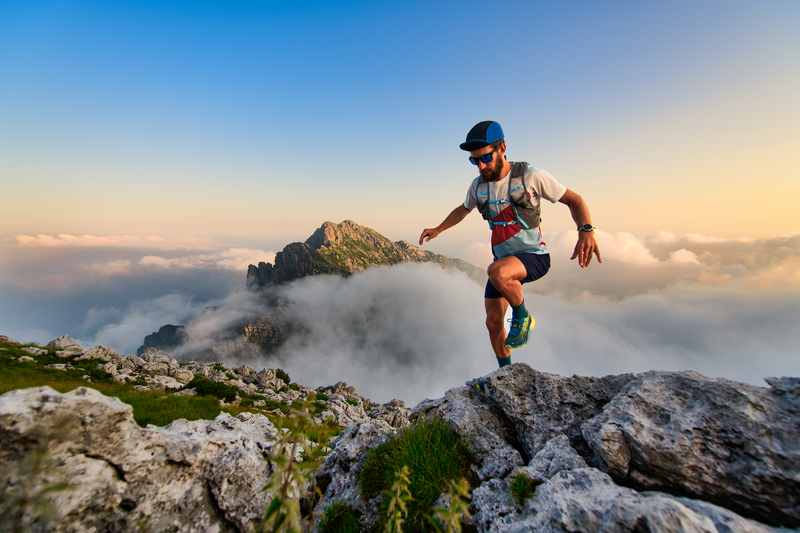
95% of researchers rate our articles as excellent or good
Learn more about the work of our research integrity team to safeguard the quality of each article we publish.
Find out more
REVIEW article
Front. Gastroenterol. , 14 January 2025
Sec. Hepatology
Volume 3 - 2024 | https://doi.org/10.3389/fgstr.2024.1534431
Non-alcoholic fatty liver disease (NAFLD) has a rapidly growing incidence worldwide, affecting approximately one-third of world population. The disturbance of gut commensal bacteria impacting host’s homeostasis is referred to as gut dysbiosis. The gut microbiome contributes to the pathogenesis of NAFLD through various pathways. Gut microbiota is at constant interactions with the intestinal epithelial barrier and affects its integrity. Through gut-liver axis, gut microbiota may influence liver immune function. The release of lipopolysaccharides (LPS) from intestines to portal vein which are transported to the liver, may trigger hepatic inflammation, steatosis and even fibrosis. Moreover, the gut microbiome induces the conversion of primary bile acids (BAs) to secondary BAs, which activates intestinal receptors, such as FXR and TGR5. FXR activation decreases fat absorption and thus reduces hepatic lipid accumulation, while TGR5 activation promotes the release of glucagon-like peptide-1 (GLP-1) in blood. Furthermore, gut ethanol-producing bacteria has been implicated in NAFLD development. Additionally, in NAFLD there is a reduction in intestinal levels of short-chain fatty acids, such as butyrate, propionate and acetate. Many bacterial alterations have been observed in NAFLD, including the increased Bacteroidetes and decreased Firmicutes. Many probiotics have been tried in NAFLD prevention and management, including a plethora of strains from Lactobacilli, Bifidobacteria and Streptococcus and some of them have promising perspectives. There is also some promising data from the administration of prebiotics (such as inulin and fructo-oligosaccharides) and symbiotics (probiotics plus prebiotics). Faecal microbiota transplantation (FMT) is yet to be evaluated for its efficacy against NAFLD.
Non-alcoholic fatty liver disease (NAFLD) is a liver disease defined by the accumulation of more than 5% of fat in the liver as triglycerides inside the hepatocytes (1). NAFLD includes a wide spectrum of diseases that range from simple liver steatosis without inflammation to more severe conditions such as non-alcoholic steatohepatitis (NASH), which is also known as metabolic dysfunction-associated steatohepatitis (MASH), characterised by inflammation, which causes varying degrees of liver fibrosis and even cirrhosis (2). This disease affects approximately one-third of the population worldwide, and its incidence is higher in men than women (3). The vast majority of the affected patients may not experience any symptoms until the disease progresses to more advanced stages, making NAFLD a potential silent killer shortly (4). Moreover, NAFLD is related to many metabolic disorders such as diabetes mellitus type 2, central obesity, hypertension, dyslipidemia and abnormal liver function tests (5). NAFLD is a common cause of chronic liver disease and cirrhosis, among other aetiologies such as alcohol consumption and viral hepatitis, while cirrhosis caused by NAFLD has considerably increased worldwide over the past decades (6). Moreover, there are many reports of hepatocellular carcinoma in NAFLD, and some of them occurred even without the presence of cirrhosis (7).
Gut microbiota is defined as the microbial community that resides inside the whole gastrointestinal tract, while the microbiome refers to the genetic information within the microbiota, including bacteria, fungi and viruses, all of which exist on and in the human body, which is the host (8). The human intestinal tract is colonised with approximately 3,8 x 1013 bacteria, whereas the human cells account for roughly 3 x 1013 (9). Furthermore, gut microbiome sequences contain 3,3 x 106 microbial genes, a huge number that is 150 times bigger than the human genes, which are 2,2 x 104 (10). Microbiome has a crucial role in the development of the host’s immune system (11). Parallelly, gut microbiome disturbance is associated with a plethora of diseases, such as cardiovascular (12), autoimmune and inflammatory diseases (13), and it is affiliated with various types of cancer (14). In addition, the gut microbiome’s diversity has an impact on neurological and psychiatric disorders, like Alzheimer’s disease and depression, respectively, via the gut-brain axis (15, 16). The type of childbirth and the complementary feeding transition during the first year of life are critical parameters in the acquisition and development of the infant microbiome (17, 18). Nevertheless, constantly throughout the whole lifespan of the host, the gut microbiome remains susceptible to alterations due to many factors such as lots of medications, dietary habits and preferences, health status, stress, various environmental agents and ageing (19).
Most cells of the immune system are located in the intestinal lumen, containing innate lymphoid cells, γδ T cells, type 1 interferon-producing plasmacytoid dendritic cells and mucosa-associated invariant T cells (20). The intestinal epithelial barrier, established at the luminal surface, consists of physical, microbial and immunologic constituents, compromising tight intracellular junctions between intestinal epithelial cells (21). The gut microbiome and intestinal epithelial barrier are in continuous dynamic interaction with the immune cells, epithelial cells and the gut microbiome being the protagonists in this beneficial coexistence (22). A secure and regulated intestinal barrier has an important role in preventing the translocation of bacteria outside of the intestinal lumen (23). Commensal bacteria reinforce intestinal barrier integrity via Toll-like receptors (TLR) signalling, inducing gut epithelial cell proliferation as well as stimulation of cell-mediated immunity (24). By secreting immunoglobulin IgA and antimicrobial peptides such as α-defensins, β-defensins, lysozyme, C-type lectins and cathelicidins, the immune system contributes to the integrity of the intestinal barrier (25). However, a potential disturbance in the commensal gut microbial communities can cause intestinal disease by the activation of the immune system and malfunction of the intestinal barrier and, thus, harmful intestinal permeability (26). This condition is known as gut dysbiosis, and it is related to many diseases, either intestinal or systemic, such as inflammatory bowel disease (IBD) (27), irritable bowel syndrome (IBS) (28), diabetes mellitus type 2 (29), etc. In addition, intestinal permeability, which refers to the disturbance of tight intercellular junctions, has been linked to NAFLD occurrence (30).
The liver and gut are in constant interaction through the gut-liver axis, which refers to the physical connection via the portal vein and blood inflow directed to the liver from the intestines (31). Growing investigational data supports the theory of intestinal barrier dysfunction blamed for triggering inflammation in the liver tissue, leading to NAFLD progression (32). Conversely, restoration of intestinal barrier function may have an alleviative impact on liver inflammation and thus a mitigation to NAFLD and fibrosis development (33). Disturbance of the intestinal barrier and, thus, intestinal permeability permits the entrance of gut microorganisms into the bloodstream, and via the portal vein, they direct at the liver, provoking hepatocellular injury through activation of immune cells (34). This phenomenon of bacteria and their membrane molecules, such as lipopolysaccharides (LPS), directed to the liver through portal vein is also known as translocation (35). Inside the liver, Kupffer cells (macrophage cells) with their toll-like receptor 4 (TLR4) can recognise LPS and other pathogen associated microbial patterns (PAMPs) that are components of the bacteria and activate further inflammatory cells (including neutrophils, monocytes and T lymphocytes) directed to liver by expressing cytokines (such as TNF-α, IL-1β, IL-6, IL-12, IL-18) and other signalling molecules (36). This procedure can lead to a more severe form of NAFLD, namely non-alcoholic steatohepatitis (NASH), which is also known as metabolic dysfunction-associated steatohepatitis (MASH), evoking inflammation with hepatocellular injury, which may cause different degrees of fibrosis by the activation of hepatic stellate cells, and this can lead even to liver cirrhosis (35, 37). Vice versa, it is observed that animal models lacking TLR4 receptors are not capable of binding LPS, and thus, they are protected from hepatic steatosis development (38). Moreover, damaged parenchymal and nonparenchymal cells from intestines and liver are able to release damage-associated molecular patterns (DAMPs), which as well as PAMPs can trigger hepatic innate immune cells through TLRs activation (39). Both DAMPs and PAMPs (such as LPS) can activate inflammasome sensors that can stimulate the intracellular increase of multiprotein complex as the effector protein caspase-1, which can lead to IL-1β and IL-18 release and stimulation of the pyroptotic protein gasdermin D (GSDMD), provoking hepatic cells death (40). In NAFLD patients, elevated serum levels of IL-1β and IL-18 have been observed as well as increased NLRP3 (NLR family pyrin domain containing 3) inflammasome activation, inducing hepatocytes death (41) (Figure 1).
The primary bile acids (BAs), chenodeoxycholic acid and cholic acid, are synthesised in the liver, and then through conjugation with either taurine or glycine, they form bile salts as a component of bile, which is stored in the gallbladder and through the biliary system it is secreted into the intestinal lumen (42). In the gut, bile salt hydrolases (BSHs) deconjugate BAs from bile, while BSHs have been found in gut microbial phyla, such as Bifidobacterium, Bacteroides, and microbial genera, including Lactobacillus, Clostridium spp. and Enterococcus (43). Afterwards, inside the intestine, they are metabolised into diverse secondary bile acids (deoxycholic acid from cholic acid and lithocholic acid from chenodeoxycholic acid) by gut microbiota, with the enzyme 7alpha-dehydroxylase, which is mostly synthesised by species from the Firmicutes phylum (43–45). The vast majority (95%) of all bile acids are actively reabsorbed in the last part of the small intestine (Ileum), and through the portal vein, they return to the liver, where they are reused many times daily (46).
