- Genetics of Eukaryotic Microorganisms, Institute of Microbiology and Genetics, Göttingen Center for Molecular Biosciences (GZMB), University of Göttingen, Göttingen, Germany
Understanding the interactions between fungal plant pathogens and host roots is crucial for developing effective disease management strategies. This study investigates the molecular mechanisms underpinning the chemotropic responses of the maize anthracnose fungus Colletotrichum graminicola to maize root exudates. Combining the generation of a deletion mutant with monitoring of disease symptom development and detailed analysis of chemotropic growth using a 3D-printed device, we identify the 7-transmembrane G-protein coupled receptor (GPCR) CgSte3 as a key player in sensing both plant-derived class III peroxidases and diterpenoids. Activation of CgSte3 initiates signaling through CgSo, a homolog to the Cell Wall Integrity Mitogen-Activated Protein Kinase (CWI MAPK) pathway scaffold protein identified in other filamentous fungi, facilitating the pathogen's growth towards plant defense molecules. The NADPH oxidase CgNox2 is crucial for peroxidase sensing but not for diterpenoid detection. These findings reveal that CgSte3 and CWI MAPK pathways are central to C. graminicola's ability to hijack plant defense signals, highlighting potential targets for controlling maize anthracnose.
1 Introduction
Root-infecting fungi pose a significant threat to food-producing plants, reducing both the yield and quality of harvested crops and fruits (Fisher et al., 2012). Understanding the mechanisms of fungal host root detection is crucial for developing sustainable crop protection strategies. The hemibiotrophic ascomycete Colletotrichum graminicola causes anthracnose in Zea mays, a disease characterized by brown lesions on leaves and stems (Bergstrom and Nicholson, 1999). The resulting yield loss is estimated at 10-20% worldwide annually for anthracnose stalk rot alone (Belisario et al., 2022). C. graminicola produces two types of asexual spores, oval- and falcate-shaped conidia, both capable of causing lesions on leaves (Nordzieke et al., 2019b). Oval conidia, asexual spores formed from hyphae in parenchyma cells adjacent to the vascular system, are responsible for root infection (Panaccione et al., 1989; Sukno et al., 2008; Belisario et al., 2022). After rapid germination in soil, these oval-shaped spores sense and redirect their growth towards host root exudates (chemotropism), followed by invasion of the host via its roots (Rudolph et al., 2024). In contrast, falcate conidia, produced in asexual fruiting bodies (acervuli), fail to invade roots under natural infection conditions (Panaccione et al., 1989; Rudolph et al., 2024).
In recent years, class III peroxidases (Prx) have been identified as attractant molecules for root pathogenic Fusarium and Verticillium species (Turra et al., 2015; Vangalis et al., 2023). For successful perception of plant Prx, these enzymes are activated by H2O2 generated by the reduction of O2 by fungal NADPH oxidase (Nox) 2 complex (O2 → O2⁻) and extracellular superoxide dismutase (Sod) (O2⁻ → H2O2) (Nordzieke et al., 2019a). The activated Prx is then sensed via fungal pheromone receptors Ste2 and Ste3, first described for their role in sensing α- and a-pheromones in the yeast S. cerevisiae (Nakayama et al., 1985; Turra et al., 2015; Nordzieke et al., 2019a; Sridhar et al., 2020; Vangalis et al., 2023; Ramaswe et al., 2024). Since Prx activity is essential for proper chemotropic growth induction, it is hypothesized that substrate oxidation by this enzyme directly or indirectly results in receptor activation (Nordzieke et al., 2019a). However, the mechanism by which Prx activates Ste2 and Ste3 remains unknown. The provision of cellular reactive oxygen species (ROS) by Nox complexes is a well-known phenomenon in fungal, mammalian, and plant cells, regulating reproduction, signaling, defense against harmful organisms, and pathogenicity (Sagi and Fluhr, 2006; Lambert and Brand, 2009; Ryder et al., 2013; Dirschnabel et al., 2014; Nordzieke et al., 2019a; Vermot et al., 2021). In fungi, Nox1/A and Nox2/B complexes contribute to proper development and interaction with host plants. Despite sharing a set of common regulatory proteins, their functions are highly specific and not interchangeable. Phenotypic characterization of deletion mutants revealed that Nox1/A is required for developmental processes such as germling fusion and sexual development, while the Nox2/B complex regulates the germination of sexual spores (Cano-Dominguez et al., 2008; Brun et al., 2009; Dirschnabel et al., 2014; Wang et al., 2014). Apart from Nox2’s role in host recognition processes, both Nox complexes are involved in the penetration of plant surfaces. In Pyricularia oryzae and Fusarium species, Nox1/A and Nox2/B are required for proper septin ring formation, a prerequisite for penetration peg formation and elongation, respectively (Ryder et al., 2013; Wang et al., 2014; Nordzieke et al., 2019a). However, reports on Botrytis cinerea and Colletotrichum species suggest that Nox1/A, but not Nox2/B, is dispensable for the proper function of appressoria (Segmüller et al., 2008; Fu et al., 2022; Liu et al., 2022). Although there is experimental evidence that Nox-derived ROS functions via the regulation of actin (Ryder et al., 2013; Liu et al., 2022), the molecular processes resulting in Nox activation and induced downstream processing are not fully understood.
In contrast to the described findings, our lab recently identified maize-secreted diterpenoids, rather than Prx, as the attractant molecules responsible for inducing C. graminicola chemotropic growth towards maize roots. The a-pheromone receptor Ste3 of C. graminicola is responsible for the perception of these secondary metabolites (Rudolph et al., 2024). In this study, we elucidate conserved molecular processes determining chemotropic growth to plant root signals. We demonstrate that C. graminicola germlings can sense and redirect their growth to Prx signals in patterns similar to those observed in Fusarium oxysporum f. sp. Lycopersici. Investigation of a Cgnox2 deletion mutant provides evidence that the Nox2 complex is essential for adequate leaf penetration via appressoria and hyphopodia and for chemotropic sensing of Prx. However, Cgnox2 is dispensable for host plant-derived diterpenoid sensing and maize root infection by C. graminicola. Using genetic experiments, we show that the a-pheromone receptor CgSte3 and the Cell Wall Integrity (CWI) Mitogen-Activated Protein Kinase (MAPK) scaffold protein CgSo mediate the perception of diterpenoids and Prx. Our findings reveal that conserved molecular pathways for the perception of root-derived signals are shared among several plant pathogenic fungi, independent of their importance for recognizing the appropriate host.
2 Materials and methods
2.1 Strains, growth conditions, and collection of spores
As wildtype strain, the sequenced C. graminicola (Ces.) G.W. Wilson (teleomorph Glomerella graminicola D. J. Politis) strain M2 was used (also referred to as M1.001) (Forgey et al., 1978; O'Connell et al., 2012). Oval and falcate conidia were cultivated and harvested as described previously (Rudolph et al., 2024).
2.2 Generation of plasmids and C. graminicola strains
A ΔCgnox2 deletion mutant and the complemented strain ΔCgnox2::Cgnox2 were generated. DNA hydrolysis and sequencing, using appropriate enzymes and primers, verified all plasmids. The oligonucleotides, strains, and plasmids used are listed in Supplementary Tables S1-S3.
For the generation of Cgnox2 (GLRG_09327) deletion, the plasmid pCgnox2_KO was assembled using a split marker approach (Catlett et al., 2003) followed by subcloning in pJET1.2/Blunt (Thermo Fisher Scientific). 5’ and 3’ regions of Cgnox2 were amplified using oligonucleotide pairs nox2_PF/nox2_PR (1,149 bp) and nox2_TF/nox2_TR (1,160 bp), respectively. In a second step, those regions were fused to an inverted hph cassette (hpf-f/hph-r, 1,417 bp), mediating the resistance to hygromycine B, with the oligonucleotides nox2_PFN/nox2_TRN (3,436 bp). The plasmid pCgnox2_nat for was assembled using the NEBuilder HiFi DNA Assembly Cloning Kit (New England Biolabs) according to the instruction manual. 5’ and 3’ regions of Cgnox2 were amplified together with the Cgnox2 gene in a PCR using genomic DNA (gDNA) of C. graminicola as a template and the oligonucleotides nox2_P_comp_fw/nox2_T_comp_rv (4,093 bp). As the backbone for the assembly reaction served pJet_nat linearized with EcoRV (Nordzieke, 2022), mediating resistance to nourseothricin-dihydrogen sulfate in C. graminicola transformed with this plasmid.
