- Department of Biochemistry and Microbiology, University of Victoria, Victoria, BC, Canada
Nanoparticles (NPs), materials that have one dimension less than 100 nm, are used in manufacturing, health, and food products, and consumer products including cosmetics, clothing, and household appliances. Their utility to industry is derived from their high surface-area-to-volume ratios and physico-chemical properties distinct from their bulk counterparts, but the near-certainty that NPs will be released into the environment raises the possibility that they could present health risks to humans and wildlife. The thyroid hormones (THs), thyroxine, and 3,3′,5-triiodothyronine (T3), are involved in development and metabolism in vertebrates including humans and frogs. Many of the processes of anuran metamorphosis are analogous to human post-embryonic development and disruption of TH action can have drastic effects. These shared features make the metamorphosis of anurans an excellent model for screening for endocrine disrupting chemicals (EDCs). We used the cultured tailfin (C-fin) assay to examine the exposure effects of 0.1–10 nM (~8–800 ng/L) of three types of ~20 nm TiO2 NPs (P25, M212, M262) and micron-sized TiO2 (μ TiO2) ±10 nM T3. The actual Ti levels were 40.9–64.7% of the nominal value. Real-time quantitative polymerase chain reaction (QPCR) was used to measure the relative amounts of mRNA transcripts encoding TH-responsive THs receptors (thra and thrb) and Rana larval keratin type I (rlk1), as well as the cellular stress-responsive heat shock protein 30 kDa (hsp30), superoxide dismutase (sod), and catalase (cat). The levels of the TH-responsive transcripts were largely unaffected by any form of TiO2. Some significant effects on stress-related transcripts were observed upon exposure to micron-sized TiO2, P25, and M212 while no effect was observed with M262 exposure. Therefore, the risk of adversely affecting amphibian tissue by disrupting TH-signaling or inducing cellular stress is low for these compounds relative to other previously-tested NPs.
Introduction
Nanoparticles (NPs) are materials that have been purposefully manufactured to the nanometer scale (Handy et al., 2008). They are used in a diverse array of consumer goods and industrial processes, and their use is expected to expand in the future (Oberdörster et al., 2007). This utility derives from the unique properties of materials at this scale: the high surface-area-to-volume ratio is thought to raise the reactivity relative to the bulk form of the material (Wijnhoven et al., 2009). Titanium dioxide NPs are increasingly entering the marketplace due to their usefulness in multiple applications. The estimated current production is approximately 44,400 tonnes (2.5% of total TiO2 production), but by 2015 it is expected to reach approximately 260,000 tonnes (10% of total production) (Robichaud et al., 2009). These NPs are used in personal care products such as sunscreens, toothpastes, and cosmetics, industrial products such as paints, lacquers, and papers, and photocatalytic processes such as water treatment (Schmid and Riediker, 2008; Robichaud et al., 2009). The growth of the NP industry indicates an increased likelihood that significant quantities will be released into the environment (Scown et al., 2010). Possible routes of environmental release include production and transport spills, production wastes, and from products during their use, reuse, and end disposal (Baun et al., 2009). Due to these exposure risks, increased attention is being paid to possible negative health effects of NPs on humans, along with the concern that wildlife and the environment may be affected as well (Oberdörster et al., 2007; Handy et al., 2008; Baun et al., 2009).
Although TiO2 NPs have been used as non-bioactive controls in nanotoxicology studies, exposure to 1 mg/L P25 TiO2 NPs affected 170 gene transcripts in Danio rerio after 48 h (Griffitt et al., 2009). Realistically, wildlife are exposed to lower concentrations and, although it is not yet possible to reliably determine the contribution of TiO2 NPs vs. bulk counterpart in wastewater effluents, the estimated median range of Ti-containing NPs is 0.1–0.4 μg/L (0.5–2 μg/L maximum) in wastewater effluents based upon projected NP production of 2.5–10% (Westerhoff et al., 2011). Generally, TiO2 NPs present low classic toxicity with LC50 values in the high mg/L range or not achieved for a variety of species (Clemente et al., 2011). However, chemical concentrations that have no observable effects (NOEC) in terms of morphology are capable of disrupting sensitive endocrine signaling; a phenomenon which has been observed at very low NP concentrations (Colborn et al., 1993; Rajapakse et al., 2002; Hinther et al., 2010b). Chemicals or compounds that mimic or block the activity of naturally-circulating hormones with respect to transcriptional activation of their target genes are referred to as endocrine disrupting chemicals (EDCs) (Tabb and Blumberg, 2006). This can affect histone acetylation/deacetylation, DNA methylation, and transcriptional regulation, all of which may lead to developmental and reproductive anomalies (Tabb and Blumberg, 2006).
A process that lends itself well to the study of EDCs is amphibian metamorphosis, as it is driven solely by thyroid hormone (TH) (Shi, 2000; Hinther et al., 2010a). The TH pathway is highly conserved in vertebrates, so data on EDCs gathered from amphibian studies could be extended to other species, including humans. We have previously developed a rapid cultured tailfin (C-fin) assay (Hinther et al., 2010a) that is capable of evaluating TH signaling disruption. The C-fin assay involves collecting multiple biopsies from the tailfins of premetamorphic Rana catesbeiana tadpoles and culturing them ex vivo for 48 h in a concentration range of test chemical in the presence or absence of TH. A biopsy from each animal is cultured in each treatment condition, which allows the screening of multiple chemicals or concentrations of a certain chemical on the same individuals simultaneously without compromising complex tissue structure (Hinther et al., 2010a). Furthermore, inter-individual variation in response to the treatments can be determined because of the repeated-measures design of the assay.
