- 1 Service of Gynecology and Obstetrics, Virgen de la Arrixaca Hospital, Murcia, Spain
- 2 Dr. von Haunersches Kinderspital, Ludwig-Maximilians University, Munich, Germany
- 3 Department of Physiology, Faculty of Biology, University of Murcia, Murcia, Spain
The concentration of long chain polyunsaturated fatty acid (LCPUFA) in the fetal brain increases dramatically from the third trimester until 18 months of life. Several studies have shown an association between the percentage of maternal plasma docosahexaenoic acid (DHA) during gestation and development of cognitive functions in the neonate. Since only very low levels of LCPUFA are synthesized in the fetus and placenta, their primary source for the fetus is the maternal circulation. Both in vitro and human in vivo studies using labeled fatty acids have shown preferential transfer of LCPUFA from the placenta to the fetus compared with other fatty acids, although the mechanisms involved are still uncertain. The placenta takes up circulating maternal non-esterified fatty acids (NEFA) and fatty acids released mainly by maternal lipoprotein lipase and endothelial lipase. These NEFA may enter the cell by passive diffusion or by means of membrane carrier proteins. Once in the cytosol, NEFA bind to cytosolic fatty acid-binding proteins for transfer to the fetal circulation or can be oxidized within the trophoblasts, and even re-esterified and stored in lipid droplets. Although trophoblast cells are not specialized for lipid storage, LCPUFA may up-regulate peroxisome proliferator activated receptor-γ (PPARγ) and hence the gene expression of fatty acid transport carriers, fatty acid acyl-CoA-synthetases and adipophilin or other enzymes involved in lipolysis, modifying the rate of placental transfer, and metabolism. The placental transfer of LCPUFA during pregnancy seems to be a key factor in the neurological development of the fetus. Increased knowledge of the factors that modify placental transfer of fatty acids would contribute to our understanding of this complex process.
Long Chain Polyunsaturated Fatty Acids and Pregnancy
Long chain polyunsaturated fatty acids (LCPUFA), mainly docosahexaenoic acid (DHA, 22:6 n−3), are highly concentrated in the cell membranes of the retina and brain, where they acts as important modulators of membrane function, neurogenesis, photoreceptor differentiation, activation of the visual pigment rhodopsin, the function of ion channels, the activity of several enzymes, and the levels and metabolism of neurotransmitters and eicosanoids (Neuringer et al., 1988; Lauritzen et al., 2001; Innis, 2007). During the last trimester of gestation a spurt in brain growth accompanied by considerable lipid accretion occurs in the human fetus (Widdowson, 1968; Dobbing and Sands, 1979; Martínez, 1992). This period, therefore, is critical for the materno-fetal provision of LCPUFA to the developing central nervous system.
Numerous observational studies have demonstrated that fatty acid concentration in both maternal plasma and erythrocytes increases during pregnancy, as a direct consequence of physiological gestational hyperlipidemia (Otto et al., 1997; Matorras et al., 2001; Vlaardingerbroek and Hornstra, 2004). The concentration of individual fatty acids increases during gestation, although the extent differs between acids. The maximum increase is seen in the concentration of DHA (Stewart et al., 2007). This cannot be explained simply by the modification of dietary habits, and the most accepted hypothesis is its enhanced mobilization from the maternal adipose tissue depots, although an increase in the activity of the enzymes involved in fatty acid synthesis, particularly the synthesis of DHA, in the mother cannot be excluded. The placenta also partly regulates the mobilization of fatty acids from adipose tissue through leptin secretion to the maternal and fetal circulation (Haggarty, 2004), although a leptin resistance situation is produced at the end of pregnancy.
Despite the increase in the absolute fatty acid concentration during pregnancy, the levels of both essential fatty acids (EFA, 18:2 n−6 linoleic acid and 18:3 n−3 α-linolenic acid) and LCPUFA, such as DHA, as a percentage of total fatty acids decreases. This observation suggests that pregnancy is associated with a reduction in the relative amounts of EFA and DHA in the maternal circulation (Al et al., 1995, 2000; Vlaardingerbroek and Hornstra, 2004) emphasizing the importance of these compounds for fetal development.
Fatty Acid Supply to the Fetus
Physiologically, the fetus may synthesize some saturated fatty acids (SFA) and monounsaturated fatty acids (MUFA) de novo from glucose (Nelson, 1992). However, the fetus depends on the placental supply of maternal EFA and LCPUFA (especially arachidonic acid, AA, and DHA). EFA cannot be synthesized by humans and they have to be consumed in the maternal diet. As regards LCPUFA, neither the placenta nor the fetus possesses sufficient desaturase activity to synthesize these fatty acids (Chambaz et al., 1985), which are considered essential nutrients for the fetus. Although endogenous synthesis is likely to be higher in preterm than in term infants (Uauy et al., 2000), the amount of LCPUFA produced from EFA is insufficient to match the in utero accretion rate (Lapillonne and Jensen, 2009). Therefore, the primary source of LCPUFA for the fetus is also that of maternal origin.
In vitro experimental studies (Campbell et al., 1997), placental perfusion studies (Haggarty et al., 1999), and in vivo studies in pregnant women using fatty acids labeled with stable isotopes (Larque et al., 2003; Gil-Sanchez et al., 2010) have shown preferential LCPUFA placental transfer with respect to other fatty acids (Figure 1). In addition, the percentage of LCPUFA in umbilical cord plasma lipids is higher than in the maternal plasma lipids at the time of birth (Crawford et al., 1989; Al et al., 1990; Ruyle et al., 1990; Berghaus et al., 1998), although, the proportion of EFA is lower in the neonate than in the mother. This selective LCPUFA enrichment in the fetal circulation is known as “biomagnification” (Crawford et al., 1976), and highlights the role of the placenta for the preferential transfer of these compounds to the fetus. However, the mechanisms involved are still unknown, and the selective uptake of these compounds by the placenta and even a different rate for lipogenesis/lipolysis within the placenta have been suggested. The mechanisms involved in the preferential transfer of DHA across the placenta is a major issue that needs to be better understood to allow for the design of further nutritional strategies to improve fatty acid availability to the fetus.
