- 1Laboratorio de Bioquímica e Fisiologia de Insetos, Instituto Oswaldo Cruz, Fiocruz, Rio de Janeiro, Brazil
- 2Instituto Nacional de Ciência e Tecnologia em Entomologia Molecular, Rio de Janeiro, Brazil
The application of microorganisms as bio-control agents against arthropod populations is a need in many countries, especially in tropical, subtropical, and neotropical endemic areas. Several arthropod species became agricultural pests of paramount economic significance, and many methods have been developed for field and urban applications to prevent their, the most common being the application of chemical insecticides. However, the indiscriminate treatment based upon those substances acted as a selective pressure for upcoming resistant phenotype populations. As alternative tools, microorganisms have been prospected as complementary tools for pest and vectorial control, once they act in a more specific pattern against target organisms than chemicals. They are considered environmentally friendly since they have considerably less off-target effects. Entomopathogenic fungi are organisms capable of exerting pathogenesis in many vector species, thus becoming potential tools for biological management. The entomopathogenic fungi Metarhizium sp. have been investigated as a microbiological agent for the control of populations of insects in tropical regions. However, the development of entomopathogenic fungi as control tools depends on physiological studies regarding aspects such as mechanisms of pathogenicity, secreted enzymes, viability, and host-pathogen aspects. The following review briefly narrates current aspects of entomopathogenic fungi, such as physiology, cellular characteristics, host-pathogen interactions, and its previous applications against different insect orders with medical and economic importance. Approaches integrating new isolation, prospection, characterization, delivery strategies, formulations, and molecular and genetic tools will be decisive to elucidate the molecular mechanisms of EPFs and to develop more sustainable alternative pesticides.
Introduction
Metarhizium sp. is a filamentous fungus belonging to the order Hypocreales. It is known for its diverse ecological roles and significant applications in agriculture and biotechnology. This entomopathogenic fungus has garnered considerable attention due to its ability to parasitize a wide range of arthropod hosts, making it a promising candidate for biological pest control strategies (Skinner et al., 2014).
Initially discovered and described by Sorokin in 1883 (Ann et al., 1995), M. anisopliae has since been extensively studied for its pathogenic mechanisms and genetic diversity. The fungus exhibits a complex life cycle involving spore germination, insect cuticle penetration and subsequent colonization and growth within the host hemocoel (Bihal et al., 2023). This pathogenicity is facilitated by a range of bioactive compounds and enzymes the fungus produces, which aid in host tissue degradation and nutrient acquisition (Butt et al., 2016).
Beyond its role as a pathogen, M. anisopliae has shown potential in various biotechnological applications, including biocontrol of agricultural pests (as it is applied mainly as a mycoinsecticide for certain crops) and vectors of human and animal diseases (Iwanicki et al., 2019; Vivekanandhan et al., 2022; Faria et al., 2023). Its environmental adaptability and ability to thrive in diverse habitats underscore its potential as a sustainable alternative to chemical pesticides (Verma et al., 2023). Furthermore, ongoing research into its genomic structure and molecular biology promises insights into its evolution and adaptation strategies.
In this review, we explore the ecological significance of general fungi, specially Entomopathogenic Fungi (EPFs), with a focus on Metarhizium anisopliae. We detail it’s biological characteristics, host-pathogen interaction, and discuss the practical applications of M. anisopliae, highlighting its potential contributions to pest management and biotechnological innovations. We aim to provide a comprehensive overview of this intriguing fungus and its impact on sustainable agriculture and environmental management by synthesizing current knowledge and research trends.
The reviewed articles are summarized in Supplementary Table 1 (see Supplementary Material), which includes details on fungus lineage, target arthropod, target insect arthropod order, main results obtained from Metarhizium anisopliae, and bibliographic references.
General aspects of fungi
Fungi are a taxonomical group possessing profuse biodiversity, containing approximately 2.2 to 3.8 million species (Hawksworth and Lücking, 2017). Fungi are eukaryotic and heterotrophic organisms, single cell (like yeasts) or multicellular macroscopic fungi (like mushrooms). However, they have varied nutritional habits, being able to absorb organic matter from dead organisms (saprophytic) or infect living hosts using specialized propagules of mild duriform or sporiform shape (Mora et al., 2018).
In some reproductive cycles of filamentous fungi (conidia, zoospores, ascospores, basidia, or chlamydospores), specialized structures known as sporophores cleave themselves to form asexual propagules known as conidia with chitinized cell walls. The composition of a conidium wall may include the presence of mannans, galactans, glycans, and chitosan as constituents. After enough nutrient acquisition, the conidium develops in hyphae, which can generate the conidiophores and, sequentially, the sporophores for another cycle (Samson et al., 1988; Blackwell, 2010). In fungal sexual reproduction (found in teleomorphic fungi), the occurrence of plasmogamy and karyogamy of sexual gametes play the main role in genetic diversification and maintenance of the fungal life cycle by the generation of reproductive mycelium. Thereunto, both gametes need sexual complementarity, expressed by the existence of different mating types: mating type +/- or a/α, which varies widely across the taxonomic groups (Zimmermann, 2007; Wallen and Perlin, 2018). It is essential to mention that teleomorphic fungi and anamorphic fungi (fungi that reproduce only asexually) are capable of infecting arthropod hosts (Mora et al., 2018). From this perspective, this review aims to compile the physiological processes of host-pathogen interaction and its historical and applied aspects.
Entomopathogenic fungi (EPFs)
As previously mentioned, M. anisopliae is a highly prospected microorganism for integrated management of pests and vectors. Particularities in its biology are responsible for optimal maintenance of several important features, such as virulence, resistance to abiotic factors (such as desiccation, radiation, and temperature), suppression of other microorganisms with the synthesis of allelopathic molecules, and the ability to evade the host’s immune system once successfully infected (Sevim et al., 2012; Barelli et al., 2016; Donzelli and Krasnoff, 2016; Wang et al., 2016).
Mostly in nature, EPFs are essentially terrestrial beings, capable of infecting their host with the propagation of their conidia via passive horizontal dissemination or auto-disseminating mechanisms, which occurs by direct contact with the corpse of the sporulated host (Juarez et al., 2011; Araújo and Hughes, 2016).
The species status of the Metarhizium taxa has undergone phylogenetic reformulations and revisions over time, being promoted to a complex of species and variations, according to Bischoff et al (Bischoff et al., 2009). In this study, the authors redefine the cladistic relationships between species of the genus from quasi-total sequencing of the Genes EF-1α (Elongating Factor), RPB1 (major subunit of RNA polymerase II), β-tubulin and IGS (ribosomal nuclear intergenic spacing region), concurrent with macro and micromorphological aspects, such as size and shape of propagules (classified as conidia, blastospores and “swollen conidia”), size of hyphas, fialidis and conidiophores from 57 isolates.
Derived from this work, the relocation of 6 species was concluded: M. anisopliae (formerly a complex permeated by variations), M. guizhouense, M. pingshaense, M. acridum, M. lepidiotae, and M. majus. Receiving the status of a new species, they promoted: M. globosum, M. robertsii, and M. brunneum, the last being used as synonymy for M. anisopliae by several authors.
Physiological aspects and host-pathogen interaction
a) Physiological/molecular changes in pathogen
Fungi interact with different living beings, establishing symbiosis, commensalism, or pathogenicity relationships. Entomopathogenic fungi (EPF) are fungi capable of infecting and developing in arthropod hosts, disposing of a plethora of physical, biochemical, and biological mechanisms (Samson et al., 1988). Although the interactions between fungi and arthropods might be diverse, the classical interface involves adherence of propagules to the host’s cuticle (Butt et al., 2016; Mora et al., 2018). Once in contact with the insect, the conidia (a form derived from the asexual reproduction of the fungus) adheres to the cuticle through physical and biochemical interactions, whose main mechanisms involve hydrophobic interactions promoted by hydrophobins. Such surface proteins are coded in M. anisopliae by the HYD1/ssgA and HYD3 genes, producing class I hydrophobins and HYD 2, producing class II hydrophobins (Sevim et al., 2012). The mutation or knockout of these genes affects sporulation capacity, pigmentation, and macromorphological aspects of the fungus, causing a marked decrease in virulence since this impairs the initial mechanism of conidia-cuticle interaction (Sevim et al., 2012; Wang et al., 2016).
Adhesin-like proteins also constitute the machinery of adhesion on the insect´s cuticular surface by M. anisopliae. Proteins encoded by MAD I genes (adhesine-like I - able to promote adhesion on the surface of arthropod hosts) and MAD II (adhesine-like 2 - promoter of adhesive interaction on plant surfaces) assist in active adhesion and signaling for the budding of the appressorium, followed by penetration into the host organism and further colonization. In the case of hydrophobins, once the propagule adheres, the protein is degraded and removed from the propagule wall (Wösten, 2001; Wang and St. Leger, 2007; Wyrebek and Bidochka, 2013; Yang et al., 2023).
According to Wang and Leger (Wang and St. Leger, 2007), the imbalance in MAD I expression promoted delays in germination, low differentiation in blastospores, and high reduction in virulence to Manduca sexta caterpillars. At the same time, the impairment of Mad II expression did not compromise pathogenicity in the animal host (Wang and St. Leger, 2007).
Similar to adherence, recognition is essential for the infective capacity of the fungus. The enzyme glyceraldehyde-3-phosphate dehydrogenase is another wall constituent of M. anisopliae that is also responsible for molecular adhesion mechanisms. This enzyme acts as an adhesine-like protein. It is differentially synthesized after exposure to different carbon sources, also composing the enzymatic machinery that will promote the lysis of the host cuticle (Broetto et al., 2010). After recognition and adhesion, cascades of biochemical signs, such as those of protein class kinases A, promote a change in the composition of the fungal cell wall. This change allows the increase of turgor, favoring the budding and emergence of the appressorium (Fang et al., 2009; Butt et al., 2016).
During differentiation, the fungus utilizes energy reserves stocked up as carbon sources like lipids, trehalose, glycogen, and erythritol (Hallsworth and Magan, 1996). Perilipin-like proteins are evolutionarily conserved in various organisms, such as fungi, frogs, and mammals, and are responsible for triacylglycerol breakdown (Miura et al., 2002; Bickel et al., 2010). In the genus Metarhizium, perilipin-like proteins are encoded by the Mpl 1 gene. They can convert these sources of carbon from lipid droplets, which will be consumed mainly during the differentiation of fungal apressorium at the time of penetration into the host cuticle (Wang and St. Leger, 2006; Bickel et al., 2010; Butt et al., 2016).
The key enzymes that promote penetration of the appressorium are chitinases and proteases (Butt et al., 2016). The Pr1 serine endopeptidases are the main proteases in Metarhzium sp and consist of 11 isoforms distributed throughout the genus, subdivided into two classes and three subfamilies (Andreis et al., 2019). In synergism, such different isoforms of Protease Pr1 together with lipases, N-acetylglycosaminidases, and chitinases, will aid in cuticular degradation, allowing the invasion of the arthropod hemocele and consequently trigger immunological responses (Small and Bidochka, 2005).
In the context of later stages of infection, EPF propagules developed adaptations to avoid the humoral and cellular components of the host, as they stimulate the host’s immune system. According to Verma et al (Wang and St. Leger, 2006). one of the most notorious examples of avoidance mechanisms is performed by the MCL1 gene in M. anisopliae. This gene encodes a hydrophilic trimeric protein containing an N-terminal region with 14 cysteine residues, negatively charged with tandem regions, and a C-terminal region. The C-terminal contains an attachment site to the cell wall, dependent on glycosylphosphatidylinositol, which, simultaneously with other physiological aspects, provides the fungus with anti-adhesive capacity, making it difficult to adhere to plasmatocytes and other phagocytic hemocytes.
