characiformes, Erythrinidae) highlights contrasting karyotype evolution among congeneric species. Mol. Cytogenet. 8:56. doi: 10.1186/s13039-015-0161-4
Oliveira, E. A., Sember, A., Bertollo, L. A. C., Yano, C. F., Ezaz, T., Moreira-Filho, O., et al. (2018). Tracking the evolutionary pathway of sex chromosomes among fishes: characterizing the unique XX/XY1Y2 system in Hoplias malabaricus (Teleostei, Characiformes). Chromosoma 127, 115–128. doi: 10.1007/s00412-017-0648-3
Oyakawa, O. T. (2003). “Family Erythrinidae,” in Check List of the freshwater fishes of South and Central America, eds R. E. Reis, S. O. Kullander, and C. J., Ferraris Jr (Porto Alegre: Edipucrs), 238–240.
Oyakawa, O. T., and Mattox, G. M. T. (2009). Revision of the Neotropical trahiras of the Hoplias lacerdae species-group (Ostariophysi: Characiformes: Erythrinidae) with descriptions of two new species. Neotrop. Ichthyol. 7, 117–140. doi: 10.1590/S1679-62252009000200001
Pennell, M. W., Kirkpatrick, M., Otto, S. P., Vamosi, J. C., Peichel, C. L., Valenzuela, N., et al. (2015). Y fuse? Sex chromosome fusions in fishes and reptiles. PLoS Genet. 11:e1005237. doi: 10.1371/journal.pgen.1005237
Pokorná, M., Altmanová, M., and Kratochvíl, L. (2014). Multiple sex chromosomes in the light of female meiotic drive in amniote vertebrates. Chromosome Res. 22, 35–44. doi: 10.1007/s10577-014-9403-2
Portela-Bens, S., Merlo, M. A., Rodríguez, M. E., Cross, I., Manchado, M., Kosyakova, N., et al. (2017). Integrated gene mapping and synteny studies give insights into the evolution of a sex protochromosome in Solea senegalensis. Chromosoma 126, 261–277. doi: 10.1007/s00412-016-0589-2
Rantin, F. T., Glass, M. L., Kalinin, A. L., Verzola, R. M. M., and Fernandes, M. N. (1993). Cardio-respiratory responses in two ecologically distinct erythrinids (Hoplias malabaricus and Hoplias lacerdae) exposed to graded environmental hypoxia. Environ. Biol. Fish. 36, 93–97. doi: 10.1007/BF00005983
Rantin, F. T., Kalinin, A. L., Glass, M. L., and Fernandes, M. N. (1992). Respiratory responses to hypoxia in relation to mode of life of two erythrinid species (Hoplias malabaricus and Hoplias lacerdae). J. Fish. Biol. 41, 805–812. doi: 10.1111/j.1095-8649.1992.tb02708.x
Ravi, V., and Venkatesh, B. (2008). Rapidly evolving fish genomes and teleost diversity. Curr. Opin. Genet. Dev. 18:544–550. doi: 10.1016/j.gde.2008.11.001
Reichwald, K., Petzold, A., Koch, P., Downie, B. R., Hartmann, N., Pietsch, S., et al. (2015). Insights into sex chromosome evolution and aging from the genome of a short-lived fish. Cell 163, 1527–1538. doi: 10.1016/j.cell.2015.10.071
Rios, F. S., Kalinin, A. L., and Rantin, F. T. (2002). The effects of long-term food deprivation on respiration and hematology of the neotropical fish Hoplias malabaricus. J. Fish. Biol. 61, 85–95. doi: 10.1111/j.1095-8649.2002.tb01738.x
Rosa, R., Laforga Vanzela, A. L., Rubert, M., Martins-Santos, I. C., and Giuliano-Caetano, L. (2009). Differentiation of Y chromosome in the X1X1X2X2/X1X2Y sex chromosome system of Hoplias malabaricus (Characiformes, Erythrinidae). Cytogenet. Genome Res. 127, 54–60. doi: 10.1159/000269736