Prior to transformation, the plasmids pCgnox2_KO and pCgnox2_nat were linearized using the enzymes HindIII and PvuI, respectively. Oval conidia of CgM2 (transformation of pCgnox2_KO) or ΔCnox2 (transformation of pCgnox2_nat) served as the basis for the generation of protoplasts as described previously (Groth et al., 2021). After transformation, regenerating protoplasts were selected on a medium containing hygromycin B (500 µg/ml, transformation of pCgnox2_KO) or nourseothricin-dihydrogen sulfate (70 µg/ml, transformation of pCgnox2_nat). Single spore isolations were performed of antibiotic-resistant and PCR-verified primary transformants to obtain homokaryotic strains (Nordzieke, 2022). Single spore isolates of ΔCnox2 were verified by Southern Blot analyses. The hydrolysis of gDNA was performed with the enzyme BglII (Thermo Fisher Scientific). For visualization of successful deletion of the Cgnox2 gene, the 3’ region of Cgnox2 was amplified in a PCR reaction (Nox2_probe_F/Nox2_TRN, 1,909 bp) and used as a specific probe in the following hybridization reaction (expected sizes: CgM2 1,763 bp, ΔCgnox2 3,572 bp, Supplementary Figure S1). Re-integration of Cgnox2 into the ΔCgnox2 deletion strain was tested using the primer pair nox2_P_comp_fw and nox2_eGFP_YR_rv (2,892 bp) in a PCR approach (Supplementary Figure S2).
2.3 Chemotropic growth assays
Chemotropic growth towards different chemoattractants, maize root exudate (MRE), dihydrotanshinone I (DHT, Sigma Aldrich), and peroxidase from horseradish (HRP, Sigma Aldrich), was quantified after 6 h of incubation on agar in a 3D printed device as described previously (Schunke et al., 2020; Groth et al., 2021). The difference between attraction and non-stimulation was calculated shown with the calculated chemotropic index (Turra et al., 2015). Root exudate of maize plant was generated like described previously (Rudolph et al., 2024). To test the effect of heat on the activity of MRE and HRP, both were exposed to 99°C for 20 min.
2.4 Analysis of leaf infection
The ability of C. graminicola asexual spores to penetrate maize plant material was analyzed on the second leaves of the Z. mays cultivar ‘Mikado’ (KWS SAAT SE, Einbeck, Germany). Unless otherwise stated, incubation of plants was performed in a PK 520 WLED plant chamber (Poly Klima Climatic Growth System, Freising, Germany) using a day/night cycle of 12 h 26°C/12 h 18°C. The strains CgM2, ΔCnox2, and ΔCgnox2::Cgnox2 were used for the infection experiments. Oval and falcate conidia were adjusted to 105 spores per ml in 0.01% Tween. The second lowest maze leaves were fixated on wet blotting paper (BF2 580x 600mm, Sartorius, Göttingen, Germany) in square Petri dishes (82.9923.422, Sarstedt, Nümbrecht, Germany). Drops of 10 µl of the spore solution were added on top of the leaves. Analysis of symptom development was done after 5 days of incubation at 23°C with a rating of four different categories (no symptoms, minor symptoms, symptoms, severe symptoms) as described earlier (Nordzieke et al., 2019b). At least 40 individual spots were rated for fungal infection and mock infections (10 µl droplets of 0.01% Tween 20).
2.5 Analysis of root infection outgoing of spore-enriched vermiculite
The natural root infection outgoing from C. graminicola conidia present in growth substrated was simulated (Rudolph et al., 2024). Therefore, maize seeds were planted in 40 g of vermiculite (Vermiculite Palabora, grain size 2-3 mm, Isola Vermiculite GmbH, Sprockhövel, Germany) enriched with oval conidia of CgM2, ΔCgnox2 and ΔCgnox2::Cgnox2 in a concentration of 7.5 x 104 x ml-1. As a mock control, seeds were sown in vermiculite mixed with water. Pots were sealed in disposal plastic bags (Sarstedt, Nümbrecht, Germany) to ensure high humidity. 21 dpi, length and biomass of the above-ground plant were determined.
2.6 Microscopy
Visualization of fungal structures on leaves was performed with light (differential interference contrast (DIC)) microscopy with the Axiolmager M1 microscope (Zeiss, Jena, Germany). The Photometrix coolSNAP HQ camera (Roper Scientific, Photometrics, Tucson, AZ, USA) was used to capture images. For image processing the ZEISS ZEN software (version 2.3, Zeiss) was used. For better visibility of penetration structures, leaf infection experiments were stopped after 3 d and the corresponding leaves stained with chlorazol Black E (Brachmann et al., 2001).
2.7 Vegetative growth assay
A defined agar plug [Ø 9 mm, CM medium (Rudolph et al., 2024)] overgrown with fungal mycelium of the strains CgM2, ΔCgnox2, ΔCgnox2::Cgnox2, ΔCgste3 and ΔCgste3::Cgste3 to CM plates and incubated for 7 days at 23°C. Pictures were taken with a scanner (Epson Perfection V600 Photo, Epson, Tokyo, Japan). Growth areas were calculated with FIJI and growth rates were calculated for two following days.
2.8 Statistics
For all experiments in this study, the T-test for unequal variances was used (Ruxton, 2006).
3 Results
3.1 Oval conidia of Colletotrichum graminicola are attracted by peroxidases
Tomato root exudated class III peroxidases (Prx) attracting F. oxysporum f. sp. lycopersici can be replaced by commercially available horseradish peroxidase (HRP) sharing a 37–39% amino acid similarity (Turra et al., 2015). In this study, the attraction of C. graminicola oval conidia to HRP was analyzed (Figure 1). Our data show that the amount of germlings able to re-direct growth to HRP is dose-dependent. The attraction of germlings peaked at an HRP gradient originating from 4 µM, whereas increasing concentrations did not provoke a redirection of germlings. However, comparatively higher doses of 128 µM HRP are again attracting germlings of the maize pathogen. Intriguingly, the resulting dose-response curve is highly similar to experimental data of F. oxysporum (Nordzieke et al., 2019a), raising the question whether molecular components required for Prx sensing might be conserved in C. graminicola as well.