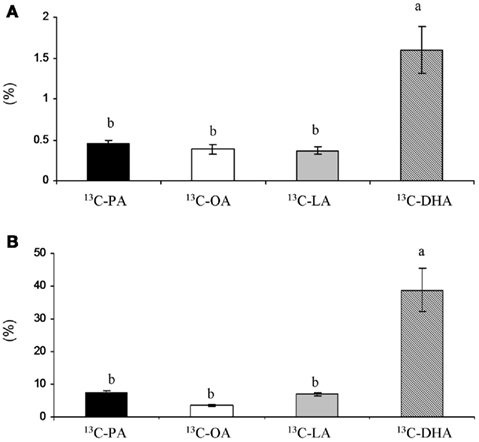
Figure 1. (A) Ratio (%) between placenta and maternal plasma AUC concentration of 13C-fatty acids administered 4 or 12 h before cesarean section. (B) Ratio (%) between cord plasma and maternal plasma AUC concentration of 13C-fatty acids administered 4 or 12 h before cesarean section. Results are expressed as ± SEM. T-test, *P < 0.05.
Mechanisms of Placental Uptake of Fatty Acids from Maternal Circulation
The placenta, like other tissues, may capture non-esterified fatty acids (NEFA) and fatty acids released from maternal lipoproteins by local endothelial lipases (EL). The difference in NEFA concentration between the maternal and fetal circulation increases during gestation and, at the time of delivery, the maternal NEFA concentration is approximately three times that of the fetus (Haggarty, 2004). The maternal hyperlipidemia that occurs during gestation also contributes fatty acids to the fetus, following their release from the maternal lipoproteins by the placental lipoprotein lipase (LPL) and EL (Figure 2).
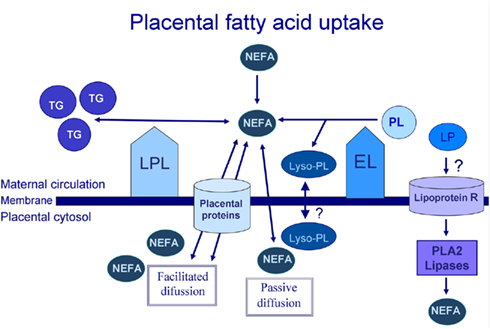
Figure 2. Placental fatty acid uptake process. TG, triglycerides; NEFA, non-esterified fatty acids; PL, phospholipids; Lyso-PL, lysophospholipids; LP, lipoproteins; R, receptor; LPL, lipoprotein lipase; EL, endothelial lipase. PLA2, phospholipase A2.
The LPL enzyme has classically been described as being involved in fatty acid uptake in many tissues, including adipose tissue, muscle, and placental tissue. LPL is an enzyme that is found in the placental microvillus membrane in contact with the maternal blood (Waterman et al., 1998), in the trophoblast and also in isolated macrophages (Bonet et al., 1992). It shows triglyceride lipase activity and is responsible for TG hydrolysis in chylomicrons (CM) and very-low-density lipoproteins (VLDL), releasing two fatty acids for tissue uptake (Figure 2; Mead et al., 2002; Wang and Eckel, 2009). In the literature, this is the most widely accepted mechanism to explain the placental fatty acid uptake process.
Endothelial lipase is a major enzyme in the placenta, regardless of gestational age. At the end of pregnancy, EL continues to be expressed while LPL is virtually absent in the trophoblast. However, in placental vessels, increased expression of both enzymes has been observed (Gauster et al., 2007). EL mainly has A1 phospholipase activity, releasing the fatty acid from the sn−1 position of the phospholipids (PL), and producing a lysophospholipid (lyso-PL), although it also has minimal levels of triglyceride lipase activity (Choi et al., 2002). Since SFA are found predominantly in the sn−1 position of the PL, while polyunsaturated fatty acids are typically esterified in the sn−2 position (Macdonald and Sprecher, 1991), EL activity might result in enhanced levels of maternal plasma lyso-PL enriched in LCPUFA. These lyso-PL could be an additional source of fatty acids for the placenta. This is supported by reports that lyso-phosphatidylcholine in the brain may be a preferred physiological carrier of DHA, rather than NEFA (Thies et al., 1992; Lagarde et al., 2001). Similarly, lyso-phosphatidylcholine seems to deliver 13C-DHA more efficiently to erythrocytes than NEFA (Brossard et al., 1997). Furthermore, some authors have also suggested that maternal erythrocytes, which take lyso-PL into their membranes, may be a potential store of LCPUFA, and a vehicle for their transport to the placenta (Ghebremeskel et al., 2000).
Magnusson et al. (2004) investigated placental LPL in normal gestations in comparison with gestations complicated by intrauterine growth restriction (IUGR), insulin dependent diabetes mellitus (IDDM), or gestational diabetes mellitus (GDM). This study demonstrated that the placental LPL activity was reduced by 47% in IUGR, but increased by 39% in IDDM with respect to the controls. Lindegaard et al. (2006) reported increases in placental LPL activity in gestations complicated with IDDM in association with fetal overgrowth. Hence, these studies support the hypothesis that alterations in LPL contribute to changes in the transport of the fatty acids to the fetus and, consequently may modify its growth (Magnusson et al., 2004).
Other lipases that could be involved in the release of fatty acids from maternal plasma lipoproteins are phospholipase A2 type II, which is mainly expressed in placenta and the choriodecidua (Freed et al., 1997), and triacylglycerol hydrolase, with a pH optimum (6.0) that differs from LPL, which is expressed in the syncytiotrophoblast microvillus membrane (Waterman et al., 2000). Part of the phospholipase A2 type II is secreted to the placental extracellular compartment, where it continues to be active, not only intracellularly, but also extracellularly (Rice et al., 1998). Also, the placental tissue shows lipoprotein receptors of HDL, LDL, etc. (Naoum et al., 1987; Herrera, 2002). Thus, after binding to these receptors, some lipoproteins can undergo endocytosis to provide cholesterol to the placenta; this last mechanism could also provide fatty acids to placental tissue once they are released by the intracellular lipases, although the relevance of this mechanism for the placental uptake of fatty acids is still uncertain.