Along with the immunological pressures exerted on the propagules of EPFs, the fungus needs adaptation to the osmotic pressures of the host’s hemolymph. In M. anisopliae, the Mos1 gene encodes the transmembrane protein Mos1, structurally similar to the osmotic regulators found in yeasts such as Candida albicans and Saccharomyces cerevisae, the specific SHO1 and SLN1 receptors, that are positive regulators of the map kinase pathway controlling the cell cycle (Alonso Monge et al., 2006; Tatebayashi et al., 2007). Widely distributed in Fungi, these osmoregulatory proteinscan in M. anisopliae can preserve the integrity of the cell wall when exposed to oxidative stress. It was observed that its silencing reduced the viability of the fungus in the hemocoel, further compromising the regulation of genes related to growth factors and differentiation in the hemolymph during host colonization (Wang et al., 2008).
Furthermore, in yeasts of the species Saccharomyces cerevisae, PacC/Rim101 transcription factors regulate gene expression in an alkaline environment, repressing it when in a very acidic one (Peñalva et al., 2008). However, in Metarhizium robertsii, another generalist EPF, this same gene family is essential for evading the immunological components of the host; besides being responsible for survival in an osmotically stressful environment, penetration of the cuticle, colonization of hemolymph in the host, and other aspects that characterize virulence (Huang et al., 2015; Xie et al., 2019).
Other fungal molecular and cellular mechanisms for evading the host immune system remain unknown and require further studies since such interaction interfaces present complexities not only at physiological but also at the evolutionary-adaptive level. After overcoming the physiological barriers of the insect, conidia differentiate into blastospores, which propagate inside the hemocoel. After successful colonization of the host, the fungus emerges by lytic activity mediated by proteases, chitinases, lipases, acetylglycosaminidases, and secondary metabolites of the most varied molecular classes, breaking the cuticle from the inside to assume the filamentous form in the exterior of the host carcass. Then, sporulation and dispersion of propagules take place (Barelli et al., 2016; Butt et al., 2016).
b) Molecular level perspective in the host after host-pathogen interaction
After the invasion of the insect’s celomatic cavity, the immune system is activated through recognition by receptors associated with molecular patterns (Lu et al., 2014) to counteract the presence of a pathogenic foreign microorganism. This invasion is illustrated in Figure 1, which depicts the different stages of Metarhizium sp. infection (labeled 1 to 6) in an aphid, along with the detailed immune system response of the insect at stage 4.
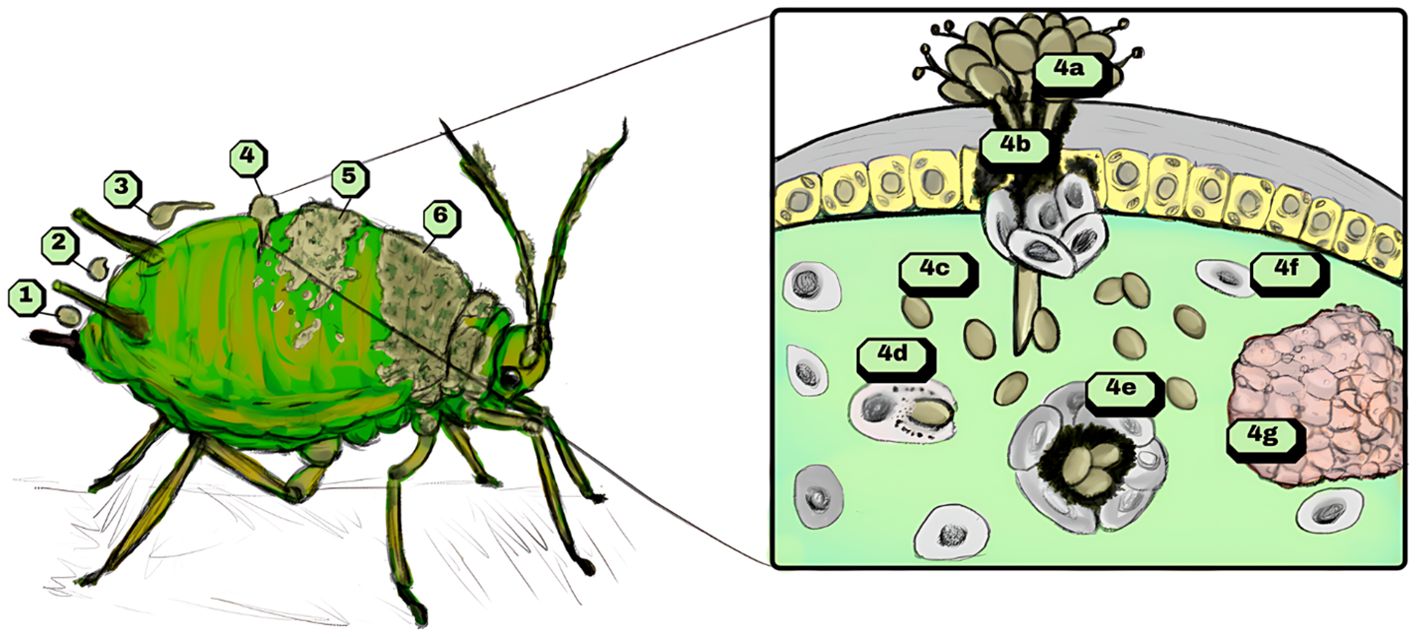
Figure 1. Process of infection of entomopathogenic fungi Metarhizium sp. in an insect host. Recognition and adherence are depicted in infection phases 1 and 2. Appressorium formation is stimulated shortly after adherence (3), and enzymatic secretion along with penetration force results in the invasion of fungi in the host celome (4). The host’s immune reaction resulting from the infection may manifest by humoral and cellular responses, as follows: melanization from phenoloxidase cascade (4a), which surrounds and encapsulates the fungal propagules with circulating cascade precursors. Simultaneously, cellular encapsulation mitigates the infection by recruiting other immune cells (4b). As fungi differentiate from hyphal structures to blastospores in the celome (4c), phagocytosis by plasmatocytes and granular cells (4d), deposition of melanin by oenocytes (4e) and formation of nodules around propagules by recruited immune cells (4f, 4g) are common immunological responses to fungal infection. After evasion from the host’s immune system, the pathogen spreads (5) and finishes its cycle, producing spores across the cuticle (6).
During the infection, one of the first components of the host’s humoral immune response to be activated is the phenoloxidase (PO) cascade. The PO cascade consists of the sequential activation of several serine proteases, which will cleave the prophenoloxidase zymogen (pre-proPO) into its second form, prophenoloxidase (proPO). Other serine proteases of unknown nature cleave the second zymogen for the activation of proPO into PO. Then, the active PO catalyzes the oxidation of phenols in quinones, which are components of the polymerization of melanin, a molecule that will control the propagation of the pathogen (Cerenius and Söderhäll, 2004; Binggeli et al., 2014; Lu et al., 2014).
As a result of the recognition of molecular patterns associated with pathogenic microorganisms, the synthesis of antimicrobial peptides (AMP) is essential to combat a variety of infections. These AMPs are small molecules with, on average, less than 10 kDa (equivalent to peptides between 12 and 50 amino acids). The fat body is the main site of expression and synthesis of these peptides. This organ comes ontologically from the mesoderm and is the host organism’s largest source of immunological responses (cellular and systemic) (Lemaitre and Hoffmann, 2007). About 20 immunologically induced AMPs have been described, with great antimicrobial specificity, depending on the invasive pathogen. For example, attacins have a more significant effect on gram-negative bacteria, defensins on gram-positive bacteria, and drosomycins and cecropins have higher antifungal activity (Lemaitre and Hoffmann, 2007; Cohen et al., 2020).
Most of these AMPs occur throughout the Hexapoda class and can be divided into three groups: (i) peptides rich in proline and glycine residues, (ii) defensins with three to four disulfide bridges between conserved cysteine residues, and (iii) cecropins, linear peptides that are rich in alpha-helix (Wu et al., 2018).
As mentioned above, drosomycins and cecropins, among other antimicrobial peptides, have antifungal capabilities for controlling a possible EPF infection. The signaling cascade responsible for this particular response is the Toll pathway, which is highly conserved in mammals. In most insects, this pathway encompasses recognizing specific fungal molecules such as chitosan and beta-1,3-glucans, for example (De Lima Batista et al., 2018).
After recognizing such fungal wall components, the Toll pathway is activated by the binding of one of the cleaved forms of the Spätzle cytokine, which is still immature. After successive cleavages of the cytokine, it binds as a dimer in the Toll receptor, promoting intracellular recruitment of three proteins containing Death domains (molecular subunits that constitute adapters in receptors responsible for the transfer of chemical groups (kinases in Drosophila and other Diptera, for example) or, in mammals, by activation of the procaspase pathways 8 and 10 (Kaufmann and Hengartner, 2001; Cooper et al., 2009; Walderdorff et al., 2019).
Proteins as MyD88, Tube, and Pelle will act in the negative regulation of other systemic immunological pathways (more precisely, IMD and Cactus) by the ubiquitination of immunological components that are posteriorly directed to the proteasome. In the cells of the fat body, the transcription factors positively regulated (Dif and Dorsal) by the action of these three proteins act on specific genes of cecropins, drosocins, metchnikowins, and other AMP genes (Ligoxygakis et al., 2002; Tauszig-Delamasure et al., 2002; Lemaitre and Hoffmann, 2007).
Another immune response against EPF infections in insects is the cellular response, related to hemocytes activated after recognition of Pathogen Associated Molecular Patterns (PAMPs). There are different types of hemocytes in the insect hemolymph, each with a specific activity. Prohemocytes are pluripotent immature cellular populations, capable of differentiating mainly in lamelocytes (these occurring mostly in larvae of holometabolous individuals), granulocytes, and plasmatocytes (Pondeville et al., 2020). Plasmatocytes in different insect species are capable of phagocyte invading microorganisms (Ratcliffe and Gagen, 1977; Lackie, 1988; Walderdorff et al., 2019). Granulocytes, in synergism with plasmatocytes, can encapsulate and promote the nodulation of foreign agents, whether cells or parasitic/parasitoid organisms. Oenocytoids and crystal cells play an essential role in the PO cascade. The inactive PPO is stored in these cells, and after recognition of PAMPs, they degranulate, releasing the enzyme into the hemolymph (Lu et al., 2014). The hemocyte types vary according to the insect species; some cells such as spherocytes and coagulocytes have unknown functions. However, they may play physiological roles related to the cellular and humoral immune systems (Lemaitre and Hoffmann, 2007; Hernandez et al., 2010; Cho and Cho, 2019; Ross et al., 2020).
Historical aspects of arthropods’ control
Taking together the environmental benefits, mechanisms of action, and practical aspects of EPF biopesticides, several studies have been carried out on M. anisoplae and its activity in different orders of Insecta. These studies aim to prospect the potential for large-scale employment against the most diverse pests and vectors. In this context, it is relevant to consider the most important facts related to insecticide evolution for arthropod pests and vectors and when the EPF’s biopesticides started to be developed.
Along with the ascension of civilization and agriculture populations of insect pests and vectors gradually began to adapt to human environments in a coevolutionary process. This led to the prospection of measures to restrict these organisms’ population size, aiming at reducing their impact on health and economy. As the most well-known measures used in the current scenario, chemical insecticides are broadly utilized as populational deterrents. According to the World Health Organization (WHO), the most common chemical insecticides are currently represented by molecular classes such as pyrethroids, organochlorides, and organophosphates, which act in the target organisms as neurological disruptors (Nicolopoulou-Stamati et al., 2016; Wilson et al., 2020).