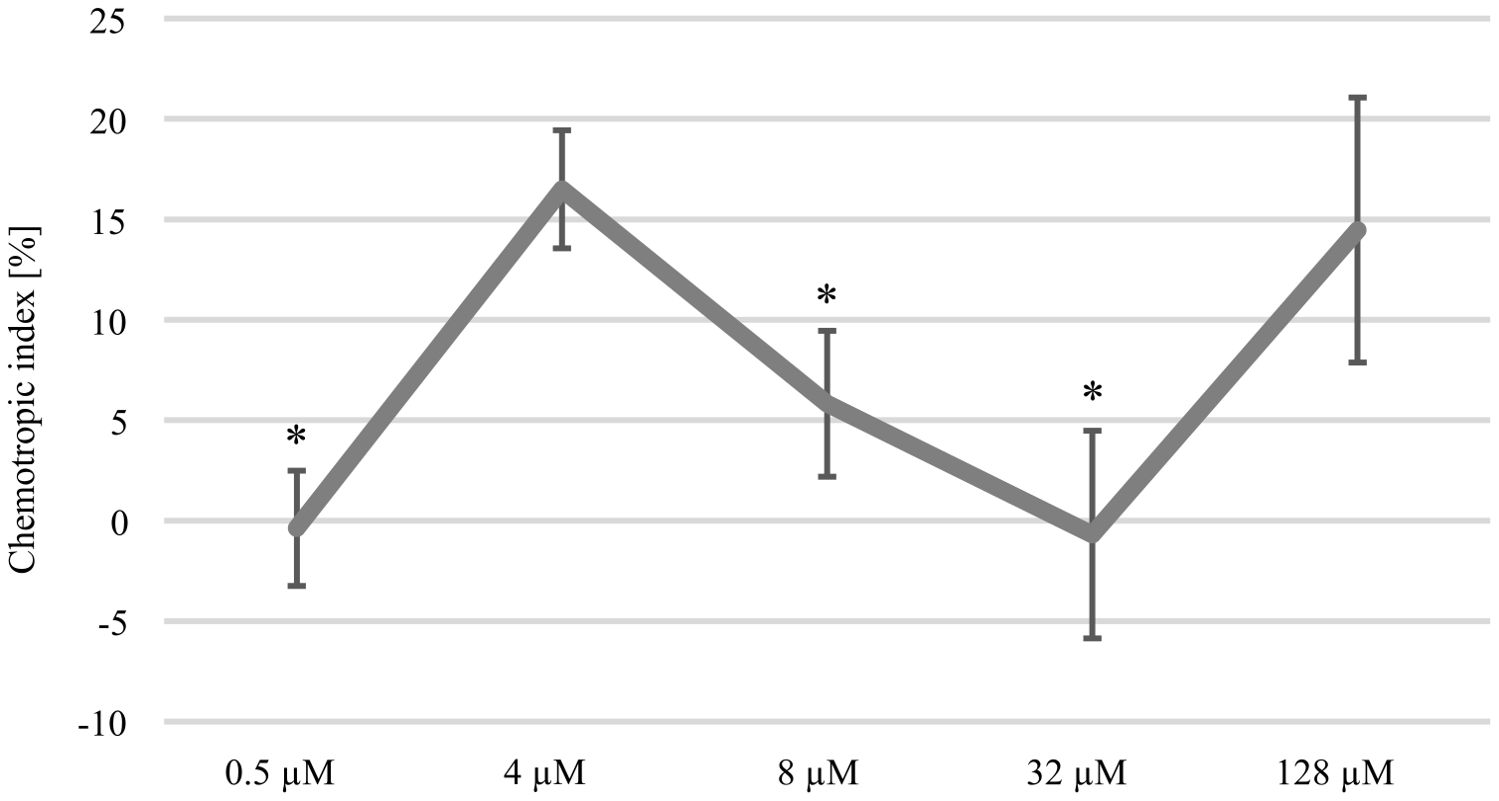
Figure 1. Dose-response curve to horse radish peroxidase (HRP). Chemotropic index displaying the attraction of CgM2 oval conidia by different concentrations of HRP. Redirection of growth was estimated after incubation for 6 h in a 3D printed device developed to analyze directed growth responses (Schunke et al., 2020). Error bars represent SD calculated from n ≥ 4 experiments, *p < 0.05, calculated with a two-tailed t-test.
3.2 Deletion of Cgnox2 impairs the functionality of melanized penetration structures
Homologs of Cgnox2, the catalytic subunit of fungal Nox2 complexes, are responsible for plant-derived Prx sensing by F. oxysporum (Nordzieke et al., 2019a) and contribute to proper penetration structure function in several ascomycetes. To investigate the impact of CgNox2 on the development of maize anthracnose, a deletion mutant of the corresponding gene was generated and analyzed regarding its impact on leaf and root infection processes.
Dependent on falcate or oval conidia inoculum, C. graminicola produces melanized penetration structures directly from spores (appressoria) or outgoing from fungal hyphae (hyphopodia), respectively (Nordzieke et al., 2019b). To assess the impact of Cgnox2 deletion on the functionality of both infection structures, leaf infection experiments were conducted. Symptom rating after 5 days of inoculation revealed that independent of the spore type tested, ΔCgnox2 strains did not cause anthracnose lesions. This phenotype was fully rescued in experiments using inocula of oval or falcate conidia of the complemented strain ΔCgnox2::Cgnox2 (Figures 2A, B). In several plant pathogenic fungi, a drop in disease symptom development of investigated mutants was tracked down to reduced vegetative growth. We thus quantified the growth area of wildtype and the ΔCgnox2 deletion mutant and found a slight reduction of mutant growth, which was, however, not statistically different at all time points analyzed (Supplementary Figure S3). When we monitored fungal development in planta 3 dpi, melanized penetration structures were visible for all strains and spore types tested, indicating that the formation of appressoria and hyphopodia is not hampered by Cgnox2 deletion. Detailed imaging of chlorazol-stained leaves revealed that primary hyphae are visible in planta in CgM2 and ΔCgnox2::Cgnox2 experiments. In experiments using ΔCgnox2 inocula, we were unable to monitor any leaf-colonizing hyphae, indicating that Cgnox2 is required for proper penetration outgoing from appressoria and hyphopodia (Figure 2C).
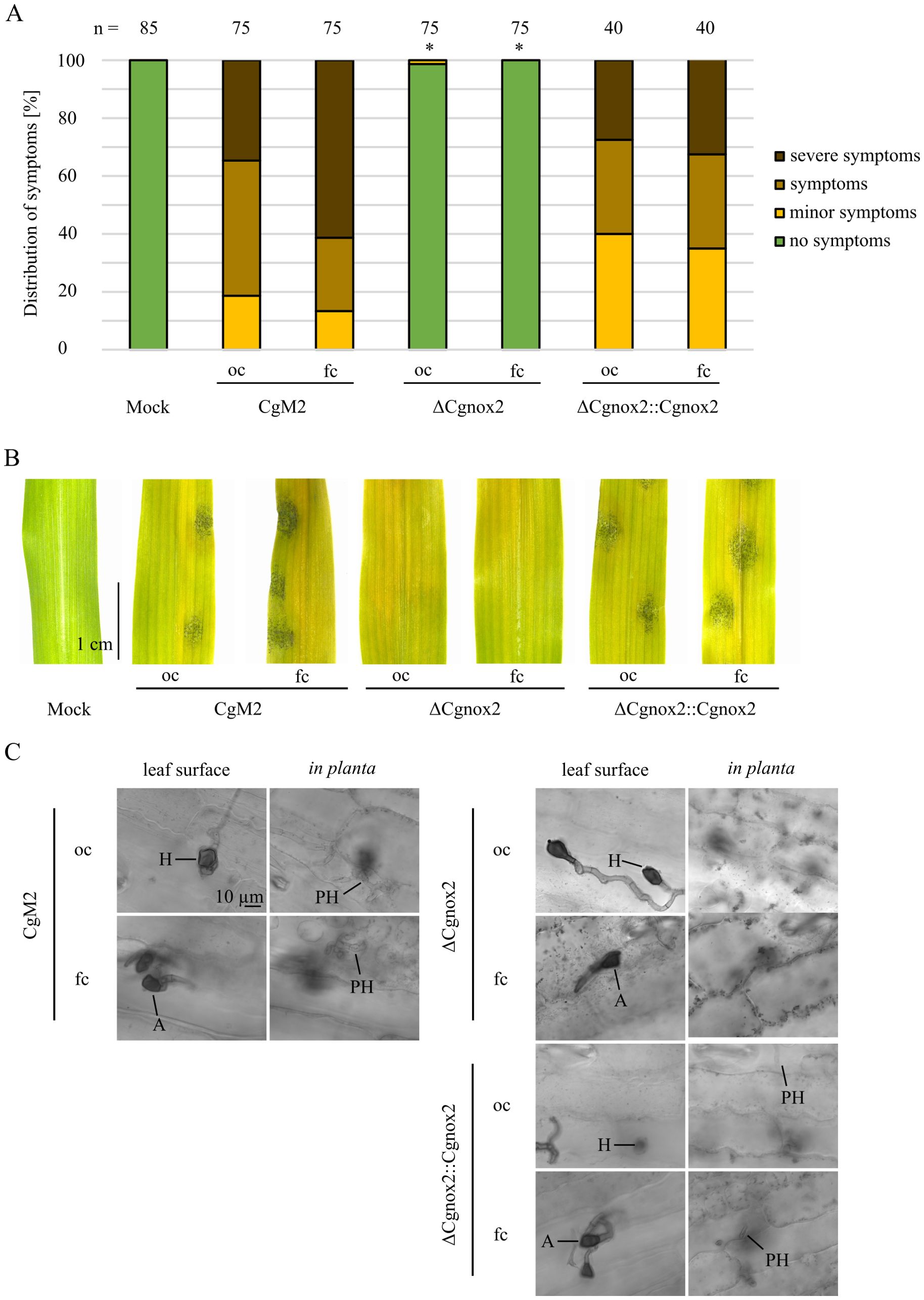
Figure 2. Symptom development on leaves provoked by different C. graminicola strains. (A–C) Infection of 16-day old maize leaves with oval (oc) and falcate (fc) conidia of the wild type CgM2, the mutant strain ΔCgnox2 and complemented strain ΔCgnox2::Cgnox2. 103 spores were applied per infection site on the leaves. (A, B) Evaluation after 5 dpi. Symptoms are classified into four categories (no symptoms, minor symptoms, symptoms, severe symptoms) as described before (Nordzieke et al., 2019b). Infection spots evaluated n ≥ 40. *p < 0.05, calculated with two-tailed t-test in comparison to symptom distribution of CgM2 infected leaves. (A) Quantification of symptoms in percent. (B) Representative pictures of mock-treated and conidia-infected leaves. Size bar = 1 cm. (C) Monitoring of leaf infection sites 3 dpi. After staining with chlorazol Black E, cross-sections were taken, Size bar = 10 µm, H, hyphopodia; A, appressoria; PH, primary hyphae.