Fatty Acid Carriers in Placental Membranes
Once fatty acids have been released by the lipases as NEFA, they enter the cell through passive diffusion or by means of membrane carrier proteins known as fatty acid-binding protein plasma membrane (FABPpm), placental plasma membrane fatty acid-binding protein (p-FABPpm), fatty acid translocase (FAT/CD36), and fatty acid transport proteins (FATP-1 to -6; Dutta-Roy, 2000; Hanebutt et al., 2008; Figure 3). These proteins are located in the syncytiotrophoblast basal and microvillus membrane, except for p-FABPpm, which has only been reported in the microvillus membrane (Campbell et al., 1998). FATPS, FAT/CD36, and FABPpm have been described in many tissues, but only p-FABPpm seems to be exclusive to placental tissue (Campbell and Dutta-Roy, 1995).
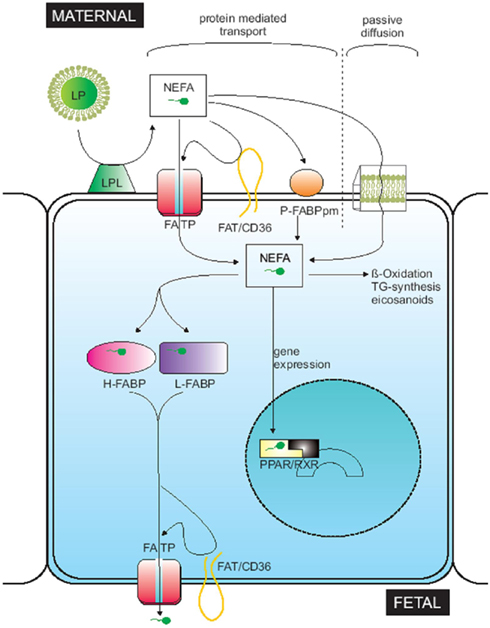
Figure 3. Figure taken from Hanebutt et al. (2008). Theoretical model of fatty acid movement across placental tissue. LP, lipoprotein; LPL, lipoprotein lipase; NEFA, non-esterified fatty acid; FATP, fatty acid transport protein; FAT, fatty acid translocase; P-FABPpm, placental plasma membrane fatty acid binding protein; L-FABP, liver-fatty acid binding protein; H-FABP, heart-fatty acid binding protein; PPAR, peroxisome proliferator activated receptor; RXR, retinoid X receptor.
Although the role of these membrane proteins in the placental fatty acid uptake, metabolism, and transfer process is not completely understood, it has been suggested that the enrichment of LCPUFA in the fetal circulation could be related to their interaction with some fatty acid carriers such as p-FABPpm (Campbell and Dutta-Roy, 1995; Larque et al., 2006) and FATP4 (Larque et al., 2006).
BeWo cells incubated with a p-FABPpm antibody reduced their uptake of DHA and AA by 64 and 68%, respectively, while OA uptake was only reduced by 32% (Campbell et al., 1997). These findings suggest that p-FABPpm could be directly involved in DHA and AA uptake. Nevertheless, the existence of p-FABPpm has only been demonstrated by Dutta-Roy’s group and has not even been genetically sequenced, so that more evidence concerning the structure and function of this protein in the placenta is needed.
When Larque et al. (2006) evaluated the association between DHA dietary supplementation and placental transport protein gene expression in pregnant women, they found that placental FATP1 and FATP4 gene expressions were directly correlated with the percentage of DHA in the placental and maternal plasma PL. Furthermore, FATP4 expression was significantly correlated with the DHA in the PL of fetal blood. These data suggest that FATP4 could be involved in the selective placental transport of DHA and so of n−3 LCPUFA. FATP is a family of proteins that presents acyl-coA-synthetase activity and may directly translocate free fatty acids across the membrane and also direct acyl-CoA conversion (Jia et al., 2007), facilitating their metabolism within the cells.
LCPUFA could themselves have an important regulatory function in fatty acid trophoblast uptake and metabolism through the direct modification of intracellular acyl-CoA-synthetase gene expression (Johnsen et al., 2009), which could also influence LCPUFA biomagnification in comparison with other fatty acids.
In an article published in Science, de Urquiza et al. (2000) reported that DHA acts as a powerful activator of the retinoid X receptor (RXR). Therefore, DHA may modulate fatty acid carrier expression through activation of RXR signaling pathways in the placenta, brain or other tissue, and thus alter the expression of adipophilin, acyl-CoA-synthetase, or other enzymes related to lipid metabolism.
Intracellular Movement of Fatty Acids within the Placenta
Once the fatty acids are in the cytosol, they bind to cytosolic fatty acid-binding proteins (FABP) for movement. Richieri et al. (2000) studied FABP affinity and did not observe any particular fatty acid affinity, although the authors described how the affinity was reduced with short chain fatty acids and increased as the number of double bonds increased. Nine FABP have been identified in mammals, with different tissue expression patterns and with common and specific functions (Storch and Corsico, 2008).
Fatty acid-binding protein structure-function studies suggest that the three-dimensional changes that result from fatty acid binding may determine the particular function of the FABP (Storch and McDermott, 2009). In mammals, FABPs are believed to act in the cytoplasmic compartment by means of specific interactions with subcellular organelles, including the endoplasmic reticulum, mitochondria, lipid droplets (LDs), and peroxisomes. Coordinate function with the membrane fatty acid carrier FAT/CD36 and the cytosolic enzyme hormone-sensitive lipase (HSL) have also been reported (Figure 4). FABPs are also likely to function in the nucleus through the delivery of specific ligands to nuclear transcription factors, such as the peroxisome proliferators-activated receptors (PPAR; reviewed by Storch and Corsico, 2008).
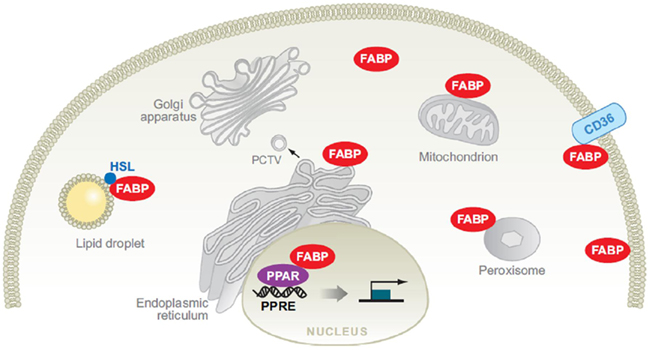
Figure 4. Potential functions of fatty acid-binding proteins (FABPs) in intracellular fatty acid disposition. In Storch and Corsico (2008).
