- 1MIGAL – Galilee Research Institute, Plant Sciences Department, Kiryat Shmona, Israel
- 2Faculty of Sciences, Tel-Hai College, Tel Hai, Israel
Magnaporthiopsis maydis is a maize pathogen that causes severe damage to commercial corn fields in the late growth stages. Late wilt disease (LWD) has spread since its discovery in the 1960s in Egypt and is now reported in about 10 countries. The pathogen has a hidden endophytic lifecycle in resistant corn plants and secondary hosts such as green foxtail, watermelon lupin and cotton. At the same time, it could be an opportunist and hinder the host development under the right conditions. This study uncovered M. maydis interactions with newly identified maize endophytes. To this end, six fungi were isolated from the seeds of three sweet corn cultivars having varying susceptibility to LWD. These isolates were identified using colony morphology and microscopic characterization, universal internal transcribed spacer (ITS) molecular targeting and phylogenetic analysis. Most of them belonged to pathogenic species. Compared to three previously identified bioprotective microorganisms, the new species were tested for their ability to secrete metabolites that repress M. maydis in vitro and to antagonize it in a solid media confront test and a seedlings pathogenicity assay. The opportunistic fungal species Aspergillus flavus (ME1), Aspergillus terreus (PE3) and the reference biocontrol bacteria Bacillus subtilis (R2) achieved the highest M. maydis inhibition degree in the plates tests (74-100% inhibition). The seedlings’ pathogenicity assay that predicts the seeds’ microflora resistance to M. maydis highlighted the bio-shielding potential of most species (23% or more epicotyl elongation over the infected control). Fusarium sp. (ME2) was the leading species in this measure (43% enhancement), and B. subtilis gave the best protection in terms of seeds’ germination (50%) and sprouts’ biomass (34%). The results of this study could enhance our understanding of the pathobiome’s role in the context of LWD and represent a first step in using the seeds’ natural protective microflora to develop novel management strategies.
1 Introduction
Maize (corn, Zea mays L.) is considered one of the world’s most influential human food sources and the third leading crop after wheat and rice (Zhao et al., 2017). Additionally, many countries have been growing corn as an essential animal feed source and for other uses. Maize late wilt disease (LWD) has spread since its discovery in the 1960s in Egypt (Sabet et al., 1961; Samra et al., 1962). It has been reported in 10 countries (Degani, 2021), with a higher incidence in Israel (Degani and Gordani, 2022), Egypt (El-Shabrawy and Shehata, 2018), India (Rakesh et al., 2022), Spain (Ortiz-Bustos et al., 2019) and Portugal (Patanita et al., 2020). However, the disease’s prevalence and impact are expected to increase due to global warming (Pecsi and Nemeth, 1998; Degani, 2021).
The pathogen Magnaporthiopsis maydis (former names Cephalosporium maydis and Harpophora maydis) (Samra et al., 1963; Saleh and Leslie, 2004; Luo and Zhang, 2013) causes severe damage to corn fields in the late growth stages. M. maydis is a potentially high-risk, hemibiotroph, seed-borne (Michail et al., 1999) and soil-borne phytopathogen (Tej et al., 2018). It is reported as being an almost asymptomatically endophyte in LWD-resistant corn cultivars (Kumar et al., 2021). It can also survive in secondary host plants such as lupine (Lupinus termis L.) (Sahab et al., 1985), cotton (Gossypium hirsutum) (Sabet et al., 1966), green foxtail (Setaria viridis) and watermelon (Citrullus lanatus) (Dor and Degani, 2019). Yet, it may become pathogenic and cause disease in some alternative hosts under certain conditions (encouraging host and environmental states) (Degani et al., 2022a). Without the host plant, the pathogen can spread and survive through infected soils and crop residues (Sabet et al., 1970).
In susceptible sweet corn hybrids, LWD is characterized by a relatively rapid wilting of the above soil parts that usually occurs 60-80 days from seeding, from before the flowering stage (tasseling) to physiological maturation (Darwesh and Elshahawy, 2023). Dehydration signs start to appear nearly 50 days post-sowing, progressing from the lower stem and leaves to the plant’s upper part, potentially damaging the cobs (Johal et al., 2004). In severe cases, the disease can cause nearly total dehydration and yield loss (El-Naggarr et al., 2015; Degani et al., 2020). Nevertheless, most outbursts of LWD have resulted in 30-50% economic losses (El-Shehawy et al., 2014; Sunitha et al., 2020).
Presently, the primary approach for LWD management is cultivating disease-resistant corn varieties (Kumar et al., 2021). This strategy is economical, eco-friendly and compatible with any growth method but requires constant effort to scan and identify new resistant corn hybrids. Moreover, the continual growth of a specific resistant maize cultivar for several seasons could ultimately lead to a selection pressure that would, in the long run, lead to an outburst of aggressive virulent M. maydis strains capable of causing the disease, as previously reported in Egypt, Spain and Israel (Zeller et al., 2002; García-Carneros et al., 2011; Ortiz-Bustos et al., 2015; Shofman et al., 2022).
Over the years, many strategies and control methods have been tested for LWD. A traditional approach involving chemical pesticides has produced promising results (Singh and Siradhana, 1989; Ghazy et al., 2017; Degani et al., 2018; Degani et al., 2020). Yet, public concern regarding this method’s health and environmental risks encourages searching for more eco-friendly solutions. Moreover, using a single ingredient-based chemical pesticide to protect the crops, as suggested lately (Degani and Gordani, 2022), may lead to fungal resistance development and effectiveness losses (Corkley et al., 2022). Specifically, this concern is regarding Azoxystrobin, an ingredient known to be one of the most effective fungicides to control LWD (Degani et al., 2014; Degani et al., 2019). The reason is that there is a high risk of emerging Azoxystrobin-resistant M. maydis strains as it occurs in other pathogens (Avila-Adame and Koller, 2003; Fernández-Ortuño et al., 2010; Castroagudín et al., 2015).
Therefore, significant research on alternative LWD management has suggested several impactable options, including biological methods (Ghazy and El-Nahrawy, 2020; Degani and Dor, 2021; El-Naggar and Yassin, 2024), agrotechnical methods such as balanced soil fertility (Samra et al., 1966; Singh and Siradhana, 1990) and watering (Singh and Siradhana, 1987a), solar heating (Fayzalla et al., 1994) and allelochemical methods (Tej et al., 2018). One focused research effort addresses the identification of potential antagonistic endophytes in commercial corn hybrids, using them as biocontrol agents to shield plants from the pathogen (Degani et al., 2021).