The modern advent of chemical pesticides as a major population control measure for pests and vectors began in the 1940s, using the insecticide dichlorodiphenyltrichloroethane (DDT) to control Anopheles mosquitoes that transmit the etiologic agent of malaria, the Plasmodium parasite. Other chemical classes gradually replaced this insecticide due to its high toxicity and residuality. New active substances against insects were discovered with several modes of action and properties, such as acetylcholinesterase (AChE) inhibitors, gaba-gated chlorine channel blockers, sodium channel modulators, and nicotinic acetylcholine receptor (nAChR) competitive modulators. However, all these compounds target the same insect’s physiological system, the nervous, providing fast action (Casida and Quistad, 1998; Bate, 2007; Oberemok et al., 2015; Nicolopoulou-Stamati et al., 2016; Isman, 2020; IRAC, 2023). Although they have been and necessary for insect control, these chemical compounds have triggered detrimental effects, similar to DDT, such as poisoning of non-target beneficial species as well as mammals. However, current chemical classes, such as pyrethroids, present low toxicity to humans. In addition, the residual accumulation of these substances in various trophic chains poses a significant threat to ecosystems. Besides that, inappropriate use of chemical insecticides may lead to the selection of resistant populations with physiological changes for the individual, such as increased cuticle thickness, increased synthesis of detoxifying enzymes (e.g., cytochrome p450 class proteins), behavioral changes culminating in the avoidance of the control measures, and other adaptations (Hemingway and Ranson, 2000; Puinean et al., 2010; Kasai et al., 2014; Nicolopoulou-Stamati et al., 2016; Zalucki and Furlong, 2017).
The investigation of alternative ways to contour the obstacles linked to the use of chemical pesticides led to the prospection of new classes of products, for example, plant-based insecticides, which can act as hormonal analogs or antagonists, such as neem. Also, microorganism-based pesticides can infect the insects, leading to their death. It exerts negative ecological pressure on target organisms, being more selective and causing less residual and harmful effects to the environment, being more sustainable to pests/vectors populational management (Carvalho, 2017; Senthil-Nathan, 2020).
The plant-based insecticides (derived from secondary plant metabolites or phytochemicals) are highly prospected products for pest/vector management, acting by different mechanisms over its physiological system, whether in the cellular, tissue, or systemic levels. For example, bruchelin and podofilotoxin, two molecules belonging to the class of neolignans, were able to alter the excretion system of the blood-feeding triatomine Rhodnius prolixus (Hemiptera, Reduviidae), an important vector in the transmission of Trypanosoma cruzi, the etiological agent of Chagas disease. These compounds reduce diuretic hormones in the hemolymph and Malpighian tubules (Garcia et al., 2000). It was also seen that gonadulin, an insulin-like peptide, is highly expressed in the calyx of the R. prolixus females’ reproductive system. This peptide is associated with the modulation of ovulation and oviposition, implying that the insulin cascade is essential for egg production and is, therefore, a possible target for populational control of this insect (Leyria et al., 2023).
In Aedes aegypti (Diptera, Culicidae, a mosquito of great sanitary relevance for its’ competence as a vector of several viral diseases like yellow fever, dengue, Zika, Mayaro, and chikungunya), phytochemicals have a broad spectrum with systemic action. Such substances are effective in promoting changes in the cuticle (tissue level) and membrane components (such as the lipid bilayer), different proteins, such as enzymes, transmembrane receptors, cellular messengers, and transmembrane ionic channels, and may also affect the insect at the genetic level (DNA and RNA) (Senthil-Nathan, 2020). For example, Workman et al. (2020) observed the larvicidal potential of orange essential oil encapsulated in the Saccharomyces cerevisae yeast. The compound is formed mainly by limonene (89.6%), having as secondary constituents myrcene (2.4%), γ-terpinene (1.6%), and 8.2% of other molecules, causing lethality in 90% of larvae treated at the concentration of 18.9 mg/L (or 18.9 ppm). Besides that, the encapsulation process promoted the dispersion in the water, as well as the ingestion of the product by the insects, optimizing its insecticide effect (Workman et al., 2020).
Biopesticides from microorganisms or their metabolites are used to control different species of arthropods with medical and economic relevance, being considered, as well as phytochemicals, substitutes for conventional chemical insecticides. The formulation and constitution of biological pesticides can vary greatly, from virulent molecules isolated from fungi, active in the interior of the target, to infective microorganisms, predators, or parasitic/parasitoid macroorganisms, in addition to possible combinations between both (Chandler et al., 2011; Balog et al., 2017). For example, in the case of the bacteria Bacillus thuringiensis var. israelensis (Bti) and Lysinibacillus sphaericus, both the microorganism itself and its insecticidal crystalline inclusions (ICPs) can be marketed for field and semi-field application, with its formulations focused both on aquatic environments or on terrestrial environments with varying moisture rates (FAO and WHO, 2017; Thakur et al., 2020).
Belonging to the order Rickettsiales, the endosymbiont gram-positive α-proteobacteria of the genus Wolbachia likewise represents a promising perspective for the control of various vectors, including Ae. aegypti. From a specific mechanism in the symbiont-host interaction known as cytoplasmic incompatibility, mosquitoes may be led to the induction of reproductive alterations such as parthenogenesis and feminization, which is acquired from the crossing of transfected males (infected with bacterial strains like wMel) with wild females. The mechanism may further result in a constituent offspring of unviable eggs, infertile individuals, or insects refractory to viral infections (Stouthamer et al., 1999; Caragata et al., 2019; Reyes et al., 2021; Tantowijoyo et al., 2022). The programmed releases of Ae. aegypti males transfected with the bacterium in endemic and high-risk areas is being carried out around the globe, with the objective of gradual replacement of the populations of the vector that are competent for the development of arboviruses by modified refractory populations (Ross et al., 2020).
Similarly, EPFs constitute a group of microorganisms studied for the development of sustainable insecticides for the control of arthropods. The prospection of the insecticide capacity of EPFs began with the Russian microbiologist Elie Metchnikoff, who first described M. anisopliae in 1878 and was the father of cellular immunology, being responsible for pointing out, in 1883, the occurrence of macrophages involved in the immune system of anurans (Ann et al., 1995).
In 1878, after the discovery of the infection that affected pest beetles of the Austrian genus Anisoplia by a fungus, the pathogen was baptized by this scientist as green muscaridine, with the binomial nomenclature Entomopthora anisopliae. Later, in 1880, this fungus was renamed Isaria destructor, and finally, in 1883, by Sorokin, definitively defined as M. anisopliae (Ann et al., 1995; Merien, 2016).
EPFs are present in various orders and clades of organisms (COOKE et al., 1892). There are four groups of parasitic fungi with activity against insects: Phylum Zoopagomycota - Order Entomophtorales, Phylum Mucormycota – Order Mucorales Phylum Oomycota – Orders Saprolegniales and Lagenidiales, and Phylum Ascomycota (Samson et al., 1988; Spatafora et al., 2016). More recent studies describe the occurrence of these microorganisms in 6 different phyla (including Basidiomycota and the removed phylum Deuteromycota) and 12 classes (Abdelghany, 2015). They are characterized by their great complexity in the life cycle and a high degree of infectivity over general or specific hosts. The following topic will revise the advances on controlling main groups of arthropods that are relevant in the economic and sanitary sphere using Metarhizium spp.
Application of EPFs against insect orders
Microbial pesticides currently consist of about 1.3% of the total global pesticides used to control economically relevant insects (Um et al., 2018). Inserted as its constituents, EPF-based pesticides are present in 90% of the applications of new biopesticide formulations (Hajek and St Leger, 1994). The group has between 700 and 1000 species already described (St. Leger and Wang, 2010; Mora et al., 2018). The most studied and used EPF in the development of formulations for biopesticides are Beauveria bassiana (Balls) Vuill (33.9%), Lecanicilium sp. (6.4%), and M. anisopliae (Metch) sensu lato (33.9%) (Faria and Wraight, 2007). The main orders of arthropods targets of bioinsecticides are Lepidoptera, Coleoptera, Diptera, Hemiptera, and Ixodida. Below, we present a review of the fungal bioinsecticides used to control each main order of arthropods.
Lepidoptera: In the last fifteen years, studies focused on pest control of species of the order Lepidoptera by M. anisopliae involved Gelechiidae and Noctuiidae.
Lepidopteran insect management consists of a solid state of art in current biological control literature, since a diversity of economically important plants are highly affected. Some of the latest advances in the prospection and utilization of Metarhizum spp. Against Lepidopteran are summarized in Table 1.
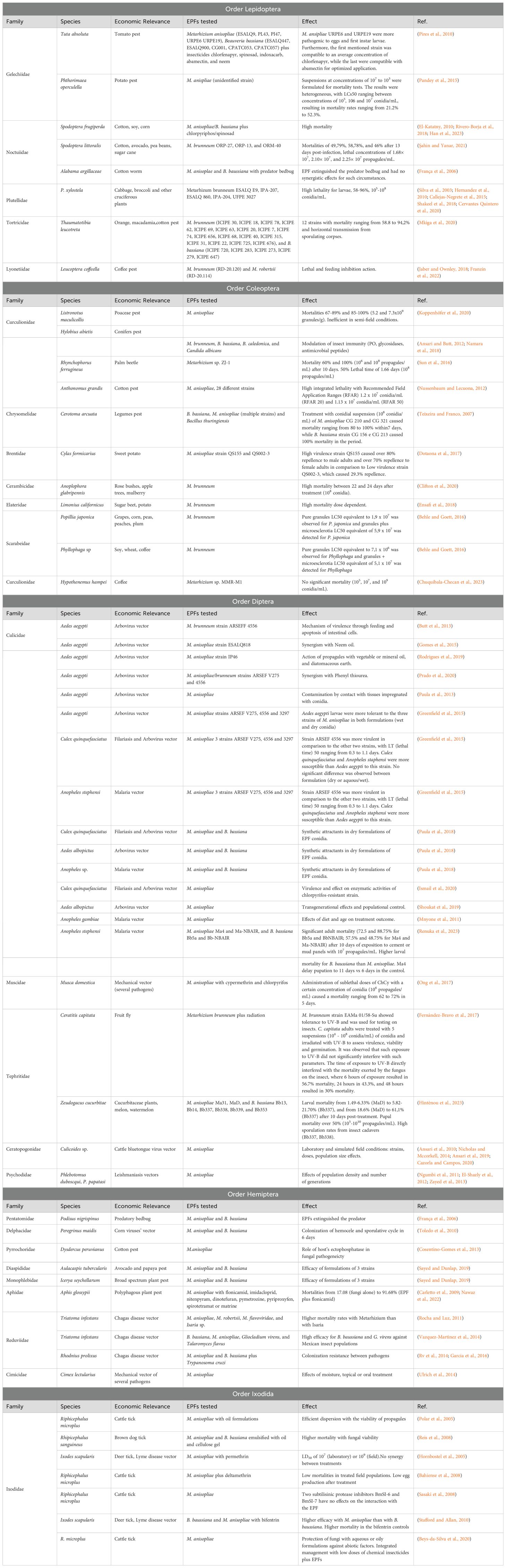
Table 1. Summary of the studies on the application Metarhizum sp. for controlling pests and vectors of different orders of arthropods.
Coleoptera: Studies on the use of M. anisopliae (and other EPFs) in laboratory and semi-field conditions, with integrations to other insecticides or not, against the order Coleoptera, occur mainly using the family Curculionidae as a target
Alternatively, other Coleoptera representatives with the most varied economic relevance are aimed for populational management, such as the families Chrysomelidae Brentidae, Cerambicidae, Elateridae, Scarabeidae, among others, already have a history of investigations for single and integrated management with EPFs in the most varied formulations (see Table 1 for details).
Diptera: In the order Diptera, an important family desired for population control is Culicidae (Suborder Nematocera), a clade representative of the main vector species of arboviruses and parasitic diseases in endemic areas. Muscoid dipteras of Suborder Brachycera, likewise, are potential targets for management.