3.3 CgNox2 is dispensable for maize root infection and diterpenoid sensing
Recently, we identified diterpenoids as required signals exuded from maize roots, which attract C. graminicola germlings. To test whether also peroxidases might have a substantial impact on the overall attraction by maize roots, we tested whether boiling affects the attraction potential of maize root exudate and HRP. As depicted in Figure 3A, attractant molecules in maize root exudate are heat stable, whereas HPR activity is lost after boiling, indicating that peroxidases are not guiding C. graminicola germlings to host roots. Next, we analyzed a probable involvement of CgNox2 on the infection of roots and sensing of maize root exudate and diterpenoids. For root infection experiments, we mixed oval conidia of different C. graminicola strains with vermiculite and inseminated the contaminated soil with maize seeds. This experimental setup mimics the natural infection situation in the field in which soil-borne fungal pathogens have to grow towards roots of their host prior to infection (Rudolph et al., 2024). As depicted in Figures 3B, C, the C. graminicola wildtype CgM2 is able to stunt the above-ground tissue of growing maize plants. Likewise, the Cgnox2 deletion strain showed a strong stunting phenotype, indicating that CgNox2 is dispensable for root sensing and its infection. These finding are supported by experiments in which the amount of germlings attracted by maize root exudate (MRE) and the diterpenoid dihydrotanshinone I (DHT) was analyzed (Figure 3D). C. graminicola wildtype and ΔCgnox2 germlings show a strong chemotropic response to MRE as well as DHT, indicating that Cgnox2 is not required for the sensing of the applied attractants.
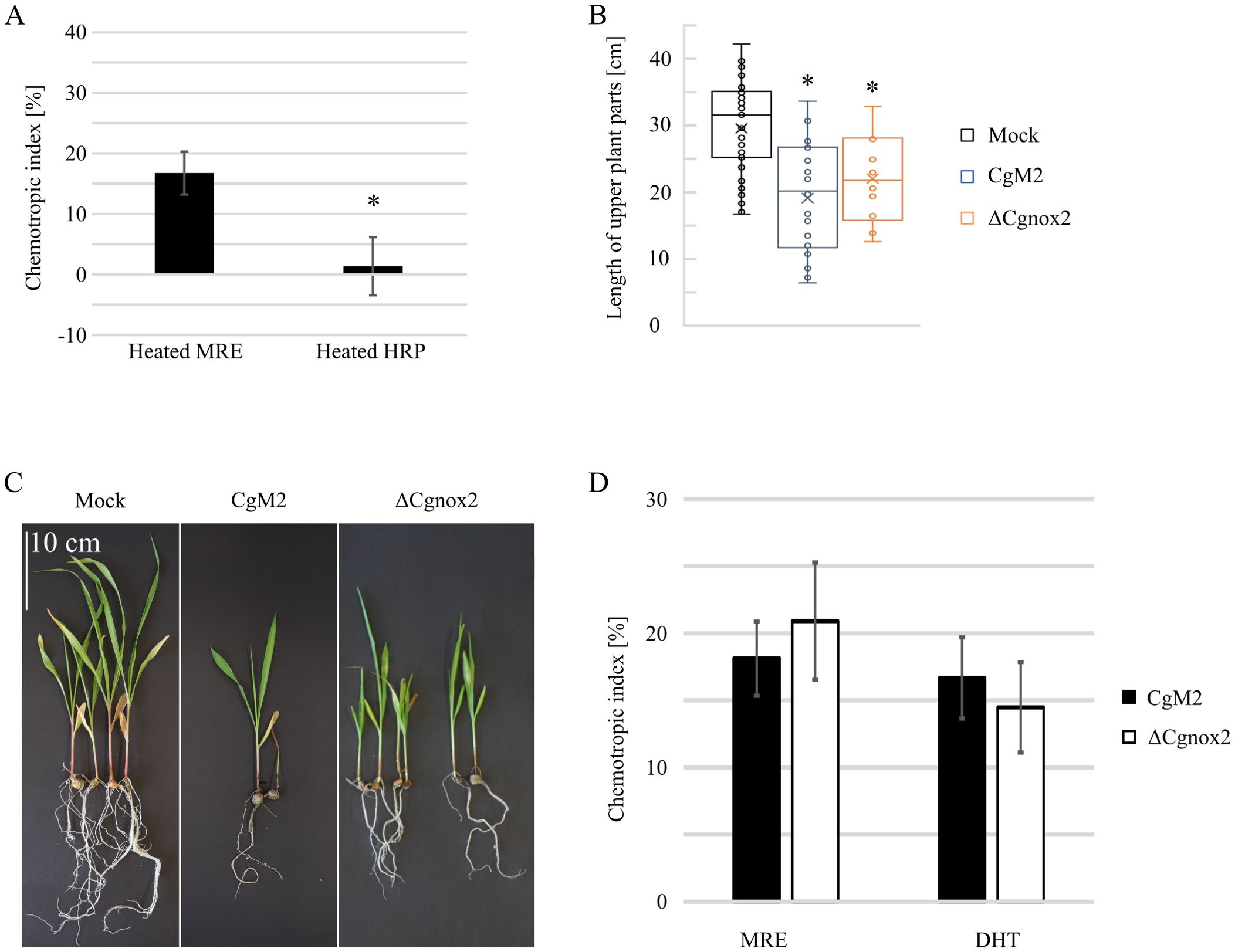
Figure 3. Relevance of CgNox2 for maize root infection. (A) Chemotropic index of CgM2 facing gradients of heated MRE and heated HRP, n ≥ 3. (B, C) Symptom development of plants co-incubated with oval conidia (oc) of CgM2 and ΔCgnox2. (B) Quantification of length of above-ground plant parts. The error bars represent the SD from ≥ 6 plants. (C) Representative depiction of the development of infected plants, scale bar = 10 cm. (D) Chemotropic index of CgM2 and ΔCgnox2 oval conidia facing gradients of maize root exudates (MRE) and the diterpenoid DHT, n ≥ 3. *p < 0.05, calculated with two-tailed t-tests.