Secondary BAs are crucial for the absorption of lipids and other nutrients from the intestinal tract (42). Moreover, secondary BAs have a ligand role in numerous receptors, such as the nuclear farnesoid X receptor (FXR), regulating bile synthesis and metabolism (47). In addition, BAs may activate or modulate bile acid nuclear receptors, such as FXR, PXR and TGR5, a vitamin D receptor (NR1I1), and transporters, such as the ileal apical sodium-dependent bile acid transporter (ASBT), some of which seem to contribute to NAFLD and NASH pathogenesis and progression, as well as they have an impact on insulin resistance (48). In experimental models, it has been found that activation of FXR by agonist agents reduces lipid absorption and decreases the accumulation of monosaturated and polyunsaturated fatty acids in the liver (49). In a clinical trial with NASH patients, Rinella ME et al. (2022) observed improvements in non-invasive liver tests in the obeticholic acid (FXR agonist agent) group to the placebo group (50). Activation of TGR5 receptor promotes the increase of glucagon-like peptide-1 (GLP-1) levels in blood (51). Administration of GLP-1 receptor agonists to diabetic patients has been linked with decreased fatty liver, hyperlipidemia and hypertension (52). Furthermore, in experimental models, TGR5 receptor activation downregulates (NF-kappaB)-mediated inflammation and thus, it has an anti-inflammatory effect on the liver in rodents (53) (Figure 2). Experimental inhibition of ASBT transporter has shown a reduction in body weight, intestinal fat absorption and hepatic steatosis in mice, but further research is needed (54).
When commensal diverse gut microbiota is disrupted, there is a decrease in converting primary to secondary BAs and thus reduced activation of bile acid receptors. Also, decreased secondary BAs evoke further disturbance to bacterial symbiosis (55). Via FXR signalling, BAs can protect commensal gut microbiota from gut bacteria overgrowth and reinforce intestinal epithelial barrier (56). In NAFLD, there is a reduction in gut bacteria that convert primary into secondary BAs (57). It has been found that the increased ratio of conjugated chenodeoxycholic acid (CDCA)/muricholic acid (MCA) in serum was related to worse NASH progression in 134 individuals having NAFLD (58). Furthermore, in FXR lacking rodents, a reduction in deconjugation of bile salts decreases the release of taurine, which has a beneficial effect on hepatic inflammation and steatosis (59). Ursodeoxycholic acid (UDCA) belongs to secondary BAs, which can be administrated orally as a medication, and with its primary colonic metabolite, lithocholic acid, they can have anti-inflammatory effects on the colon (60). In an experiment with mice, co-administration of UDCA with a statin (rosuvastatin) and ezetimibe decreased the accumulation of collagen in rodents’ liver and ALT (alanine aminotransferase) levels in serum and improved fibrosis-related markers, seeming to be a promising therapy in NAFLD deterioration, but further investigation is needed (61). Furthermore, external factors, such as dietary habits and medications, may influence the bile acid pool and its actions indirectly through their effect on the gut microbiome (62).
The characteristics of liver steatosis and inflammation are very similar in alcoholic fatty liver disease and in non-alcoholic fatty liver disease (NAFLD) (63). The gut microbial community can produce ethanol, which is absorbed from the intestine and through the portal vein, which proceeds to the liver (64, 65). Baker SS, et al. (2010) found an increased ADH (alcohol dehydrogenase) gene transcription in the NASH group compared to the control group, suggesting increased blood alcohol levels in NASH patients and increased activity of metabolising the circulating alcohol in NASH livers (65). Ethanol-producing gut microbiota is one of the multiple factors and mechanisms of the progression of NAFLD and deterioration to NASH (66). In NASH subjects, an increased proportion of alcohol-producing bacteria has been found in their gut, as well as increased ethanol blood levels (67).
From the gut bacteria, a high-alcohol-producing species of Klebsiella pneumoniae was associated with NAFLD in humans, and when this species was transferred into rodents’ intestines, it provoked NAFLD as well (68). Furthermore, Mbaye B, et al. (2023) observed increased ethanol and glucose in the faeces of NASH individuals, which was related to dysbiosis and alteration of gut microbiome with augmentation of ethanol-producing bacteria, such as Enterocloster bolteae, Limosilactobacillus fermentum, Streptococcus mutans and Mediterraneibacter gnavus (69). In the liver, alcohol can provoke mitochondrial dysfunction with pathological fatty acid oxidation and impaired oxidative phosphorylation, causing oxidative stress in hepatocytes, which can lead to steatohepatitis (70). Meijnikman AS, et al. (2022) observed median ethanol concentrations in the portal vein were 187 times higher compared to fasting peripheral blood. Also, ethanol levels were increased proportionally from individuals without liver steatosis to NAFLD and even increased in NASH (71). In the same study, applying inhibition of ADH (a liver enzyme which metabolises ethanol) in NAFLD individuals increased 15 times ethanol concentrations in peripheral blood, but this phenomenon was ameliorated after the administration of antibiotics (71). Ethanol-producing bacteria, among many others, play a role in the pathogenesis of NAFLD, and the gut microbiome might be one of the potential therapeutic targets of this disease (72).
Short chain fatty acids (SCFAs) are produced inside the intestinal lumen by the gut commensal bacteria during the fermentation of dietary fibres, with the main SCFAs being butyrate, propionate and acetate (73). Inadequate fibre consumption may compromise the production of SCFAs, impairing the host’s immune system function, which may be associated with variant diseases (74). SCFAs improve the function of epithelial intestinal cells by regulating their proliferation and differentiation, providing energy to gut epithelial cells, enhancing the host’s metabolism and reinforcing the epithelial gut barrier (75). A high fat/carbohydrate diet may promote dysbiosis in gut microbiota by the predominance of Prevotella, Firmicutes (Clostridium) and Methanobrevibacter, diminishing the beneficial bacteria (Bifidobacterium, Bacteroides, Akkermansia and Lactobacillus), which is related to reduced SCFAs production, increased inflammation, dyslipidemia and obesity (76). Butyrate has the most anti-inflammatory properties by activating T-regulating immune cells, which act with the inhibition of T cells and Th17, intervening in the inflammatory cascade (77).
Alteration of gut microbiota may impair the SCFAs production, which may cause obesity-related diseases, including NAFLD (78). Decreased levels of butyrate are linked to intestinal barrier dysfunction, intestinal permeability, and translocation of bacterial endotoxins (LPS), causing liver steatosis (79). Zhou D, et al. (2017) administrate sodium butyrate to high-fat diet rodents, which resulted in the restoration of gut microbiota dysbiosis, improvement of gut barrier function and amelioration of inflammation and fat accumulation in the liver (80). Furthermore, SCFAs, through the activation of free fatty acid receptors in the intestine, release hormones, such as glucagon-like peptide-1 (GLP-1) and peptide YY, that regulate the host’s glucose levels, appetite and energy metabolism (81). In high-fat diet animal models, sodium butyrate can upregulate intestinal L cells to release GLP-1, which activates the GLP-1 receptor with a beneficial effect on the progression of NAFLD and NASH, while butyrate can also upregulate the expression of GLP-1 receptors in the liver (82). Moreover, sodium butyrate seems to attenuate the deterioration of NAFLD by reducing the inflammation in the liver and protecting melatonin production and receptor expression in the liver and small intestine (83).
Endocannabinoid system seems to play a noteworthy role in the regulation of lipid, glucose and energy metabolism, as well as in immune function and inflammation (84). Obesogenic high‐fat diets may affect microbiota-gut-brain axis by increasing endocannabinoid levels in peripheral tissues and brain (85). Furthermore, the endocannabinoid system, among other metabolic systems, regulates the intestinal barrier function (86). It has been found that pharmacological activation of endocannabinoid system may lessen intestinal barrier integrity and provoke adipogenesis (87). Gut microbiota is able to produce variant metabolites, including endocannabinoids, regulating the development of adipose tissue and its metabolic function (88). Agonists of cannabinoid receptors (type 1 and 2), like bioactive lipids from N-acylethanolamine family, may promote metabolic disorders and hepatic steatosis (89). Disrupted endocannabinoid system regulation and tissue metabolism have been found in obese and diabetic mice with altered gut microbiota (90). Moreover, changes in intestinal and plasma endocannabinoid levels may cause modifications in hypothalamic Pomc neurons’ function, inducing hyperphagic behaviour and exacerbating obesity and hepatic steatosis in rodents (91).
Choline is an essential nutrient for normal liver function by packaging and exporting triglycerides in very low-density lipoprotein (VLDL) from hepatic tissue, while low-choline diet is associated with NAFLD (92). In high-fat diet pig model, gut microbiota may catabolise choline to trimethylamine that is converted to trimethylamine-N-oxide which can accumulate into liver and, along with choline depletion, may induce NASH (93). The metabolism of choline by gut microbiota regulates the bioavailability of choline from the diet (94). Moreover, higher dietary choline is related with lower risk of developing NAFLD compared to inadequate choline consumption in both males and females (95). Furthermore, methionine-choline-deficient-diet has been used in many animal studies to provoke NAFLD models (54, 96, 97).
It has been observed in a plethora of studies that there is a tight relationship between intestinal dysbiosis and NAFLD (98). Wigg AJ, et al. (2001) found increased small intestinal bacterial overgrowth (SIBO) prevalence in NASH to control individuals (assessed by breath test with ingestion (14)C-D-xylose-lactulose), as well as higher tumour necrosis factor alpha levels in NASH group, proposing the contribution of SIBO in NASH pathogenesis (99). Zhang X, et al. (2020) observed in mice that a high-cholesterol diet can provoke gut microbiota dysbiosis by increasing some species (Anaerotruncus, Desulfovibrionaceae, Desulfovibrio and Mucispirillum) while decreasing others (Bacteroides and Bifidobacterium), inducing the development and deterioration of NAFLD (100). In stool samples, Mouzaki M, et al. (2013) found a decreased percentage of Prevotella species (Bacteroides) in the NASH group in comparison with the simple steatosis group and healthy liver group, regardless of BMI and dietary fat intake (101). The prevalence level of SIBO is higher in NASH in healthy individuals, inducing inflammation and fibrosis in the liver through the increased levels of endotoxins, which activate the immune system with upregulation of toll-like receptor 4 (TLR-4) expression and releasing the pro-inflammatory cytokine, interleukin 8 (IL-8) (102).
In NAFLD, there is a reduced diversity in gut microbiota, and as for the phyla, compared to healthy controls, the NAFLD individuals have increased Bacteroidetes and decreased Firmicutes in their faecal samples (103). Furthermore, increased gut Proteobacteria has been observed in obese non-diabetic women with hepatic steatosis (104). In addition to Proteobacteria, Fusobacteria phyla are also increased in NALFD and regarding gut bacterial families in NAFLD, there is an augmentation in Enterobacteriaceae and Lachnospiraceae, while the Ruminococcaceae and Prevotellaceae families are depleted compared to healthy individuals (105). Moreover, decreased Oscillospira and Rikenellaceae families have been found in NALFD, while increased Dorea genus in the gut microbiome relates to the deterioration of NAFLD to NASH (57). Additionally, increased Escherichia genus and Peptoniphilus genus have been observed in NAFLD patients (57, 67). On the contrary, a reduction is present in the genera of Faecalibacterium (67), Coprococcus (103), Anaerosporobacter (103), Eubacterium (102) and Prevotella (106) in NAFLD gut microbiota samples (Table 1). The former gut microbiota imbalance and gut dysbiosis have promoted the introduction of microbiome-target therapies, including prebiotics, probiotics, synbiotics (probiotics plus prebiotics) and faecal microbiota transplantation (FMT) (107).