Obtaining new and effective environmentally-friendly LWD control options is a continuous scientific aim. Specifically, the full potential protective ability of endophytes in the maize plant’s microbiome to resist LWD is yet to be revealed. In addition, the intriguing interactions of M. maydis and other pathogens in the maize’s pathobiome could play a crucial role in the disease outcome and the effectiveness of the control treatments. Plants are threatened by diverse pathogen species living in complex communities that can enhance diseases or oppress one another.
The classic triangle in phytopathology requires the interaction of a susceptible host, a virulent pathogen and an environment favorable for disease development (Francl, 2001). The plant microbiome affects both host resistance and the plants’ close surroundings. These microorganism communities inhabit the plant’s rhizosphere (the roots’ nearby habitat) or phyllosphere (the plant’s aboveground habitat), and comprise opportunistic pathogens and non-pathogenic members that may protect the plant from pathogens (Compant et al., 2019). They are formed by bacteria and fungi inhabiting the same ecological niche and cooperating or competing for the same plant resources. Plant-friendly endophytes can now provide new ways of controlling plant diseases.
Studying the role of maize endophytes in restricting the impact of M. maydis is an essential initial step towards developing a new, environmentally-friendly control interface based on strengthening the plant’s microbiome. The protective partners in the natural microflora of maize seeds can reduce the pathogen’s development and spread within the host roots, improve the plant’s immunity to diseases and enhance growth indices. Seed treatment as a management protocol is considered economically feasible and easily applied, and could be integrated with other practices, regardless of the cultivation and irrigation methods.
In the current study, we hypothesized that fungal and bacterial microflora, natural inhabitants of various maize grains, may positively impact plant health and serve as a fundamental front barrier against the late wilt pathogen. Therefore, non-pathogenic species can be used as bio-pesticides for sustainable agriculture. In contrast, virulence species in the maize seeds’ microbiome can affect LWD in intricate ways. To test this hypothesis, we isolated microorganisms from several maize cultivars’ grains, identified them using colony morphology, microscopic traits and molecular characteristics, and tested them against M. maydis on media plates. Subsequently, microorganisms capable of restricting pathogen growth by direct contact or by secreting antifungal metabolites into the medium were applied to protect seedlings at the sensitive pathogen-invasive phase.
2 Materials and methods
2.1 Preparation of the maize grains
To isolate new endophytes from sweet corn seeds, we choose cultivars varying in susceptibility to LWD. All seeds were commercial and were provided courtesy of the seed companies listed in Table 1. Seeds were pretreated by the companies with Captan (cis-N-trichloromethylthio-4-cyclohexene-1,2-diacarboximide) according to Israel’s Plant Protection and Inspection Services (PPIS) regulations. Before being subjected to endophyte isolation, the grains were rinsed with tap water and soap while stirring 4-5 times and changing the water until the seed coating was washed. The seeds were then disinfected externally using 1% sodium hypochlorite (NaOCl) for 1 min and sterilized twice with 70% ethanol. Subsequently, the seeds were rinsed twice in sterile double-distilled water (DDW). Finally, they were dried in a biological hood on sterile paper for two hours.
2.2 Isolation and identification of endophytes from the maize grains
The endophytes Isolation and identification method was previously described in (Degani et al., 2021). The corn grains were cut lengthwise using a sterile scalpel and placed on potato dextrose agar (PDA; Difco Laboratories, Detroit, MI, USA) growth plates, with the cutting surface downwards. The Petri plates (90-mm-diameter plate with 10 half-seeds each) were incubated at 28 ± 1°C in the dark for 2-3 days. The developed endophytes (fungi and bacteria) were isolated into new PDA plates and grown for 5-6 days under the above conditions. The isolates (ca. 30) were divided into groups having similar colony characteristics. Transfer to new plates was performed for representative isolates from each group, and the process continued until pure colonies were obtained. Each colony was transferred to a new plate by taking a small agar disk from the colony’s edge and closely observing its appearance as it grew.
The mycelial mats or conidia were gently removed from the plates, and a small portion was mixed with 10 µL of either potato dextrose broth (PDB) or DDW. These mixtures were then applied onto sterile glass slides for microscopic examination using a light microscope at a magnification of 400× without staining. The microscopic identification of the species (mainly spores and conidiophores characteristics) was conducted using taxonomic keys.
2.3 Molecular identification of the endophytes
DNA was extracted from the mycelia of PDA-grown endophyte colonies using the Master Pure Yeast DNA Purification Set Kit (Sigma, Rehovot, Israel). Molecular identification by PCR and sequencing was performed by targeting the endophyte’s small subunit ribosomal RNA gene (16s small rRNA gene) using the universal internal transcribed spacer (ITS4 and ITS5) primers (Table 2). PCR was done using the Rapidcycler (Idaho Technology, Salt Lake City, UT, USA) in a total volume of per reaction; of each primer (concentration of ), of commercial reaction mixture RedTaq® ReadyMix (Sigma, Rehovot, Israel), of template DNA and autoclaved DDW. PCR conditions were 94°C for 2 min, 30 rounds of 94°C for 30 sec, 55°C for 30 sec, 72°C for one min, and a finishing step of 72°C for 5 min (Degani et al., 2021). The PCR products were kept at 4°C until use. PCR products were sequenced in the ITS4 forward and ITS5 reverse directions by Hy Labs (Rehovot, Israel). Sequences were used to conduct a homology search against GenBank using the BLASTN tool (National Center for Biotechnology Information, Bethesda, MD, USA, at: http://www.ncbi.nlm.nih.gov, accessed on 15 May 2024). For the Fusarium species identification, the Fusarioid-ID database pairwise alignment (at www.fusarium.org, accessed on 15 May 2024) was used.
Sequence comparison of the ITS gene and phylogenetic tree construction were performed using the SeaView version 5.0 software (http://doua.prabi.fr/software/seaview, accessed on 15 May 2024) (Gouy et al., 2010). Sequences were aligned using the CLUSTALW program (https://www.genome.jp/tools-bin/clustalw, accessed on 15 May 2024), and the similarity percentages between sequences in the phylogenetic tree were constructed using the parsimony DNA-level algorithm (dnapars) method with the default parameters, ignore positions with gaps and bootstrap with 1000 replications. The parsimony score for each tree is the sum of the smallest number of substitutions needed for each site. The method assumes that the tree with the lowest parsimony score, the simplest of the set, is most likely to be correct. The ITS genes from reference strains found in a previous study (Degani et al., 2021) and others selected from the GenBank were incorporated into the analysis to assist the taxonomic assignment.