3.4 Conserved pathway components mediate the perception of peroxidases and diterpenoids
The fungal pheromone receptors Ste2 and Ste3 of Fusarium species function in recognizing Nox2-activated peroxidase, inducing the phosphorylation cascade of the downstream CWI MAPK pathway (Turra et al., 2015; Nordzieke et al., 2019a; Sharma et al., 2022). To analyze whether a conserved Prx sensing machinery exists in C. graminicola, we confronted different deletion strains with the peaking HRP concentrations of 4 and 128 µM (Figure 1). Similar to findings in F. oxysporum, ΔCgnox2 germlings were unable to redirect growth to 4 µM HRP, but fully able to recognize 128 µM (Figure 4A). A deletion mutant of the a-pheromone receptor gene Cgste3 (Rudolph et al., 2024), however, was not attracted by either HRP concentrations applied. To investigate a probable requirement for CWI MAPK components, we further employed the role of Cgso (Nordzieke, 2022), encoding for a scaffolding protein of this pathway, for Prx sensing. Similarly to ΔCgste3, the applied HRP gradients did not elicit a chemotropic growth response in ΔCgso. Attraction was fully restored in the complementation strains ΔCgnox2::Cgnox2, ΔCgste3::Cgste3, and ΔCgso::Cgso. Together our results indicate that central molecular components for Prx sensing are conserved among F. oxysporum and C. graminicola. For further characterization of diterpenoid sensing, we confronted C. graminicola wildtype, ΔCgste3, ΔCgste3::Cgste3, ΔCgso, and ΔCgso::Cgso germlings with MRE and DHT gradients (Figure 4B). Similar to our observation for HRP sensing, the Cgso deletion mutant was unable to sense those molecules. Comparable to ΔCgnox2, also the deletion of Cgste3 and Cgso do result in slightly reduced growth patterns (Supplementary Figure S3) (Nordzieke, 2022), which are however, not statistically different to wildtype at all time points analyzed. Together, these results indicate that the sensing of chemically very different root-exudated molecules is routed via identical molecular pathways and is conserved among different fungal root pathogens (Figure 4C).
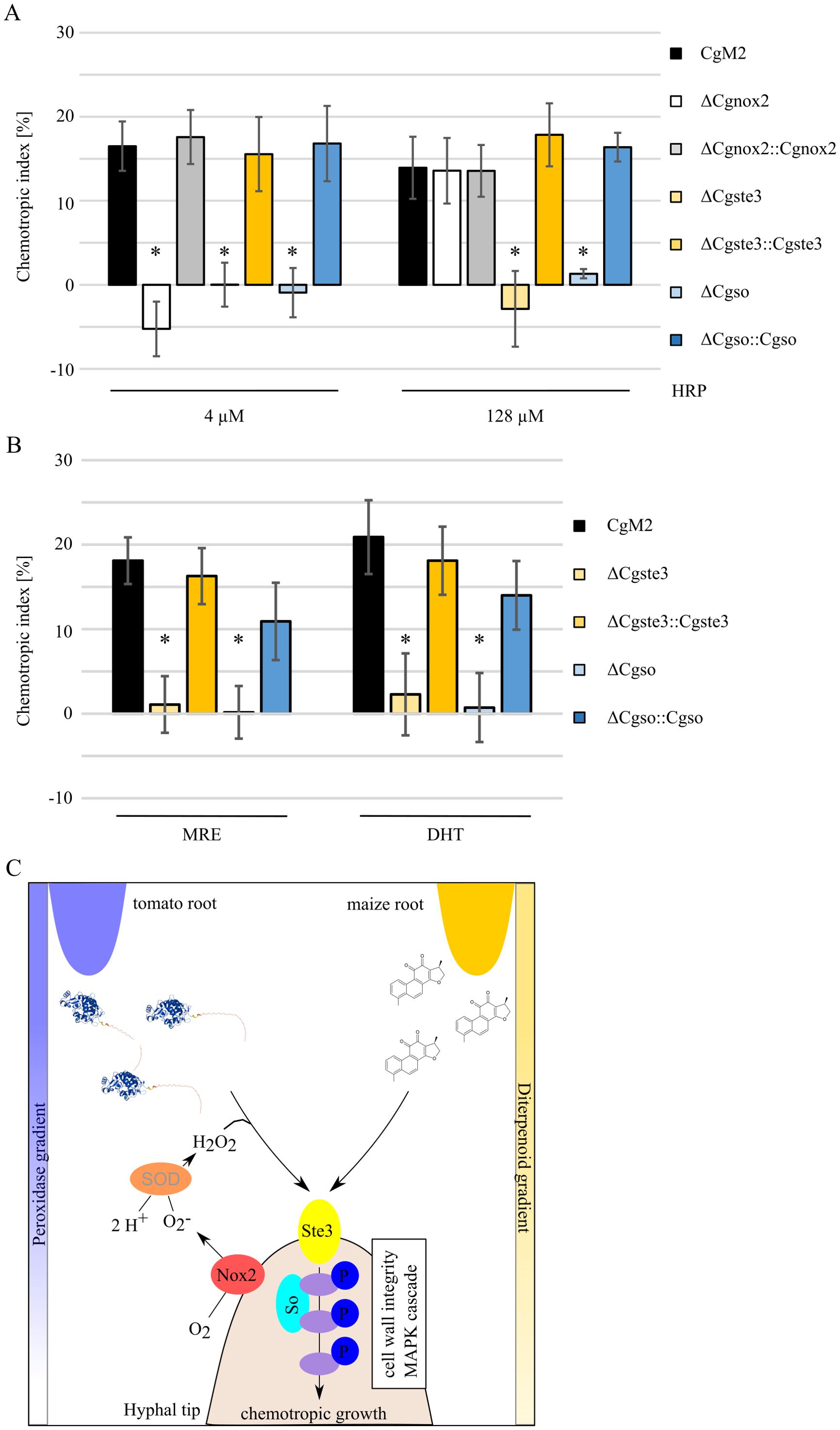
Figure 4. Signaling pathway components required for recognition of diterpenoids and peroxidases. (A, B) Oval conidia (oc) of C. graminicola deletion mutants facing gradients of 4 and 128 µM (A) or maize root exudate (MRE) and the diterpenoid dihydrotanshione I [DHT, (B)] as indicated. The error bars represent the SD from ≥ 4 replicates, *p < 0.05, calculated with two-tailed t-tests. (C) Model of conserved molecular pathways leading to growth re-direction due to peroxidase or diterpenoid gradient sensing, Nox2 = NADPH oxidase complex 2, Ste3 = a-pheromone receptor, So = scaffold protein of the cell wall integrity MAPK module, SOD = extracellular superoxide dismutase. Molecular factors investigated in this study or included from literature are written in black or grey letters, respectively. Molecule structure of peroxidase was generated by AlphaFold 3 (HRP_22489.1), chemical structure of DHT was drawn with ACD/ChemSketch.
4 Discussion
The rhizosphere is an environment full of different compounds such as nutrients and plant-based metabolites to attract or kill microbes (Vives-Peris et al., 2020). As numerous studies showed, plant exudates have a central role in shaping the root environment and are constantly adapted to fulfil the needs of the plant (Guerrieri et al., 2002; Khorassani et al., 2011; Chaparro et al., 2013; Cheng and Cheng, 2015). Knowing this changeable environment is crucial for plant-interacting fungi and a prerequisite to find their hosts and to avoid hazardous environments. Increasing evidence exist that fungal root pathogens have explored a possibility to hijack plant defense mechanisms to identify a close-by host (Turra et al., 2015; Sridhar et al., 2020; Vangalis et al., 2023; Ramaswe et al., 2024). In Fusarium and Verticillum species interacting with tomato plants, class III peroxidases (Prx) secreted from host roots induce a redirection of germling growth towards those plant defense molecules, instead of being repelled (Turra et al., 2015; Vangalis et al., 2023). Recently, we reported a similar growth response to be induced by plant-derived diterpenoids, a further known class of plant defense molecules (Mafu et al., 2018; Rudolph et al., 2024). In this study, we provide evidence that independent for their relevance for host recognition, C. graminicola is able to sense and react to Prx as well as ditpernoids. Several molecular factors like the a-pheromone receptor CgSte3 and the Cell Wall Integrity MAPK scaffold protein CgSo are required for the sensing of Prx and diterpenoids, despite the very different chemical properties of these molecules. In contrast, the activity of CgNox2 is specific for the recognition of Prx, but dispensable for diterpenoid sensing.