Other families of sanitary importance, such as Ceratopogonidae and Psychodidae, are, to a lesser extent, studied in the context of the use of M. anisopliae for their population control to reduce the transmission of particular etiological agents. Ceratopogonidae is a family whose medical and veterinary importance comes from the ability of many species of the genus Culicoides to transmit viruses (such as the cattle bluetongue virus: Reoviridae), nematodes of the family Filariidae, among other pathogens (Cazorla and Campos, 2020).
Psychodidae includes important sandfly species that are vectors of Leishmaniasis, the main ones being those of the genus Lutzomyia (New World) and Phlebotomus (Old World). The last advances in control of dipterans using EPFs are summarized in Table 1.
Hemiptera: The Hemiptera order contains numerous species of agricultural importance (cochineals, cicadas, aphids, cotton strainers), and vectors of pathogens (bed bugs and kissing bugs). Studies involving pathogen-host interactions, formulation development in laboratory, semi-field, field, and integrated management were performed for various families, as Pentatomidae, Delphacidae, Pyrrochoridae. Diaspididae, and Aphidae, among others.
Besides the research on hemipteran agricultural pests, the prospection of EPFs has also been conducted for the biological control of vectors, with focus on kissing bugs (Reduviidae: subfamily Triatominae) and bed bugs (Cimicidae).
Studies also consider the control of hemipteran pests and vectors, exploring the scope of application in different environments and physiological and molecular aspects of the pathogen-host interaction, providing more information regarding their management with mycopesticides (Fancelli et al., 2013; Negrete González et al., 2018; Moreno-Salazar et al., 2020, See Table 1).
Acarina - Ixodida: Another arthropod group of sanitary and economic relevance that is a target for populational management in agricultural and urban environments. Ticks are considered the ectoparasites of cattle and wild animals that cause significant morbidity in their hosts due to their vector capacity, responsible for transmitting pathogens such as protozoa, viruses, and bacteria, such as Rickettsia (Pérez de León et al., 2020). Among the most important tick families are Ixodidae (hard ticks) and Argasidae (soft ticks), encompassing key genera such as Rhipicephalus, Amblioma, Ixodes, Ornithodorus, and Otobius (Pérez de León et al., 2020).
The prospection of the alternative control of ticks using EPFs and their formulations has been performed in recent decades (see Table 1). The veterinary and medical relevance of ticks, as well as the problems inherent in chemical pesticides, require more detailed investigations and more profound questions for the future development of sustainable pesticides for employment in agriculture.
Conclusions and perspectives
The increase in human populations entails risks, responsibilities, and changes on a global scale over many habitats. Due to the anthropic expansion, it is expected that by 2050, approximately 300 million hectares of land will be occupied for agricultural purposes (Schmitz et al., 2014). Besides the economic issues, zoonotic diseases have increased as a result of the expansion of human cities. Approximately 60% of emerging infectious diseases in endemic countries are zoonotic, contaminating men and animals, and about 72% of these diseases came from wildlife due to human invasion (Jones et al., 2008; Nyhus, 2016).
Aiming at optimal pest management in the agricultural environment, of vectors in the socio-sanitary scope, and the avoidance of the problems inherent to the use of chemical pesticides, the development of mycopesticides has been prospected since the end of the 19th century, with the first description of an EPF. Since approximately 6% of mycoinsecticides are composed of fungi of the genera Beauveria and Metarhizium (species and variation anisopliae) (Mesquita et al., 2023).
Although EPFs are promising options for developing large-scale insecticides for crop pests and vectorial control, many innate limitations must be considered for the successful establishment of such products.
Difficulties for isolation and characterization of endophytic fungal species comprise a central problem that compromises advances in EPF prospection. Since many species and strains exhibit specific conditions for reproduction, growth, sporulation, and pathogenicity, identification and standardization of tests for efficacy screening may offer obstacles in a first moment. Fortunately, there are media-based, biochemical, and molecular methods capable of promoting a reliable characterization and specification for successive assays using in vitro and in vivo techniques (Tsui et al., 2011; Bamisile et al., 2021).
Natural abiotic conditions associated with geographical locations are also determinants for fungal pathogenicity, virulence, and dispersion potential. Climatic conditions, environmental fragmentation, soil type, mesophilic conditions, and solar incidence were shown to interfere with the physiology of fungal entomopathogens since many clades develop and disperse under average conditions of temperature, radiation, osmolarity, and humidity. According to reports, the probability of discovering new strains of fungal entomopathogens is greater in regions classified as remote and less affected by human activities, with few exceptions explored, in which the tropical conditions for EPF development might be preserved (Zimmermann, 2007; Dong et al., 2016; Bamisile et al., 2021).
Another restriction in implementing EPFs as microbiological agents and mycoinsecticides is the rapid decline of fungal efficacy during the temporal window which comprises recognition, infection and ends in colonization and sporulation. As mentioned, insects and other arthropod hosts can generate immunologic responses. Such physiological reactions, combined with harmful environmental conditions and the fungal specificity of action (as a generalist or specialist fungi), may minimize the control potential of EPFs over a determinate target organism in short periods, thus compromising its impact. Integrated approaches regarding the molecular characterization and genetic engineering of fungal strains, isolation of specialist EPF species, prospection of propagules delivery strategies using artifacts or synthetic products, and formulation with pesticides are viable methods for efficacy optimization of fungal control (Paula et al., 2018; Bamisile et al., 2021; Wang et al., 2021; Wakil et al., 2023).
Due to the low requirements for its effective growth and virulence maintenance, in addition to a simple reproductive cycle and heteroxenic capacity, M. anisopliae has been highly prospected as a candidate for biopesticides aimed at various pests and vectors. The genetic diversity of the species, reflected in the large number of strains and variations, also allows for more detailed investigations, at the molecular level, on pathogen-host interactions, which remain largely unknown.
As previously mentioned, due to new technologies of manipulation and genetic engineering (such as CRISPR Cas9 and electroporation insertions), it will be possible to improve these strains for the overexpression of factors of virulence, resistance to radiation, temperature, and desiccation. Additionally, exogenous genes may be inserted to express toxic proteins against various species of economic and medical importance (Fang et al., 2012; Zhao et al., 2016; Chen et al., 2017). This may make the application of EPFs available in various ways for different purposes, with significant environmental benefits.
Thus, further studies are expected over the next few years to elucidate the molecular mechanisms of EPFs and species of interest, to obtain alternative pesticides. For this, governmental and private investments in research are highly desirable, as well as collaborations between the most varied institutions of health, agriculture, and research on a global scale.
Author contributions
RM: Conceptualization, Data curation, Writing – original draft, Writing – review & editing. TS: Data curation, Visualization, Writing – original draft, Writing – review & editing. DC: Funding acquisition, Resources, Supervision, Writing – original draft, Writing – review & editing. FG: Funding acquisition, Project administration, Resources, Supervision, Writing – original draft, Writing – review & editing.
Funding
The author(s) declare that financial support was received for the research, authorship, and/or publication of this article. RM was a Ph.D. student in the Cellular and Molecular Biology Post-graduation program at the Oswaldo Cruz Institute (CAPES). FG is a research fellow of the agencies CNPq (Produtividade em Pesquisa, grant no. 312305/2022-2) and Faperj (Cientistas do Nosso Estado, grant no. E-26/200.454/2023). This work was also funded by Fundação Oswaldo Cruz (grant.no. IOC-008-FIO-22-2-21) and FAPERJ (INCT-EM, grant no. E-26/200.966/2018; WBI/CONFAP Program, grant no. E-26/201.665/2023).
Conflict of interest
The authors declare that the research was conducted in the absence of any commercial or financial relationships that could be construed as a potential conflict of interest.
The author(s) declared that they were an editorial board member of Frontiers, at the time of submission. This had no impact on the peer review process and the final decision.
Publisher’s note
All claims expressed in this article are solely those of the authors and do not necessarily represent those of their affiliated organizations, or those of the publisher, the editors and the reviewers. Any product that may be evaluated in this article, or claim that may be made by its manufacturer, is not guaranteed or endorsed by the publisher.