2.4 Endophytes’ metabolites assay
In a non-indirect method, the growth restriction of M. maydis was tested using endophyte-secreted metabolites (Degani and Dor, 2021). Ten 6-mm-diameter agar disks were cut from the culture margins of each endophyte isolate colony (grown for 4-6 days at 28 ± 1°C in the dark) and added to 150 mL sterile potato dextrose broth (PDB) in a 250 mL Erlenmeyer flask. Cultures were incubated at 28 ± 1°C in a rotary shaker at 150 rpm for six days in the dark. The liquid medium of each endophyte culture was filtered through two Whatman No.1 filter papers using a Büchner funnel. To prepare endophytes’ extrolites-based solid growth medium, 5.85 grams of PDA powder was added to a 300 mL bottle, mixed with each filtrated liquid growth medium, and the pH was adjusted to 5-5.3 with NaOH (PDB pH value). The liquid medium was autoclave sterilized for 30 min. Then 25 mL was poured into a 90 mm-diameter Petri dish (five repeats for each endophyte isolate) and dried in a biological hood for 24 h. M. maydis 6-mm-diameter agar disks were cut from a culture’s margins, and one colony agar disk was placed on each dish. PDA plates without extrolites were used as a control.
2.5 Plates’ confrontation assay
Selected endophytes were identified by their biocontrol potential to restrict M. maydis growth in a direct confront test according to (Degani et al., 2021). Such potential includes their antifungal compounds’ secretion, direct hyphae contact growth inhibition, or the ability of the endophyte to grow on top of the M. maydis colony surface. The mycoparasitism test was performed by placing endophyte colony agar disks (6 mm in diameter cut from culture margins) on a 90-mm-diameter PDA culture Petri dish in front of similar disks from M. maydis. Plates were labeled and incubated at 28 ± 1°C in the dark. The interactions between M. maydis and each isolated endophyte were documented and photographed after seven days. Endophytes that managed to restrict M. maydis growth were marked as having a microparasitic potential. Each endophyte was tested in five repeats.
2.6 Percentage of inhibition of M. maydis
After six days of growth, the inhibition for the metabolites assay experiment (Section 2.4) was calculated using (%I) = [((A+B)/2)x100/R], where R = radial growth of the control; A+B = radial growth of treatment. The percentage of M. maydis inhibition for the confrontation assay experiment (Section 2.5) was calculated using (%I) = [(R-DM)x100/R], where R = radial growth of the control; DM = radial growth of the treatment (Chagas et al., 2022). The isolated endophyte with a high %I was considered an efficient antagonist toward the pathogen M. maydis.
2.7 Seedings pathogenicity assay
Endophytes-treated maize seeds were tested in a pathogenicity assay to evaluate the level of M. maydis bio-shielding (as in (Degani et al., 2021)). The sweet corn Prelude cv. seeds were rinsed in DDW, soaked in 1% NaOCl for 1 min, and then rinsed twice with DDW. The endophytes’ cultures were grown in a liquid PDB medium, as described in Section 2.4. The cultures were ground in a blender for 2 minutes to obtain a blend of short mycelial segments. This mycelia and growth medium combination was used to prepare the seed-coating suspension. Sixty seeds were then soaked for 15 min in the growth medium of each endophyte culture (150 mL) mixed with 7 ml of Tween 80. Ten treated grains were transferred to a 90 mm-diameter Petri dish in which sterile Whatman paper was soaked in 5 ml DDW water. A 6-mm-diameter agar disk of M. maydis (grown previously on PDA in the dark at 28 ± 1°C) was added to each plate center. A sterile 6-mm-diameter PDA disk was added to the control group. Three ml DDW was added to each plate every three days to maintain moisture and to allow efficient seed germination and pathogen inoculation. After six days of incubation at 28 ± 1°C in the dark, the seeds were photographed, and their germination percentages, epicotyl length and fresh biomass were assessed.
2.8 Statistical analysis
The data from the in vitro plates and seedlings pathogenicity assays were analyzed using Microsoft Excel (version 2401 Build 16.0.17231.20290) and GraphPad Prism software (version 9.5.1.733, GraphPad Software Inc., San Diego, CA, USA). Statistical analysis included a Shapiro-Wilk normality test followed by a one-way analysis of variance (ANOVA) and a post-doc of Dunnett’s test (which compares experimental groups to a single control group) or the Fisher’s Least Significant Difference (LSD) test, and at a significance level of p < 0.05.
3 Results
The current work aimed at isolating and identifying maize seeds’ endophytes and exploring their bioprotective potential against the late wilt (LWD) pathogen M. maydis. First, six fungal species were isolated and identified molecularly (Figure 1; Table 3). These selected endophytes belong to the genus Aspergillus, Fusarium, Mucor and Cladosporium. The homology search using the BLASTN and Fusarioid-ID tools resulted in 95.30-100% similarity to GenBank species. The isolates’ identity (confirmed by colony morphology and microscopic traits) was verified by phylogenetic analysis (Figure 2; Supplementary Figure S2). The phylogenetic tree consists of all maize seeds’ endophyte species identified in Israel so far and provides a more comprehensive understanding of these endophytes’ communities. It may be drawn from the analysis that the species composition is usually not linked to a specific maize cultivar. One exception is the Chetomium species, which was isolated from Megaton seeds (a highly LWD susceptible genotype). The endophytes’ population is divided into two major branches. The first includes two sub-branches: the Rhizopus and Alternaria sub-branch; and the Magnaporthiopsis, Trichoderma and Chetomium sub-branch. The second consists of Mucor circinelloides and Macrophomina phaseolina, which are separate from the Penicillium and Aspergillus species group and the Cladosporium and Fusarium spp. group.