Prx take part in several plant defense processes, including cell wall enforcement, auxin metabolism, phytoalexin synthesis and the generation of reactive oxygen and nitrogen species (ROS, RNS) (Almagro et al., 2009). Despite of being defense enzymes, Prx are constantly expressed to a basal level, but their generation is accelerated in the presence of plant pathogenic fungi and bacteria (Young et al., 1995; Sasaki et al., 2004; Lavania et al., 2006; De-la-Pena et al., 2008). Several products of Prx activity like ROS, RNS and phytoalexins are well studied for their negative impact on membranes, resulting in lipid oxidations, alteration of the activity of membrane associated enzymes, impairment of membrane fluidity and increase of membrane permeability (Di Meo et al., 2016; Su et al., 2019; Endale et al., 2023; Jeandet et al., 2023). Diterpenoids are C20 compounds based on four isoprene (C5H8) units, which form a large and structurally diverse class of natural products found in plants, animals and fungi (Hanson, 2009; De Sousa et al., 2018). The antifungal activity against several maize pathogenic species including B. cinerea or Rhizopus microsporus was reported (Mendoza et al., 2009; Schmelz et al., 2011). As the products of Prx activity, diterpenoids can interact with membranes in various ways. For several diterpenoid molecules with antimicrobial functions, damaging interactions with membranes were reported (De Sousa et al., 2018; Saha et al., 2022) as well as their potential to induce ROS formation (Sun et al., 2021).
The a- and α-pheromone receptors Ste3 and Ste2 were first identified in yeast for their role in the recognition of the vice versa pheromones during mating (Hagen and Sprague, 1984; Nakayama et al., 1985; Jenness et al., 1986). In filamentous fungi, the homologous G-protein coupled receptors (GPCRs) have acquired additional functions, including regulation of germination and the sensing of class III peroxidases and ditpernoids besides the sensing of pheromones (Turra et al., 2015; Vitale et al., 2019; Sharma et al., 2022; Rudolph et al., 2024). Together, these results raise the question, how a single GPCR respond to such a diverse set of molecules. GPCRs represent the largest class of signaling receptors known and are able to respond to various ligands, including protons, lipids, nutrients, and pheromones (Roth et al., 2017). After ligand recognition, GPCR activate downstream MAPK pathways, which in turn mediate the induction of various cellular responses (Xue et al., 2008; Braunsdorf et al., 2016). Besides the classical GPCR, there are several unconventional 7 transmembrane receptors. Adhesion GPCRs (aGPCRs) are characterized by an extended extracellular N-terminal region, which is able to bind several ligands each on a distinct protein fold (Araç et al., 2016). Further GPCRs can interact with other receptors types like protein tyrosine kinase receptors (PTKRs) and serine/threonine kinase receptors (S/TKRs) in a process termed transactivation (Schafer and Blaxall, 2017; Mohamed et al., 2019). Intriguingly, GPCRs are described to activate release of PTKR or S/TKR ligand upon activation, but also the activation of GPCRs by a primary activated PTKR or S/TKR is described (Kilpatrick and Hill, 2021). In this way, a GPCR can serve as a signaling hub for very different primary ligands. A central second messenger mediating transactivation are ROS, metabolites that are generated during peroxidase and diterpenoid plant defense responses. Whether ROS is also involved in direct or indirect GPCR activation in fungal chemotropic growth remains to be studied in future investigations.
Taken together, our results show that the 7 transmembrane G-protein coupled receptor (GPCR) CgSte3 is central for the sensing of chemically very different plant molecules as class III peroxidases and diterpenoids in the anthracnose fungus Colletotrichum graminicola. Upon activation, CgSte3 induces signaling via the downstream Cell Wall Integrity Mitogen Activated Protein Kinase pathway, resulting in a directed growth response of the plant pathogen towards a gradient of defense molecules, thus hitchhiking the original plant defense response. Upstream of CgSte3, we identified the NADPH oxidase CgNox2 as a specific factor for peroxidase sensing, which is dispensable for the perception of diterpenoids. The detailed molecular processes enabling a single GPCR to sense such chemically distinct molecules have to be revealed in future investigations.
5 Conclusions
This study explores the molecular mechanisms by which Colletotrichum graminicola interacts with plant root-secreted defense molecules. The 7-transmembrane G-protein coupled receptor (GPCR) CgSte3 is identified as crucial for sensing maize root exudates, including class III peroxidases and diterpenoids. Activation of CgSte3 triggers the Cell Wall Integrity Mitogen-Activated Protein Kinase (CWI MAPK) pathway, directing fungal growth towards these plant defense compounds. The NADPH oxidase CgNox2 is essential for peroxidase detection, highlighting a specific sensing mechanism. These findings reveal that CgSte3 and CWI MAPK pathways are central to C. graminicola’s ability to exploit maize defenses, offering promising targets for controlling maize anthracnose. Future research should focus on understanding how CgSte3 detects diverse signals, which could lead to innovative disease management strategies.
Data availability statement
The raw data supporting the conclusions of this article will be made available by the authors, without undue reservation.
Author contributions
AR: Investigation, Validation, Writing – original draft, Writing – review & editing, Methodology. CS: Investigation, Writing – review & editing. DN: Investigation, Writing – original draft, Writing – review & editing, Conceptualization, Funding acquisition, Resources, Supervision, Validation.
Funding
The author(s) declare financial support was received for the research, authorship, and/or publication of this article. This work was funded by the Deutsche Forschungsgemeinschaft (Bonn-Bad Godesberg). Grant was provided to DN (project NO 1230/3-1 (447175909). This work was partly supported by the Göttingen Graduate Center for Neurosciences, Biophysics, and Molecular Biosciences at the Georg-August-Universität Göttingen.
Acknowledgments
We thank Gabriele Beyer and Gertrud Stahlhut for excellent technical assistance. The generative AI ChatGPT Version 3.5 was used to check this manuscript for grammar and typing errors. We acknowledge support by the Open Access Publication Funds of the Göttingen University. This article was added to the preprint server BioRxiv (doi: 10.1101/2024.07.27.605416).
Conflict of interest
The authors declare that the research was conducted in the absence of any commercial or financial relationships that could be construed as a potential conflict of interest.
Publisher’s note
All claims expressed in this article are solely those of the authors and do not necessarily represent those of their affiliated organizations, or those of the publisher, the editors and the reviewers. Any product that may be evaluated in this article, or claim that may be made by its manufacturer, is not guaranteed or endorsed by the publisher.