Supplementary material
The Supplementary Material for this article can be found online at: https://www.frontiersin.org/articles/10.3389/ffunb.2024.1456964/full#supplementary-material
References
Abdelghany T. M. (2015). Entomopathogenic fungi and their role in biological control. Entomopathogenic Fungi And Their Role In Biol. Control 13, 8012–8023. doi: 10.4172/978-1-63278-065-2-66
Alonso Monge R., Román E., Nombela C., Pla J. (2006). The MAP kinase signal transduction network in Candida albicans. Microbiology 152, 905–912. doi: 10.1099/mic.0.28616-0
Andreis F. C., Schrank A., Thompson C. E. (2019). Molecular evolution of Pr1 proteases depicts ongoing diversification in Metarhizium spp. Mol. Genet. Genomics [Internet]. 294, 901–917. doi: 10.1007/s00438-019-01546-y
Ansari M. A., Butt T. M. (2012). Susceptibility of different developmental stages of large pine weevil Hylobius abietis (Coleoptera: Curculionidae) to entomopathogenic fungi and effect of fungal infection to adult weevils by formulation and application methods. J. Invertebr Pathol. 111, 33–40. doi: 10.1016/j.jip.2012.05.006
Ansari M. A., Carpenter S., Butt T. M. (2010). Susceptibility of Culicoides biting midge larvae to the insect-pathogenic fungus, Metarhizium anisopliae: Prospects for bluetongue vector control. Acta Trop. 113, 1–6. doi: 10.1016/j.actatropica.2009.08.022
Ansari M., Walker M., Dyson P. (2019). Fungi as biocontrol agents of culicoides biting midges, the putative vectors of bluetongue disease. Vector-Borne Zoonotic Dis. 19, 395–399. doi: 10.1089/vbz.2018.2300
Araújo J. P. M., Hughes D. P. (2016). Diversity of entomopathogenic fungi. Which groups conquered the insect body? Adv. Genet. 94, 1–39. doi: 10.1016/bs.adgen.2016.01.001
Bahiense T. C., Fernandes É. K. K., Angelo I. D. C., Perinotto W. M. S., Bittencourt V. R. E. P. (2008). Performance of Metarhizium anisopliae and its combination with deltamethrin against a pyrethroid-resistant strain of Boophilus microplus in a stall test. Ann. N Y Acad. Sci. 1149, 242–245. doi: 10.1196/nyas.2008.1149.issue-1
Balog A., Hartel T., Loxdale H. D., Wilson K. (2017). Differences in the progress of the biopesticide revolution between the EU and other major crop-growing regions. Pest Manag Sci. 73, 2203–2208. doi: 10.1002/ps.2017.73.issue-11
Bamisile B. S., Akutse K. S., Siddiqui J. A., Xu Y. (2021). Model application of entomopathogenic fungi as alternatives to chemical pesticides: prospects, challenges, and insights for next-generation sustainable agriculture. Front. Plant Sci. 12. doi: 10.3389/fpls.2021.741804
Barelli L., Moonjely S., Behie S. W., Bidochka M. J. (2016). Fungi with multifunctional lifestyles: endophytic insect pathogenic fungi. Plant Mol. Biol. 90, 657–664. doi: 10.1007/s11103-015-0413-z
Bate R. (2007). The rise, fall, rise, and imminent fall of DDT. Am. Enterp Inst Public Policy Res. 14, 1-9. doi: 20.500.12592/d8b9wq
Behle R. W., Goett E. J. (2016). Dosage response mortality of Japanese beetle, masked chafer, and June beetle (Coleoptera: Scarabaeidae) adults when exposed to experimental and commercially available granules containing Metarhizium brunneum. J. Econ Entomol 109, 1109–1115. doi: 10.1093/jee/tow080
Beys-da-Silva W. O., Rosa R. L., Berger M., Coutinho-Rodrigues C. J. B., Vainstein M. H., Schrank A., et al. (2020). Updating the application of Metarhizium anisopliae to control cattle tick Rhipicephalus microplus (Acari: Ixodidae). Exp. Parasitol. 208, 107812. doi: 10.1016/j.exppara.2019.107812
Bickel P. E., Tansey J. T., Welte M. A. (2010). PAT proteins, an ancient family of lipid droplet proteins that regulate cellular lipid stores. Biochem. Biophys. Acta 1791, 419–440. doi: 10.1016/j.bbalip.2009.04.002
Bihal R., Al-Khayri J. M., Banu A. N., Kudesia N., Ahmed F. K., Sarkar R., et al. (2023). Entomopathogenic fungi: an eco-friendly synthesis of sustainable nanoparticles and their nanopesticide properties. Microorganisms 11, 1–24. doi: 10.3390/microorganisms11061617
Binggeli O., Neyen C., Poidevin M., Lemaitre B. (2014). Prophenoloxidase activation is required for survival to microbial infections in drosophila. PloS Pathog. 10, e1004067. doi: 10.1371/journal.ppat.1004067
Bischoff J. F., Rehner S. A., Humber R. A. (2009). A multilocus phylogeny of the Metarhizium anisopliae lineage. Mycologia 101, 512–530. doi: 10.3852/07-202
Blackwell M. (2010). Fungal evolution and taxonomy. BioControl 55, 7–16. doi: 10.1007/s10526-009-9243-8
Broetto L., Da Silva W. O. B., Bailão A. M., De Almeida Soares C., Vainstein M. H., Schrank A. (2010). Glyceraldehyde-3-phosphate dehydrogenase of the entomopathogenic fungus Metarhizium anisopliae: Cell-surface localization and role in host adhesion. FEMS Microbiol. Lett. 312, 101–109. doi: 10.1111/j.1574-6968.2010.02103.x
Butt T. M., Coates C. J., Dubovskiy I. M., Ratcliffe N. A. (2016). Entomopathogenic fungi: new insights into host-pathogen interactions. Adv. Genet. 94, 307–364. doi: 10.1016/bs.adgen.2016.01.006
Butt T. M., Greenfield B. P. J., Greig C., Maffeis T. G. G., Taylor J. W. D., Piasecka J., et al. (2013). Metarhizium anisopliae pathogenesis of mosquito larvae: A verdict of accidental death. PloS One. 8, e81686. doi: 10.1371/journal.pone.0081686
Callejas-Negrete O. A., Torres-Guzmán J. C., Padilla-Guerrero I. E., Esquivel-Naranjo U., Padilla-Ballesteros M. F., García-Tapia A., et al. (2015). The Adh1 gene of the fungus Metarhizium anisopliae is expressed during insect colonization and required for full virulence. Microbiol. Res. 172, 57–67. doi: 10.1016/j.micres.2014.11.006
Caragata E. P., Rocha M. N., Pereira T. N., Mansur S. B., Dutra H. L. C., Moreira L. A. (2019). Pathogen blocking in Wolbachia-infected Aedes aEgypti is not affected by zika and dengue virus co-infection. PloS Negl. Trop. Dis. 13, 1–26. doi: 10.1371/journal.pntd.0007443
Carletto J., Lombaert E., Chavigny P., BrÉvault T., Lapchin L., Vanlerberghe-Masutti F. (2009). Ecological specialization of the aphid Aphis gossypii Glover on cultivated host plants. Mol. Ecol. 18, 2198–2212. doi: 10.1111/j.1365-294X.2009.04190.x
Carvalho F. P. (2017). Pesticides, environment, and food safety. Food Energy Secur. 6, 48–60. doi: 10.1002/fes3.2017.6.issue-2
Casida J. E., Quistad G. B. (1998). Golden age of insecticide research: Past, present, or future? Annu. Rev. Entomol 43, 1–16. doi: 10.1146/ento.1998.43.issue-1
Cazorla C. G., Campos R. E. (2020). Ceratopogonidae (Diptera) communities in a protected area threatened by urbanization. Neotrop Entomol. 49, 361-368. doi: 10.1007/s13744-020-00768-9
Cerenius L., Söderhäll K. (2004). The prophenoloxidase-activating system in invertebrates. Immunol. Rev. 198, 116–126. doi: 10.1111/j.0105-2896.2004.00116.x
Cervantes Quintero K. Y., Padilla Guerrero I. E., Torres Guzmán J. C., Villa Martínez B. G., Valencia Félix A., González Hernández G. A. (2020). Members of the nitronate monooxygenase gene family from Metarhizium brunneum are induced during the process of infection to Plutella xylostella. Appl. Microbiol. Biotechnol. 104, 2987–2997. doi: 10.1007/s00253-020-10450-0
Chandler D., Bailey A. S., Mark Tatchell G., Davidson G., Greaves J., Grant W. P. (2011). The development, regulation and use of biopesticides for integrated pest management. Philos. Trans. R Soc. B Biol. Sci. 366, 1987–1998. doi: 10.1098/rstb.2010.0390
Chen J., Lai Y., Wang L., Zhai S., Zou G., Zhou Z., et al. (2017). CRISPR/Cas9-mediated efficient genome editing via blastospore-based transformation in entomopathogenic fungus Beauveria bassiana. Sci. Rep. 8, 1–10. doi: 10.1038/srep45763
Cho Y., Cho S. (2019). Hemocyte-hemocyte adhesion by granulocytes is associated with cellular immunity in the cricket, Gryllus bimaculatus. Sci. Rep. 9, 1–12. doi: 10.1038/s41598-019-54484-5
Chuquibala-Checan B., Torres-De La Cruz M., Leiva S., Hernandez-Diaz E., Rubio K., Goñas M., et al. (2023). In Vitro Biological Activity of Beauveria bassiana, Beauveria Peruviensis, and Metarhizium sp. against Hypothenemus hampei (Coleoptera: Curculionidae). Int. J. Agron. 4982399, 9. doi: 10.1155/2023/4982399
Clifton E. H., Jaronski S. T., Hajek A. E. (2020). Virulence of commercialized fungal entomopathogens against asian longhorned beetle (Coleoptera: cerambycidae). J. Insect Sci. 20, 1. doi: 10.1093/jisesa/ieaa006
Cohen L. B., Lindsay S. A., Xu Y., Lin S. J. H., Wasserman S. A. (2020). The daisho peptides mediate drosophila defense against a subset of filamentous fungi. Front. Immunol. 11, 1–12. doi: 10.3389/fimmu.2020.00009
Cooper D. M., Granville D. J., Lowenberger C. (2009). The insect caspases. Apoptosis 14, 247–256. doi: 10.1007/s10495-009-0322-1
Cosentino-Gomes D., Rocco-MaChado N., Santi L., Broetto L., Vainstein M. H., Meyer-Fernandes J. R., et al. (2013). Inhibition of ecto-phosphatase activity in conidia reduces adhesion and virulence of Metarhizium anisopliae on the host insect Dysdercus Peruvianus. Curr. Microbiol. 66, 467–474. doi: 10.1007/s00284-012-0296-z
De Lima Batista A. C., De Souza Neto F. E., De Souza Paiva W. (2018). Review of fungal chitosan: past, present and perspectives in Brazil. Polimeros 28, 275–283. doi: 10.1590/0104-1428.08316
Dong T., Zhang B., Jiang Y., Hu Q. (2016). Isolation and classification of fungal whitefly entomopathogens from soils of Qinghai-Tibet Plateau and Gansu Corridor in China. PloS One 11, 1–12. doi: 10.1371/journal.pone.0156087
Donzelli B. G. G., Krasnoff S. B. (2016). Molecular genetics of secondary chemistry in Metarhizium fungi. Adv. Genet. 94, 365–436. doi: 10.1016/bs.adgen.2016.01.005
Dotaona R., Wilson B. A. L., Ash G. J., Holloway J., Stevens M. M. (2017). Sweetpotato weevil, Cylas formicarius (Fab.) (Coleoptera: Brentidae) avoids its host plant when a virulent Metarhizium anisopliae isolate is present. J. Invertebr Pathol. 148, 67–72. doi: 10.1016/j.jip.2017.05.010