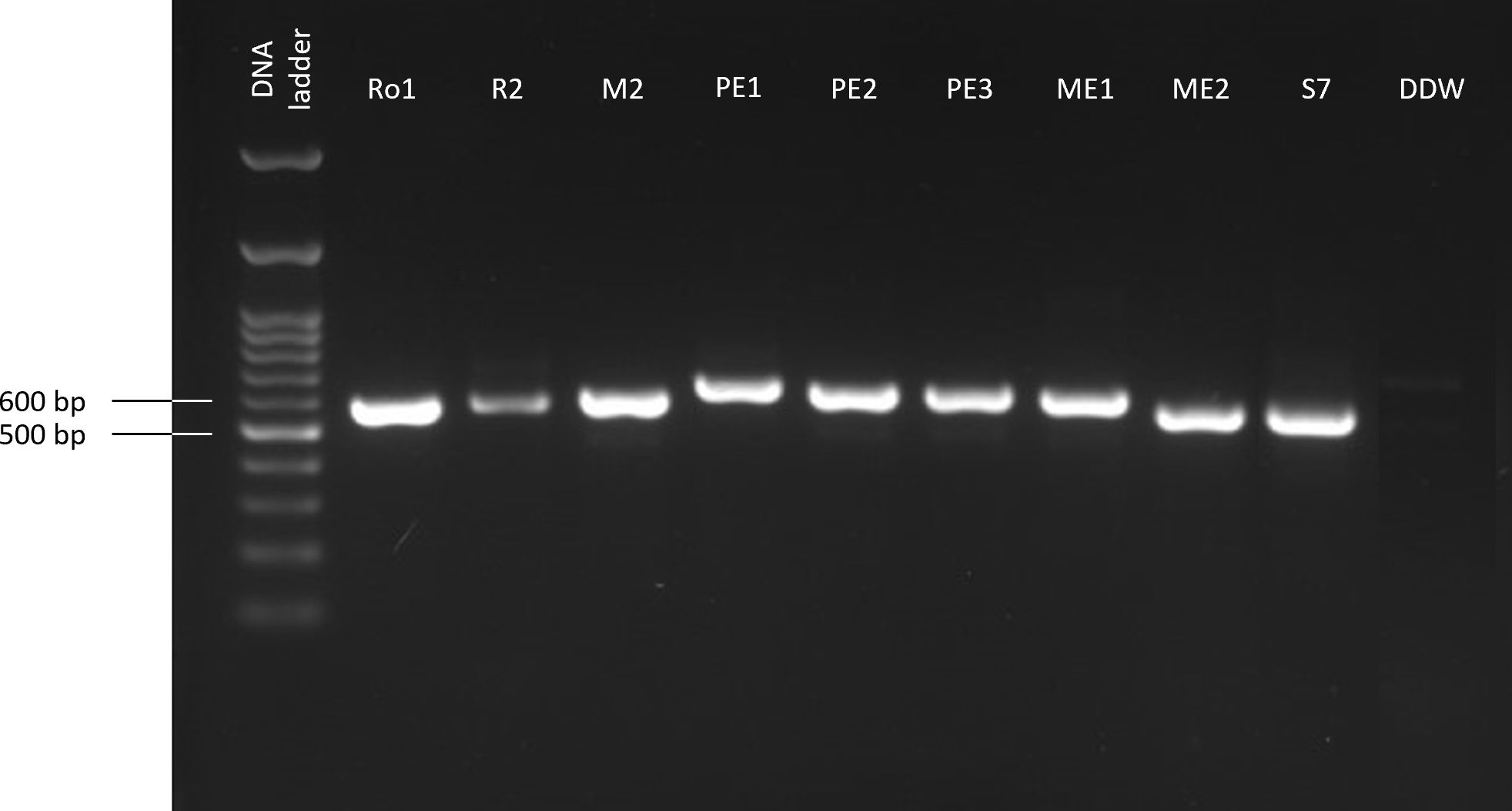
Figure 1 PCR-based molecular identification of the maize seeds’ endophytes. The gel presented is cropped and rearranged to improve the presentation’s clarity and conciseness. The full-length gel is presented in Supplementary Figure S1. The amplified product (ITS, targeting the small subunit ribosomal RNA gene using the universal internal transcribed spacer) was detected by gel electrophoresis. The negative control (DDW) includes sterile twice-distilled water instead of the DNA template.
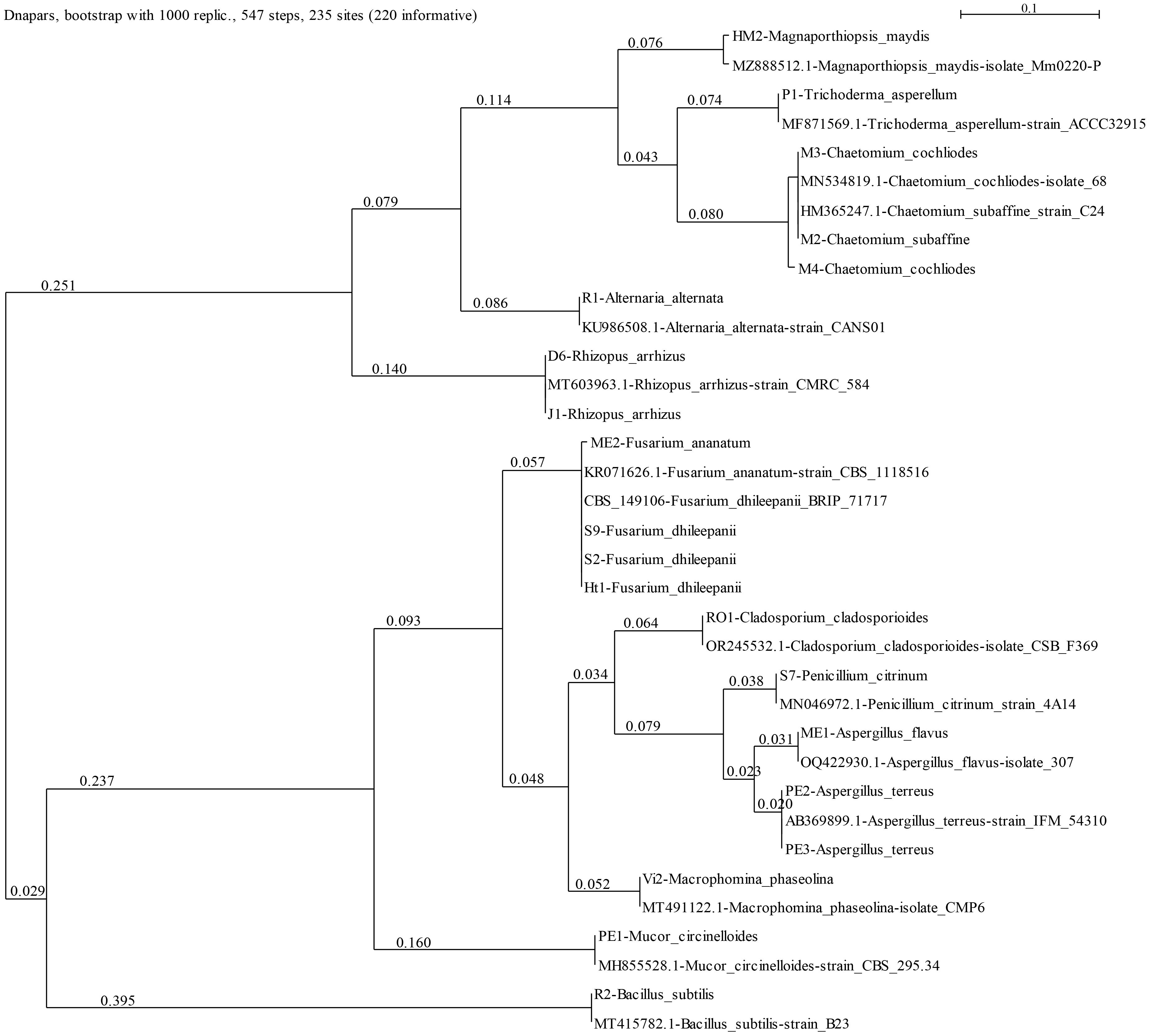
Figure 2 Phylogenetic analysis of the ITS4 gene of the endophytes’ isolates (presented in Table 3). The SeaView version 5.05 program (http://doua.prabi.fr/software/seaview, accessed on 15 May 2024) generated the phylogenetic tree using the parsimony DNA-level algorithm (dnapars)-based method with the default parameters and bootstrap with 1000 replications. The analysis contains the ITS gene from reference strains from a previous work (Degani et al., 2021) to present all maize seeds’ endophyte species identified in Israel so far and others selected from the GenBank to assist the taxonomic assignment. These can be identified by their NCBI accession number. The scale describes the genetic resemblance percentages between sequences. The statistical measures (bootstrap support and posterior probability) for each node are displayed.