The definition of probiotics is live microorganisms that, when administered in adequate amounts, confer a health benefit on the host (108). Administration of probiotics may be a potent therapy in NAFLD with a decrease in liver fat accumulation and restoration of liver aminotransferases blood levels (109). Probiotics can modulate gut microbiota, enhance intestinal barrier, improve hepatic and serum lipid profiles, reduce liver steatosis and have anti-inflammatory effects (110). There are variant probiotic bacteria that have been administered experimentally in rodents with NAFLD with beneficial results, including Lactobacilli, Bifidobacteria and Streptococcus (111). Naudin CR, et al. (2020) noticed that supplementation of Lactococcus lactis Subspecies cremoris to western-style (high-fat and/or high-carbohydrate) diet female mice mitigates hepatic inflammation and ameliorates liver steatosis (112). Okubo H, et al. (2013) observed that the administration of Lactobacillus casei strain Shirota protects from the development of NASH in methionine-choline-deficient-diet mice through augmentation of gut lactic acid bacteria (Bifidobacterium and Lactobacillus) (96). In a different animal model, Lactobacillus casei strain Shirota prevented the development of NAFLD in fructose-induced steatosis mice by downregulation of Toll-like receptor 4 (TLR4) activity and upregulation of peroxisome proliferator-activated receptor γ (PPAR-γ) activation (113). Additionally, administration of Bifidobacterium Pseudocatenulatum CECT 7765, beyond metabolic amelioration, improves immune function in obese high-fat-diet mice, decreasing interleukin six levels and enhancing dendritic and macrophage cells signalling and functioning (114).
Zhao et al. (2020) reported the administration of Lactobacillus plantarum NA136 led to improvement of NALFD in high-fat and fructose diet mice at various levels, including correction of gut microbiota disturbances, reinforcement of intestinal barrier and decrease of liver inflammation (115). Furthermore, Lactobacillus plantarum strains reduced fat accumulation in histopathological examination and improved blood biochemical liver markers in NAFLD rodents, according to Park EJ, et al. (2020) (116). The probiotic Akkermansia muciniphila mitigates immune-induced liver histopathological injury triggered by Concanavalin A in a mouse model (117). Administration of Lactobacillus rhamnosus GG in high-fructose diet mice with NAFLD enhances gut barrier integrity, decreases lipopolysaccharide levels in the portal vein and lessens the release of pro-inflammatory cytokines, such as TNF-α, IL-8R and IL-1β, from the liver, as well as reduce fat accumulation in liver and ALT-aminotransferase blood levels (118). In addition, Lactobacillus rhamnosus GG in high-fat diet obese mice decreases serum cholesterol and triglyceride levels, reduces hepatic fat accumulation, lessens pro-inflammatory and lipogenic gene activation in the liver and downregulates FGF15 and FXR signalling compared to solely high-fat diet obese mice (119). Moreover, on NAFLD mice induced by a high-fat/high-fructose diet plus intermittent hypoxia exposure, Lactobacillus rhamnosus GG has a protective effect on insulin resistance, glucose intolerance, hepatic injury and steatosis, and upregulates liver PPARα signalling and increases butyrate faecal levels (120). Lactobacillus paracasei ameliorates NASH in mice by inducing the dominance of M2 Kupffer cells and downregulation of M1 Kupffer cells in the liver (121). Lactobacillus johnsonii BS15 can prevent the development of NAFLD in mice by protecting hepatocytes from oxidative stress and attenuating mitochondrial dysfunction (122). In western-type-diet FXR receptor knockout mice, which develop NASH, administration of probiotic VSL#3 has a protective effect through activation of alternative bile acid pathway (activation of GPBAR1 receptor), modulation of gut microbiota resulting in increased production of butyrate and thus anti-inflammatory and metabolic benefits (123).
In a double-blind, randomised clinical trial (RCT) with obese children suffering from NAFLD, Alisi, et al. (2014) showed that daily administration of VSL#3 probiotics (a cluster of 8 distinct lactic acid-producing bacteria) results in amelioration of fatty liver in ultrasonographic examination at 4 months, via upregulation of glucagon-like peptide 1 (GLP-1) signalling (124). Famouri F, et al. (2017) noticed sonographic liver improvement as well as a decrease in aminotransferases blood levels induced by the concurrent administration of 4 probiotic bacteria to obese children having biochemical and sonographic NAFLD (125). Daily consumption of 500 million Lactobacillus bulgaricus and Streptococcus thermophilus alleviated liver aminotransferases blood levels in patients with NAFLD in a double-blind (2011) RCT (126). Vajro P, et al. (2011) noticed alanine aminotransferase (ALT) reduction after treatment with Lactobacillus rhamnosus strain GG in children suffering from obesity-related NAFLD (127). Probiotics can inhibit harmful bacterial proliferation and enhance gut barrier integrity, resulting in a reduction of lipopolysaccharide (LPS) and, thus, downregulation of toll-like receptor four signalling in the liver (128).
A 2023 meta-analysis with 41 RCTs showed that the administration of probiotics, prebiotics or synbiotics can ameliorate sonographic liver steatosis, improve fibrosis and reduce blood levels of aminotransferases (AST and ALT) and gamma-glutamyl transpeptidase (GGT) (129). In a 2019 meta-analysis with 28 clinical trials, including 1555 individuals with NAFLD, probiotics reduce serum levels of aminotransferases (AST and ALT), GGT, total cholesterol and insulin, improve insulin resistance and decrease BMI, while there is no significant impact on lipid profile and TNF-a levels (130). Moreover, a 2021 meta-analysis with 352 patients suffering from NAFLD showed that probiotics can significantly decrease serum levels of aminotransferases (AST and ALT) and total cholesterol, but there is no effect on BMI, insulin resistance and levels of TNF, although there is a reduction in BMI when probiotic treatment surpasses 3 months (131). Either probiotic mixtures or single-strain probiotics can prevent the development of diet-induced NAFLD by restoring gut microbial composition, enhancing gut barrier integrity, inducing fatty acid oxidation and downregulation of lipogenesis in the liver (132). In the elderly, which can potentially have gut microbial alterations, probiotics may restore gut dysbiosis, decrease oxidative stress and thus prevent or mitigate the progression of NAFLD (133).
A Prebiotic is defined as a substrate that is selectively utilised by the host’s microorganisms, conferring a health benefit, according to the International Scientific Association for Probiotics and Prebiotics (ISAPP) statement in 2016 (134). Prebiotics are food components which are not digested or absorbed from the intestine, but they are fermented by gut microbiomes, altering the composition of gut microbiota in a favourable way for the host (135). For instance, the consumption of prebiotics, such as galacto-oligosaccharides (GOS), can promote the augmentation of Bifidobacteria and Lactobacilli inside the intestine (136).
Administration of fructo-oligosaccharides (FOS) to obesity-induced (from injection with monosodium glutamate) mice with NAFLD promotes augmentation of SCFAs production from gut microbiota, and thus reduction of hepatic inflammation and amelioration of steatohepatitis (137). Furthermore, in a high-fat/high-sugar diet mouse model, FOS ameliorates hepatic lipid accumulation, decreases serum levels of total cholesterol, transaminases (ALT and AST) and inflammatory cytokines (IL-6 and TNF-a) and improves lipid profile (138). Consumption of choline and FOS by rodents with NAFLD induces fat degradation in the liver, and parallelly, choline treatment increases the levels of vitamin E and glutathione in hepatic and cardiac tissue (139). In mice which were fed with a methionine-choline-deficient diet, the addition of FOS to their diet prevents the Lactobacillales spp. Reduction and Clostridium cluster XI augmentation in gut microbiota, increases faecal SCFAs and IgA concentrations, downregulates toll-like receptors 4 (TLR4) function in the liver and mitigates hepatic inflammation and steatosis (97). Furthermore, in a mouse model underlain n-3 PUFA-depleted diet, FOS supplementation induces augmentation of Bifidobacterium spp. and reduction in Roseburia spp. in the gut, and it mitigates fat accumulation in the liver through PPAR-α genes upregulation (140).
Inulin, as a fructan-type prebiotic, can increase, through fermentation, the production of SCFAs by gut microbiota, increase omega-3 and odd-chain fatty acids levels and downregulate the expression of genes promoting lipogenesis (Fasn, Gpam) in the liver, as observed in rodents (141). In a 2020 randomised, double-blind clinical trial with patients suffering from NAFLD, who underlain weight loss through a very-low-calorie diet, the administration of inulin plus short therapy with metronidazole to these patients decreases alanine aminotransferase (ALT) levels (142). Administration of inulin-type fructans, including oligofructose, to patients with NASH reduces aminotransferases and insulin blood levels (143). Supplementation with inulin to western-type diet obese mice enhances intestinal barrier integrity, decreases endotoxemia and ameliorates hepatic steatosis (79). Moreover, inulin restores gut microbiota disturbances, increases SCFAs (particularly butyrate and propionate) synthesis inside the intestine, increases activation of PPAR-α receptor and mitigates liver inflammation and steatosis in high-sucrose diet rodents with NAFLD (144). In addition, inulin may alleviate NAFLD through augmentation of bile acids synthesis in the liver, increased bile acids excretion to the intestine and upregulation of FXR signalling, as observed in mice (145). Furthermore, inulin administration to high-fat rodents prevents hepatic triglyceride accumulation by modifying gut microbiota by increasing 5-fold the species Akkermansia muciniphila (146). However, in a 2024 randomised clinical trial with patients suffering from NAFLD maintaining a stable body weight during the trial, who supplemented with 16g per day of inulin-type fructans, even though the prebiotics increased faecal Bifidobacterium bacterial concentration, they did not impact neither hepatic fat accumulation nor inflammatory and hepatic markers (147).
Synbiotics are referred to as a mixture comprising live microorganisms (such as probiotics) and substrates (including prebiotics) selectively utilised by host microorganisms that confer a health benefit on the host (148). Oral administration of synbiotics (FOS plus probiotic strains) to patients with NAFLD for 28 weeks in combination with lifestyle modifications is more effective than lifestyle modifications alone in ameliorating NAFLD, especially through further reduction in hepatic and inflammatory markers (149). Supplementation with Bifidobacterium longum plus FOS with lifestyle changes (exercise and diet) to NASH is superior to solely lifestyle changes by an additional decrease in aminotransferases (particularly AST) levels, insulin resistance, inflammatory markers, serum endotoxemia and liver steatosis (150). In rodents with NAFLD induced by a high-fructose diet, the addition of FOS plus Lactobacillus fermentum CECT5716 to their diet improves gut dysbiosis, enhances gut barrier integrity, and thus prevents the development of liver steatosis (151). Furthermore, it seems that the administration of synbiotics to obese children may decrease their body mass index (BMI) and improve their lipid profile (152, 153). In addition, Alves CC, et al. (2017) showed that supplementation synbiotics to rodents increases PPAR-α activity, which upregulates β-oxidation of lipids and decreases lipogenesis by downregulation of SREBP-1c and FAS genes activation and thus ameliorates steatosis (154).