Disruptions in TH signaling can be detected by examining changes in transcript levels of known TH-responsive genes. The TRs bind TH directly and are essential for execution of the cellular response to TH through gene activation or inactivation (Zhang and Lazar, 2000; Schreiber et al., 2001; Das et al., 2010). The genes encoding the TH receptors (TR) alpha (thra) and beta (thrb) are up-regulated upon TH exposure whereas the expression of Rana larval type I keratin (rlk1) decreases in the tail fin tissue of R. catesbeiana and Rana clamitans in response to TH (Domanski and Helbing, 2007; Hammond et al., 2013). Perturbations to the tail fin tissue in the absence of TH, such as induction of the cellular stress response, may also be interrogated through measurement of steady-state levels of mRNA from genes such as superoxide dismutase (sod) and catalase (cat), which are involved in the cellular metabolism of reactive oxygen species (ROS), and the general cell stress indicator, heat shock protein 30 (hsp30). The present study used the C-fin assay to evaluate the effects of three TiO2 NPs and micron-sized TiO2 particles (μ TiO2) on TH signaling and induction of cellular stress.
Materials and Methods
Preparation of TiO2 NP, and μTiO2 Test Suspensions
The micro- and NPs are standard materials obtained through the Organization for Economic Cooperation and Development (OECD) Sponsorship program and the European Commission Joint Research Centre (JRC). These characterized stocks (see Table 1 for characteristics) were distributed to a variety of researchers from a common stock. The μ TiO2 test compound was prepared as a 1000 × stock of each treatment concentration, made by addition of powdered TiO2 (μTiO2; TIONA Titanium Dioxide Pigment, Cristal Global, Brussels, Belgium) to ddH2O and serially diluted to 10 μM, 1.0 μM and 0.1 μM based on the % TiO2 content listed in Table 1. The nano-sized TiO2 stock suspensions were made by addition of powdered M212 (UV-Titan M212 (NM-104), Sachtleben Chemie GmbH, Duisburg, Germany) or P25 (Aeroxide® TiO2 P25, Evonik Industries AG, Essen, Germany) TiO2 NP to ddH2O, or M262 (UV-Titan M262 (NM-103), Sachtleben) to DMSO. These preparations were then sonicated for 10 min using a Bioruptor UCD200 (Diagenode Inc., Sparta NJ, USA) on the “low” setting prior to 1000 × stock preparation. As above, the 1000 × stocks were made by serial dilution to 10 μM, 1.0 μM, and 0.1 μM TiO2, based on the % TiO2 content listed in Table 1. Therefore, the final nominal exposure concentrations were between 0.1 and 10 nM (equivalent to ~8–800 ng/L TiO2). These and the actual (see below) concentrations reflect a reasonable level of expected NP in the environment and permit direct comparison to previously published work on other NP types (Hinther et al., 2010b). For simplicity of presentation, the nominal concentrations are used throughout the present study.
Particle Characterization
Stock solutions for characterization of each particle type were prepared with sonication (as above) at 1 mM (80 mg/L) in dH2O, based on manufacturer-declared TiO2 content. The M262 suspension included 0.1% (v/v) DMSO to facilitate solubilization. Average particle size, stability and Ti concentration were determined with dynamic light scattering (DLS), ζ-potential and inductively-coupled plasma mass spectrometry (ICP-MS), respectively. DLS and ζ-potential were measured using ZetaPALS (Brookhaven Instruments Corp., Holtsville, NY, USA) Particle Sizing Software and PALS Zeta Potential Analyzer, respectively. Stocks were sonicated and the average of three measurements at room temperature was taken for each particle type. For ICP-MS analysis, stocks were digested with nitric acid (20% m/m) and hydrogen peroxide (30% v/v) at 80°C for 4 h, followed by further sonication and incubation at 65°C for 3 h. Triton X100 was added to samples at 2% (v/v) to aid Ti detection of undigested particles (Shaw et al., 2013), performed with a Nexlon 300x (Perkin Elmer, Woodbridge, ON, Canada).
Experimental Animals
Taylor and Kollros (TK) (Taylor and Kollros, 1946) VI–VIII R. catesbeiana tadpoles were caught locally (Victoria, BC, Canada). The care and treatment of animals used in the present study was in accordance with the guidelines of the Canadian Council on Animal Care under the guidance of the Animal Care Committee of the University of Victoria. Animals were housed in the University of Victoria aquatics facility and maintained in 100 gallon fiberglass tanks containing recirculated water at 12°C. Tadpoles were fed daily with spirulina (Aquatic ELO-systems) and acclimated to lab conditions for 24 h prior to anaesthetization in a 0.1% (w/v) tricaine methanesulfonate (MS-222, Syndel Laboratories, Qualicum Beach, Canada).