The newly identified endophyte species’ bioprotective potential was tested compared to three previously recognized species (Degani et al., 2021) by assessing their secreted metabolites’ inhibition capability and direct confront antagonism against M. maydis (Table 3). These tests are presented in detail below.
The non-directed method assesses the antagonism derived from the secretion of antipathogenic compounds that block M. maydis colony growth. Summarizing the qualitative results (Figure 3) revealed that four of the isolates were able to repress the pathogen through the secretion of such metabolites into the growth medium: the bacteria Bacillus subtilis (R2) and the three fungal colonists – Aspergillus terreus (PE2, PE3), and Aspergillus flavus (ME1). Specifically, one species, A. terreus (PE3), excelled in this test and completely inhibited the pathogen. In contrast, in the presence of Cladosporium sp. (RO1) extrolites, M. maydis grows without apparent interference.
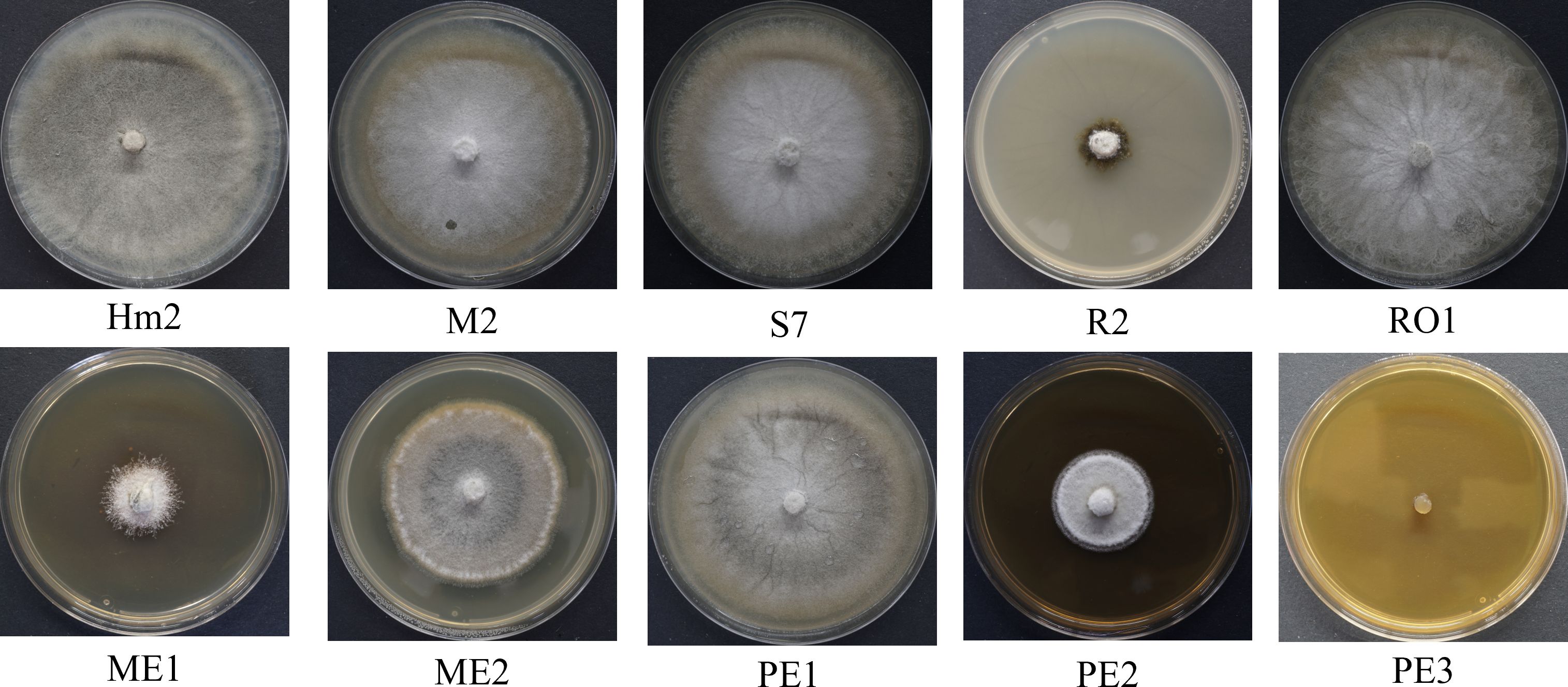
Figure 3 Endophyte’s secreted metabolites inhibition assay to identify M. maydis antagonism. This non-directed method identifies the interaction between the pathogen M. maydis and selected endophytes listed in Table 3. The control was an M. maydis (Hm2 isolate) culture grown on a regular PDA medium (without extrolites). Growth inhibition of M. maydis after six days of incubation indicates the endophyte secretion antifungal properties. Each photo represents five repeats for each seed colonist’s assay.
On solid media, after six days, the seed colonists M. maydis confrontation assay results (Figure 4) supported the above results. This examination revealed that some endophytes could restrict M. maydis colony growth by secreting their extrolites (for example, S7, R2, PE2, PE3) or by physical hypha-hypha contact (for instance, Chaetomium cochliodes, M2). One isolate, Mucor circinelloides (PE1), grew above the M. maydis colony and inhibited its growth. Unexpectedly, this species was unsuccessful in the metabolites assay (Figure 3). In contrast, some isolates such as RO1 were poor M. maydis antagonists in both assays. Quantitative analysis of both the extrolites and confront experiments is presented in Figure 5. The highest inhibition scores (%I) were for Aspergillus terreus (PE3) in the metabolites assay and Mucor circinelloides (PE1) in the mycoparasitism experiment. An intriguing result regards S7, which was unsuccessful in the metabolites assay but showed strong non-direct antagonism in plate confrontation against M. maydis. Bio-protective species that excel in both trials (p < 0.005) are ME1 (A. flavus), ME2 (Fusarium sp.), PE2, PE3 (A. terreus), and the control species R2 (B. subtilis) and M2 (C. cochliodes). The newly discovered species were further examined in the seedlings’ pathogenicity assay.