Musazadeh V, et al. (2024) observed in a meta-analysis participating 1,188 patients with NAFLD who were supplemented with synbiotics within 8 to 56 weeks that synbiotics decrease hepatic (AST, ALT and GGT) and inflammatory (CRP and TNF-a) markers as well as improve lipid profile and obesity indicators (155). Liu L, et al. (2019), in a meta-analysis including 782 patients suffering from NAFLD, showed that the administration of probiotics and synbiotics reduces aminotransferases (AST and ALT) and TNF-α levels, ameliorates liver steatosis, decreases liver stiffness and improves lipid profile, although there was not a significant impact on BMI and fasting blood sugar (156). Although microbiome-target therapies (including probiotics, prebiotics and synbiotics) are linked to the amelioration of NAFLD, there is a wide diversity in probiotic strains, dosages and formulations in the literature (129). Supplementation with Lactobacillus paracasei N1115 plus FOS to mice with NAFLD induced improvement in liver steatosis, reduction in TNF-a serum levels and retardation in cirrhosis development (157). However, the administration of synbiotics for 1 year to 24 patients with NAFLD plus significant liver fibrosis (≥F2) did not improve either adipose tissue dysfunction or inflammatory markers, as observed in the 2024 clinical trial (158). In a 2020 clinical trial with 104 patients suffering from NAFLD who supplemented for 1 year with prebiotics (FOS plus Bifidobacterium animalis subspecies lactis BB-12), it was shown that there was an alteration in the faecal microbiome, but there was observed a reduction neither in hepatic fat accumulation nor in hepatic fibrosis markers (159). Moreover, in a 2024 meta-analysis including 12,682 individuals with NAFLD who were supplemented with either probiotics, prebiotics or synbiotics, it was shown an overall reduction in aminotransferases (AST and ALT) levels, amelioration of hepatocytes injury indicators, decrease in inflammatory markers (such as TNF-a) and improvement to lipid profile (160).
Faecal microbiota transplantation (FMT) is described as the transfer of faecal material containing a minimally manipulated community of microorganisms to a human recipient from a healthy human donor (including autologous transfer), intending a beneficial effect on the recipient’s health through restoration of the gut microbiome (161). For instance, FMT is an effective treatment for recurrent infection from Clostridioides difficile (formerly known as Clostridium difficile) in immunocompetent individuals (162). There is a rising number of liver diseases (such as hepatic encephalopathy, alcoholic hepatitis and primary sclerosing cholangitis) that FMT may be a potential treatment (34, 163).
Zhou D, et al. (2017) observed that FMT to high-fat diet rodents with NASH induced the mitigation of steatohepatitis through augmentation of beneficial bacteria (such as Christensenellaceae and Lactobacillus) in the intestinal lumen, increased butyrate faecal levels and enhancement of gut barrier integrity in a mouse model (164). Craven et al. (2020), in a randomised clinical trial (RCT) including 21 participants suffering from NAFLD, noticed that FMT mitigated intestinal permeability after 6 weeks, although it did not impact either hepatic fat accumulation or insulin resistance (165). In another RCT, Witjes JJ, et al. (2020) observed that allogenic FMT from lean vegan donors to obese recipients with NAFLD ameliorates necro-inflammatory histological score and there were some significant changes in the expression of some hepatic genes associated with inflammation and lipid metabolism in comparison with the autologous FMT group with obese NAFLD participants (166). Furthermore, Xue L, et al. (2022), in an RCT with 75 individuals, found that the FMT group had a significant decrease in liver fat accumulation through alterations in gut microbiota, and FMT was more effective in gut microbiota modulation in lean than in obese individuals with NAFLD (167).
Gut microbiota dysbiosis seems to contribute to NAFLD pathogenesis through multiple pathways (Table 2). Emerging data suggests that gut microbiome induces the development of NAFLD mainly through liver inflammation. Since the incidence of NAFLD is increasing worldwide, there is a need for new preventative and therapeutic strategies. Microbiota-target therapies may have a major or supplementary role in NAFLD management in the future. There are some promising data about the administration of probiotics, prebiotics and synbiotics. The future will tell whether the FMT will be indicated for NAFLD prevention or treatment, as it is indicated for recurrent Clostridium difficile infection.
AM: Conceptualization, Data curation, Investigation, Resources, Writing – original draft, Writing – review & editing. CT: Data curation, Formal analysis, Methodology, Project administration, Resources, Supervision, Validation, Visualization, Writing – review & editing. IB: Data curation, Formal analysis, Methodology, Project administration, Resources, Supervision, Validation, Visualization, Writing – review & editing. EB: Data curation, Formal analysis, Methodology, Project administration, Resources, Supervision, Validation, Visualization, Writing – review & editing. ES: Data curation, Formal analysis, Methodology, Project administration, Resources, Supervision, Validation, Visualization, Writing – review & editing.
The author(s) declare financial support was received for the research, authorship, and/or publication of this article. This work was funded by the Master in Food, Nutrition and Microbiome of the Medical School Democritus University of Thrace.
This work was supported by the Master in “Food, Nutrition and Microbiome” of the Medical School Democritus University of Thrace.
The authors declare that the research was conducted in the absence of any commercial or financial relationships that could be construed as a potential conflict of interest.
The author(s) declared that they were an editorial board member of Frontiers, at the time of submission. This had no impact on the peer review process and the final decision.
The author(s) declare that no Generative AI was used in the creation of this manuscript.
All claims expressed in this article are solely those of the authors and do not necessarily represent those of their affiliated organizations, or those of the publisher, the editors and the reviewers. Any product that may be evaluated in this article, or claim that may be made by its manufacturer, is not guaranteed or endorsed by the publisher.
ADH, alcohol dehydrogenase; ALT, alanine aminotransferase; ASBT, apical sodium-dependent bile acid transporter; AST, aspartate aminotransferase; Bas, bile acids; BMI, body mass index; BSHs, bile salt hydrolases; CRP, c-reactive protein; FMT, faecal microbiota transplantation; FOS, fructo-oligosaccharides; FXR, farnesoid X receptor; GGT, gamma-glutamyl transpeptidase; GLP-1, glucagon-like peptide-1; GOS, galacto-oligosaccharides; IBD, inflammatory bowel disease; IBS, irritable bowel syndrome; IL, interleukin; LPS, lipopolysaccharides; MASH, metabolic dysfunction-associated steatohepatitis; NAFLD, non-alcoholic fatty liver disease; NASH, non-alcoholic steatohepatitis; PAMPs, pathogen associated microbial patterns; PPAR, peroxisome proliferator-activated receptor; RCT, randomised clinical trial; SCFAs, short chain fatty acids; SIBO, small intestinal bacterial overgrowth; TGR5, Takeda G protein-coupled receptor 5; TLR, toll-like receptor; TNF-a, tumor necrosis factor alpha; UDCA, ursodeoxycholic acid.
1. Brunt EM, Wong VW, Nobili V, Day CP, Sookoian S, Maher JJ, et al. Nonalcoholic fatty liver disease. Nat Rev Dis Primers. (2015) 1:15080. doi: 10.1038/nrdp.2015.80
2. Ganz M, Szabo G. Immune and inflammatory pathways in NASH. Hepatol Int. (2013) 7 Suppl 2:771–81. doi: 10.1007/s12072-013-9468-6
3. Riazi K, Azhari H, Charette JH, Underwood FE, King JA, Afshar EE, et al. The prevalence and incidence of NAFLD worldwide: a systematic review and meta-analysis. Lancet Gastroenterol Hepatol. (2022) 7:851–61. doi: 10.1016/S2468-1253(22)00165-0
4. Sivell C. Nonalcoholic fatty liver disease: A silent epidemic. Gastroenterol Nurs. (2019) 42:428–34. doi: 10.1097/SGA.0000000000000443
5. Pouwels S, Sakran N, Graham Y, Leal A, Pintar T, Yang W, et al. Non-alcoholic fatty liver disease (NAFLD): a review of pathophysiology, clinical management and effects of weight loss. BMC Endocr Disord. (2022) 22:63. doi: 10.1186/s12902-022-00980-1
6. Huang DQ, Terrault NA, Tacke F, Gluud LL, Arrese M, Bugianesi E, et al. Global epidemiology of cirrhosis - aetiology, trends and predictions. Nat Rev Gastroenterol Hepatol. (2023) 20:388–98. doi: 10.1038/s41575-023-00759-2
7. Shah PA, Patil R, Harrison SA. NAFLD-related hepatocellular carcinoma: The growing challenge. Hepatology. (2023) 77:323–38. doi: 10.1002/hep.32542
8. Shanahan F, Ghosh TS, O’Toole PW. The healthy microbiome-what is the definition of a healthy gut microbiome. Gastroenterology. (2021) 160:483–94doi: 10.1053/j.gastro.2020.09.057
9. Sender R, Fuchs S, Milo R. Revised estimates for the number of human and bacteria cells in the body. LoS Biol. (2016) 14:e1002533. doi: 10.1371/journal.pbio.1002533
10. Qin J, Li R, Raes J, Arumugam M, Burgdorf KS, Manichanh C, et al. A human gut microbial gene catalogue established by metagenomic sequencing. Nature. (2010) 464:59–65. doi: 10.1038/nature08821
11. Kuziel GA, Rakoff-Nahoum S. The gut microbiome. Curr Biol. (2022) 32:R257–64. doi: 10.1016/j.cub.2022.02.023