El-Katatny M. H. (2010). Virulence potential of some fungal isolates and their control-promise against the Egyptian cotton leaf worm, Spodoptera littoralis. Arch. Phytopathol. Plant Prot 43, 332–356. doi: 10.1080/03235400701806278
El-Shazly M. M., Soliman M. M., Zayed A. (2012). Seasonal abundance, number of annual generations, and effect of an entomopathogenic fungus on Phlebotomus papatasi (Diptera: psychodidae). Environ. Entomol. 41, 11–19. doi: 10.1603/EN11109
Ensafi P., Crowder D. W., Esser A. D., Zhao Z., Marshall J. M., Rashed A. (2018). Soil type mediates the effectiveness of biological control against Limonius californicus (Coleoptera: Elateridae). J. Economic Entomology. 111, 2053–2058. doi: 10.1093/jee/toy196
Fancelli M., Dias A. B., Delalibera I. Jr, de Jesus S. C., do Nascimento A. S., Silva Sde O., et al. (2013). Beauveria bassiana strains for biological control of cosmopolites sordidus (Germ.) (Coleoptera: Curculionidae) in plantain. BioMed. Res. Int. 2013, 184756. doi: 10.1155/2013/184756
Fang W., Azimzadeh P., St. Leger R. J. (2012). Strain improvement of fungal insecticides for controlling insect pests and vector-borne diseases. Curr. Opin. Microbiol. 15, 232–238. doi: 10.1016/j.mib.2011.12.012
Fang W., Pava-ripoll M., Wang S., St. Leger R. (2009). Protein kinase A regulates production of virulence determinants by the entomopathogenic fungus, Metarhizium anisopliae. Fungal Genet. Biol. 46, 277–285. doi: 10.1016/j.fgb.2008.12.001
FAO, WHO (2017). International Code of Conduct on Pesticide Management. Guidelines for the registration of microbial, botanical and semiochemical pest control agents for plant protection and public health uses (Rome: FAO and WHO), 86. Available online at: http://www.who.int/whopes/resources/WHO_HTM_NTD_WHOPES_2017.05/en/
Faria M., Mascarin G. M., Butt T., Lopes R. B. (2023). On-farm production of microbial entomopathogens for use in agriculture: Brazil as a case study. Neotrop Entomol. 52, 122–133. doi: 10.1007/s13744-023-01033-5
Faria M., Wraight S. P. (2007). Mycoinsecticides and Mycoacaricides: A comprehensive list with worldwide coverage and international classification of formulation types. Biol. Control. 43, 237–256. doi: 10.1016/j.biocontrol.2007.08.001
Fernández-Bravo M., Flores-León A., Calero-López S., Gutiérrez-Sánchez F., Valverde-García P., Quesada-Moraga E. (2017). UV-B radiation-related effects on conidial inactivation and virulence against Ceratitis capitata (Wiedemann) (Diptera; Tephritidae) of phylloplane and soil Metarhizium sp. strains. J. Invertebr Pathol. 148, 142–151. doi: 10.1016/j.jip.2017.06.012
França Í. W. B., Marques E. J., Torres J. B., Oliveira J. V. (2006). Efeitos de Metarhizium anisopliae (Metsch.) Sorok. e Beauveria bassiana (Bals.) Vuill. sobre o percevejo predador Podisus nigrispinus (Dallas) (Hemiptera: Pentatomidae). Neotrop Entomol 35, 349–356. doi: 10.1590/S1519-566X2006000300009
Franzin M. L., Moreira C. C., da Silva L. N. P., Martins E. F., Fadini M. A. M., Pallini A., et al. (2022). Metarhizium Associated with Coffee Seedling Roots: Positive Effects on Plant Growth and Protection against Leucoptera coffeella. Agric 12, 2030. doi: 10.3390/agriculture12122030
Garcia E. S., Cabral M. M. O., Schaub G. A., Gottlieb O. R., Azambuja P. (2000). Effects of lignoids on a hematophagous bug, Rhodnius prolixus: Feeding, ecdysis and diuresis. Phytochemistry 55, 611–616. doi: 10.1016/S0031-9422(00)00228-4
Garcia A. R. M., Rocha A de P., Moreira C. C., Rocha S. L., Guarneri A. A., Elliot S. L. (2016). Screening of fungi for biological control of a triatomine vector of chagas disease: temperature and trypanosome infection as factors. PloS Negl. Trop. Dis. 10, 1–14. doi: 10.1371/journal.pntd.0005128
Gomes S. A., Paula A. R., Ribeiro A., Moraes C. O. P., Santos J. W. A. B., Silva C. P., et al. (2015). Neem oil increases the efficiency of the entomopathogenic fungus Metarhizium anisopliae for the control of Aedes aEgypti (Diptera: Culicidae) larvae. Parasites Vectors 8, 1–8. doi: 10.1186/s13071-015-1280-9
Greenfield B. P. J., Peace A., Evans H., Dudley E., Ansari M. A., Butt T. M. (2015). Identification of Metarhizium strains highly efficacious against Aedes, Anopheles and Culex larvae. Biocontrol Sci. Technol. 25, 487–502. doi: 10.1080/09583157.2014.989813
Hajek A. E., St Leger R. J. (1994). Interactions between fungal pathogens and insect hosts. Annu. Rev. Entomol 39, 293–322. doi: 10.1146/annurev.en.39.010194.001453
Hallsworth J. E., Magan N. (1996). Culture Age, temperature, and pH affect the polyol and trehalose contents of fungal propagules. Appl. Environ. Microbiol. 62, 2435–2442. doi: 10.1128/aem.62.7.2435-2442.1996
Han H., Chen B., Xu H., Qin Y., Wang G., Lv Z., et al. (2023). Control of Spodoptera frugiperda on Fresh Corn via Pesticide Application before Transplanting. Agric 13, 1–13. doi: 10.3390/agriculture13020342
Hawksworth D. L., Lücking R. (2017). Fungal diversity revisited: 2.2 to 3.8 million species. Microbiol. Spectr. 5, 52. doi: 10.1128/microbiolspec.FUNK-0052-2016
Hemingway J., Ranson H. (2000). Insecticide resistance in insect vectors of human disease. Annu. Rev. Entomol. 45, 371–391. doi: 10.1146/annurev.ento.45.1.371
Hernandez C. E. M., Guerrero I. E. P., Hernandez G. A. G., Solis E. S., Guzman J. C. T. (2010). Catalase over expression reduces the germination time and increases the pathogenicity of the fungus Metarhizium anisopliae. Appl. Microbiol. Biotechnol. 87, 1033–1044. doi: 10.1007/s00253-010-2517-3
Hintènou M. V., Omoloye A. A., Douro Kpindou O. K., Karlsson M. F., Djouaka R., Bokonon-Ganta A. H., et al. (2023). Pathogenicity of Beauveria bassiana (Balsamo-Crivelli) and Metarhizium anisopliae (Metschnikoff) isolates against life stages of Zeugodacus cucurbitae (Coquillett) (Diptera: Tephritidae). Egypt J. Biol. Pest Control 33, 45. doi: 10.1186/s41938-023-00693-0
Hornbostel V. L., Zhioua E., Benjamin M. A., Ginsberg H. S., Ostfeld R. S. (2005). Pathogenicity of Metarhizium anisopliae (Deuteromycetes) and permethrin to Ixodes scapularis (Acari: Ixodidae) nymphs. Exp. Appl. Acarol. 35, 301–316. doi: 10.1007/s10493-004-5437-z
Huang W., Shang Y., Chen P., Gao Q., Wang C. (2015). MrpacC regulates sporulation, insect cuticle penetration and immune evasion in Metarhizium robertsii. Environ. Microbiol. 17, 994–1008. doi: 10.1111/emi.2015.17.issue-4
IRAC (2023). “IRAC mode of Action Classification Scheme,” in Insectic Resist Action Comm 9.1, 1–23. Available online at: https://vegetableipmupdates.arizona.edu/sites/default/files/2021-09/190320_irac_moa-classification_v9.1_1feb19.pdf.
Ismail H. M., Freed S., Naeem A., Malik S., Ali N. (2020). The effect of entomopathogenic fungi on enzymatic activity in chlorpyrifos-resistant mosquitoes, Culex quinquefasciatus (Diptera: culicidae). J. Med. Entomol. 57, 204–213. doi: 10.1093/jme/tjz143
Isman M. B. (2020). Botanical insecticides in the twenty-first century—Fulfilling their promise? Annu. Rev. Entomol 65, 233–249. doi: 10.1146/annurev-ento-011019-025010
Iwanicki N. S., Pereira A. A., Botelho A. B. R. Z., Rezende J. M., Moral R de A., Zucchi M. I., et al. (2019). Monitoring of the field application of Metarhizium anisopliae in Brazil revealed high molecular diversity of Metarhizium spp in insects, soil and sugarcane roots. Sci. Rep. 9, 1–12. doi: 10.1038/s41598-019-38594-8
Jaber L. R., Ownley B. H. (2018). Can we use entomopathogenic fungi as endophytes for dual biological control of insect pests and plant pathogens? Biol. Control 116, 36–45. doi: 10.1016/j.biocontrol.2017.01.018
Jones K. E., Patel N. G., Levy M. A., Storeygard A., Balk D., Gittleman J. L., et al. (2008). Global trends in emerging infectious diseases. Nature 451, 990–993. doi: 10.1038/nature06536
Juarez M. P., Forlani L., Pedrini N. (2011). Contribution of the horizontal transmission of the entomopathogenic fungus Beauveria bassiana to the overall performance of a fungal powder formulation against Triatoma infestans. Res. Rep. Trop. Med. 135, 135-140. doi: 10.2147/RRTM.S22961
Kasai S., Komagata O., Itokawa K., Shono T., Ng L. C., Kobayashi M., et al. (2014). Mechanisms of pyrethroid resistance in the dengue mosquito vector, Aedes aEgypti: target site insensitivity, penetration, and metabolism. PloS Negl. Trop. Dis. 8, e2948. doi: 10.1371/journal.pntd.0002948
Kaufmann S. H., Hengartner M. O. (2001). Programmed cell death: Alive and well in the new millennium. Trends Cell Biol. 11, 526–534. doi: 10.1016/S0962-8924(01)02173-0
Koppenhöfer A. M., Wu S., Kostromytska O. S. (2020). Microsclerotial Granular Formulation of the Entomopathogenic Fungus Metarhizium brunneum and Its Combinations with Hydrogel and Imidacloprid against the Annual Bluegrass Weevil (Coleoptera: Curculionidae). J. Econ Entomol. 113, 1118–1128. doi: 10.1093/jee/toaa052
Lackie A. M. (1988). Immune mechanisms in insects. Parasitol. Today 4, 98–105. doi: 10.1016/0169-4758(88)90035-X
Lemaitre B., Hoffmann J. (2007). The host defense of drosophila melanogaster. Annu. Rev. Immunol. 25, 697–743. doi: 10.1146/annurev.immunol.25.022106.141615
Leyria J., Guarneri A. A., Lorenzo M. G., Nouzova M., Noriega F. G., Benrabaa S. A. M., et al. (2023). Effects of mating on female reproductive physiology in the insect model, Rhodnius prolixus, a vector of the causative parasite of Chagas disease. PloS Negl. Trop. Dis. 17, 1–17. doi: 10.1371/journal.pntd.0011640
Ligoxygakis P., Pelte N., Hoffmann J. A., Reichhart J. M. (2002). Activation of Drosophila toll during fungal infection by a blood serine protease. Sci. (80-). 297, 114–116. doi: 10.1126/science.1072391
Lu A., Zhang Q., Zhang J., Yang B., Wu K., Xie W., et al. (2014). Insect prophenoloxidase: The view beyond immunity. Front. Physiol. 5, 1–16. doi: 10.3389/fphys.2014.00252
Merien F. (2016). A journey with elie metchnikoff: from innate cell mechanisms in infectious diseases to quantum biology. Front. Public Heal 4. doi: 10.3389/fpubh.2016.00125