The following FABP have been described in the placenta: FABP1 or liver FABP (L-FABP), FABP3 or heart FABP (H-FABP), FABP4 or adipocyte FABP (A-FABP), FABP5 or keratinocyte FABP (K-FABP), and FABP7 or brain FABP (B-FABP; Cunningham and McDermott, 2009).
Campbell et al. (1998) were the first to describe L-FABP and H-FABP expression in human syncytiotrophoblast. Biron-Shental et al. (2007) detected L-FABP, A-FABP, and H-FABP gene expression in primary human trophoblast and in placental tissue. However, the presence of A-FABP in macrophages has been described (Storch and Corsico, 2008), and it would be interesting to know the localization of A-FABP in the placenta (trophoblast or macrophages). As regards B-FABP expression in the trophoblast, two recent studies provided discrepancies in their results (Larque et al., 2006; Biron-Shental et al., 2007).
Storage of Fatty Acids in Lipid Droplets
Non-esterified fatty acids can be oxidized within trophoblast or re-esterified and stored as TG and other lipid fractions in the placental cells. As in other cells, excessive cellular concentrations of NEFA are toxic and, to avoid toxicity, fatty acids are esterified with glycerol, and the resulting TG deposited in LDs. Later, the fatty acids can be released from cellular LD by the action of TG hydrolases in a process called lipolysis (Coleman and Haynes, 1987; Haggarty et al., 1999; Larque et al., 2006). The differential fatty acid processing inside the placenta may also affect the rate of fatty acid transfer to the fetal circulation.
Lipid droplets
The basic structure of the LD is similar to that of lipoproteins: a hydrophobic core of neutral lipids (TG and CE) surrounded by a membrane monolayer of PL with associated coat proteins. While lipoproteins and LDs both contribute to neutral lipid trafficking, their functions are different. Lipoproteins participate in extracellular lipid trafficking, while LDs are confined to the cytoplasm, where they mediate intracellular lipid storage, and metabolism. Together with membrane bilayers, LDs represent one of the two major intracellular lipid reservoirs (Ackerman et al., 2007).
Lipid droplets exhibit a marked heterogeneity regarding size, cellular location, and associated protein composition within a given cell or between different tissues. Recent advances in our understanding of LD formation and function have emphasized the importance of LD-associated proteins, the so-called PAT family. PAT is a family of proteins who were initially constituted by three members: Perilipin (with 3 isoforms A, B, C), Adipophilin (or adipocyte differentiation-related protein, ADRP), and tail-interacting protein of 47 kDa (TIP47). Two other proteins, S3–12 and oxidative tissues-enriched PAT protein (OXPAT), or myocardial lipid droplet protein (MLDP) or lipid storage droplet protein 5 (LSDP5), were further bound to the PAT family in mice and humans (Bickel et al., 2009).
PAT proteins protect the neutral lipids stored in the LD from hydrolysis by cytoplasmatic lipases. It is known that only perilipin, among mammal PAT proteins, changes its activity depending on whether it has been phosphorylated by protein kinase A (PKA) or not. In the basal state, perilipin inhibits TG hydrolysis in adipocytes. However, upon phosphorylation, the role of perilipin A shifts from storage to the mobilization of stored neutral lipids, promoting lipolysis.
In recent years, the newly discovered protein–protein interactions between perilipin and the intracellular lipases adipose triglyceride lipase (ATGL) and HSL, as well as the ATGL activator comparative gene identification-58 (CGI-58), have provided clues as to how perilipin might organize lipolysis, although the exact mechanisms remains unclear (Zimmermann et al., 2009). On the other hand, adipophilin would impair the lipolysis, preventing the association of these lipases to LD, although the regulation of this mechanism remains to be elucidated (Bickel et al., 2009). Nevertheless, in contrast to adipocytes and monocytes, trophoblast cells are not specialized for lipid storage, and the role of the PAT family (especially, adipophilin, perilipin) in the trophoblasts might even differ from their role in other cell types. Better knowledge of the activity of these proteins in the placenta would improve our understanding of the fatty acid transport process in this tissue.
Adipophilin is expressed in human villous trophoblast, where it inhibits the process of lipolysis (Bildirici et al., 2003). Furthermore, the expression of adipophilin is regulated by PPARγ, a protein that plays a crucial role in the development of the rodent fetal–placental unit and in human trophoblast differentiation (Barak et al., 1999; Schaiff et al., 2000). PPARγ ablation is associated with a reduced number of LD in rodent placenta (Barak et al., 1999), which suggests that the trophoblast fatty acid accumulation is necessary in LD formation, and that it is regulated during trophoblast differentiation and placental development.
DHA might regulate its own metabolic intracellular metabolism, through the control of lipolysis. LCPUFA are more efficient at stimulating the expression of adipophilin mRNA in BeWo cells than short chain fatty acids (Tobin et al., 2006). In one study, Suzuki et al. (2009) described that the incubation of BeWo cells with DHA, induced adipophilin expression, by acting as an agonist of the RXR. BeWo cells incubated with oleic acid showed a greater accumulation of TG and LD than when incubated with DHA; however, DHA transfer through the BeWo cell layer was four times higher than the corresponding transfer of oleic acid; Tobin et al. (2009) suggested that the lower accumulation of DHA in the LD may result in a greater placental transfer.
Lipolysis
The current evidence suggests that intracellular TG hydrolysis occurs in a sequential way that involves different lipases. The principal TG-catabolic enzymes are the HSL and ATGL (reviewed in adipocytes by Zimmermann et al., 2009).