12. Zhao Y, Wang Z. Gut microbiome and cardiovascular disease. Curr Opin Cardiol. (2020) 35:207–18. doi: 10.1097/HCO.0000000000000720
13. Clemente JC, Manasson J, Scher JU. The role of the gut microbiome in systemic inflammatory disease. BMJ. (2018) 360:j5145. doi: 10.1136/bmj.j5145
14. Tong Y, Gao H, Qi Q, Liu X, Li J, Gao J, et al. High fat diet, gut microbiome and gastrointestinal cancer. Theranostics. (2021) 11:5889–910. doi: 10.7150/thno.56157
15. Vogt NM, Kerby RL, Dill-McFarland KA, Harding SJ, Merluzzi AP, Johnson SC, et al. Gut microbiome alterations in Alzheimer’s disease. Sci Rep. (2017) 7:13537. doi: 10.1038/s41598-017-13601-y
16. Winter G, Hart RA, Charlesworth RPG, Sharpley CF. Gut microbiome and depression: what we know and what we need to know. Rev Neurosci. (2018) 29:629–43. doi: 10.1515/revneuro-2017-0072
17. Ferretti P, Pasolli E, Tett A, Asnicar F, Gorfer V, Fedi S, et al. Mother-to-infant microbial transmission from different body sites shapes the developing infant gut microbiome. Cell Host Microbe. (2018) 24:133–145.e5. doi: 10.1016/j.chom.2018.06.005
18. McKeen S, Roy NC, Mullaney JA, Eriksen H, Lovell A, Kussman M, et al. Adaptation of the infant gut microbiome during the complementary feeding transition. PloS One. (2022) 17:e0270213. doi: 10.1371/journal.pone.0270213
19. Cresci GA, Bawden E. Gut microbiome: what we do and don’t know. Nutr Clin Pract. (2015) 30:734–46. doi: 10.1177/0884533615609899
20. Mowat AM, Agace WW. Regional specialization within the intestinal immune system. Nat Rev Immunol. (2014) 14:667–85. doi: 10.1038/nri3738
21. Chopyk DM, Grakoui A. Contribution of the intestinal microbiome and gut barrier to hepatic disorders. Gastroenterology. (2020) 159:849–63. doi: 10.1053/j.gastro.2020.04.077
22. Takiishi T, Fenero CIM, Câmara NOS. Intestinal barrier and gut microbiota: Shaping our immune responses throughout life. Tissue Barriers. (2017) 5:e1373208. doi: 10.1080/21688370.2017.1373208
23. Wells JM, Brummer RJ, Derrien M, MacDonald TT, Troost F, Cani PD, et al. Homeostasis of the gut barrier and potential biomarkers. Am J Physiol Gastrointest Liver Physiol. (2017) 312:G171–93. doi: 10.1152/ajpgi.00048.2015
24. Abreu MT. Toll-like receptor signalling in the intestinal epithelium: how bacterial recognition shapes intestinal function. Nat Rev Immunol. (2010) 10:131–44. doi: 10.1038/nri2707
25. Mukherjee S, Hooper LV. Antimicrobial defense of the intestine. Immunity. (2015) 42:28–39. doi: 10.1016/j.immuni.2014.12.028
26. Shi N, Li N, Duan X, Niu H. Interaction between the gut microbiome and mucosal immune system. Mil Med Res. (2017) 4:14. doi: 10.1186/s40779-017-0122-9
27. Frank DN, St Amand AL, Feldman RA, Boedeker EC, Harpaz N, Pace NR. Molecular-phylogenetic characterization of microbial community imbalances in human inflammatory bowel diseases. Proc Natl Acad Sci U S A. (2007) 104:13780–5. doi: 10.1073/pnas.0706625104
28. Altomare A, Di Rosa C, Imperia E, Emerenziani S, Cicala M, Guarino MPL. Diarrhea predominant-irritable bowel syndrome (IBS-D): effects of different nutritional patterns on intestinal dysbiosis and symptoms. Nutrients. (2021) 13:1506. doi: 10.3390/nu13051506
29. Qin J, Li Y, Cai Z, Li S, Zhu J, Zhang F, et al. A metagenome-wide association study of gut microbiota in type 2 diabetes. Nature. (2012) 490:55–60. doi: 10.1038/nature11450
30. Miele L, Valenza V, La Torre G, Montalto M, Cammarota G, Ricci R, et al. Increased intestinal permeability and tight junction alterations in nonalcoholic fatty liver disease. Hepatology. (2009) 49:1877–87. doi: 10.1002/hep.22848
31. Kolodziejczyk AA, Zheng D, Shibolet O, Elinav E. The role of the microbiome in NAFLD and NASH. EMBO Mol Med. (2019) 11:e9302. doi: 10.15252/emmm.201809302
32. Gupta B, Rai R, Oertel M, Raeman R. Intestinal barrier dysfunction in fatty liver disease: roles of microbiota, mucosal immune system, and bile acids. Semin Liver Dis. (2022) 42:122–37. doi: 10.1055/s-0042-1748037
33. Yu S, Jiang J, Li Q, Liu X, Wang Z, Yang L, et al. Schisantherin A alleviates non-alcoholic fatty liver disease by restoring intestinal barrier function. Front Cell Infect Microbiol. (2022) 12. doi: 10.3389/fcimb.2022.855008
34. Lechner S, Yee M, Limketkai BN, Pham EA. Fecal microbiota transplantation for chronic liver diseases: current understanding and future direction. Dig Dis Sci. (2020) M65:897–905. doi: 10.1007/s10620-020-06100-0
35. Arab JP, Arrese M, Trauner M. Recent insights into the pathogenesis of nonalcoholic fatty liver disease. Annu Rev Pathol. (2018) 13:321–50. doi: 10.1146/annurev-pathol-020117-043617
36. Koyama Y, Brenner DA. Liver inflammation and fibrosis. J Clin Invest. (2017) 127:55–64. doi: 10.1172/JCI88881
37. Schuster S, Cabrera D, Arrese M, Feldstein AE. Triggering and resolution of inflammation in NASH. Nat Rev Gastroenterol Hepatol. (2018) 15:349–64. doi: 10.1038/s41575-018-0009-6
38. Saberi M, Woods NB, de Luca C, Schenk S, Lu JC, Bandyopadhyay G, et al. Hematopoietic cell-specific deletion of toll-like receptor 4 ameliorates hepatic and adipose tissue insulin resistance in high-fat-fed mice. Cell Metab. (2009) 10:419–29. doi: 10.1016/j.cmet.2009.09.006
39. Tanwar S, Rhodes F, Srivastava A, Trembling PM, Rosenberg WM. Inflammation and fibrosis in chronic liver diseases including non-alcoholic fatty liver disease and hepatitis C. World J Gastroenterol. (2020) 1426:109–33. doi: 10.3748/wjg.v26.i2.109
40. Broz P, Dixit VM. Inflammasomes: mechanism of assembly, regulation and signalling. Nat Rev Immunol. (2016) 16:407–20. doi: 10.1038/nri.2016.58
41. de Carvalho Ribeiro M, Szabo G. Role of the inflammasome in liver disease. Annu Rev Pathol. (2022) 17:345–65. doi: 10.1146/annurev-pathmechdis-032521-102529
42. Ridlon JM, Kang DJ, Hylemon PB. Bile salt biotransformations by human intestinal bacteria. J Lipid Res. (2006) 47:241–59. doi: 10.1194/jlr.R500013-JLR200
43. Song Z, Cai Y, Lao X, Wang X, Lin X, Cui Y, et al. Taxonomic profiling and populational patterns of bacterial bile salt hydrolase (BSH) genes based on worldwide human gut microbiome. Microbiome. (2019) 7:9. doi: 10.1186/s40168-019-0628-3
44. Devlin AS, Fischbach MA. A biosynthetic pathway for a prominent class of microbiota-derived bile acids. Nat Chem Biol. (2015) 11:685–90. doi: 10.1038/nchembio.1864
45. Staels B, Fonseca VA. Bile acids and metabolic regulation: mechanisms and clinical responses to bile acid sequestration. Diabetes Care. (2009) 32 Suppl 2:S237–45. doi: 10.2337/dc09-S355
46. Chiang JYL, Ferrell JM. Bile acid metabolism in liver pathobiology. Gene Expr. (2018) 18:71–87. doi: 10.3727/105221618X15156018385515
47. Nie YF, Hu J, Yan XH. Cross-talk between bile acids and intestinal microbiota in host metabolism and health. J Zhejiang Univ Sci B. (2015) 16:436–46. doi: 10.1631/jzus.B1400327
48. Arab JP, Karpen SJ, Dawson PA, Arrese M, Trauner M. Bile acids and nonalcoholic fatty liver disease: Molecular insights and therapeutic perspectives. Hepatology. (2017) 65:350–62. doi: 10.1002/hep.28709
49. Clifford BL, Sedgeman LR, Williams KJ, Morand P, Cheng A, Jarrett KE, et al. FXR activation protects against NAFLD via bile-acid-dependent reductions in lipid absorption. Cell Metab. (2021) 33:1671–1684.e4. doi: 10.1016/j.cmet.2021.06.012
50. Rinella ME, Dufour JF, Anstee QM, Goodman Z, Younossi Z, Harrison SA, et al. Non-invasive evaluation of response to obeticholic acid in patients with NASH: Results from the REGENERATE study. J Hepatol. (2022) 76:536–48. doi: 10.1016/j.jhep.2021.10.029
51. Chiang JY, Pathak P, Liu H, Donepudi A, Ferrell J, Boehme S. Intestinal farnesoid X receptor and takeda G protein couple receptor 5 signaling in metabolic regulation. Dig Dis. (2017) 35:241–5. doi: 10.1159/000450981
52. Onoviran OF, Li D, Toombs Smith S, Raji MA. Effects of glucagon-like peptide 1 receptor agonists on comorbidities in older patients with diabetes mellitus. Ther Adv Chronic Dis. (2019) 10:2040622319862691. doi: 10.1177/2040622319862691
53. Wang YD, Chen WD, Yu D, Forman BM, Huang W. The G-protein-coupled bile acid receptor, Gpbar1 (TGR5), negatively regulates hepatic inflammatory response through antagonizing nuclear factor kappa light-chain enhancer of activated B cells (NF-kappaB) in mice. Hepatology. (2011) 54:1421–32. doi: 10.1002/hep.24525
54. Rao A, van de Peppel IP, Gumber S, Karpen SJ, Dawson PA. Attenuation of the hepatoprotective effects of ileal apical sodium dependent bile acid transporter (ASBT) inhibition in choline-deficient L-amino acid-defined (CDAA) diet-fed mice. Front Med (Lausanne). (2020) 7:60. doi: 10.3389/fmed.2020.00060
55. Chen J, Thomsen M, Vitetta L. Interaction of gut microbiota with dysregulation of bile acids in the pathogenesis of nonalcoholic fatty liver disease and potential therapeutic implications of probiotics. J Cell Biochem. (2019) 120:2713–20. doi: 10.1002/jcb.27635
56. Inagaki T, Moschetta A, Lee YK, Peng L, Zhao G, Downes M, et al. Regulation of antibacterial defense in the small intestine by the nuclear bile acid receptor. Proc Natl Acad Sci U S A. (2006) 103:3920–5. doi: 10.1073/pnas.0509592103
57. Del Chierico F, Nobili V, Vernocchi P, Russo A, De Stefanis C, Gnani D, et al. Gut microbiota profiling of pediatric nonalcoholic fatty liver disease and obese patients unveiled by an integrated meta-omics-based approach. Hepatology. (2017) 65:451–64. doi: 10.1002/hep.28572