Supplementary material
The Supplementary Material for this article can be found online at: https://www.frontiersin.org/articles/10.3389/ffunb.2024.1454633/full#supplementary-material
References
Almagro L., Gomez Ros L., Belchi-Navarro S., Bru R., Ros Barcelo A., Pedreño M. (2009). Class III peroxidases in plant defence reactions. J. Exp. Bot. 60, 377–390. doi: 10.1093/jxb/ern277
Araç D., Sträter N., Seiradake E. (2016). “Understanding the structural basis of adhesion GPCR functions,” in Langenhan T., Schöneberg T. Eds. Adhesion G Protein-coupled Receptors. Handbook of Experimental Pharmacology, vol 234. Cham: Springer, 67–82. doi: 10.1007/978-3-319-41523-9_4
Belisario R., Robertson A. E., Vaillancourt L. J. (2022). Maize anthracnose stalk rot in the genomic era. Plant Dis. 106, 2281–2298. doi: 10.1094/PDIS-10-21-2147-FE
Bergstrom G. C., Nicholson R. L. (1999). The biology of corn anthracnose: knowledge to exploit for improved management. Plant Dis. 83, 596–608. doi: 10.1094/PDIS.1999.83.7.596
Brachmann A., Weinzierl G., Kämper J., Kahmann R. (2001). Identification of genes in the bW/bE regulatory cascade in Ustilago maydis. Mol. Microbiol. 42, 1047–1063. doi: 10.1046/j.1365-2958.2001.02699.x
Braunsdorf C., Mailänder-Sanchez D., Schaller M. (2016). Fungal sensing of host environment. Cell. Microbiol. 18, 1188–1200. doi: 10.1111/cmi.12610
Brun S., Malagnac F., Bidard F., Lalucque H., Silar P. (2009). Functions and regulation of the Nox family in the filamentous fungus Podospora anserina: a new role in cellulose degradation. Mol. Microbiol. 74, 480–496. doi: 10.1111/j.1365-2958.2009.06878.x
Cano-Dominguez N., Alvarez-Delfin K., Hansberg W., Aguirre J. (2008). NADPH oxidases NOX-1 and NOX-2 require the regulatory subunit NOR-1 to control cell differentiation and growth in Neurospora crassa. Eukaryot. Cell 7, 1352–1361. doi: 10.1128/EC.00137-08
Catlett N. L., Lee B.-N., Yoder O., Turgeon B. G. (2003). Split-marker recombination for efficient targeted deletion of fungal genes. Fungal Genet. Rep. 50, 9–11. doi: 10.4148/1941-4765.1150
Chaparro J. M., Badri D. V., Bakker M. G., Sugiyama A., Manter D. K., Vivanco J. M. (2013). Root exudation of phytochemicals in Arabidopsis follows specific patterns that are developmentally programmed and correlate with soil microbial functions. PLoS One 8, e55731. doi: 10.1371/annotation/51142aed-2d94-4195-8a8a-9cb24b3c733b
Cheng F., Cheng Z. (2015). Research progress on the use of plant allelopathy in agriculture and the physiological and ecological mechanisms of allelopathy. Front. Plant Sci. 6, 160714. doi: 10.3389/fpls.2015.01020
De-la-Pena C., Lei Z., Watson B. S., Sumner L. W., Vivanco J. M. (2008). Root-microbe communication through protein secretion. J. Biol. Chem. 283, 25247–25255. doi: 10.1074/jbc.M801967200
De Sousa I. P., Sousa Teixeira M. V., Jacometti Cardoso Furtado N. A. (2018). An overview of biotransformation and toxicity of diterpenes. Molecules 23, 1387. doi: 10.3390/molecules23061387
Di Meo S., Reed T. T., Venditti P., Victor V. M. (2016). Role of ROS and RNS sources in physiological and pathological conditions. Oxid. Med. Cell. Longev. 2016, 1245049. doi: 10.1155/2016/1245049
Dirschnabel D. E., Nowrousian M., Cano-Dominguez N., Aguirre J., Teichert I., Kück U. (2014). New insights into the roles of NADPH oxidases in sexual development and ascospore germination in Sordaria macrospora. Genetics 196, 729–744. doi: 10.1534/genetics.113.159368
Endale H. T., Tesfaye W., Mengstie T. A. (2023). ROS induced lipid peroxidation and their role in ferroptosis. Front. Cell Dev. Biol. 11, 1226044. doi: 10.3389/fcell.2023.1226044
Fisher M. C., Henk D. A., Briggs C. J., Brownstein J. S., Madoff L. C., McCraw S. L., et al. (2012). Emerging fungal threats to animal, plant and ecosystem health. Nature 484, 186–194. doi: 10.1038/nature10947
Forgey W., Blanco M., Loegering W. (1978). Differences in pathological capabilities and host specificity of Colletotrichum graminicola on Zea mays. Plant Dis. Rep. 62, 573–576.
Fu T., Lee N.-H., Shin J.-H., Kim K. S. (2022). NADPH oxidases are required for appressorium-mediated penetration in Colletotrichum scovillei-pepper fruit pathosystem. Plant Pathol. J. 38, 345–354. doi: 10.5423/PPJ.OA.05.2022.0066
Groth A., Schunke C., Reschka E. J., Pöggeler S., Nordzieke D. E. (2021). Tracking fungal growth: Establishment of Arp1 as a marker for polarity establishment and active hyphal growth in filamentous ascomycetes. J. Fungi 7, 580. doi: 10.3390/jof7070580
Guerrieri E., Poppy G., Powell W., Rao R. P. (2002). Plant-to-plant communication mediating in-flight orientation of Aphidius ervi. J. Chem. Ecol. 28, 1703–1715. doi: 10.1023/A:1020553531658
Hagen D. C., Sprague G. F. Jr (1984). Induction of the yeast α-specific STE3 gene by the peptide pheromone a-factor. J. Mol. Biol. 178, 835–852. doi: 10.1016/0022-2836(84)90314-0
Jeandet P., Trotel-Aziz P., Jacquard C., Clement C., Mohan C., Morkunas I., et al. (2023). Use of elicitors and beneficial bacteria to induce and prime the stilbene phytoalexin response: applications to grapevine disease resistance. Agronomy 13, 2225. doi: 10.3390/agronomy13092225
Jenness D. D., Burkholder A. C., Hartwell L. H. (1986). Binding of α-factor pheromone to Saccharomyces cerevisiae a cells: dissociation constant and number of binding sites. Mol. Cell Biol. 6, 318–320. doi: 10.1128/mcb.6.1.318
Khorassani R., Hettwer U., Ratzinger A., Steingrobe B., Karlovsky P., Claassen N. (2011). Citramalic acid and salicylic acid in sugar beet root exudates solubilize soil phosphorus. BMC Plant Biol. 11, 121. doi: 10.1186/1471-2229-11-121
Kilpatrick L. E., Hill S. J. (2021). Transactivation of G protein-coupled receptors (GPCRs) and receptor tyrosine kinases (RTKs): Recent insights using luminescence and fluorescence technologies. Curr. Opin. Endocr. Metab. Res. 16, 102–112. doi: 10.1016/j.coemr.2020.10.003
Lambert A. J., Brand M. D. (2009). “Reactive oxygen species production by mitochondria,” in Stuart J. A. ed. Mitochondrial DNA. Methods in Molecular Biology™. Humana Press. vol 554, 165–181. doi: 10.1007/978-1-59745-521-3_11
Lavania M., Chauhan P. S., Chauhan S., Singh H. B., Nautiyal C. S. (2006). Induction of plant defense enzymes and phenolics by treatment with plant growth–promoting rhizobacteria Serratia marcescens NBRI1213. Curr. Microbiol. 52, 363–368. doi: 10.1007/s00284-005-5578-2
Liu N., Wang W., He C., Luo H., An B., Wang Q. (2022). NADPH Oxidases Play a Role in Pathogenicity via the Regulation of F-Actin Organization in Colletotrichum gloeosporioides. Front. Cell. Infect. Microbiol. 12, 845133. doi: 10.3389/fcimb.2022.845133
Mafu S., Ding Y., Murphy K. M., Yaacoobi O., Addison J. B., Wang Q., et al. (2018). Discovery, biosynthesis and stress-related accumulation of dolabradiene-derived defenses in maize. Plant Physiol. 176, 2677–2690. doi: 10.1104/pp.17.01351
Mendoza L., Espinoza P., Urzua A., Vivanco M., Cotoras M. (2009). In vitro antifungal activity of the diterpenoid 7α-hydroxy-8 (17)-labden-15-oic acid and its derivatives against Botrytis cinerea. Molecules 14, 1966–1979. doi: 10.3390/molecules14061966
Mohamed R., Janke R., Guo W., Cao Y., Zhou Y., Zheng W., et al. (2019). GPCR transactivation signalling in vascular smooth muscle cells: role of NADPH oxidases and reactive oxygen species. Vas. Biol. 1, R1–R11. doi: 10.1530/VB-18-0004
Nakayama N., Miyajima A., Arai K. (1985). Nucleotide sequences of STE2 and STE3, cell type-specific sterile genes from Saccharomyces cerevisiae. EMBO J. 4, 2643–2648. doi: 10.1002/j.1460-2075.1985.tb03982.x
Nordzieke D. E. (2022). Hyphal fusions enable efficient nutrient distribution in colletotrichum graminicola conidiation and symptom development on maize. Microorganisms 10, 1146. doi: 10.3390/microorganisms10061146