Organ Culture of Tailfin Biopsies
The C-fin assay was performed as described previously (Hinther et al., 2010a). In brief, one assay per test chemical was run consisting of eight 6 mm biopsies from each of eight R. catesbeiana tadpoles. Therefore, eight exposure conditions could be tested with eight biological replicates per assay. Biopsies were cultured in 24 well Primaria culture plates (BD Biosciences, Mississauga, ON, Canada) in 1.0 mL of 70% strength Liebovitz's L15 medium (Life Technologies Inc., Burlington, ON, Canada) with 10 mM HEPES pH 7.5, 50 units/mL penicillin G sodium, 50 μg/mL streptomycin sulphate (Life Technologies) and 50 μg/mL neomycin (Sigma-Aldrich Canada Co., Oakville, ON, Canada) with particle vehicle control or one of three concentrations of a particular particle. The 1000 × TiO2 particle stocks were applied at 1 μ L/mL of media to final concentrations of 10, 1.0, or 0.1 nM (see Table 1 for additional information). The biopsies were incubated with the particles or their vehicle controls for 2 h prior to the addition of 10 nM T3 (Sigma-Aldrich Canada Co.) in 400 nM NaOH or 400 nM NaOH alone, final concentrations. After 48 h, the biopsies were harvested into 100 μ L RNAlater (Life Technologies) and stored at 4°C overnight before being moved to −20°C.
Isolation of RNA and Quantitation of Gene Expression
The biopsy tissues were loaded into 0.5 mL Safe-Lock Eppendorf tubes containing 300 μ L TRIzol reagent and a 1-mm diameter tungsten-carbide bead for mechanical disruption by a Retsch MM301 Mixer Mill (Thermo Fisher Scientific, Markham, ON, Canada) run at 20 Hz twice for 3 min, with the chambers rotated between cycles. Twenty microgram glycogen (Roche Diagnostics, Laval, QC, Canada) was added as a carrier prior to isopropanol precipitation to maximize RNA yield. Isolated RNA was resuspended in 10 μ L diethyl pyrocarbonate (DEPC)-treated (Sigma-Aldrich) RNase-free water and stored at −80°C. Complimentary DNA (cDNA) was synthesized using the High Capacity cDNA Reverse Transcription Kit (Life Technologies) from 1 μ g total RNA following the manufacturer's instructions. The reaction mixture was incubated at 25°C for 10 min, and then at 42°C for 2 h and 5 min at 85°C. The cDNA was diluted 20-fold with DEPC-dH2O and stored at −20°C.
Transcript abundance was determined for TH-response genes [TH receptors thra and thrb, and Rana larval keratin 1 (rlk1)], stress-response genes [catalase (cat), heat shock protein 30 (hsp30), and superoxide dismutase (sod)], and the normalizer gene transcript [ribosomal protein L8 (rpl8)] using a MX3005P real-time QPCR system (Agilent Technologies Canada, Inc., Mississauga, ON, Canada). Primers and hydrolysis probes (Table 2) were ordered from Integrated DNA Technologies (IDT, Coralville, IA, USA). The thra, thrb, and rpl8 QPCR reactions contained 0.01% Tween 20, 0.8% glycerol, 10 mM Tris-HCl (pH 8.3 at 20°C), 50 mM KCl, 3 mM MgCl2, 200 μ M dNTPs, 69.4 nM ROX reference dye (Invitrogen), one unit of Immolase DNA polymerase (Bioline USA Inc., Taunton, MA, USA), and 2 μ L of diluted cDNA (see Table 2 for sequences and amounts of primers and hydrolysis probes added). The cat, hsp30, and sod reactions were the same as the thra-containing reactions, but with the amount of primers and hydrolysis probes noted in Table 2. The rlk1 QPCR reactions contained 0.01% Tween 20, 0.8% glycerol, 10 mM Tris-HCl (pH 8.3 at 20°C), 50 mM KCl, 3 mM MgCl2, 4,0000-fold dilution of SYBR Green I (Molecular Probes, Invitrogen), 200 μ M dNTPs, 69.4 nM ROX reference dye (Invitrogen), one unit of Immolase DNA polymerase (Bioline), 2 μ L of diluted cDNA (see Table 2 for amount of primers used). The thermocycle program for thra, thrb, cat, hsp30,sod, and rpl8 consisted of an initial enzyme activation step at 95°C (9 min) followed by 40 cycles of 95°C denaturation (15 s), 64°C annealing (30 s), and 72°C elongation (30 s). The thermocycle program for rlk1 was the same as above, except that the annealing temperature was 55°C (30 s) and the elongation phase was 45 s. A control lacking cDNA template was included to determine specificity of target cDNA amplification. Reactions were performed in quadruplicate for each sample and data averaged and normalized to expression of the invariant rpl8 control transcript (Figure 1) using the comparative threshold method (ΔΔ Ct) method (Livak and Schmittgen, 2001). Amplification reaction integrity of the primers was confirmed by the presence of a single DNA product following gel electrophoresis and by digestion and restriction mapping of the product or through amplicon sequencing. Absence of genomic DNA (gDNA) contamination in the samples was confirmed through analysis of post-amplification denaturation of the rlk1 reactions: amplicons generated from gDNA produce a denaturation profile different from that generated from cDNA template alone (data not shown). Use of the ΔΔ Ct method was validated by confirmation that the efficiencies of the target amplifications were approximately equal, i.e., that the slope of the line generated from the plotting of the log2 dilution vs. the Δ Ct values between the test gene transcript and rpl8 is between −0.1 and 0.1 (Table 2).
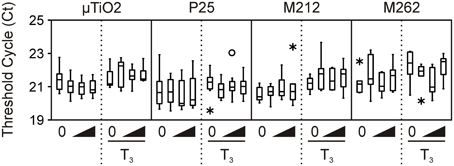
Figure 1. Transcript levels of invariant normalizer gene rpl8 in the μ TiO2 and TiO2 NP C-fin assays as indicated by the threshold cycle (Ct) values obtained by QPCR. Bevels indicate increasing concentrations of the indicated compound in the absence or presence of T3. The medians are indicated by solid black lines within the boxes, where the box denotes the 25th and 75th percentiles, and the whiskers indicate the minimum and maximum values that lie within 1.5 box-lengths of the median. Outlier (between 1.5 and 3 box lengths) and extreme values (>3 box lengths) are shown as open circles or asterisks, respectively.