58. Chen J, Zheng M, Liu J, Luo Y, Yang W, Yang J, et al. Chinese NAFLD clinical research network (CNAFLD CRN)Ratio of conjugated chenodeoxycholic to muricholic acids is associated with severity of nonalcoholic steatohepatitis. Obesity (Silver spring). Obesity A Res J. (2019) 27:2055–66. doi: 10.1002/oby.22627
59. Miyata M, Funaki A, Fukuhara C, Sumiya Y, Sugiura Y. Taurine attenuates hepatic steatosis in a genetic model of fatty liver disease. J Toxicol Sci. (2020) 45:87–94. doi: 10.2131/jts.45.87
60. Ward JBJ, Lajczak NK, Kelly OB, O’Dwyer AM, Giddam AK, Ní Gabhann J, et al. 2017Ursodeoxycholic acid and lithocholic acid exert anti-inflammatory actions in the colon. Am J Physiol Gastrointest Liver Physiol. (2017) 312:G550–8. doi: 10.1152/ajpgi.00256.2016
61. Seo SH, Lee DH, Lee YS, Cho KJ, Park HJ, Lee HW, et al. Co-administration of ursodeoxycholic acid with rosuvastatin/ezetimibe in a non-alcoholic fatty liver disease model. Gastroenterol Rep (Oxf). (2022) 10:goac037. doi: 10.1093/gastro/goac037
62. Collins SL, Stine JG, Bisanz JE, Okafor CD, Patterson AD. Bile acids and the gut microbiota: metabolic interactions and impacts on disease. Nat Rev Microbiol. (2023) 21:236–47. doi: 10.1038/s41579-022-00805-x
63. Williams CD, Stengel J, Asike MI, Torres DM, Shaw J, Contreras M, et al. Prevalence of nonalcoholic fatty liver disease and nonalcoholic steatohepatitis among a largely middle-aged population utilizing ultrasound and liver biopsy: a prospective study. Gastroenterology. (2011) 140:124–31. doi: 10.1053/j.gastro.2010.09.038
64. Cope K, Risby T, Diehl AM. Increased gastrointestinal ethanol production in obese mice: implications for fatty liver disease pathogenesis. Gastroenterology. (2000) 119:1340–7. doi: 10.1053/gast.2000.19267
65. Baker SS, Baker RD, Liu W, Nowak NJ, Zhu L. Role of alcohol metabolism in non-alcoholic steatohepatitis. PloS One. (2010) 5:e9570. doi: 10.1371/journal.pone.0009570
66. Boursier J, Diehl AM. Nonalcoholic fatty liver disease and the gut microbiome. Clin Liver Dis. (2016) 20:263–75. doi: 10.1016/j.cld.2015.10.012
67. Zhu L, Baker SS, Gill C, Liu W, Alkhouri R, Baker RD, et al. Characterization of gut microbiomes in nonalcoholic steatohepatitis (NASH) patients: a connection between endogenous alcohol and NASH. Hepatology. (2013) 57:601–9. doi: 10.1002/hep.26093
68. Yuan J, Chen C, Cui J, Lu J, Yan C, Wei X, et al. Fatty liver disease caused by high-alcohol-producing klebsiella pneumoniae. Cell Metab. (2019) 30:675–88. doi: 10.1016/j.cmet.2019.08.018
69. Mbaye B, Magdy Wasfy R, Borentain P, Tidjani Alou M, Mottola G, Bossi V, et al. Increased fecal ethanol and enriched ethanol-producing gut bacteria Limosilactobacillus fermentum, Enterocloster bolteae, Mediterraneibacter gnavus and Streptococcus mutans in nonalcoholic steatohepatitis. Front Cell Infect Microbiol. (2023) 13:1279354. doi: 10.3389/fcimb.2023.1279354
70. Fromenty B, Roden M. Mitochondrial alterations in fatty liver diseases. J Hepatol. (2023) 78:415–29. doi: 10.1016/j.jhep.2022.09.020
71. Meijnikman AS, Davids M, Herrema H, Aydin O, Tremaroli V, Rios-Morales M, et al. Microbiome-derived ethanol in nonalcoholic fatty liver disease. Nat Med. (2022) 28:2100–6. doi: 10.1038/s41591-022-02016-6
72. Sumida Y, Yoneda M. Current and future pharmacological therapies for NAFLD/NASH. J Gastroenterol. (2018) 53:362–76. doi: 10.1007/s00535-017-1415-1
73. Albillos A, de Gottardi A, Rescigno M. The gut-liver axis in liver disease: Pathophysiological basis for therapy. J Hepatol. (2020) 72:558–77. doi: 10.1016/j.jhep.2019.10.003
74. Tan JK, Macia L, Mackay CR. Dietary fiber and SCFAs in the regulation of mucosal immunity. J Allergy Clin Immunol. (2023) 151:361–70. doi: 10.1016/j.jaci.2022.11.007
75. Martin-Gallausiaux C, Marinelli L, Blottière HM, Larraufie P, Lapaque N. SCFA: mechanisms and functional importance in the gut. Proc Nutr Soc. (2021) 80:37–49. doi: 10.1017/S0029665120006916
76. Amabebe E, Robert FO, Agbalalah T, Orubu ESF. Microbial dysbiosis-induced obesity: role of gut microbiota in homoeostasis of energy metabolism. Br J Nutr. (2020) 123:1127–37. doi: 10.1017/S0007114520000380
77. Chen J, Vitetta L. Inflammation-modulating effect of butyrate in the prevention of colon cancer by dietary fiber. Clin Colorectal Cancer. (2018) 17:e541–4. doi: 10.1016/j.clcc.2018.05.001
78. Coppola S, Avagliano C, Calignano A, Berni Canani R. The protective role of butyrate against obesity and obesity-related diseases. Molecules. (2021) 26:682. doi: 10.3390/molecules26030682
79. Beisner J, Filipe Rosa L, Kaden-Volynets V, Stolzer I, Günther C, Bischoff SC. Prebiotic inulin and sodium butyrate attenuate obesity-induced intestinal barrier dysfunction by induction of antimicrobial peptides. Front Immunol. (2021) 12:678360. doi: 10.3389/fimmu.2021.678360
80. Zhou D, Pan Q, Xin FZ, Zhang RN, He CX, Chen GY, et al. Sodium butyrate attenuates high-fat diet-induced steatohepatitis in mice by improving gut microbiota and gastrointestinal barrier. World J Gastroenterol. (2017) 23:60–75. doi: 10.3748/wjg.v23.i1.60
81. Kaji I, Karaki S, Kuwahara A. Short-chain fatty acid receptor and its contribution to glucagon-like peptide-1 release. Digestion. (2014) 89:31–6. doi: 10.1159/000356211
82. Zhou D, Chen YW, Zhao ZH, Yang RX, Xin FZ, Liu XL, et al. Sodium butyrate reduces high-fat diet-induced non-alcoholic steatohepatitis through upregulation of hepatic GLP-1R expression. Exp Mol Med. (2018) 50:1–12. doi: 10.1038/s12276-018-0183-1
83. Baumann A, Jin CJ, Brandt A, Sellmann C, Nier A, Burkard M, et al. Oral supplementation of sodium butyrate attenuates the progression of non-alcoholic steatohepatitis. Nutrients. (2020) 12:951. doi: 10.3390/nu12040951
84. Cani PD, Plovier H, Van Hul M, Geurts L, Delzenne NM, Druart C, et al. Endocannabinoids–at the crossroads between the gut microbiota and host metabolism. Nat Rev Endocrinol. (2016) 12:133–43. doi: 10.1038/nrendo.2015.211
85. Forte N, Fernández-Rilo AC, Palomba L, Di Marzo V, Cristino L. Obesity affects the microbiota-gut-brain axis and the regulation thereof by endocannabinoids and related mediators. Int J Mol Sci. (2020) 21:1554. doi: 10.3390/ijms21051554
86. Muccioli GG, Naslain D, Bäckhed F, Reigstad CS, Lambert DM, Delzenne NM, et al. The endocannabinoid system links gut microbiota to adipogenesis. Mol Syst Biol. (2010) 6:392. doi: 10.1038/msb.2010.46
87. Geurts L, Muccioli GG, Delzenne NM, Cani PD. Chronic endocannabinoid system stimulation induces muscle macrophage and lipid accumulation in type 2 diabetic mice independently of metabolic endotoxaemia. PloS One. (2013) 8:e55963. doi: 10.1371/journal.pone.0055963
88. Cani PD, Van Hul M. Gut microbiota in overweight and obesity: crosstalk with adipose tissue. Nat Rev Gastroenterol Hepatol. (2024) 21:164–83. doi: 10.1038/s41575-023-00867-z
89. de Vos WM, Tilg H, Van Hul M, Cani PD. Gut microbiome and health: mechanistic insights. Gut. (2022) 71:1020–32. doi: 10.1136/gutjnl-2021-326789
90. Suriano F, Manca C, Flamand N, Depommier C, Van Hul M, Delzenne NM, et al. Exploring the endocannabinoidome in genetically obese (ob/ob) and diabetic (db/db) mice: Links with inflammation and gut microbiota. Biochim Biophys Acta Mol Cell Biol Lipids. (2022) 1867:159056. doi: 10.1016/j.bbalip.2021.159056
91. Everard A, Plovier H, Rastelli M, Van Hul M, Geurts L, Druart C, et al. Intestinal epithelial N-acylphosphatidylethanolamine phospholipase D links dietary fat to metabolic adaptations in obesity and steatosis. Nat Commun. (2019) 10:457. doi: 10.1038/s41467-018-08051-7
92. Corbin KD, Zeisel SH. Choline metabolism provides novel insights into nonalcoholic fatty liver disease and its progression. Curr Opin Gastroenterol. (2012) 28:159–65. doi: 10.1097/MOG.0b013e32834e7b4b
93. Hernandez GV, Smith VA, Melnyk M, Burd MA, Sprayberry KA, Edwards MS, et al. Dysregulated FXR-FGF19 signaling and choline metabolism are associated with gut dysbiosis and hyperplasia in a novel pig model of pediatric NASH. Am J Physiol Gastrointest Liver Physiol. (2020) 318:G582–609. doi: 10.1152/ajpgi.00344.2019
94. Arias N, Arboleya S, Allison J, Kaliszewska A, Higarza SG, Gueimonde M, et al. The relationship between choline bioavailability from diet, intestinal microbiota composition, and its modulation of human diseases. Nutrients. (2020) 12:2340. doi: 10.3390/nu12082340
95. Chai C, Chen L, Deng MG, Liang Y, Liu F, Nie JQ. Dietary choline intake and non-alcoholic fatty liver disease (NAFLD) in U.S. adults: National Health and Nutrition Examination Survey (NHANES) 2017-2018. Eur J Clin Nutr. (2023) 77:1160–6. doi: 10.1038/s41430-023-01336-1
96. Okubo H, Sakoda H, Kushiyama A, Fujishiro M, Nakatsu Y, Fukushima T, et al. Lactobacillus casei strain Shirota protects against nonalcoholic steatohepatitis development in a rodent model. Am J Physiol Gastrointest Liver Physiol. (2013) 305:G911–8. doi: 10.1152/ajpgi.00225.2013
97. Matsumoto K, Ichimura M, Tsuneyama K, Moritoki Y, Tsunashima H, Omagari K, et al. Fructo-oligosaccharides and intestinal barrier function in a methionine-choline-deficient mouse model of nonalcoholic steatohepatitis. PloS One. (2017) 12:e0175406. doi: 10.1371/journal.pone.0175406
98. Han H, Jiang Y, Wang M, Melaku M, Liu L, Zhao Y, et al. Intestinal dysbiosis in nonalcoholic fatty liver disease (NAFLD): focusing on the gut-liver axis. Crit Rev Food Sci Nutr. (2023) 63:1689–706. doi: 10.1080/10408398.2021.1966738
99. Wigg AJ, Roberts-Thomson IC, Dymock RB, McCarthy PJ, Grose RH, Cummins AG. The role of small intestinal bacterial overgrowth, intestinal permeability, endotoxaemia, and tumour necrosis factor alpha in the pathogenesis of non-alcoholic steatohepatitis. Gut. (2001) 48:206–11. doi: 10.1136/gut.48.2.206