Waterman et al. (1998) First identified the existence of HSL (pH 6.0) in placental cytosol. HSL it is an intracellular enzyme and is active in energy demanding processes. It is activated through phosphorylation, which may be mediated by several hormones including catecholamines, growth hormone, serotonin, and glucagon, while insulin inactivates it by dephosphorylation. HSL has been shown to exhibit broad substrate specificity, and is capable of hydrolyzing cholesterylester, tri-, di-, and mono-acylglycerol, retinyl ester, and numerous water soluble ester substrates. The last step of the lipolysis is accomplished by monoglyceride lipase (MGL), which hydrolyses monoglycerides to form glycerol and to release a free fatty acid. Lindegaard et al. (2006) found an increase in HSL and EL mRNA expression in the placenta of diabetic women, which could reflect the movement of fatty acids to the fetus that occurs in diabetic pregnancies.
Adipose triglyceride lipase selectively performs the first step in TG hydrolysis, resulting in the formation of diacylglycerol (DG) and NEFA. However, to date, ATGL has not been identified in the placenta, although its possible involvement in the regulation of the placental transfer of fatty acids, cannot be excluded.
Exportation of fatty acids from placental tissue to fetal circulation. The most efficient system to transport lipids from tissues to blood involves the synthesis and secretion of apoB-containing lipoproteins. These lipoproteins can contain large amounts of TG and also act as carriers of essential lipids (lipophilic vitamins and glycolipids; Davis, 1999). Madsen et al. (2004) demonstrated that human placenta may synthesize and produce apoB, as well as a kind of lipoprotein that could transport lipids to the fetal circulation. However, the extent of placental lipoprotein secretion capacity is still controversial, and most studies indicate that the placenta transports intracellular fatty acids to the fetal circulation directly through facilitated diffusion or via fatty acids transport proteins, FAT/CD36, and FATP, located in the syncytiotrophoblast basal membrane (Hanebutt et al., 2008).
Once in the fetal circulation, the fatty acids bind to α-fetoprotein and reach the liver where they are re-esterified and exported to the fetal circulation within the lipoproteins (mainly as TG). The placenta may re-accept NEFA but not esterified fatty acids. The selective esterification of LCPUFA within the fetal circulation has been suggested as another possible mechanism for the selective accumulation of certain LCPUFA in the fetal circulation (Kuhn and Crawford, 1986).
Placental Fatty Acid Transfer in Complicated Pregnancies
An impaired materno-fetal LCPUFA transport may occur in pregnancies that are complicated by abnormal placental function, for example GDM or IUGR (Gauster et al., 2007). GDM is a pathological condition in which glucose intolerance is first detected during pregnancy. In GDM a significant discrepancy between normal LCPUFA contents in erythrocyte and plasma phospholipids of the mother but reduced LCPUFA contents in plasma phospholipids of the neonate has been reported (Wijendran et al., 1999, 2000; Thomas et al., 2004). These data suggest that LCPUFA transport from the placenta to the fetal circulation could be impaired in women with gestational diabetes. The poor LCPUFA status in the newborns of diabetic mothers could in part be responsible for the delayed brain maturity in these newborns compared to controls. Indeed, maternal diabetes during pregnancy has been reported to affect behavioral and intellectual development in the offspring (Rizzo et al., 1991). Pregestational or GDM was found to adversely affect attention span and motor function of the offspring at school age (Ornoy et al., 2001). These effects were negatively correlated with the degree of maternal glycemic control. Thus, it will be important to explore in future studies the extent to which fatty acid transfer is altered by diabetes in pregnancy, and whether such alterations can be overcome by adaptation of maternal dietary intake.
The placental transfer of n−3 LCPUFA during pregnancy may influence the neurological development of the fetus and several studies have shown an association between maternal plasma DHA status, dietary intake of DHA during gestation, and the development of cognitive functions in the neonate and thereafter in the child (Helland et al., 2001, 2003; Hibbeln et al., 2007). The influence of dietary fatty acids on the activity of fatty acid carriers and enzymes related with lipolysis and lipid storage within the placenta is under evaluation in pregnancies showing normal and abnormal placental function.
In conclusion, numerous mechanisms have evolved to protect human offspring from LCPUFA deficiency in the maternal diet during pregnancy. Placental tissue preferentially take up LCPUFA from the maternal circulation and regulate their transfer to fetal circulation. However the mechanisms used for such regulation are still under evaluation. The study of enzymes related to fatty acid metabolism within the placenta in normal and abnormal placentas may contribute in the future to clarifying the relevance of the different steps for the preferential transfer of LCPUFA and future nutritional strategies to improve health in neonates.
Conflict of Interest Statement
The authors declare that the research was conducted in the absence of any commercial or financial relationships that could be construed as a potential conflict of interest.
References
Ackerman, W. E., Robinson, J. M., and Kniss, D. A. (2007). Association of PAT proteins with lipid storage droplets in term fetal membranes. Placenta 28, 465–476.
Al, M. D., Hornstra, G., van der Schouw, Y. T., Bulstra-Ramakers, M. T., and Huisjes, H. J. (1990). Biochemical EFA status of mothers and their neonates after normal pregnancy. Early Hum. Dev. 24, 239–248.
Al, M. D., van Houwelingen, A. C., and Hornstra, G. (2000). Long-chain polyunsaturated fatty acids, pregnancy, and pregnancy outcome. Am. J. Clin. Nutr. 71, 285S–291S.
Al, M. D., van Houwelingen, A. C., Kester, A. D., Hasaart, T. H., de Jong, A. E., and Hornstra, G. (1995). Maternal essential fatty acid patterns during normal pregnancy and their relationship to the neonatal essential fatty acid status. Br. J. Nutr. 74, 55–68.
Barak, Y., Nelson, M. C., Ong, E. S., Jones, Y. Z., Ruiz-Lozano, P., Chien, K. R., Koder, A., and Evans, R. M. (1999). PPAR gamma is required for placental, cardiac, and adipose tissue development. Mol. Cell 4, 585–595.
Berghaus, T. M., Demmelmair, H., and Koletzko, B. (1998). Fatty acid composition of lipid classes in maternal and cord plasma at birth. Eur. J. Pediatr. 157, 763–768.
Bickel, P. E., Tansey, J. T., and Welte, M. A. (2009). PAT proteins, an ancient family of lipid droplet proteins that regulate cellular lipid stores. Biochim. Biophys. Acta 1791, 419–440.