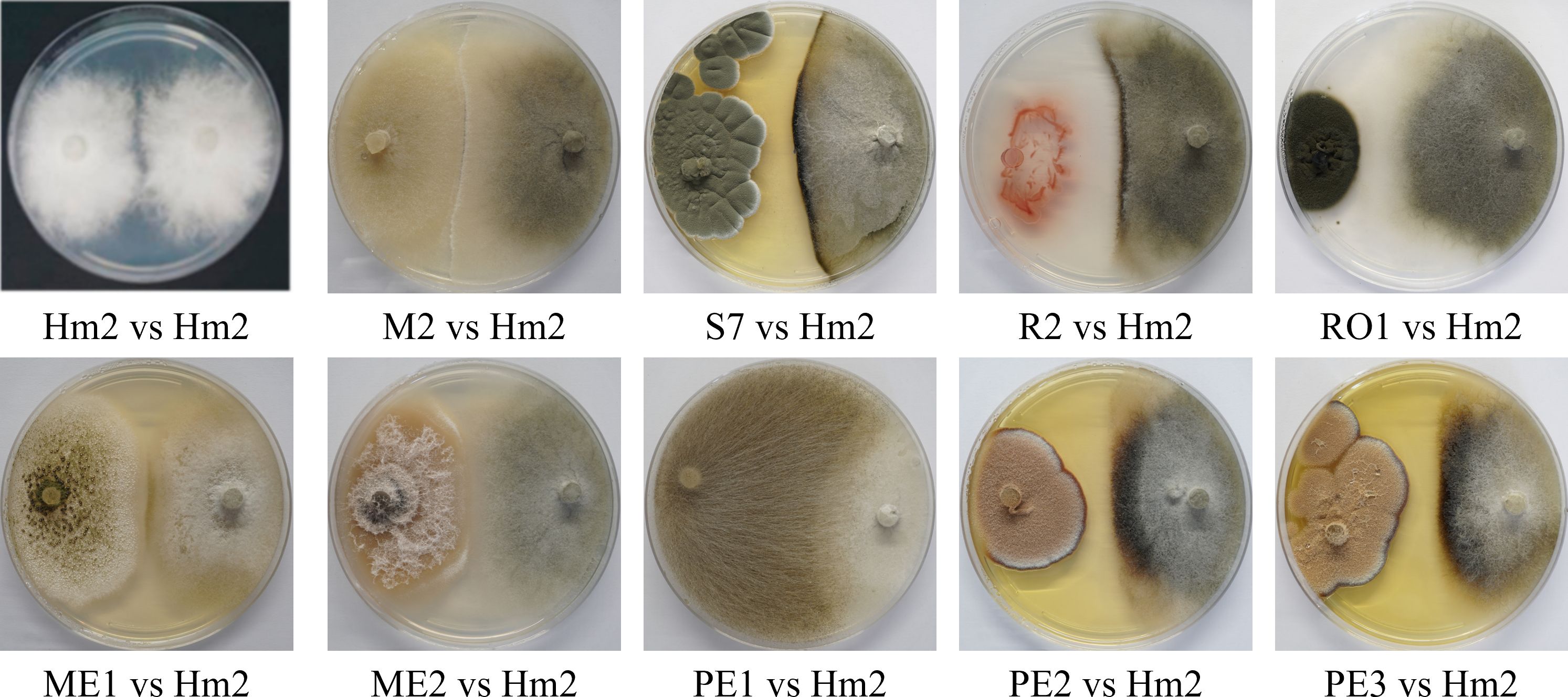
Figure 4 Plate confrontation assay to identify M. maydis antagonism. The PDA plate mycoparasitism assay after seven days of incubation was used as a direct way to identify interactions between M. maydis and selected endophytes studied here. Tested microorganisms are listed in Table 3. The control was a growth medium where the pathogen M. maydis seeded on both poles (Hm2 vs. Hm2). Each photo represents five repeats for each confrontation assay. Species that managed to restrict M. maydis growth were marked as having microparasitic potential (grow above M. maydis mycelium or inhibit it by hypha contact or by creating antifungal compounds). In weak antagonists, as can be seen for example in RO1 (on the left) vs. Hm2 (on the right), the M. maydis’s mycelium covered almost the entire dish.
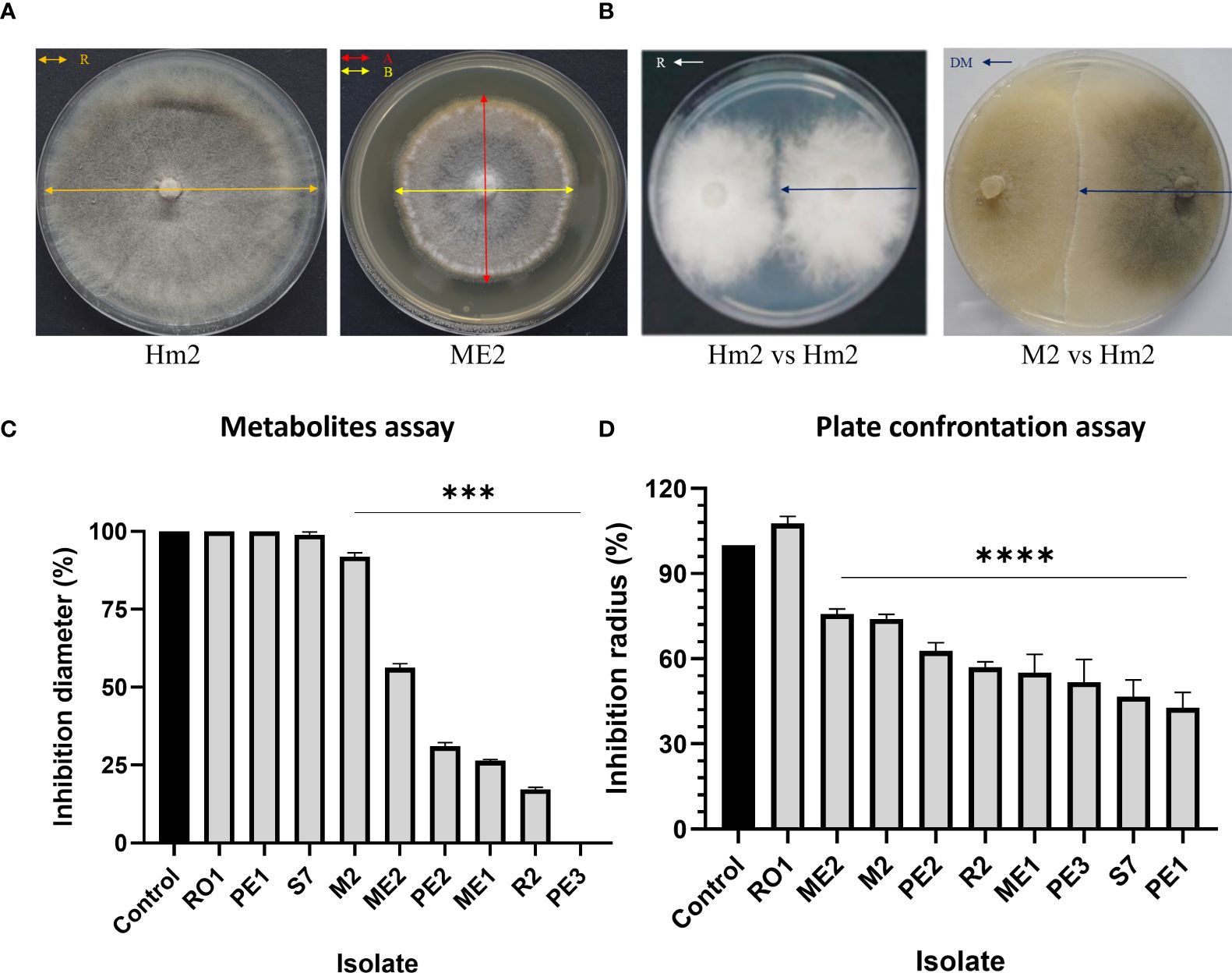
Figure 5 Percentage of inhibition of M. maydis (%I) in the metabolites (Figure 3) and plate confrontation (Figure 4) assays. The following calculation measured M. maydis’ inhibition (A, B). For the metabolites assay (C): (%I) = [((A+B)/2)x100/R], where R = radial growth of the control; A+B = radial growth of treatment. For the mycoparasitism experiment (D), the calculation is (%I) = [(R-DM) x100/R], where R = radial growth of the control; DM = radial expansion of treatment (Chagas et al., 2022). The vertical upper bars represent the standard error of the mean of the 5-6 replicates. Asterisks indicate a significant difference from the control [p < 0.0005 (***), < 0.00005 (****)].