Rosa, R., Rubert, M., Martins-Santos, I., and Giuliano-Caetano, L. (2012). Evolutionary trends in Hoplerythrinus unitaeniatus (Agassiz 1829) (Characiformes, Erythrinidae). Rev. Fish Biol. Fish. 22, 467–475. doi: 10.1007/s11160-011-9237-3
Ross, J. A., Urton, J. R., Boland, J., Shapiro, M. D., and Peichel, C. L. (2009). Turnover of sex chromosomes in the stickleback fishes (Gasterosteidae). PLoS Genet. 5:e1000391. doi: 10.1371/journal.pgen.1000391
Rovatsos, M., Johnson Pokorná, M., Altmanová, M., and Kratochvíl, L. (2016). Mixed-up sex chromosomes: identification of sex chromosomes in the X1X1X2X2/X1X2Y system of the legless lizards of the genus Lialis (Squamata: Gekkota: Pygopodidae). Cytogenet. Genome Res. 149, 282–289. doi: 10.1159/000450734
Salvadori, S., Deiana, A. M., Deidda, F., Lobina, C., Mulas, A., and Coluccia, E. (2018). XX/XY sex chromosome system and chromosome markers in the snake eel Ophisurus serpens (Anguilliformes: Ophichtidae). Mar. Biol. Res. 14, 158–164. doi: 10.1080/17451000.2017.1406665
Sambrook, J., and Russell, D. W. (2001). Molecular Cloning: A Laboratory Manual, 3rd Edn. New York, NY: Cold Spring Harbor Laboratory Press.
Santos, U., Völcker, C. M., Belei, F. A., Cioffi, M. B., Bertollo, L. A., Paiva, S. R., et al. (2009). Molecular and karyotypic phylogeography in the Neotropical Hoplias malabaricus (Erythrinidae) fish in eastern. Brazil. J. Fish Biol. 75, 2326–2343. doi: 10.1111/j.1095-8649.2009.02489.x
Schartl, M. (2004). Sex chromosome evolution in non-mammalian vertebrates. Curr. Opin. Genet. Dev. 14, 634–641. doi: 10.1016/j.gde.2004.09.005
Schartl, M., Schmid, M., and Nanda, I. (2016). Dynamics of vertebrate sex chromosome evolution: from equal size to giants and dwarfs. Chromosoma 125, 553–571. doi: 10.1007/s00412-015-0569-y
Schoumans, J., Nielsen, K., Jeppesen, I., Anderlid, B. M., Blennow, E., Brøndum-Nielsen, K., et al. (2004). A comparison of different metaphase CGH methods for the detection of cryptic chromosome aberrations of defined size. Eur. J. Hum. Genet. 12, 447–454. doi: 10.1038/sj.ejhg.5201175
Sutherland, B. J. G., Rico, C., Audet, C., and Bernatchez, L. (2017). Sex chromosome evolution, heterochiasmy, and physiological QTL in the salmonid brook charr Salvelinus fontinalis. G3 7, 2749–2762. doi: 10.1534/g3.117.040915
Symonová, R., Flajšhans, M., Sember, A., Havelka, M., Gela, D., Korínková, T., et al. (2013a). Molecular cytogenetics in artificial hybrid and highly polyploid sturgeons: an evolutionary story narrated by repetitive sequences. Cytogenet. Genome Res. 141, 153–162. doi: 10.1159/000354882
Symonová, R., Majtánová, Z., Sember, A., Staaks, G. B., Bohlen, J., Freyhof, J., et al. (2013b). Genome differentiation in a species pair of coregonine fishes: an extremely rapid speciation driven by stress-activated retrotransposons mediating extensive ribosomal DNA multiplications. BMC Evol. Biol. 13:42. doi: 10.1186/1471-2148-13-42
Symonová, R., Sember, A., Majtánová, Z., and Ráb, P. (2015). “Characterization of fish genomes by GISH and CGH,” in Fish techniques, Ray-Fin Fishes and Chondrichthyans, eds C. Ozouf-Costaz, E. Pisano, F. Foresti, and L. F. de Almeida Toledo (Boca Ranton, FL: CRC Press), 118–131.