Bildirici, I., Roh, C. R., Schaiff, W. T., Lewkowski, B. M., Nelson, D. M., and Sadovsky, Y. (2003). The lipid droplet-associated protein adipophilin is expressed in human trophoblasts and is regulated by peroxisomal proliferator-activated receptor-gamma/retinoid X receptor. J. Clin. Endocrinol. Metab. 88, 6056–6062.
Biron-Shental, T., Schaiff, W. T., Ratajczak, C. K., Bildirici, I., Nelson, D. M., and Sadovsky, Y. (2007). Hypoxia regulates the expression of fatty acid-binding proteins in primary term human trophoblasts. Am. J. Obstet. Gynecol. 197, 516.
Bonet, B., Brunzell, J. D., Gown, A. M., and Knopp, R. H. (1992). Metabolism of very-low-density lipoprotein triglyceride by human placental cells: the role of lipoprotein lipase. Metab. Clin. Exp. 41, 596–603.
Brossard, N., Croset, M., Normand, S., Pousin, J., Lecerf, J., Laville, M., Tayot, J. L., and Lagarde, M. (1997). Human plasma albumin transports [13C]docosahexaenoic acid in two lipid forms to blood cells. J. Lipid Res. 38, 1571–1582.
Campbell, F. M., Bush, P. G., Veerkamp, J. H., and Dutta-Roy, A. K. (1998). Detection and cellular localization of plasma membrane-associated and cytoplasmic fatty acid-binding proteins in human placenta. Placenta 19, 409–415.
Campbell, F. M., Clohessy, A. M., Gordon, M. J., Page, K. R., and Dutta-Roy, A. K. (1997). Uptake of long chain fatty acids by human placental choriocarcinoma (BeWo) cells: role of plasma membrane fatty acid-binding protein. J. Lipid Res. 38, 2558–2568.
Campbell, F. M., and Dutta-Roy, A. K. (1995). Plasma membrane fatty acid-binding protein (FABPpm) is exclusively located in the maternal facing membranes of the human placenta. FEBS Lett. 375, 227–230.
Chambaz, J., Ravel, D., Manier, M. C., Pepin, D., Mulliez, N., and Bereziat, G. (1985). Essential fatty acids interconversion in the human fetal liver. Biol. Neonate 47, 136–140.
Choi, S. Y., Hirata, K., Ishida, T., Quertermous, T., and Cooper, A. D. (2002). Endothelial lipase: a new lipase on the block. J. Lipid. Res. 43, 1763–1769.
Coleman, R. A., and Haynes, E. B. (1987). Synthesis and release of fatty acids by human trophoblast cells in culture. J. Lipid Res. 28, 1335–1341.
Crawford, M. A., Doyle, W., Drury, P., Lennon, A., Costeloe, K., and Leighfield, M. (1989). n-6 and n-3 fatty acids during early human development. J. Intern. Med. 731, S159–S169.
Crawford, M. A., Hassam, A. G., and Williams, G. (1976). Essential fatty acids and fetal brain growth. Lancet 1, 452–543.
Cunningham, P., and McDermott, L. (2009). Long chain PUFA transport in human term placenta. J. Nutr. 139, 636–639.
Davis, R. A. (1999). Cell and molecular biology of the assembly and secretion of apolipoprotein B-containing lipoproteins by the liver. Biochim. Biophys. Acta 25, 1–31.
de Urquiza, A. M., Liu, S., Sjoberg, M., Zetterstrom, R. H., Griffiths, W., Sjovall, J., and Perlmann, T. (2000). Docosahexaenoic acid, a ligand for the retinoid X receptor in mouse brain. Science 290, 2140–2144.
Dobbing, J., and Sands, J. (1979). Comparative aspects of the brain growth spurt. Early Hum. Dev. 3, 79–83.
Dutta-Roy, A. K. (2000). Cellular uptake of long-chain fatty acids: role of membrane-associated fatty-acid-binding/transport proteins. Cell. Mol. Life Sci. 57, 1360–1372.
Freed, K. A., Moses, E. K., Brennecke, S. P., and Rice, G. E. (1997). Differential expression of type II, IV and cytosolic PLA2 messenger RNA in human intrauterine tissues at term. Mol. Hum. Reprod. 3, 493–499.
Gauster, M., Hiden, U., Blaschitz, A., Frank, S., Lang, U., Alvino, G., Cetin, I., Desoye, G., and Wadsack, C. (2007). Dysregulation of placental endothelial lipase and lipoprotein lipase in intrauterine growth-restricted pregnancies. J. Clin. Endocrinol. Metab. 92, 2256–2263.
Ghebremeskel, K., Min, Y., Crawford, M. A., Nam, J. H., Kim, A., Koo, J. N., and Suzuki, H. (2000). Blood fatty acid composition of pregnant and nonpregnant Korean women: red cells may act as a reservoir of arachidonic acid and docosahexaenoic acid for utilization by the developing fetus. Lipids 35, 567–574.
Gil-Sanchez, A., Larque, E., Demmelmair, H., Acien, M. I., Faber, F. L., Parrilla, J. J., and Koletzko, B. (2010). Maternal-fetal in vivo transfer of [13C]docosahexaenoic and other fatty acids across the human placenta 12 h after maternal oral intake. Am. J. Clin. Nutr. 92, 115–122.
Haggarty, P. (2004). Effect of placental function on fatty acid requirements during pregnancy. Eur. J. Clin. Nutr. 58, 1559–1570.
Haggarty, P., Ashton, J., Joynson, M., Abramovich, D. R., and Page, K. (1999). Effect of maternal polyunsaturated fatty acid concentration on transport by the human placenta. Biol. Neonate 75, 350–359.
Hanebutt, F. L., Demmelmair, H., Schiessl, B., Larque, E., and Koletzko, B. (2008). Long-chain polyunsaturated fatty acid (LCPUFA) transfer across the placenta. Clin. Nutr. 27, 685–693.
Helland, I. B., Saugstad, O. D., Smith, L., Saarem, K., Solvoll, K., Ganes, T., and Drevon, C. A. (2001). Similar effects on infants of n-3 and n-6 fatty acids supplementation to pregnant and lactating women. Pediatrics 108, E82.