100. Zhang X, Coker OO, Chu ES, Fu K, Lau HCH, Wang YX, et al. Dietary cholesterol drives fatty liver-associated liver cancer by modulating gut microbiota and metabolites. Gut. (2021) 70:761–74. doi: 10.1136/gutjnl-2019-319664
101. Mouzaki M, Comelli EM, Arendt BM, Bonengel J, Fung SK, Fischer SE, et al. Intestinal microbiota in patients with nonalcoholic fatty liver disease. Hepatology. (2013) 58:120–7. doi: 10.1002/hep.26319
102. Shanab AA, Scully P, Crosbie O, Buckley M, O’Mahony L, Shanahan F, et al. Small intestinal bacterial overgrowth in nonalcoholic steatohepatitis: association with toll-like receptor 4 expression and plasma levels of interleukin 8. Dig Dis Sci. (2011) 56:1524–34. doi: 10.1007/s10620-010-1447-3
103. Wang B, Jiang X, Cao M, Ge J, Bao Q, Tang L, et al. Altered fecal microbiota correlates with liver biochemistry in nonobese patients with non-alcoholic fatty liver disease. Sci Rep. (2016) 6:32002. doi: 10.1038/srep32002
104. Hoyles L, Fernández-Real JM, Federici M, Serino M, Abbott J, Charpentier J, et al. Molecular phenomics and metagenomics of hepatic steatosis in non-diabetic obese women. Nat Med. (2018) 24:1070–80. doi: 10.1038/s41591-018-0061-3
105. Shen F, Zheng RD, Sun XQ, Ding WJ, Wang XY, Fan JG. Gut microbiota dysbiosis in patients with non-alcoholic fatty liver disease. Hepatobiliary Pancreat Dis Int. (2017) 16:375–81. doi: 10.1016/S1499-3872(17)60019-5
106. Boursier J, Mueller O, Barret M, MaChado M, Fizanne L, Araujo-Perez F, et al. The severity of nonalcoholic fatty liver disease is associated with gut dysbiosis and shift in the metabolic function of the gut microbiota. Hepatology. (2016) 63:764–75. doi: 10.1002/hep.28356
107. Aron-Wisnewsky J, Vigliotti C, Witjes J, Le P, Holleboom AG, Verheij J, et al. Gut microbiota and human NAFLD: disentangling microbial signatures from metabolic disorders. Nat Rev Gastroenterol Hepatol. (2020) 17:279–97. doi: 10.1038/s41575-020-0269-9
108. Hill C, Guarner F, Reid G, Gibson GR, Merenstein DJ, Pot B, et al. Expert consensus document. The International Scientific Association for Probiotics and Prebiotics consensus statement on the scope and appropriate use of the term probiotic. Nat Rev Gastroenterol Hepatol. (2014) 11:506–14. doi: 10.1038/nrgastro.2014.66
109. Wong VW, Won GL, Chim AM, Chu WC, Yeung DK, Li KC, et al. Treatment of nonalcoholic steatohepatitis with probiotics. A proof-of-concept study. Ann Hepatol. (2013) 12:256–62. doi: 10.1016/S1665-2681(19)31364-X
110. Sabirin F, Lim SM, Neoh CF, Ramasamy K. Hepatoprotection of probiotics against non-alcoholic fatty liver disease in vivo: A systematic review. Front Nutr. (2022) 9:844374. doi: 10.3389/fnut.2022.844374
111. Briskey D, Heritage M, Jaskowski LA, Peake J, Gobe G, Subramaniam VN, et al. Probiotics modify tight-junction proteins in an animal model of nonalcoholic fatty liver disease. Therap Adv Gastroenterol. (2016) 9:463–72. doi: 10.1177/1756283X16645055
112. Naudin CR, Maner-Smith K, Owens JA, Wynn GM, Robinson BS, Matthews JD, et al. Lactococcus lactis Subspecies cremoris Elicits Protection Against Metabolic Changes Induced by a Western-Style Diet. Gastroenterology. (2020) 159:639–51. doi: 10.1053/j.gastro.2020.03.010
113. Wagnerberger S, Spruss A, Kanuri G, Stahl C, Schröder M, Vetter W, et al. Lactobacillus casei Shirota protects from fructose-induced liver steatosis: a mouse model. J Nutr Biochem. (2013) 24:531–8. doi: 10.1016/j.jnutbio.2012.01.014
114. Cano PG, Santacruz A, Trejo FM, Sanz Y. Bifidobacterium CECT 7765 improves metabolic and immunological alterations associated with obesity in high-fat diet-fed mice. Obes (Silver Spring). (2013) 21:2310–21. doi: 10.1002/oby.20330
115. Zhao Z, Chen L, Zhao Y, Wang C, Duan C, Yang G, et al. Lactobacillus plantarum NA136 ameliorates nonalcoholic fatty liver disease by modulating gut microbiota, improving intestinal barrier integrity, and attenuating inflammation. Appl Microbiol Biotechnol. (2020) 104:5273–82. doi: 10.1007/s00253-020-10633-9
116. Park EJ, Lee YS, Kim SM, Park GS, Lee YH, Jeong DY, et al. Beneficial effects of lactobacillus plantarum strains on non-alcoholic fatty liver disease in high fat/high fructose diet-fed rats. Nutrients. (2020) 12:542. doi: 10.3390/nu12020542
117. Wu W, Lv L, Shi D, Ye J, Fang D, Guo F, et al. Protective Effect of Akkermansia muciniphila against Immune-Mediated Liver Injury in a Mouse Model. Front Microbiol. (2017) 8:1804. doi: 10.3389/fmicb.2017.01804
118. Ritze Y, Bárdos G, Claus A, Ehrmann V, Bergheim I, Schwiertz A, et al. Lactobacillus rhamnosus GG protects against non-alcoholic fatty liver disease in mice. PloS One. (2014) 9:e80169. doi: 10.1371/journal.pone.0080169
119. Kim B, Park KY, Ji Y, Park S, Holzapfel W, Hyun CK. Protective effects of Lactobacillus rhamnosus GG against dyslipidemia in high-fat diet-induced obese mice. Biochem Biophys Res Commun. (2016) 473:530–6. doi: 10.1016/j.bbrc.2016.03.107
120. Liu Q, Liu Y, Li F, Gu Z, Liu M, Shao T, et al. Probiotic culture supernatant improves metabolic function through FGF21-adiponectin pathway in mice. J Nutr Biochem. (2020) 75:108256. doi: 10.1016/j.jnutbio.2019.108256
121. Sohn W, Jun DW, Lee KN, Lee HL, Lee OY, Choi HS, et al. Lactobacillus paracasei induces M2-dominant kupffer cell polarization in a mouse model of nonalcoholic steatohepatitis. Dig Dis Sci. (2015) 60:3340–50. doi: 10.1007/s10620-015-3770-1
122. Xin J, Zeng D, Wang H, Ni X, Yi D, Pan K, et al. Preventing non-alcoholic fatty liver disease through Lactobacillus johnsonii BS15 by attenuating inflammation and mitochondrial injury and improving gut environment in obese mice. Appl Microbiol Biotechnol. (2014) 98:6817–29. doi: 10.1007/s00253-014-5752-1
123. Jena PK, Sheng L, Li Y, Wan YY. Probiotics VSL3 are effective in reversing non-alcoholic steatohepatitis in a mouse model. Hepatobiliary Surg Nutr. (2020) 9:170–82. doi: 10.21037/hbsn.2019.09.07
124. Alisi A, Bedogni G, Baviera G, Giorgio V, Porro E, Paris C, et al. Randomised clinical trial: The beneficial effects of VSL3 in obese children with non-alcoholic steatohepatitis. Aliment Pharmacol Ther. (2014) 39:1276–85. doi: 10.1111/apt.12758
125. Famouri F, Shariat Z, Hashemipour M, Keikha M, Kelishadi R. Effects of probiotics on nonalcoholic fatty liver disease in obese children and adolescents. J Pediatr Gastroenterol Nutr. (2017) 64:413–7. doi: 10.1097/MPG.0000000000001422
126. Aller R, De Luis DA, Izaola O, Conde R, Gonzalez Sagrado M, Primo D, et al. Effect of a probiotic on liver aminotransferases in nonalcoholic fatty liver disease patients: a double blind randomized clinical trial. Eur Rev Med Pharmacol Sci. (2011) 15:1090–5.
127. Vajro P, Mandato C, Licenziati MR, Franzese A, Vitale DF, Lenta S, et al. Effects of Lactobacillus rhamnosus strain GG in pediatric obesity-related liver disease. J Pediatr Gastroenterol Nutr. (2011) 52:740–3. doi: 10.1097/MPG.0b013e31821f9b85
128. Xue L, He J, Gao N, Lu X, Li M, Wu X, et al. Probiotics may delay the progression of nonalcoholic fatty liver disease by restoring the gut microbiota structure and improving intestinal endotoxemia. Sci Rep. (2017) 7:45176. doi: 10.1038/srep45176
129. Rong L, Ch’ng D, Jia P, Tsoi KKF, Wong SH, Sung JJY. Use of probiotics, prebiotics, and synbiotics in non-alcoholic fatty liver disease: A systematic review and meta-analysis. J Gastroenterol Hepatol. (2023) 38:1682–94. doi: 10.1111/jgh.16256
130. Xiao MW, Lin SX, Shen ZH, Luo WW, Wang XY. Systematic review with meta-analysis: the effects of probiotics in nonalcoholic fatty liver disease. Gastroenterol Res Pract. (2019) 2019:1484598. doi: 10.1155/2019/1484598
131. Yang R, Shang J, Zhou Y, Liu W, Tian Y, Shang H. Effects of probiotics on nonalcoholic fatty liver disease: a systematic review and meta-analysis. Expert Rev Gastroenterol Hepatol. (2021) 15:1401–9. doi: 10.1080/17474124.2022.2016391
132. Arellano-García L, Portillo MP, Martínez JA, Milton-Laskibar I. Usefulness of probiotics in the management of NAFLD: evidence and involved mechanisms of action from preclinical and human models. Int J Mol Sci. (2022) 23:3167. doi: 10.3390/ijms23063167
133. Campagnoli LIM, Marchesi N, Vairetti M, Pascale A, Ferrigno A, Barbieri A. Age-related NAFLD: the use of probiotics as a supportive therapeutic intervention. Cells. (2022) 11:2827. doi: 10.3390/cells11182827
134. Gibson GR, Hutkins R, Sanders ME, Prescott SL, Reimer RA, Salminen SJ, et al. Expert consensus document: The International Scientific Association for Probiotics and Prebiotics (ISAPP) consensus statement on the definition and scope of prebiotics. Nat Rev Gastroenterol Hepatol. (2017) 14:491–502. doi: 10.1038/nrgastro.2017.75
135. Davani-Davari D, Negahdaripour M, Karimzadeh I, Seifan M, Mohkam M, Masoumi SJ, et al. Prebiotics: definition, types, sources, mechanisms, and clinical applications. Foods. (2019) 8:92. doi: 10.3390/foods8030092
136. Louis P, Flint HJ, Michel C. How to manipulate the microbiota: prebiotics. Adv Exp Med Biol. (2016) 902:119–42. doi: 10.1007/978-3-319-31248-4_9
137. Takai A, Kikuchi K, Ichimura M, Tsuneyama K, Moritoki Y, Matsumoto K, et al. Fructo-oligosaccharides ameliorate steatohepatitis, visceral adiposity, and associated chronic inflammation via increased production of short-chain fatty acids in a mouse model of non-alcoholic steatohepatitis. BMC Gastroenterol. (2020) 20:46. doi: 10.1186/s12876-020-01194-2