Statistical Analyses
The relative fold change data from the QPCR were determined to be not normally-distributed by the Shapiro–Wilk test, and to have unequal variances by Levene's test. Therefore, the non-parametric Friedman test for repeated measures was performed on all data sets using RStudio software (RStudio, Inc, Boston, MA, USA). Three comparisons were then made. The first was to assess the ability of the tissues from each animal to respond to T3 treatment. Adequate responsiveness of the tissues to T3 was determined by QPCR analysis: a 2-fold increase of thrb and 1.2-fold decrease of rlk1 was the minimal accepted response. Animals that did not display this response (n = 5 in entire study) were removed from further analysis. The second comparison was to determine NP effects by pairwise analyses of each NP treatment alone relative to the vehicle control (NaOH). The third comparison was to identify NP-induced alteration of the normal T3 response by comparing T3 alone to each T3 + NP combination. All data were expressed as median values in box plots. Statistical significance was considered at p-value < 0.05.
Results and Discussion
For the past three decades, TiO2 was classified as an inert nuisance dust that was only a health risk if large amounts were inhaled (Ferin and Oberdorster, 1985). However, evidence that pulmonary exposure to TiO2 NPs in rats increased the incidence of lung tumors prompted the International Agency for Research on Cancer (IARC) to classify all TiO2 compounds as possibly carcinogenic to humans (class 2B carcinogens) (Baan et al., 2006). Other exposure studies on the effects of TiO2 NPs on biological systems have employed classic toxicological techniques, such as acute exposure of a model organism in order to calculate the LC50 or the use of immortalized cell lines(Jin et al., 2008; Zhu et al., 2008; Baun et al., 2009; Falck et al., 2009). The present study is the first to examine the effects of sublethal concentrations of TiO2 NPs and a micron-sized counterpart within the context and sensitive endpoints of amphibian tissues. Given that smaller particle sizes have been strongly associated with deleterious effects in biological systems (Griffitt et al., 2008; Jiang et al., 2008), the TiO2 NPs were expected to disrupt gene expression to a greater extent than the μ TiO2 particles.
The levels of titanium measured in the nominal 80 mg/L titanium dioxide particle stocks were 40.9–64.7% of the nominal value (Table 1). All of the particles studied were found to form aggregates when suspended in dH2O or L15 medium (Table 1). These aggregates were between 1095.0 and 1285.2 μm in diameter in L15, which was two to five-times greater than dH2O (Table 1). This aggregation is consistent with other studies of TiO2 NPs, which have found that these NPs rapidly coalesce in various natural and buffered liquid matrices (Keller et al., 2010; Thio et al., 2011). Interestingly, the aggregates formed by the NPs were generally comparable in size to those formed by the μ TiO2 (Table 1). The ζ-potential measurements indicated that the TiO2 particles were generally more stable in dH2O than L15 (Table 1). This decreased stability likely contributes to the large degree of aggregation noted by DLS analysis.
We then determined that the tissue biopsies were capable of responding to TH. As expected, treatment with 10 nM T3 consistently increased median levels of thra and thrb mRNAs by 1.7–6.7-fold and 8.2–48.5-fold, respectively, and decreased rlk1 transcript levels by 1.2–20-fold across the four C-fin assays (Figure 2). In contrast, very little if any effect was observed for the three stress indicators, hsp30, sod, and cat (Figure 3). Hsp30 is involved in the cellular defense against heat shock and general stress in many eukaryotes (Nover et al., 1983; Krone and Heikkila, 1988; Helbing et al., 1996). During cellular stress HSP30 and other small heat shock proteins form multimers and bind to denatured proteins to prevent their aggregation until the bound proteins are reactivated by other chaperones and allowed to refold (Heikkila, 2004). The level of hsp30 mRNA was not significantly affected by T3 in any of the exposures. The cellular metabolism of ROS is essential to minimizing the destructive effects of molecules such as hydrogen peroxide and superoxide within the cell. ROS are a byproduct of energy metabolism under normal conditions, but ROS levels can increase dramatically during environmental stress and cause damage to the cellular structure and contents (Valavanidis et al., 2006). The dismutation of superoxide to hydrogen peroxide and molecular oxygen is catalyzed by sod, while cat catalyzes the decomposition of hydrogen peroxide to water and oxygen. The complementary activity of these latter two enzymes means that they are typically coregulated by ROS, both at the mRNA and protein level (Mates et al., 1999; Rodriguez et al., 2004). Induction of stress-response genes was minimal in response to T3 treatment: steady-state levels of sod transcripts were increased modestly by T3 in the P25 exposure set (1.9-fold, Figure 3), and cat transcript levels were slightly decreased in the M212 exposure set (1.5-fold, Figure 3).