Helland, I. B., Smith, L., Saarem, K., Saugstad, O. D., and Drevon, C. A. (2003). Maternal supplementation with very-long-chain n-3 fatty acids during pregnancy and lactation augments children’s IQ at 4 years of age. Pediatrics 111, e39–e44.
Herrera, E. (2002). Implications of dietary fatty acids during pregnancy on placental, fetal and postnatal development – a review. Placenta 23, S9–S19.
Hibbeln, J. R., Davis, J. M., Steer, C., Emmett, P., Rogers, I., Williams, C., and Golding, J. (2007). Maternal seafood consumption in pregnancy and neurodevelopmental outcomes in childhood (ALSPAC study): an observational cohort study. Lancet 369, 578–585.
Jia, Z., Pei, Z., Maiguel, D., Toomer, C. J., and Watkins, P. A. (2007). The fatty acid transport protein (FATP) family: very long chain acyl-CoA synthetases or solute carriers? J. Mol. Neurosci. 33, 25–31.
Johnsen, G. M., Weedon-Fekjaer, M. S., Tobin, K. A., Staff, A. C., and Duttaroy, A. K. (2009). Long-chain polyunsaturated fatty acids stimulate cellular fatty acid uptake in human placental choriocarcinoma (BeWo) cells. Placenta 30, 1037–1044.
Kuhn, D. C., and Crawford, M. (1986). Placental essential fatty acid transport and prostaglandin synthesis. Prog. Lipid Res. 25, 345–353.
Lagarde, M., Bernoud, N., Brossard, N., Lemaitre-Delaunay, D., Thies, F., Croset, M., and Lecerf, J. (2001). Lysophosphatidylcholine as a preferred carrier form of docosahexaenoic acid to the brain. J. Mol. Neurosci. 16, 201–204.
Lapillonne, A., and Jensen, C. L. (2009). Reevaluation of the DHA requirement for the premature infant. Prostaglandins Leukot. Essent. Fatty Acids 81, 143–150.
Larque, E., Demmelmair, H., Berger, B., Hasbargen, U., and Koletzko, B. (2003). In vivo investigation of the placental transfer of (13)C-labeled fatty acids in humans. J. Lipid Res. 44, 49–55.
Larque, E., Krauss-Etschmann, S., Campoy, C., Hartl, D., Linde, J., Klingler, M., Demmelmair, H., Caño, A., Gil, A., Bondy, B., and Koletzko, B. (2006). Docosahexaenoic acid supply in pregnancy affects placental expression of fatty acid transport proteins. Am. J. Clin. Nutr. 84, 853–861.
Lauritzen, L., Hansen, H. S., Jorgensen, M. H., and Michaelsen, K. F. (2001). The essentiality of n-3 long chain fatty acids in relation to development and function of the brain and retina. Prog. Lipid Res. 40, 1–94.
Lindegaard, M. L., Damm, P., Mathiesen, E. R., and Nielsen, L. B. (2006). Placental triglyceride accumulation in maternal type 1 diabetes is associated with increased lipase gene expression. J. Lipid Res. 47, 2581–2588.
Macdonald, J. I., and Sprecher, H. (1991). Phospholipid fatty acid remodeling in mammalian cells. Biochim. Biophys. Acta 1084, 105–121.
Madsen, E. M., Lindegaard, M. L., Andersen, C. B., Damm, P., and Nielsen, L. B. (2004). Human placenta secretes apolipoprotein B-100-containing lipoproteins. J. Biol. Chem. 31, 55271–55276.
Magnusson, A. L., Waterman, I. J., Wennergren, M., Jansson, T., and Powell, T. L. (2004). Triglyceride hydrolase activities and expression of fatty acid binding proteins in the human placenta in pregnancies complicated by intrauterine growth restriction and diabetes. J. Clin. Endocrinol. Metab. 89, 4607–4614.
Martínez, M. (1992). Tissue levels of polyunsaturated fatty acids during early human development. J. Pediatr. 120, S129–S138.
Matorras, R., Ruiz, J. I., Perteagudo, L., Barbazan, M. J., Diaz, A., Valladolid, A., and Sanjurjo, P. (2001). Longitudinal study of fatty acids in plasma and erythrocyte phospholipids during pregnancy. J. Perinat. Med. 29, 293–297.
Mead, J. R., Irvine, S. A., and Ramji, D. P. (2002). Lipoprotein lipase: structure, function, regulation, and role in disease. J. Mol. Med. 80, 753–769.
Naoum, H. G., De Chazal, R. C., Eaton, B. M., and Contractor, S. F. (1987). Characterization and specificity of lipoprotein binding to term human placental membranes. Biochim. Biophys. Acta 902, 193–199.
Nelson, G. J. (1992). “Dietary fatty acids and lipid metabolism,” in Fatty Acids in Foods and Their Health Implications, ed. C. K. Chow (New York: Dekker Inc.), 437–472.
Neuringer, M., Anderson, G. J., and Connor, W. E. (1988). The essentiality of n-3 fatty acids for the development and function of the retina and brain. Ann. Rev. Nutr. 8, 517–541.
Ornoy, A., Ratzon, N., Greenbaum, C., Wolf, A., and Dulitzky, M. (2001). School-age children born to diabetic mothers and to mothers with gestational diabetes exhibit a high rate of inattention and fine and gross motor impairment. J. Pediatr. Endocrinol. Metab. 14(Suppl. 1), 681–689.
Otto, S. J., Houwelingen, A. C., Antal, M., Manninen, A., Godfrey, K., Lopez-Jaramillo, P., and Hornstra, G. (1997). Maternal and neonatal essential fatty acid status in phospholipids: an international comparative study. Eur. J. Clin. Nutr. 51, 232–242.
Rice, G. E., Wong, M. H., Farrugia, W., and Scott, K. F. (1998). Contribution of type II phospholipase A2 to in vitro phospholipase A2 enzymatic activity in human term placenta. J. Endocrinol. 157, 25–31.
Richieri, G. V., Ogata, R. T., Zimmerman, A. W., Veerkamp, J. H., and Kleinfeld, A. M. (2000). Fatty acid binding proteins from different tissues show distinct patterns of fatty acid interactions. Biochemistry 39, 7197–7204.