The seeds’ endophytes that passed both the metabolites and the confront antagonism tests were selected for a seedling pathogenicity assessment. The germination values of the LWD susceptible sweet maize Prelude cv. seeds enriched with the protective endophytes were measured under the pathogen stress in Petri dishes (Figure 6). While statistical significance could only be reached compared to the non-infected control, all the endophytes studied (except ME1) enhanced the germinating seeds’ epicotyl elongation (23% or more), with Fusarium sp. (ME2) being the most successful (reaching 43% enhancement over the infected control). Also, B. subtilis (R2) evidently promoted the seeds’ germination (by 50%) and the sprouts’ biomass (by 34%) compared to the infected control. In contrast, other species (particularly A. terreus, PE2) caused some decrease in those measures (up to 50% and 41% reduction compared to this control).
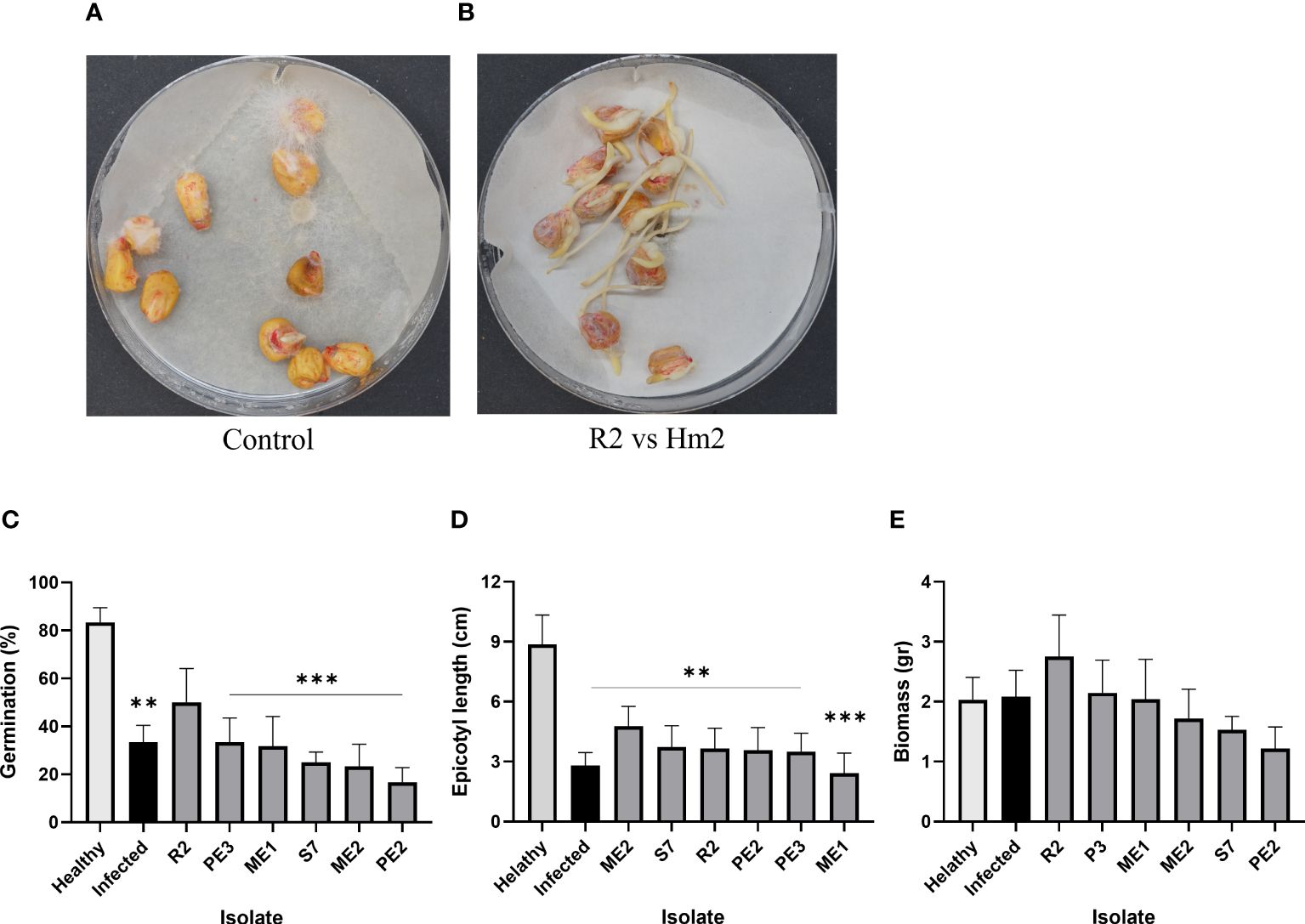
Figure 6 Seedlings pathogenicity assay. The inhibition influence of M. maydis on the seeds’ germination and the sprouts’ first development in vitro. The seeds were enriched by the endophytes’ secreted metabolites (15 min incubation in their growth fluid). (A) The control plate included M. maydis stressed seeds (Infected) and mock uninfected seeds (Healthy); (B) representative plate of B subtilis (R2) versus M. maydis (Hm2). Six endophytes were added separately to the seeds in vitro. Seeds germination percentage (C), epicotyl length (D) and sprouts’ fresh biomass (E) were measured six days after incubation at 28 ± 1°C in the dark. Vertical upper bars represent the standard error of the mean of six replicates (Petri dishes, each containing 10 seeds). Asterisks indicate a significant difference from the mock control [p < 0.005 (**), < 0.0005 (***)].