Nordzieke D. E., Fernandes T. R., El Ghalid M., Turrà D., Di Pietro A. (2019a). NADPH oxidase regulates chemotropic growth of the fungal pathogen Fusarium oxysporum towards the host plant. New Phytol. 224, 1600–1612. doi: 10.1111/nph.v224.4
Nordzieke D. E., Sanken A., Antelo L., Raschke A., Deising H. B., Pöggeler S. (2019b). Specialized infection strategies of falcate and oval conidia of Colletotrichum graminicola. Fungal Genet. Biol. 133, 103276. doi: 10.1016/j.fgb.2019.103276
O'Connell R. J., Thon M. R., Hacquard S., Amyotte S. G., Kleemann J., Torres M. F., et al. (2012). Lifestyle transitions in plant pathogenic Colletotrichum fungi deciphered by genome and transcriptome analyses. Nat. Genet. 44, 1060–1065. doi: 10.1038/ng.2372
Panaccione D. G., Vaillancourt L. J., Hanau R. M. (1989). Conidial dimorphism in Colletotrichum graminicola. Mycologia 81, 876–883. doi: 10.1080/00275514.1989.12025677
Ramaswe J. B., Steenkamp E. T., De Vos L., Fru F. F., Adegeye O. O., Wingfield B. D. (2024). Sex pheromone receptor Ste2 orchestrates chemotropic growth towards pine root extracts in the pitch canker pathogen Fusarium circinatum. Pathogens 13, 425. doi: 10.3390/pathogens13050425
Roth B. L., Irwin J. J., Shoichet B. K. (2017). Discovery of new GPCR ligands to illuminate new biology. Nat. Chem. Biol. 13, 1143–1151. doi: 10.1038/nchembio.2490
Rudolph A. Y., Schunke C., Sasse C., Antelo L., Gerke J., Braus G., et al. (2024). Maize diterpenoid sensing via Ste3 a-pheromone receptor and rapid germination of Colletotrichum graminicola oval conidia facilitating root infection. bioRxiv. doi: 10.1101/2024.04.05.588234
Ruxton G. D. (2006). The unequal variance t-test is an underused alternative to Student's t-test and the Mann–Whitney U test. Behav. Ecol. 17, 688–690. doi: 10.1093/beheco/ark016
Ryder L. S., Dagdas Y. F., Mentlak T. A., Kershaw M. J., Thornton C. R., Schuster M., et al. (2013). NADPH oxidases regulate septin-mediated cytoskeletal remodeling during plant infection by the rice blast fungus. Proc. Natl. Acad. Sci. U.S.A. 110, 3179–3184. doi: 10.1073/pnas.1217470110
Sagi M., Fluhr R. (2006). Production of reactive oxygen species by plant NADPH oxidases. Plant Physiol. 141, 336–340. doi: 10.1104/pp.106.078089
Saha P., Rahman F. I., Hussain F., Rahman S. A., Rahman M. M. (2022). Antimicrobial diterpenes: Recent development from natural sources. Front. Pharmacol. 12, 820312. doi: 10.3389/fphar.2021.820312
Sasaki K., Iwai T., Hiraga S., Kuroda K., Seo S., Mitsuhara I., et al. (2004). Ten rice peroxidases redundantly respond to multiple stresses including infection with rice blast fungus. Plant Cell Physiol. 45, 1442–1452. doi: 10.1093/pcp/pch165
Schafer A. E., Blaxall B. C. (2017). G protein coupled receptor-mediated transactivation of extracellular proteases. J. Cardiovasc. Pharmacol. 70, 10–15. doi: 10.1097/FJC.0000000000000475
Schmelz E. A., Kaplan F., Huffaker A., Dafoe N. J., Vaughan M. M., Ni X., et al. (2011). Identity, regulation, and activity of inducible diterpenoid phytoalexins in maize. Proc. Natl. Acad. Sci. 108, 5455–5460. doi: 10.1073/pnas.1014714108
Schunke C., Pöggeler S., Nordzieke D. E. (2020). A 3D printed device for easy and reliable quantification of fungal chemotropic growth. Front. Microbiol. 11, 584525. doi: 10.3389/fmicb.2020.584525
Segmüller N., Kokkelink L., Giesbert S., Odinius D., van Kan J., Tudzynski P. (2008). NADPH oxidases are involved in differentiation and pathogenicity in Botrytis cinerea. Mol. Plant-Microbe Interact. 21, 808–819. doi: 10.1094/MPMI-21-6-0808
Sharma T., Sridhar P. S., Blackman C., Foote S. J., Allingham J. S., Subramaniam R., et al. (2022). Fusarium graminearum ste3 G-protein coupled receptor: A mediator of hyphal chemotropism and pathogenesis. mSphere 7, e00456–e00422. doi: 10.1128/msphere.00456-22
Sridhar P. S., Trofimova D., Subramaniam R., González-Peña Fundora D., Foroud N. A., Allingham J. S., et al. (2020). Ste2 receptor-mediated chemotropism of Fusarium graminearum contributes to its pathogenicity against wheat. Sci. Rep. 10, 10770. doi: 10.1038/s41598-020-67597-z
Su L.-J., Zhang J.-H., Gomez H., Murugan R., Hong X., Xu D., et al. (2019). Reactive oxygen species-induced lipid peroxidation in apoptosis, autophagy, and ferroptosis. Oxid. Med. Cell. Longev. 2019, 5080843. doi: 10.1155/2019/5080843
Sukno S. A., Garciía V. N. M., Shaw B. D., Thon M. R. (2008). Root infection and systemic colonization of maize by Colletotrichum graminicola. Appl. Environ. Microbiol. 74, 823–832. doi: 10.1128/AEM.01165-07
Sun Y., Qiao Y., Liu Y., Zhou J., Wang X., Zheng H., et al. (2021). ent-Kaurane diterpenoids induce apoptosis and ferroptosis through targeting redox resetting to overcome cisplatin resistance. Redox Biol. 43, 101977. doi: 10.1016/j.redox.2021.101977
Turra D., El Ghalid M., Rossi F., Di Pietro A. (2015). Fungal pathogen uses sex pheromone receptor for chemotropic sensing of host plant signals. Nature 527, 521–524. doi: 10.1038/nature15516
Vangalis V., Markakis E. A., Knop M., Di Pietro A., Typas M. A., Papaioannou I. A. (2023). Components of TOR and MAP kinase signaling control chemotropism and pathogenicity in the fungal pathogen Verticillium dahliae. Microbiol. Res. 271, 127361. doi: 10.1016/j.micres.2023.127361
Vermot A., Petit-Härtlein I., Smith S. M., Fieschi F. (2021). NADPH oxidases (NOX): an overview from discovery, molecular mechanisms to physiology and pathology. Antioxidants 10, 890. doi: 10.3390/antiox10060890
Vitale S., Di Pietro A., Turra D. (2019). Autocrine pheromone signalling regulates community behaviour in the fungal pathogen Fusarium oxysporum. Nat. Microbiol. 4, 1443–1449. doi: 10.1038/s41564-019-0456-z
Vives-Peris V., De Ollas C., Gomez-Cadenas A., Perez-Clemente R. M. (2020). Root exudates: from plant to rhizosphere and beyond. Plant Cell Rep. 39, 3–17. doi: 10.1007/s00299-019-02447-5
Wang L., Mogg C., Walkowiak S., Joshi M., Subramaniam R. (2014). Characterization of NADPH oxidase genes NoxA and NoxB in Fusarium graminearum. Can. J. Plant Pathol. 36, 12–21. doi: 10.1080/07060661.2013.868370
Xue C., Hsueh Y.-P., Heitman J. (2008). Magnificent seven: roles of G protein-coupled receptors in extracellular sensing in fungi. FEMS Microbiol. Lett. 32, 1010–1032. doi: 10.1111/j.1574-6976.2008.00131.x
Keywords: Colletotichum graminicola, chemotropic growth, root exudates, class III peroxidases, diterpenoids, GPCR, Cell Wall Integrity MAPK pathway, NADPH oxidase
Citation: Rudolph AY, Schunke C and Nordzieke DE (2024) Conserved perception of host and non-host signals via the a-pheromone receptor Ste3 in Colletotrichum graminicola. Front. Fungal Biol. 5:1454633. doi: 10.3389/ffunb.2024.1454633
Received: 25 June 2024; Accepted: 09 September 2024;
Published: 07 October 2024.
Edited by:
David Canovas, Sevilla University, SpainReviewed by:
Katherine A. Borkovich, University of California, Riverside, United StatesBenjamin A. Horwitz, Technion Israel Institute of Technology, Israel
Copyright © 2024 Rudolph, Schunke and Nordzieke. This is an open-access article distributed under the terms of the Creative Commons Attribution License (CC BY). The use, distribution or reproduction in other forums is permitted, provided the original author(s) and the copyright owner(s) are credited and that the original publication in this journal is cited, in accordance with accepted academic practice. No use, distribution or reproduction is permitted which does not comply with these terms.
*Correspondence: Daniela Elisabeth Nordzieke, ZG5vcmR6aUBnd2RnLmRl
†Present address: Carolin Schunke, Heidelberg University Biochemistry Center (BZH), Ruprecht Karls Universität Heidelberg, Heidelberg, Germany