Rizzo, T., Metzger, B. E., Burns, W. J., and Burns, K. (1991). Correlations between antepartum maternal metabolism and child intelligence. N. Engl. J. Med. 325, 911–916.
Ruyle, M., Connor, W. E., Anderson, G. J., and Lowensohn, R. I. (1990). Placental transfer of essential fatty acids in humans: venous-arterial difference for docosahexaenoic acid in fetal umbilical erythrocytes. Proc. Natl. Acad. Sci. U.S.A. 87, 7902–7906.
Schaiff, W. T., Carlson, M. G., Smith, S. D., Levy, R., Nelson, D. M., and Sadovsky, Y. (2000). Peroxisome proliferator-activated receptor-gamma modulates differentiation of human trophoblast in a ligand-specific manner. J. Clin. Endocrinol. Metab. 85, 3874–3881.
Stewart, F., Rodie, V. A., Ramsay, J. E., Greer, I. A., Freeman, D. J., and Meyer, B. J. (2007). Longitudinal assessment of erythrocyte fatty acid composition throughout pregnancy and post partum. Lipids 42, 335–344.
Storch, J., and Corsico, B. (2008). The emerging functions and mechanisms of mammalian fatty acid-binding proteins. Ann. Rev. Nutr. 28, 73–95.
Storch, J., and McDermott, L. (2009). Structural and functional analysis of fatty acid-binding proteins. J. Lipid Res. 50(Suppl), S126–S131.
Suzuki, K., Takahashi, K., Nishimaki-Mogami, T., Kagechika, H., Yamamoto, M., and Itabe, H. (2009). Docosahexaenoic acid induces adipose differentiation-related protein through activation of retinoid x receptor in human choriocarcinoma BeWo cells. Biol. Pharm. Bull. 32, 1177–1182.
Thies, F., Delachambre, M. C., Bentejac, M., Lagarde, M., and Lecerf, J. (1992). Unsaturated fatty acids esterified in 2-acyl-l-lysophosphatidylcholine bound to albumin are more efficiently taken up by the young rat brain than the unesterified form. J. Neurochem. 59, 1110–1116.
Thomas, B., Ghebremeskel, K., Lowy, C., Min, Y., and Crawford, M. A. (2004). Plasma AA and DHA levels are not compromised in newly diagnosed gestational diabetic women. Eur. J. Clin. Nutr. 58, 1492–1497.
Tobin, K. A., Harsem, N. K., Dalen, K. T., Staff, A. C., Nebb, H. I., and Duttaroy, A. K. (2006). Regulation of ADRP expression by long-chain polyunsaturated fatty acids in BeWo cells, a human placental choriocarcinoma cell line. J. Lipid Res. 47, 815–823.
Tobin, K. A., Johnsen, G. M., Staff, A. C., and Duttaroy, A. K. (2009). Long-chain polyunsaturated fatty acid transport across human placental choriocarcinoma (BeWo) cells. Placenta 30, 41–47.
Uauy, R., Mena, P., Wegher, B., Nietao, S., and Salem, N. (2000). Long chain polyunsaturated fatty acid formation in neonates: effect of gestational age and intrauterine growth. Pediatr. Res. 47, 127–135.
Vlaardingerbroek, H., and Hornstra, G. (2004). Essential fatty acids in erythrocyte phospholipids during pregnancy and at delivery in mothers and their neonates: comparison with plasma phospholipids. Prostaglandins Leukot. Essent. Fatty Acids 71, 363–374.
Wang, H., and Eckel, R. H. (2009). Lipoprotein lipase: from gene to obesity. Am. J. Physiol. Endocrinol. Metab. 297, E271–E288.
Waterman, I. J., Emmison, N., and Dutta-Roy, A. K. (1998). Characterisation of triacylglycerol hydrolase activities in human placenta. Biochim. Biophys. Acta 1394, 169–176.
Waterman, I. J., Emmison, N., Sattar, N., and Dutta-Roy, A. K. (2000). Further characterization of a novel triacylglycerol hydrolase activity (pH 6.0 optimum) from microvillous membranes from human term placenta. Placenta 21, 813–823.
Widdowson, E. M. (1968). “Growth and composition of the fetus and newborn,” in The Biology of Gestation, ed. N. S. Assali (New York: Academic Press), 1–49.
Wijendran, V., Bendel, R. B., Couch, S. C., Philipson, E. H., Cheruku, S., and Lammi-Keefe, C. J. (2000). Fetal erythrocyte phospholipid polyunsaturated fatty acids are altered in pregnancy complicated with gestational diabetes mellitus. Lipids 35, 927–931.
Wijendran, V., Bendel, R. B., Couch, S. C., Philipson, E. H., Thomsen, K., Zhang, X., and Lammi-Keefe, C. J. (1999). Maternal plasma phospholipid polyunsaturated fatty acids in pregnancy with and without gestational diabetes mellitus: relations with maternal factors. Am. J. Clin. Nutr. 70, 53–61.
Keywords: placenta, fatty acids, DHA, lipolysis, lipid droplets
Citation: Gil-Sánchez A, Demmelmair H, Parrilla JJ, Koletzko B and Larqué E (2011) Mechanisms involved in the selective transfer of long chain polyunsaturated fatty acids to the fetus. Front. Gene. 2:57. doi: 10.3389/fgene.2011.00057
Received: 08 March 2011; Paper pending published: 25 May 2011;
Accepted: 16 August 2011; Published online: 06 September 2011.
Edited by:
Beverly Sara Muhlhausler, The University of Adelaide, AustraliaReviewed by:
James A. Armitage, Monash University, AustraliaSheridan Gentili, University of South Australia, Australia
Copyright: © 2011 Gil-Sánchez, Demmelmair, Parrilla, Koletzko and Larqué. This is an open-access article subject to a non-exclusive license between the authors and Frontiers Media SA, which permits use, distribution and reproduction in other forums, provided the original authors and source are credited and other Frontiers conditions are complied with.
*Correspondence: Elvira Larqué, Department of Physiology, Faculty of Biology, University of Murcia, Campus Espinardo 30100, Murcia, Spain. e-mail:ZWx2aXJhZGFAdW0uZXM=