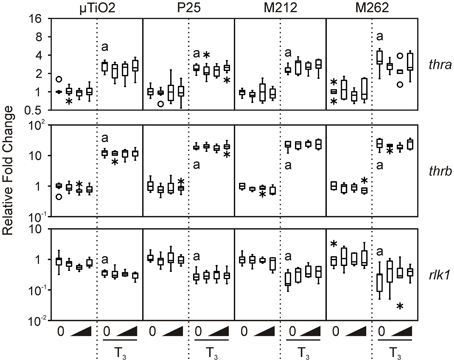
Figure 2. Assessment of the effects of exposure to 0.1, 1.0, and 10 nM μ TiO2, P25, M212, or M262 on thra, thrb, and rlk1 relative transcript levels in R. catesbeiana tailfin biopsies following 48 h exposure to NaOH vehicle or 10 nM T3. Values represent fold-change of steady-state transcript levels relative to each individual's vehicle control baseline as measured by QPCR. Bevels indicate increasing concentrations of the indicated compoundin the absence or presence of T3. Significant response to T3 relative to the vehicle control is indicated by “a” when p ≤ 0.05. thra, thyroid hormone receptor α; thrb, thyroid hormone receptor β ; rlk1, Rana larval keratin type I. See Figure 1 legend for more graph details.
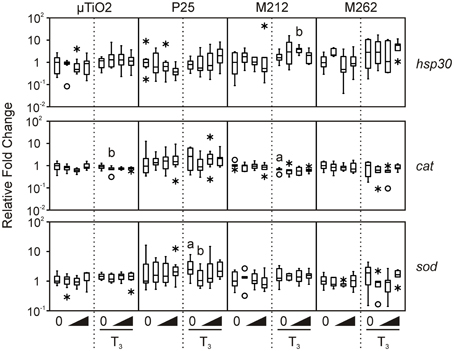
Figure 3. Assessment of the effects of exposure to 0.1, 1.0, and 10 nM μ TiO2, P25, M212, or M262 on hsp30, cat, and sod relative transcript levels in R. catesbeiana tailfin biopsies following 48 h exposure to NaOH vehicle or 10 nM T3. cat, catalase; hsp30, heat shock protein 30; sod, superoxide dismutase. See Figures 1, 2 legends for more graph details. Significance relative to the vehicle control for NPs alone or relative to the T3 only control for the T3-treated plus NP condition is indicated by “b”.
Exposure to μ TiO2, P25, M212, or M262 did not affect the relative abundance of any of the TH-responsive mRNAs examined when tested alone or together with an application of 10 nM T3 (Figure 2). These results are consistent with those observed previously for nanozinc oxide (Hinther et al., 2010b), but are in contrast with previously observed perturbations due to nanosilver and cadmium telluride quantum dots (QDs) (Hinther et al., 2010b).
In the vast majority of TiO2 NP and μ TiO2 exposures, no effect on the stress markers was observed (Figure 3), except in the presence of T3 with either μ TiO2 (0.1 nM: 1.3-fold decrease in cat), P25 (0.1 nM: 2.4-fold decrease in sod), or M212 (1.0 nM: 2.1-fold increase in hsp30) (Figure 3). The minor transcriptional perturbations varied between particles with no dose response or substantial fold changes (Figures 2, 3). Although the unique chemical properties of each could contribute to differential responses between NP types, no overall pattern was observed.
The two most common crystal structures of TiO2 (rutile and anatase) have different photocatalytic and toxic qualities of which the anatase form is considered to be the more potent of the two (Nakagawa et al., 1997; Sayes et al., 2006). The P25 NPs are predominantly anatase, whereas the M212 NPs are solely rutile (Table 1). Both particles had limited effects on expression of the stress-response genes examined (Figure 3), so neither crystal structure would appear to be especially potent in the present context.
The principal difference between the M212 and M262 NPs is their surface functionalizations which give them contrasting interactions with water (Table 1). The surface interactions of particles with their media and each other have been associated with differential effects in chemical and toxicological assays (Tiano et al., 2010). While an increase in hsp30 mRNA detected in the M212 exposure would suggest that the hydrophilic coating may be slightly more stressful to the biopsies in this assay, this difference is minor and agrees with the conclusion of Bolis et al. that the hydrophilic nature of the particle would not appear to be a good predictor of the disruptive potential of TiO2 NPs (Bolis et al., 2012). Moreover, all of the particles tested aggregated to similar degrees in the L15 medium suggesting that the surface area-dependent increase in reactivity associated with NPs was not a contributing factor.
A recent study on nanozinc oxide, nanosilver, and QDs, showed a substantial range between NP types in altering stress-induced transcripts in cultured R. catesbeiana tailfin biopsies (Hinther et al., 2010b). The nanozinc oxide had no effect, whereas the QDs caused massive increase of hsp30 transcripts and depressed cat transcript levels (Hinther et al., 2010b). The data from the present study indicate TiO2 NPs behave more like nanozinc oxide and the minor effects observed do not appear to depend on the surface functionalization or crystal structure.
It has been proposed that ROS generation is the primary cause of cellular perturbation and damage by TiO2 NPs (Gurr et al., 2005; Hao et al., 2009). P25 NPs have been shown to produce ROS in vitro, although at 1 μ g/L ROS levels were not significantly increased over the control (Du et al., 2011). ROS production by TiO2 NPs is enhanced under UV light, and in comparative studies, absence of excitation by UV radiation resulted in a lower degree of cytotoxicity and ROS production (Cermenati et al., 1997; Afaq et al., 1998; Dick et al., 2003; Tiano et al., 2010; Zhang et al., 2012). The C-fin assay is stored in a dark incubator during the 48 h exposure, so the levels of UV-generated ROS are negligible as is shown by the lack of stress response. Future studies should incorporate light exposure and simulate NP aging, both of which could alter the particle's biological effects on amphibian tissues. However, the present study indicates that in the absence of UV exposure, environmentally-relevant concentrations of TiO2 NPs are relatively inert.