Takehana, Y., Naruse, K., Hamaguchi, S., and Sakaizumi, M. (2007). Evolution of ZZ/ZW and XX/XY sex-determination systems in the closely related medaka species, Oryzias hubbsi and O. dancena. Chromosoma 116, 463–470. doi: 10.1007/s00412-007-0110-z
Traut, W., and Winking, H. (2001). Meiotic chromosomes and stages of sex chromosome evolution in fish: zebrafish, platyfish and guppy. Chromosome Res. 9, 659–672. doi: 10.1023/A:1012956324417
Utsunomia, R., Pansonato-Alves, J. C., Paiva, L. R. S., Costa Silva, G. J., Oliveira, C., Bertollo, L. A. C., et al. (2014). Genetic differentiation among distinct karyomorphs of the wolf fish Hoplias malabaricus species complex (Characiformes, Erythrinidae) and report of unusual hybridization with natural triploidy. J. Fish Biol. 85, 1682–1692. doi: 10.1111/jfb.12526
Valente, T. G., Schneider, C. H., Gross, M. C., Feldberg, E., and Martins, C. (2009). Comparative cytogenetics of cichlid fishes through genomic in-situ hybridization (GISH) with emphasis on Oreochromis niloticus. Chromosome Res. 17, 791–799. doi: 10.1007/s10577-009-9067-5
Woram, R. A., Gharbi, K., Sakamoto, T., Hoyheim, B., Holm, L. E., Naish, K., et al. (2003). Comparative genome analysis of the primary sex-determining locus in salmonid fishes. Genome Res. 13, 272–280. doi: 10.1101/gr.578503
Yano, C. F., Bertollo, L. A., Ezaz, T., Trifonov, V., Sember, A., Liehr, T., et al. (2017). Highly conserved Z and molecularly diverged W chromosomes in the fish genus Triportheus (Characiformes, Triportheidae). Heredity 118, 276–283. doi: 10.1038/hdy.2016.83
Zhou, Q., Braasch, I., Froschauer, A., and Böhne, A. (2010). A novel marker for the platyfish (Xiphophorus maculatus) W chromosome is derived from a Polinton transposon. J. Genet. Genomics 37, 181–188. doi: 10.1016/S1673-8527(09)60036-9
Keywords: fish cytogenetics, multiple sex chromosomes, sex-determining region, sex chromosome turnover, CGH, intraspecific variability, species complex, speciation
Citation: Sember A, Bertollo LAC, Ráb P, Yano CF, Hatanaka T, de Oliveira EA and Cioffi MdB (2018) Sex Chromosome Evolution and Genomic Divergence in the Fish Hoplias malabaricus (Characiformes, Erythrinidae). Front. Genet. 9:71. doi: 10.3389/fgene.2018.00071
Received: 29 August 2017; Accepted: 16 February 2018;
Published: 05 March 2018.
Edited by:
Roberto Ferreira Artoni, Ponta Grossa State University, BrazilReviewed by:
Julio Cesar Pieczarka, Universidade Federal do Pará, BrazilDaniel Pacheco Bruschi, Universidade Federal do Paraná, Brazil
Copyright © 2018 Sember, Bertollo, Ráb, Yano, Hatanaka, de Oliveira and Cioffi. This is an open-access article distributed under the terms of the Creative Commons Attribution License (CC BY). The use, distribution or reproduction in other forums is permitted, provided the original author(s) and the copyright owner are credited and that the original publication in this journal is cited, in accordance with accepted academic practice. No use, distribution or reproduction is permitted which does not comply with these terms.
*Correspondence: Marcelo de Bello Cioffi, bWJjaW9mZmlAdWZzY2FyLmJy