138. Yu R, Yin Y, Cao M, Ye D, Zhang Y, Zhou Q, et al. Fructo-oligosaccharides lower serum lipid levels and suppress high-fat/high-sugar diet-induced inflammation by elevating serum and gut levels of short-chain fatty acids. J Int Med Res. (2020) 48:300060519896714. doi: 10.1177/0300060519896714
139. Borges Haubert NJ, Marchini JS, Carvalho Cunha SF, Suen VM, Padovan GJ, Junior JAA, et al. Choline and fructooligosaccharide: non-alcoholic fatty liver disease, cardiac fat deposition, and oxidative stress markers. Nutr Metab Insights. (2015) 8:1–6. doi: 10.4137/NMI.S24385
140. Pachikian BD, Essaghir A, Demoulin JB, Catry E, Neyrinck AM, Dewulf EM, et al. Prebiotic approach alleviates hepatic steatosis: implication of fatty acid oxidative and cholesterol synthesis pathways. Mol Nutr Food Res. (2013) 57:347–59. doi: 10.1002/mnfr.201200364
141. Weitkunat K, Schumann S, Petzke KJ, Blaut M, Loh G, Klaus S. Effects of dietary inulin on bacterial growth, short-chain fatty acid production and hepatic lipid metabolism in gnotobiotic mice. J Nutr Biochem. (2015) 26:929–37. doi: 10.1016/j.jnutbio.2015.03.010
142. Chong CYL, Orr D, Plank LD, Vatanen T, O’Sullivan JM, Murphy R. Randomised double-blind placebo-controlled trial of inulin with metronidazole in non-alcoholic fatty liver disease (NAFLD). Nutrients. (2020) 12:937. doi: 10.3390/nu12040937
143. Daubioul CA, Horsmans Y, Lambert P, Danse E, Delzenne NM. Effects of oligofructose on glucose and lipid metabolism in patients with nonalcoholic steatohepatitis: results of a pilot study. Eur J Clin Nutr. (2005) 59:723–6. doi: 10.1038/sj.ejcn.1602127
144. Yang Z, Su H, Lv Y, Tao H, Jiang Y, Ni Z, et al. Inulin intervention attenuates hepatic steatosis in rats via modulating gut microbiota and maintaining intestinal barrier function. Food Res Int. (2023) 163:112309. doi: 10.1016/j.foodres.2022.112309
145. Wang R, Ren Y, Bao T, Wang T, Li Y, Liu Y, et al. Inulin activates FXR-FGF15 signaling and further increases bile acids excretion in non-alcoholic fatty liver disease mice. Biochem Biophys Res Commun. (2022) 600:156–62. doi: 10.1016/j.bbrc.2022.02.033
146. Pérez-Monter C, Álvarez-Arce A, Nuño-Lambarri N, Escalona-Nández I, Juárez-Hernández E, Chávez-Tapia NC, et al. Inulin improves diet-induced hepatic steatosis and increases intestinal akkermansia genus level. Int J Mol Sci. (2022) 23:991. doi: 10.3390/ijms23020991
147. Reshef N, Gophna U, Reshef L, Konikoff F, Gabay G, Zornitzki T, et al. Prebiotic treatment in patients with nonalcoholic fatty liver disease (NAFLD)-A randomized pilot trial. Nutrients. (2024) 16:1571. doi: 10.3390/nu16111571
148. Swanson KS, Gibson GR, Hutkins R, Reimer RA, Reid G, Verbeke K, et al. The International Scientific Association for Probiotics and Prebiotics (ISAPP) consensus statement on the definition and scope of synbiotics. Nat Rev Gastroenterol Hepatol. (2020) 17:687–701. doi: 10.1038/s41575-020-0344-2
149. Eslamparast T, Poustchi H, Zamani F, Sharafkhah M, Malekzadeh R, Hekmatdoost A. Synbiotic supplementation in nonalcoholic fatty liver disease: a randomized, double-blind, placebo-controlled pilot study. Am J Clin Nutr. (2014) 99:535–42. doi: 10.3945/ajcn.113.068890
150. Malaguarnera M, Vacante M, Antic T, Giordano M, Chisari G, Acquaviva R, et al. Bifidobacterium longum with fructo-oligosaccharides in patients with non alcoholic steatohepatitis. Dig Dis Sci. (2012) 57:545–53. doi: 10.1007/s10620-011-1887-4
151. Rivero-Gutiérrez B, Gámez-Belmonte R, Suárez MD, Lavín JL, Aransay AM, Olivares M, et al. A synbiotic composed of Lactobacillus fermentum CECT5716 and FOS prevents the development of fatty acid liver and glycemic alterations in rats fed a high fructose diet associated with changes in the microbiota. Mol Nutr Food Res. (2017) 61:1–8. doi: 10.1002/mnfr.201600622
152. Safavi M, Farajian S, Kelishadi R, Mirlohi M, Hashemipour M. The effects of synbiotic supplementation on some cardio-metabolic risk factors in overweight and obese children: a randomized triple-masked controlled trial. Int J Food Sci Nutr. (2013) 64:687–93. doi: 10.3109/09637486.2013.775224
153. Ipar N, Aydogdu SD, Yildirim GK, Inal M, Gies I, Vandenplas Y, et al. Effects of synbiotic on anthropometry, lipid profile and oxidative stress in obese children. Benef Microbes. (2015) 6:775–82. doi: 10.3920/BM2015.0011
154. Alves CC, Waitzberg DL, de Andrade LS, Dos Santos Aguiar L, Reis MB, Guanabara CC, et al. Prebiotic and synbiotic modifications of beta oxidation and lipogenic gene expression after experimental hypercholesterolemia in rat liver. Front Microbiol. (2017) 8:2010. doi: 10.3389/fmicb.2017.02010
155. Musazadeh V, Assadian K, Rajabi F, Faghfouri AH, Soleymani Y, Kavyani Z, et al. The effect of synbiotics on liver enzymes, obesity indices, blood pressure, lipid profile, and inflammation in patients with non-alcoholic fatty liver: a systematic review and meta-analysis of randomized controlled trials. Pharmacol Res. (2024) 208:107398. doi: 10.1016/j.phrs.2024.107398
156. Liu L, Li P, Liu Y, Zhang Y. Efficacy of probiotics and synbiotics in patients with nonalcoholic fatty liver disease: A meta-analysis. Dig Dis Sci. (2019) 64:3402–12. doi: 10.1007/s10620-019-05699-z
157. Yao F, Jia R, Huang H, Yu Y, Mei L, Bai L, et al. Effect of Lactobacillus paracasei N1115 and fructooligosaccharides in nonalcoholic fatty liver disease. Arch Med Sci. (2019) 15:1336–44. doi: 10.5114/aoms.2019.86611
158. Bilson J, Oquendo CJ, Read J, Scorletti E, Afolabi PR, Lord J, et al. Markers of adipose tissue fibrogenesis associate with clinically significant liver fibrosis and are unchanged by synbiotic treatment in patients with NAFLD. Metabolism. (2024) 151:155759. doi: 10.1016/j.metabol.2023.155759
159. Scorletti E, Afolabi PR, Miles EA, Smith DE, Almehmadi A, Alshathry A, et al. Synbiotics alter fecal microbiomes, but not liver fat or fibrosis, in a randomized trial of patients with nonalcoholic fatty liver disease. Gastroenterology. (2020) 158:1597–1610.e7. doi: 10.1053/j.gastro.2020.01.031
160. Pan Y, Yang Y, Wu J, Zhou H, Yang C. Efficacy of probiotics, prebiotics, and synbiotics on liver enzymes, lipid profiles, and inflammation in patients with non-alcoholic fatty liver disease: a systematic review and meta-analysis of randomized controlled trials. BMC Gastroenterol. (2024) 24:283. doi: 10.1186/s12876-024-03356-y
161. Hoffmann DE, Palumbo FB, Ravel J, Rowthorn V, von Rosenvinge E. A proposed definition of microbiota transplantation for regulatory purposes. Gut Microbes. (2017) 8:208–13. doi: 10.1080/19490976.2017.1293223
162. Minkoff NZ, Aslam S, Medina M, Tanner-Smith EE, Zackular JP, Acra S, et al. Fecal microbiota transplantation for the treatment of recurrent Clostridioides difficile (Clostridium difficile). Cochrane Database Syst Rev. (2023) 4:CD013871. doi: 10.1002/14651858.CD013871.pub2
163. Merrick B, Allen L, Masirah M Zain N, Forbes B, Shawcross DL, Goldenberg SD. Regulation, risk and safety of Faecal Microbiota Transplant. Infect Prev Pract. (2020) 2:100069. doi: 10.1016/j.infpip.2020.100069
164. Zhou D, Pan Q, Shen F, HX C, WJ D, YW C, et al. Total fecal microbiota transplantation alleviates high-fat diet-induced steatohepatitis in mice via beneficial regulation of gut microbiota. Sci Rep. (2017) 7:1529. doi: 10.1038/s41598-017-01751-y
165. Craven L, Rahman A, Nair Parvathy S, Beaton M, Silverman J, Qumosani K, et al. Allogenic fecal microbiota transplantation in patients with nonalcoholic fatty liver disease improves abnormal small intestinal permeability: A randomized control trial. Am J Gastroenterol. (2020) 115:1055–65. doi: 10.14309/ajg.0000000000000661
166. Witjes JJ, Smits LP, Pekmez CT, Prodan A, Meijnikman AS, Troelstra MA, et al. Donor fecal microbiota transplantation alters gut microbiota and metabolites in obese individuals with steatohepatitis. Hepatol Commun. (2020) 4:1578–90. doi: 10.1002/hep4.1601
Keywords: non-alcoholic fatty liver disease (NAFLD), non-alcoholic steatohepatitis (NASH), gut microbiome, gut microbiota, gut dysbiosis
Citation: Mpountouridis A, Tsigalou C, Bezirtzoglou I, Bezirtzoglou E and Stavropoulou E (2025) Gut microbiome in non-alcoholic fatty liver disease. Front. Gastroenterol. 3:1534431. doi: 10.3389/fgstr.2024.1534431
Received: 25 November 2024; Accepted: 26 December 2024;
Published: 14 January 2025.
Edited by:
Palash Mandal, Charotar University of Science and Technology, IndiaReviewed by:
Hui Xiang, Renmin Hospital of Wuhan University, ChinaCopyright © 2025 Mpountouridis, Tsigalou, Bezirtzoglou, Bezirtzoglou and Stavropoulou. This is an open-access article distributed under the terms of the Creative Commons Attribution License (CC BY). The use, distribution or reproduction in other forums is permitted, provided the original author(s) and the copyright owner(s) are credited and that the original publication in this journal is cited, in accordance with accepted academic practice. No use, distribution or reproduction is permitted which does not comply with these terms.
*Correspondence: Anastasios Mpountouridis, YS5tcG91bnRvdXJpZGlzQGdtYWlsLmNvbQ==
Disclaimer: All claims expressed in this article are solely those of the authors and do not necessarily represent those of their affiliated organizations, or those of the publisher, the editors and the reviewers. Any product that may be evaluated in this article or claim that may be made by its manufacturer is not guaranteed or endorsed by the publisher.
Research integrity at Frontiers
Learn more about the work of our research integrity team to safeguard the quality of each article we publish.