Mesquita E., Hu S., Lima T. B., Golo P. S., Bidochka M. J. (2023). Utilization of Metarhizium as an insect biocontrol agent and a plant bioinoculant with special reference to Brazil. Front. Fungal Biol. 4, 1–10. doi: 10.3389/ffunb.2023.1276287
Miura S., Gan J. W., Brzostowski J., Parisi M. J., Schultz C. J., Londos C., et al. (2002). Functional conservation for lipid storage droplet association among perilipin, ADRP, and TIP47 (PAT)-related proteins in mammals, Drosophila, and Dictyostelium. J. Biol. Chem. 277, 32253–32257. doi: 10.1074/jbc.M204410200
Mkiga A. M., Mohamed S. A., Du Plessis H., Khamis F. M., Akutse K. S., Ekesi S., et al. (2020). Metarhizium anisopliae and Beauveria bassiana: Pathogenicity, Horizontal Transmission, and Their Effects on Reproductive Potential of Thaumatotibia leucotreta (Lepidoptera: Tortricidae). J. Econ Entomol. 113, 660–668. doi: 10.1093/jee/toz342
Mnyone L. L., Kirby M. J., Mpingwa M. W., Lwetoijera D. W., Knols B. G. J., Takken W., et al. (2011). Infection of Anopheles Gambiae mosquitoes with entomopathogenic fungi: Effect of host age and blood-feeding status. Parasitol. Res. 108, 317–322. doi: 10.1007/s00436-010-2064-y
Mora M. A. E., Castilho A. M. C., Fraga M. E. (2018). Classification and infection mechanism of entomopathogenic fungi. Arq Inst Biol. (Sao Paulo). 84, 1–10. doi: 10.1590/1808-1657000552015
Moreno-Salazar R., Sánchez-García I., Chan-Cupul W., Ruiz-Sánchez E., Hernández-Ortega H. A., Pineda-Lucatero J., et al. (2020). Plant growth, foliar nutritional content and fruit yield of Capsicum chinense biofertilized with Purpureocillium lilacinum under greenhouse conditions. Sci. Hortic. (Amsterdam) 261, 108950. doi: 10.1016/j.scienta.2019.108950
Namara L. M., Griffin C. T., Fitzpatrick D., Kavanagh K., Carolan J. C. (2018). The effect of entomopathogenic fungal culture filtrate on the immune response and haemolymph proteome of the large pine weevil, Hylobius abietis. Insect Biochem. Mol. Biol. [Internet] 101, 1–13. doi: 10.1016/j.ibmb.2018.07.001
Nawaz A., Razzaq F., Razzaq A., Gogi M. D., Fernández-Grandon G. M., Tayib M., et al. (2022). Compatibility and synergistic interactions of fungi, Metarhizium anisopliae, and insecticide combinations against the cotton aphid, Aphis gossypii Glover (Hemiptera: Aphididae). Sci. Rep. 12, 1–10. doi: 10.1038/s41598-022-08841-6
Negrete González D., Ávalos Chávez M. A., Lezama Gutiérrez R., Chan Cupul W., Molina Ochoa J., Galindo Velasco E. (2018). Suitability of Cordyceps bassiana and Metarhizium anisopliae for biological control of Cosmopolites sordidus (Germar) (Coleoptera: Curculionidae) in an organic Mexican banana plantation: laboratory and field trials. J. Plant Dis. Prot. 125, 73–81. doi: 10.1007/s41348-017-0126-4
Ngumbi P. M., Irungu L. W., Ndegwa P. N., Maniania N. K. (2011). Pathogenicity of Metarhizium anisopliae (Metch) sorok and Beauveria bassiana (Bals) Vuill to adult Phlebotomus duboscqi (Neveu-Lemaire) in the laboratory. J. Vector Borne Dis. 48, 37–40. Available online at: https://pubmed.ncbi.nlm.nih.gov/21406735/
Nicholas A. H., Mccorkell B. (2014). Evaluation of Metarhizium anisopliae for the control of Culicoides brevitarsis Kieffer (Diptera: Ceratopogonidae), the principal vector of bluetongue virus in Australia. J. Vector Ecol. 39, 213–218. doi: 10.1111/j.1948-7134.2014.12089.x
Nicolopoulou-Stamati P., Maipas S., Kotampasi C., Stamatis P., Hens L. (2016). Chemical pesticides and human health: the urgent need for a new concept in agriculture. Front. Public Heal 4, 1–8. doi: 10.3389/fpubh.2016.00148
Nussenbaum A. L., Lecuona R. E. (2012). Selection of Beauveria bassiana sensu lato and Metarhizium anisopliae sensu lato isolates as microbial control agents against the boll weevil (Anthonomus grandis) in Argentina. J. Invertebr Pathol. 110, 1–7. doi: 10.1016/j.jip.2012.01.010
Nyhus P. J. (2016). Human–wildlife conflict and coexistence. Annu. Rev. Environ. Resour. 41, 143–171. doi: 10.1146/annurev-environ-110615-085634
Oberemok V. V., Laikova K. V., Gninenko Y. I., Zaitsev A. S., Nyadar P. M., Adeyemi T. A. (2015). A short history of insecticides. J. Plant Prot Res. 55, 221–226. doi: 10.1515/jppr-2015-0033
Ong S. Q., Ahmad H., Ab Majid A. H., Jaal Z. (2017). Interaction between Metarhizium anisopliae (Met.) and the insecticides used for controlling house fly (Diptera: muscidae) in poultry farm of Malaysia. J. Med. Entomol 54, 1626–1632. doi: 10.1093/jme/tjx128
Pandey S., Sporleder M., Chhetry Y., Giri Y. P., Kroschel J. (2015). Efficacy of Metarhizium anisopliue (NLetsch.) Sorokin against the Potato Tuber Moth, Phthorimaea operculellu (Zeller) in Consumable Potato, under Laboratory Conditions. Nepal Hortic. 10, 47–54. Available online at: https://horticulturenepal.org/uploads/main_attachment/1627450826_Full%20Journal%20Volume%2010-55-62.pdf
Paula A. R., Carolino A. T., Silva C. P., Pereira C. R., Samuels R. I. (2013). Testing fungus impregnated cloths for the control of adult Aedes aEgypti under natural conditions. Parasites Vectors. 6, 1–6. doi: 10.1186/1756-3305-6-256
Paula A. R., Silva L. E. I., Ribeiro A., Butt T. M., Silva C. P., Samuels R. I. (2018). Improving the delivery and efficiency of fungus-impregnated cloths for control of adult Aedes aEgypti using a synthetic attractive lure. Parasites Vectors. 11, 1–9. doi: 10.1186/s13071-018-2871-z
Peñalva M. A., Tilburn J., Bignell E., Arst H. N. (2008). Ambient pH gene regulation in fungi: making connections. Trends Microbiol. 16, 291–300. doi: 10.1016/j.tim.2008.03.006
Pérez de León A. A., Mitchell R. D., Watson D. W. (2020). Ectoparasites of cattle. Vet. Clin. North Am. - Food Anim. Pract. 36, 173–185. doi: 10.1016/j.cvfa.2019.12.004
Pires L. M., Marques E. J., de O. J. V., Alves S. B. (2010). Seleção de isolados de fungos entomopatogênicos para o controle de Tuta absoluta (Meyrick) (Lepidoptera: Gelechiidae) e sua compatibilidade com alguns inseticidas usados na cultura do tomateiro. Neotrop Entomol. 39, 977–984. doi: 10.1590/S1519-566X2010000600020
Polar P., Kairo M. T. K., Moore D., Pegram R., John S. A. (2005). Comparison of water, oils and emulsifiable adjuvant oils as formulating agents for Metarhizium anisopliae for use in control of Boophilus microplus. Mycopathologia 160, 151–157. doi: 10.1007/s11046-005-0120-4
Pondeville E., Puchot N., Parvy J. P., Carissimo G., Poidevin M., Waterhouse R. M., et al. (2020). Hemocyte-targeted gene expression in the female malaria mosquito using the hemolectin promoter from Drosophila. Insect Biochem. Mol. Biol. 120, 103339. doi: 10.1016/j.ibmb.2020.103339
Prado R., Macedo-Salles P. A., Duprat R. C., Baptista A. R. S., Feder D., Lima J. B. P., et al. (2020). Action of Metarhizium brunneum (Hypocreales: Clavicipitaceae) Against Organophosphate- and Pyrethroid-Resistant Aedes aEgypti (Diptera: Culicidae) and the Synergistic Effects of Phenylthiourea. J. Med. Entomol. 57, 454–462. doi: 10.1093/jme/tjz21410.1093/jme/tjz161
Puinean A. M., Foster S. P., Oliphant L., Denholm I., Field L. M., Millar N. S., et al. (2010). Amplification of a cytochrome P450 gene is associated with resistance to neonicotinoid insecticides in the aphid Myzus persicae. PloS Genet. 6, 1–11. doi: 10.1371/journal.pgen.1000999
Ratcliffe N. A., Gagen S. J. (1977). Studies on the in vivo cellular reactions of insects: An ultrastructural analysis of nodule formation in Galleria mellonella. Tissue Cell 9, 73–85. doi: 10.1016/0040-8166(77)90050-7
Reis R. C. S., Fernandes É. K. K., Bittencourt V. R. E. P. (2008). Fungal formulations to control Rhipicephalus sanguineus engorged females. Ann. N Y Acad. Sci. 1149, 239–241. doi: 10.1196/nyas.2008.1149.issue-1
Renuka S., Vani H. C., Alex E. (2023). Entomopathogenic fungi as a potential management tool for the control of urban malaria vector, Anopheles stephensi (Diptera: culicidae). J. Fungi. 9, 223. doi: 10.3390/jof9020223
Reyes J. I. L., Suzuki Y., Carvajal T., Muñoz M. N. M., Watanabe K. (2021). Intracellular interactions between arboviruses and wolbachia in Aedes aEgypti. Front. Cell Infect. Microbiol. 11, 1–15. doi: 10.3389/fcimb.2021.690087
Rivero-Borja M., Franco A. G., Rodriguez-Levya E., Santillán-Ortega C., Panduro A. P. (2018). Interaction of Beauveria bassiana and Metarhizium anisopliae with chlorpyrifos ethyl and spinosad in Spodoptera frugiperda larvae β -lactam. Pest Manag Sci. 52. doi: 10.1002/ps.2018.74.issue-9
Rocha L. F. N., Luz C. (2011). Activity of Metarhizium spp. and Isaria spp. from the Central Brazilian Cerrado against Triatoma infestans nymphs. Trans. R Soc. Trop. Med. Hyg 105, 417–419. doi: 10.1016/j.trstmh.2011.04.012
Rodrigues J., Borges P. R., Fernandes É. K. K., Luz C. (2019). Activity of additives and their effect in formulations of Metarhizium anisopliae s.l. IP 46 against Aedes aEgypti adults and on post mortem conidiogenesis. Acta Trop. 193, 192–198. doi: 10.1016/j.actatropica.2019.03.002
Ross P. A., Callahan A. G., Yang Q., Jasper M., Arif M. A. K., Afizah A. N., et al. (2020). An elusive endosymbiont: Does Wolbachia occur naturally in Aedes aEgypti? Ecol. Evol. 10, 1581–1591. doi: 10.1002/ece3.6012
Rv M., Anna S., Diaz-albiter H., Aguiar-martins K., Al Salem W. S., Cavalcante R. R., et al. (2014). Colonisation resistance in the sand fly gut: Leishmania protects Lutzomyia longipalpis from bacterial infection. Parasit Vectors 7, 10. doi: 10.1186/1756-3305-7-329
Şahin F., Yanar Y. (2021). Pathogenicity of some local entomopathogenic fungus isolates on the cotton leafworm larvae, Spodoptera littoralis (Boisd.) (Lepidoptera: Noctuidae). Egypt J. Biol. Pest Control [Internet]. 31, 150. doi: 10.1186/s41938-021-00494-3
Samson R. A., Evans H. C., Latgé J.-P. (1988). Atlas of entomopathogenic fungi (Berlin, Heidelberg: Springer), 1–199.