Conflict of Interest Statement
The authors declare that the research was conducted in the absence of any commercial or financial relationships that could be construed as a potential conflict of interest.
Acknowledgments
We thank Madjid Hadioui and Kevin Wilkinson for providing the ICP-MS analysis and Dr. Nik Veldhoen for expert technical advice. Funding was provided through the joint funding of Environment Canada, National Research Council-Natural Sciences and Engineering Research Council, and Business Development Canada Nanotechnology Initiative (NNBNI) of Canada.
References
Afaq, F., Abidi, P., Matin, R., and Rahman, Q. (1998). Cytotoxicity, pro-oxidant effects and antioxidant depletion in rat lung alveolar macrophages exposed to ultrafine titanium dioxide. J. Appl. Toxicol. 18, 307–312. doi: 10.1002/(SICI)1099-1263(1998090)18:5<307::AID-JAT508>3.0.CO;2-K
Baan, R., Straif, K., Grosse, Y., Secretan, B., El Ghissassi, F., and Cogliano, V. (2006). Carcinogenicity of carbon black, titanium dioxide, and talc. Lancet Oncol. 7, 295–296. doi: 10.1016/S1470-2045(06)70651-9
Baun, A., Hartmann, N. B., Grieger, K. D., and Hansen, S. F. (2009). Setting the limits for engineered nanoparticles in European surface waters—are current approaches appropriate? J. Environ. Monit. 11, 1774–1781. doi: 10.1039/b909730a
Bolis, V., Busco, C., Ciarletta, M., Distasi, C., Erriquez, J., Fenoglio, I., et al. (2012). Hydrophilic/hydrophobic features of TiO2 nanoparticles as a function of crystal phase, surface area and coating, in relation to their potential toxicity in peripheral nervous system. J. Colloid Interface Sci. 369, 28–39. doi: 10.1016/j.jcis.2011.11.058
Cermenati, L., Pichat, P., Guillard, C., and Albini, A. (1997). Probing the TiO2 photocatalytic mechanisms in water purification by use of quinoline, photo-fenton generated OH. radicals and superoxide dismutase. J. Phys. Chem. B 101, 2650–2658. doi: 10.1021/jp962700p
Clemente, Z., Castro, V. L., Jonsson, C. M., and Fraceto, L. F. (2011). Ecotoxicology of nano-TiO2—an evaluation of its toxicity to organisms of aquatic ecosystems. Int. J. Environ. Res. 6, 33–50.
Colborn, T., Saal, F. S. V., and Soto, A. M. (1993). Developmental effects of endocrine-disrupting chemicals in wildlife and humans. Environ. Health Perspect. 101, 378–384. doi: 10.1289/ehp.93101378
Das, B., Matsuda, H., Fujimoto, K., Sun, G., Matsuura, K., and Shi, Y. B. (2010). Molecular and genetic studies suggest that thyroid hormone receptor is both necessary and sufficient to mediate the developmental effects of thyroid hormone. Gen. Comp. Endocrinol. 168, 174–180. doi: 10.1016/j.ygcen.2010.01.019
Dick, C. A., Brown, D. M., Donaldson, K., and Stone, V. (2003). The role of free radicals in the toxic and inflammatory effects of four different ultrafine particle types. Inhal. Toxicol. 15, 39–52. doi: 10.1080/08958370304454
Domanski, D., and Helbing, C. C. (2007). Analysis of the Rana catesbeiana tadpole tail fin proteome and phosphoproteome during T3-induced apoptosis: identification of a novel type I keratin. BMC Dev. Biol. 7:94. doi: 10.1186/1471-213X-7-94
Du, H., Zhu, X., Fan, C., Xu, S., Wang, Y., and Zhou, Y. (2011). Oxidative damage and OGG1 expression induced by a combined effect of titanium dioxide nanoparticles and lead acetate in human hepatocytes. Environ. Toxicol. 27, 590–597. doi: 10.1002/tox.20682
Falck, G. C. M., Lindberg, H. K., Suhonen, S., Vippola, M., Vanhala, E., Catalan, J., et al. (2009). Genotoxic effects of nanosized and fine TiO2. Hum. Exp. Toxicol. 28, 339–352. doi: 10.1177/0960327109105163
Ferin, J., and Oberdorster, G. (1985). Biological effects and toxicity assessment of titanium dioxides: anatase and rutile. Am. Ind. Hyg. Assoc. J. 46, 69–72. doi: 10.1080/15298668591394419
Griffitt, R. J., Hyndman, K., Denslow, N. D., and Barber, D. S. (2009). Comparison of molecular and histological changes in zebrafish gills exposed to metallic nanoparticles. Toxicol. Sci. 107, 404–415. doi: 10.1093/toxsci/kfn256
Griffitt, R. J., Luo, J., Gao, J., Bonzongo, J. C., and Barber, D. S. (2008). Effects of particle composition and species on toxicity of metallic nanomaterials in aquatic organisms. Environ. Toxicol. Chem. 27, 1972–1978. doi: 10.1897/08-002.1
Gurr, J. R., Wang, A. S., Chen, C. H., and Jan, K. Y. (2005). Ultrafine titanium dioxide particles in the absence of photoactivation can induce oxidative damage to human bronchial epithelial cells. Toxicology 213, 66–73. doi: 10.1016/j.tox.2005.05.007
Hammond, S. A., Veldhoen, N., Kobylarz, M., Webber, N. R., Jordan, J., Rehaume, V., et al. (2013). Characterization of gene expression endpoints during postembryonic development of the northern green frog (Rana clamitans melanota). Zoolog. Sci. 5, 392–401. doi: 10.2108/zsj.30.392
Handy, R. D., von der Kammer, F., Lead, J. R., Hassellov, M., Owen, R., and Crane, M. (2008). The ecotoxicology and chemistry of manufactured nanoparticles. Ecotoxicology 17, 287–314. doi: 10.1007/s10646-008-0199-8