Sasaki S. D., de Lima C. A., Lovato D. V., Juliano M. A., Torquato R. J. S., Tanaka A. S. (2008). BmSI-7, a novel subtilisin inhibitor from Boophilus microplus, with activity toward Pr1 proteases from the fungus Metarhizium anisopliae. Exp. Parasitol. 118, 214–220. doi: 10.1016/j.exppara.2007.08.003
Sayed A. M. M., Dunlap C. A. (2019). Virulence of Some Entomopathogenic Fungi Isolates of Beauveria bassiana (Hypocreales: Cordycipitaceae) and Metarhizium anisopliae (Hypocreales: Clavicipitaceae) to Aulacaspis tubercularis (Hemiptera: Diaspididae)and Icerya seychellarum (Hemiptera: Monophl. J. Econ Entomol. 112, 2584–2596. doi: 10.1093/jee/toz187
Schmitz C., van Meijl H., Kyle P., Nelson G. C., Fujimori S., Gurgel A., et al. (2014). Land-use change trajectories up to 2050: Insights from a global agro-economic model comparison. Agric. Econ (United Kingdom). 45, 69–84. doi: 10.1111/agec.2014.45.issue-1
Senthil-Nathan S. (2020). A review of resistance mechanisms of synthetic insecticides and botanicals, phytochemicals, and essential oils as alternative larvicidal agents against mosquitoes. Front. Physiol. 10, 1–21. doi: 10.3389/fphys.2019.01591
Sevim A., Donzelli B. G. G., Wu D., Demirbag Z., Gibson D. M., Turgeon B. G. (2012). Hydrophobin genes of the entomopathogenic fungus, Metarhizium brunneum, are differentially expressed and corresponding mutants are decreased in virulence. Curr. Genet. 58, 79–92. doi: 10.1007/s00294-012-0366-6
Shakeel M., Xu X., Xu J., Li S., Yu J., Zhou X., et al. (2018). Genome-wide identification of Destruxin A-responsive immunity-related MicroRNAs in diamondback moth, Plutella xylostella. Front. Immunol. 9, 2–11. doi: 10.3389/fimmu.2018.00185
Shoukat R. F., Hassan B., Shakeel M., Zafar J., Li S., Freed S., et al. (2019). Pathogenicity and Transgenerational Effects of Metarhizium anisopliae on the Demographic Parameters of Aedes albopictus (Culicidae: Diptera). J. Med. Entomol. 57, 677-685. doi: 10.1093/jme/tjz236
Silva V. C. A., Barros R., Marques E. J., Torres J. B. (2003). Suscetibilidade de Plutella xylostella (L.) (Lepidoptera: Plutellidae) aos fungos Beauveria bassiana (Bals.) Vuill. e Metarhizium anisopliae (Metsch.) sorok. Neotrop Entomol 32, 653–658. doi: 10.1590/S1519-566X2003000400016
Skinner M., Parker B. L., Kim J. S. (2014). “Role of Entomopathogenic Fungi in Integrated Pest Management,” in Integrated Pest Management: Current Concepts and Ecological Perspective In: ed Abrol D. P. (Amsterdam: Elsevier Inc), 169–191. doi: 10.1016/B978-0-12-398529-3.00011-7
Small C. L. N., Bidochka M. J. (2005). Up-regulation of Pr1, a subtilisin-like protease, during conidiation in the insect pathogen Metarhizium anisopliae. Mycol Res. 109, 307–313. doi: 10.1017/S0953756204001856
Spatafora J. W., Chang Y., Benny G. L., Lazarus K., Smith M. E., Berbee M. L., et al. (2016). A phylum-level phylogenetic classification of zygomycete fungi based on genome-scale data. Mycologia 108, 1028–1046. doi: 10.3852/16-042
Stafford K. C., Allan S. A. (2010). Field Applications of Entomopathogenic Fungi Beauveria bassiana and Metarhizium anisopliae F52 (Hypocreales: Clavicipitaceae) for the Control of Ixodes scapularis (Acari: Ixodidae). J. Med. Entomol. 47, 1107–1115. doi: 10.1603/ME10019
St. Leger R. J., Wang C. (2010). Genetic engineering of fungal biocontrol agents to achieve greater efficacy against insect pests. Appl. Microbiol. Biotechnol. 85, 901–907. doi: 10.1007/s00253-009-2306-z
Stouthamer R., Breeuwer J. A. J., Hurst G. D. D. (1999). Wolbachia pipientis : Microbial manipulator of arthropod reproduction. Annu. Rev. Microbiol. 53, 71–102. doi: 10.1146/annurev.micro.53.1.71
Sun X., Yan W., Qin W., Zhang J., Niu X., Ma G., et al. (2016). Screening of tropical isolates of Metarhizium anisopliae for virulence to the red palm weevil Rhynchophorus ferrugineus Olivier (Coleoptera: Curculionidae) (Springerplus). 5, 1100. doi: 10.1186/s40064-016-2780-6
Tantowijoyo W., Tanamas S. K., Nurhayati I., Setyawan S., Budiwati N., Fitriana I., et al. (2022). Aedes aEgypti abundance and insecticide resistance profiles in the Applying Wolbachia to Eliminate Dengue trial. PloS Negl. Trop. Dis. 16, 1–16. doi: 10.1371/journal.pntd.0010284
Tatebayashi K., Tanaka K., Yang H. Y., Yamamoto K., Matsushita Y., Tomida T., et al. (2007). Transmembrane mucins Hkr1 and Msb2 are putative osmosensors in the SHO1 branch of yeast HOG pathway. EMBO J. 26, 3521–3533. doi: 10.1038/sj.emboj.7601796
Tauszig-Delamasure S., Bilak H., Capovilla M., Hoffmann J. A., Imler J. L. (2002). Drosophila MyD88 is required for the response to fungal and Gram-positive bacterial infections. Nat. Immunol. 3, 91–97. doi: 10.1038/ni747
Teixeira M. L. F., Franco A. A. (2007). Susceptibility of Cerotoma arcuata Olivier (Coleoptera: Chrysomelidae) larvae to Beauveria bassiana (Bals.) Vuillemin, Metarhizium anisopliae (Metsch.) Sorokin and Bacillus thuringiensis Berliner. Cienc Rural 37, 19–25. doi: 10.1590/S0103-84782007000100004
Thakur N., Kaur S., Tomar P., Thakur S., Yadav A. N. (2020). “Microbial biopesticides: Current status and advancement for sustainable agriculture and environment,” in New and Future Developments in Microbial Biotechnology and Bioengineering: Trends of Microbial Biotechnology for Sustainable Agriculture and Biomedicine Systems: Diversity and Functional Perspectives Rastegari A. A., Yadav A. N., Yadav N. (Amsterdam: Elsevier Inc), 243–282. doi: 10.1016/B978-0-12-820526-6.00016-6
Toledo A. V., de Remes Lenicov A. M. M., López Lastra C. C. (2010). Histopathology Caused by the Entomopathogenic Fungi, Beauveria bassiana and Metarhizium anisopliae, in the Adult Planthopper, Peregrinus maidis, a Maize Virus Vector. J. Insect Sci. 10, 1–10. doi: 10.1673/031.010.3501
Tsui C. K.M., Woodhall J., CHen W., Lévesque A., Lau A., Cor D. (2011). Molecular techniques for pathogen identification and fungus detection in the environment. IMA Fungus. 2, 177–189. doi: 10.5598/imafungus.2011.02.02.09
Ulrich K. R., Feldlaufer M. F., Kramer M., St. Leger R. J. (2014). Exposure of Bed Bugs to Metarhizium anisopliae at Different Humidities. J. Econ Entomol. 107, 2190–2195. doi: 10.1603/EC14294
Um M., Galadima I. B., Gambo F. M., Zakaria D. (2018). A review on the use of entomopathogenic fungi in the management of insect pests of field crops. J. Entomol Zool Stud. 6, 27–32. Available online at: https://www.entomoljournal.com/archives/2018/vol6issue1/PartA/5-5-367-216.pdf
Vazquez-Martinez M. G., Cirerol-Cruz B. E., Torres-Estrada J. L., Lopez M. H. R. (2014). Potential for entomopathogenic fungi to control Triatoma dimidiata (Hemiptera: Reduviidae), a vector of chagas disease in Mexico. Rev. Soc. Bras. Med. Trop. 47, 716–722. doi: 10.1590/0037-8682-0193-2014
Verma N. S., Kuldeep D. K., Chouhan M., Prajapati R., Singh S. K. (2023). A review on eco-friendly pesticides and their rising importance in sustainable plant protection practices. Int. J. Plant Soil Sci. 35, 200–214. doi: 10.9734/ijpss/2023/v35i224126
Vivekanandhan P., Swathy K., Murugan A. C., Krutmuang P. (2022). Insecticidal Efficacy of Metarhizium anisopliae Derived Chemical Constituents against Disease-Vector Mosquitoes. J. Fungi. 8, 300. doi: 10.3390/jof8030300
Wakil W., Kavallieratos N. G., Eleftheriadou N., Riasat T., Ghazanfar M. U., Rasool K. G., et al. (2023). The Potential of Two Entomopathogenic Fungi and Enhanced Diatomaceous Earth Mixed with Abamectin: A Comprehensive Study on Mortality, Progeny Production, Application Method, and Surface Application against Tribolium castaneum. Pathog. 12, 773. doi: 10.3390/pathogens12060773
Walderdorff L., Laval-Gilly P., Wechtler L., Bonnefoy A., Falla-Angel J. (2019). Phagocytic activity of human macrophages and Drosophila hemocytes after exposure to the neonicotinoid imidacloprid. Pestic Biochem. Physiol. 160, 95–101. doi: 10.1016/j.pestbp.2019.07.007
Wallen R. M., Perlin M. H. (2018). An overview of the function and maintenance of sexual reproduction in dikaryotic fungi. Front. Microbiol. 9, 1–24. doi: 10.3389/fmicb.2018.00503
Wang C., Duan Z., St. Leger R. J. (2008). MOS1 osmosensor of Metarhizium anisopliae is required for adaptation to insect host hemolymph. Eukaryot Cell. 7, 302–309. doi: 10.1128/EC.00310-07
Wang H., Peng H., Li W., Cheng P., Gong M. (2021). The toxins of beauveria bassiana and the strategies to improve their virulence to insects. Front. Microbiol. 12, 1–11. doi: 10.3389/fmicb.2021.705343
Wang C., St. Leger R. J. (2006). A collagenous protective coat enables Metarhizium anisopliae to evade insect immune responses. Proc. Natl. Acad. Sci. U S A. 103, 6647–6652. doi: 10.1073/pnas.0601951103
Wang C., St. Leger R. J. (2007). The Metarhizium anisopliae perilipin homolog MPL1 regulates lipid metabolism, appressorial turgor pressure, and virulence. J. Biol. Chem. 282, 21110–21115. doi: 10.1074/jbc.M609592200
Wang J. B., St. Leger R. J., Wang C. (2016). Advances in genomics of entomopathogenic fungi. Adv. Genet. 94, 67–105. doi: 10.1016/bs.adgen.2016.01.002
Wilson A. L., Courtenay O., Kelly-Hope L. A., Scott T. W., Takken W., Torr S. J., et al. (2020). The importance of vector control for the control and elimination of vector-borne diseases. PloS Negl. Trop. Dis. 14, e0007831. doi: 10.1371/journal.pntd.0007831
Workman M. J., Gomes B., Weng J. L., Ista L. K., Jesus C. P., David M. R., et al. (2020). Yeast-encapsulated essential oils: A new perspective as an environmentally friendly larvicide. Parasites Vectors 13, 1–9. doi: 10.1186/s13071-019-3870-4
Wösten H. A. B. (2001). Hydrophobins: multipurpose proteins. Annu. Rev. Microbiol. 55, 625–646. doi: 10.1146/annurev.micro.55.1.625
Wu Q., Patočka J., Kuča K. (2018). Insect antimicrobial peptides, a mini review. Toxins (Basel). 10, 1–17. doi: 10.3390/toxins10110461
Wyrebek M., Bidochka M. J. (2013). Variability in the insect and plant adhesins, mad1 and mad2, within the fungal genus Metarhizium suggest plant adaptation as an evolutionary force. PloS One 8, 1–7. doi: 10.1371/journal.pone.0059357
Xie T., Wang Y., Yu D., Zhang Q., Zhang T., Wang Z., et al. (2019). MrSVP, a secreted virulence-associated protein, contributes to thermotolerance and virulence of the entomopathogenic fungus Metarhizium robertsii. BMC Microbiol. 19, 1–10. doi: 10.1186/s12866-019-1396-8
Yang L., Yang L., Wang X., Peng C., Chen X., Wei W., et al. (2023). Toll and IMD immune pathways are important antifungal defense components in a pupal parasitoid, pteromalus puparum. Int. J. Mol. Sci. 24, 14088. doi: 10.3390/ijms241814088
Zalucki M. P., Furlong M. J. (2017). Behavior as a mechanism of insecticide resistance: evaluation of the evidence. Curr. Opin. Insect Sci. [Internet]. 21, 19–25. doi: 10.1016/j.cois.2017.05.006
Zayed A., Soliman M. M., El-shazly M. M. (2013). Infectivity of Metarhizium anisopliae (Hypocreales : Clavicipitaceae) to Phlebotomus papatasi (Diptera : Psychodidae) Under Laboratory Conditions. J. Med. Entomol. 50, 796–803. doi: 10.1603/ME12244
Zhao H., Lovett B., Fang W. (2016). “Genetically Engineering Entomopathogenic Fungi,” in Advances in Genetics (United States: Academic Press) 94, 137–163. doi: 10.1016/bs.adgen.2015.11.001
Zimmermann G. Z., Papierok B., Glare T. (1995). Elias metschnikoff, elie metchnikoff or ilya ilich mechnikov (1845-1916): A pioneer in insect pathology, the first describer of the entomopathogenic fungus Metarhizium anisopliae and how to translate a Russian name. Biocontrol Sci. Technol. 5, 527–530. doi: 10.1080/09583159550039701
Keywords: Metarhizium, biological control, arthropod, insecticide, vector control
Citation: de Miranda RPR, Soares TKdA, Castro DP and Genta FA (2024) General aspects, host interaction, and application of Metarhizium sp. in arthropod pest and vector control. Front. Fungal Biol. 5:1456964. doi: 10.3389/ffunb.2024.1456964
Received: 29 June 2024; Accepted: 14 October 2024;
Published: 20 November 2024.
Edited by:
Jose Luis Ramirez, United States Department of Agriculture (USDA), United StatesReviewed by:
Seema Ramniwas, Chandigarh University, IndiaMavis Agyeiwaa Acheampong, University of Ghana, Ghana
Copyright © 2024 de Miranda, Soares, Castro and Genta. This is an open-access article distributed under the terms of the Creative Commons Attribution License (CC BY). The use, distribution or reproduction in other forums is permitted, provided the original author(s) and the copyright owner(s) are credited and that the original publication in this journal is cited, in accordance with accepted academic practice. No use, distribution or reproduction is permitted which does not comply with these terms.
*Correspondence: Daniele Pereira Castro, ZGFuaWNhc3Ryb0Bpb2MuZmlvY3J1ei5icg==
†These authors have contributed equally to this work and share last authorship