Hao, L. H., Wang, Z. Y., and Xing, B. S. (2009). Effect of sub-acute exposure to TiO2 nanoparticles on oxidative stress and histopathological changes in Juvenile Carp (Cyprinus carpio). J. Environ. Sci. (China) 21, 1459–1466. doi: 10.1016/S1001-0742(08)62440-7
Heikkila, J. J. (2004). Regulation and function of small heat shock protein genes during amphibian development. J. Cell. Biochem. 93, 672–680. doi: 10.1002/jcb.20237
Helbing, C., Gallimore, C., and Atkinson, B. G. (1996). Characterization of a Rana catesbeiana hsp30 gene and its expression in the liver of this amphibian during both spontaneous and thyroid hormone-induced metamorphosis. Dev. Genet. 18, 223–233. doi: 10.1002/(SICI)1520-6408(1996)18:3<223::AID-DVG3>3.3.CO;2-T
Hinther, A., Domanski, D., Vawda, S., and Helbing, C. C. (2010a). C-fin: a cultured frog tadpole tail fin biopsy approach for detection of thyroid hormone-disrupting chemicals. Environ. Toxicol. Chem. 29, 380–388. doi: 10.1002/etc.44
Hinther, A., Vawda, S., Skirrow, R. C., Veldhoen, N., Collins, P., Cullen, J. T., et al. (2010b). Nanometals induce stress and alter thyroid hormone action in amphibia at or below North American water quality guidelines. Environ. Sci. Technol. 44, 8314–8321. doi: 10.1021/es101902n
Jiang, J., Oberdorster, G., Elder, A., Gelein, R., Mercer, P., and Biswas, P. (2008). Does nanoparticle activity depend upon size and crystal phase? Nanotoxicology 2, 33–42. doi: 10.1080/17435390701882478
Jin, C. Y., Zhu, B. S., Wang, X. F., and Lu, Q. H. (2008). Cytotoxicity of titanium dioxide nanoparticles in mouse fibroblast cells. Chem. Res. Toxicol. 21, 1871–1877. doi: 10.1021/tx800179f
Keller, A. A., Wang, H. T., Zhou, D. X., Lenihan, H. S., Cherr, G., Cardinale, B. J., et al. (2010). Stability and aggregation of metal oxide nanoparticles in natural aqueous matrices. Environ. Sci. Technol. 44, 1962–1967. doi: 10.1021/es902987d
Krone, P. H., and Heikkila, J. J. (1988). Analysis of hsp 30, hsp 70 and ubiquitin gene-expression in Xenopus laevis tadpoles. Development 103, 59–67.
Livak, K. J., and Schmittgen, T. D. (2001). Analysis of relative gene expression data using real-time quantitative PCR and the 2-Δ Δ Ct Method. Methods 25, 402–408. doi: 10.1006/meth.2001.1262
Mates, J. M., Perez-Gomez, C., and de Castro, I. N. (1999). Antioxidant enzymes and human diseases. Clin. Biochem. 32, 595–603. doi: 10.1016/S0009-9120(99)00075-2
Nakagawa, Y., Wakuri, S., Sakamoto, K., and Tanaka, N. (1997). The photogenotoxicity of titanium dioxide particles. Mutat. Res. 394, 125–132. doi: 10.1016/S1383-5718(97)00126-5
Nover, L., Scharf, K. D., and Neumann, D. (1983). Formation of cytoplasmic heat-shock granules in tomato cell-cultures and leaves. Mol. Cell. Biol. 3, 1648–1655.
Oberdörster, G., Stone, V., and Donaldson, K. (2007). Toxicology of nanoparticles: a historical perspective. Nanotoxicology 1, 2–25. doi: 10.1080/17435390701314761
Rajapakse, N., Silva, E., and Kortenkamp, A. (2002). Combining xenoestrogens at levels below individual No-observed-effect concentrations dramatically enhances steroid hormone action. Environ. Health Perspect. 110, 917–921. doi: 10.1289/ehp.02110917
Robichaud, C. O., Uyar, A. E., Darby, M. R., Zucker, L. G., and Wiesner, M. R. (2009). Estimates of upper bounds and trends in nano-TiO2 production as a basis for exposure assessment. Environ. Sci. Technol. 43, 4227–4233. doi: 10.1021/es8032549
Rodriguez, C., Mayo, J. C., Sainz, R. M., Antolin, I., Herrera, F., Martin, V., et al. (2004). Regulation of antioxidant enzymes: a significant role for melatonin. J. Pineal Res. 36, 1–9. doi: 10.1046/j.1600-079X.2003.00092.x
Sayes, C. M., Wahi, R., Kurian, P. A., Liu, Y. P., West, J. L., Ausman, K. D., et al. (2006). Correlating nanoscale titania structure with toxicity: a cytotoxicity and inflammatory response study with human dermal fibroblasts and human lung epithelial cells. Toxicol. Sci. 92, 174–185. doi: 10.1093/toxsci/kfj197
Schmid, K., and Riediker, M. (2008). Use of nanoparticles in Swiss industry: a targeted survey. Environ. Sci. Technol. 42, 2253–2260. doi: 10.1021/es071818o
Schreiber, A. M., Das, B., Huang, H., Marsh-Armstrong, N., and Brown, D. D. (2001). Diverse developmental programs of Xenopus laevis metamorphosis are inhibited by a dominant negative thyroid hormone receptor. Proc. Natl. Acad. Sci. U.S.A. 98, 10739–10744. doi: 10.1073/pnas.191361698
Scown, T. M., van Aerle, R., and Tyler, C. R. (2010). Review: do engineered nanoparticles pose a significant threat to the aquatic environment? Crit. Rev. Toxicol. 40, 653–670. doi: 10.3109/10408444.2010.494174
Shaw, B. J., Ramsden, C. S., Turner, A., and Handy, R. D. (2013). A simplified method for determining titanium from TiO2 nanoparticles in fish tissue with a concomitant multi-element analysis. Chemosphere 92, 1136–1144. doi: 10.1016/j.chemosphere.2013.01.065
Shi, Y.-B. (2000). Amphibian Metamorphosis: From Morphology to Molecular Biology. New York, NY: Wiley-Liss.
Tabb, M. M., and Blumberg, B. (2006). New modes of action for endocrine-disrupting chemicals. Mol. Endocrinol. 20, 475–482. doi: 10.1210/me.2004-0513
Taylor, A. C., and Kollros, J. J. (1946). Stages in the normal development of Rana pipiens larvae. Anat. Rec. 94, 7–24. doi: 10.1002/ar.1090940103
Thio, B. J. R., Zhou, D. X., and Keller, A. A. (2011). Influence of natural organic matter on the aggregation and deposition of titanium dioxide nanoparticles. J. Hazard. Mater. 189, 556–563. doi: 10.1016/j.jhazmat.2011.02.072
Tiano, L., Armeni, T., Venditti, E., Barucca, G., Mincarelli, L., and Damiani, E. (2010). Modified TiO2 particles differentially affect human skin fibroblasts exposed to UVA light. Free Radic. Biol. Med. 49:408–415. doi: 10.1016/j.freeradbiomed.2010.04.032
Valavanidis, A., Vlahogianni, T., Dassenakis, M., and Scoullos, M. (2006). Molecular biomarkers of oxidative stress in aquatic organisms in relation to toxic environmental pollutants. Ecotoxicol. Environ. Saf. 64, 178–189. doi: 10.1016/j.ecoenv.2005.03.013
Westerhoff, P., Song, G. X., Hristovski, K., and Kiser, M. A. (2011). Occurrence and removal of titanium at full scale wastewater treatment plants: implications for TiO2 nanomaterials. J. Environ. Monit. 13, 1195–1203. doi: 10.1039/c1em10017c
Wijnhoven, S. W. P., Peijnenburg, W. J. G. M., Herberts, C. A., Hagens, W. I., Oomen, A. G., Heugens, E. H. W., et al. (2009). Nano-silver—a review of available data and knowledge gaps in human and environmental risk assessment. Nanotoxicology 3, U109–U178. doi: 10.1080/17435390902725914
Zhang, J., and Lazar, M. A. (2000). The mechanism of action of thyroid hormones. Annu. Rev. Physiol. 62, 439–466. doi: 10.1146/annurev.physiol.62.1.439
Zhang, J., Wages, M., Cox, S. B., Maul, J. D., Li, Y., Barnes, M., et al. (2012). Effect of titanium dioxide nanomaterials and ultraviolet light coexposure on African clawed frogs (Xenopus laevis). Environ. Toxicol. Chem. 31, 176–183. doi: 10.1002/etc.718
Zhu, X. S., Zhu, L., Duan, Z. H., Qi, R. Q., Li, Y., and Lang, Y. P. (2008). Comparative toxicity of several metal oxide nanoparticle aqueous suspensions to Zebrafish (Danio rerio) early developmental stage. J. Environ. Sci. Health A Tox. Hazard. Subst. Environ. Eng. 43, 278–284. doi: 10.1080/10934520701792779
Keywords: nanometal, nanoparticle, titanium dioxide, thyroid hormone, amphibian, organ culture, oxidative stress
Citation: Hammond SA, Carew AC and Helbing CC (2013) Evaluation of the effects of titanium dioxide nanoparticles on cultured Rana catesbeiana tailfin tissue. Front. Genet. 4:251. doi: 10.3389/fgene.2013.00251
Received: 03 June 2013; Accepted: 04 November 2013;
Published online: 21 November 2013.
Edited by:
Chris Vulpe, University of California, Berkeley, USAReviewed by:
Natàlia Garcia-Reyero, Jackson State University, USAHelen Poynton, University of Massachusetts, Boston, USA
Copyright © 2013 Hammond, Carew and Helbing. This is an open-access article distributed under the terms of the Creative Commons Attribution License (CC BY). The use, distribution or reproduction in other forums is permitted, provided the original author(s) or licensor are credited and that the original publication in this journal is cited, in accordance with accepted academic practice. No use, distribution or reproduction is permitted which does not comply with these terms.
*Correspondence: Caren C. Helbing, Department of Biochemistry and Microbiology, University of Victoria, Petch Building, Ring Road, Victoria, BC V8W 2Y2, Canada e-mail: chelbing@uvic.ca