4 Discussion
Late wilt disease (LWD), caused by the fungus Magnaporthiopsis maydis, threatens commercial maize production in high-risk areas. Searching for control options against the pathogen is one of the top priorities in Israel, Egypt and other countries. While disease-resistant maize genotypes can reduce yield loss, aggressive variants of the fungus can overcome host resistance (Ortiz-Bustos et al., 2015; Shofman et al., 2022). Among alternative ways to control LWD, one pioneering method is to isolate, identify and study the maize seeds’ microflora bioprotective potential against LWD (Degani et al., 2021). This study aimed at expanding this research direction and enriching our understanding of the maize seeds’ natural micro-inhabitants. The results may uncover the poorly understood pathogen-pathogen crosstalk that affects LWD and assist in developing an eco-friendly method based on strengthening the bio-shielding members in those communities and, thus, the seeds’ immunity to LWD.
The first indication of endophytes-M. maydis interactions may be drawn from the plate’s confrontation assay. A typical response between two species that inhibit each other’s growth on an artificial rich solid medium is the formation of a dark border line between them (Mallett and Hiratsuka, 1986). The appearance of hyphal granules, dark gel-like structures, and vacuoles observed in fungal interactions may indicate cell death due to mycoparasitism or nutrient deprivation due to competitive interactions. A brown-black line demarking the combating fungal strains could be linked with melanin, 1,8-dihydroxynaphthalene (DHN), a defense against environmental stresses (Krause et al., 2020). For example, it was reported that in Armillaria mellea, melanized hyphal cells derived from different fungal species of complex constituted the black line (Mallett and Hiratsuka, 1986). This demarcation area was flanked on each side by vesicular cells forming the pseudosclerotial plates characteristic of each respective species.
In this study, such a dark border line was formed between M. maydis and P. citrinum (S7) and B. subtilis (R2), implying antagonism between these two fungi and the maize pathogen. Both endophytes have been well-studied for their ability to produce fungal-inhibiting secretions and promote plant growth. The fungus P. citrinum is commonly found in plants such as soybean and wheat. It produces mycotoxin citrinin, digestive enzymes (cellulase, endoglucanase and xylulase), and plant growth hormones (as summarized by (Khan et al., 2008)). The Bacillus species produces various antifungal compounds that suppress or kill fungal pathogens, making these bacteria popular for the biocontrol of plant diseases (Wu et al., 2015). These compounds include non-ribosomal cyclic lipopeptides and volatile organic compounds (VOCs) having strong antifungal activities (Zhang et al., 2020). In line with this, the secreted metabolites assay results show that B. subtilis (R2) extrolites strongly inhibit M. maydis growth.
Indeed, an important endophytic bacterial species that has been studied extensively is B. subtilis (Gond et al., 2015). This bacterial genus is commonly found in the seeds of various maize varieties and can be transmitted vertically from one plant generation to the next, similar to other endophytes (Rodriguez et al., 2009). This suggests that endophytes play a crucial role in the survival of their host plants. These findings support the idea that LWD-resistant maize genotypes acquire and inherit endophytes, which significantly enhance their immunity to the M. maydis pathogen.
Indeed, the identified partners in maize seeds’ natural microflora, such as B. subtilis reported here, can resist and even reduce the pathogen’s development and spread inside the host roots. Besides their antifungal properties, bacteria can live within the plants as symbiotic endophytes, such as Bacillus amyloliquifaciens and B. subtilis, which are naturally found in many maize varieties. Research indicates that these bacteria can help protect their host plants by producing antifungal lipopeptides such as subtilomycin (Deng et al., 2019). These compounds inhibit pathogens and trigger the activation of the plant’s pathogenesis-related genes, enhancing the plant’s systemic acquired resistance (Gond et al., 2015). Specifically, a mixture of B. subtilis and Pseudomonas koreensis produced siderophores and exhibited antagonistic activity against M. maydis (Ghazy and El-Nahrawy, 2020). Additionally, this combination prevented both pre-emergence and post-emergence damping-off and promoted plant growth under greenhouse conditions. The treatment was also highly effective in field trials, reducing infections and increasing crop yield (Ghazy and El-Nahrawy, 2020).
The results of this study support global efforts and are promoting the use of beneficial maize endophytes as a bio-barrier and protective shield against the LWD fungus. Similar to the current study, seed treatments using bio-control formulations (B. subtilis, Bacillus pumilus, Pseudomonas fluorescens, Epicoccum nigrum) have been recommended for controlling maize LWD and have shown promising results in field tests (Hamza et al., 2013). These treatments were applied over two seasons and successfully reduced the impact of M. maydis on pre-emergence damping-off, disease incidence and crop yield. In another study (El-Mehalowy et al., 2004), rhizosphere actinomycetes (Streptomyces graminofaciens, S. rochei, S. annulatus, S. gibsonii), yeasts (Candida glabrata, C. maltosa, C. slooffii) and the fungus Rhodotorula rubra significantly inhibited the growth of M. maydis in vitro and in seed treatments under controlled greenhouse conditions. When these species were applied without the LWD pathogen, they significantly improved maize plant growth parameters.
Another potential bio-shielding fungus is P. citrinum – a seed-borne protective fungal endophyte having a worldwide distribution (Ahmad et al., 2010b; Goko et al., 2021) and a producer of a wide range of fungal extrolites. Currently, P. citrinum is being explored for its production of secondary metabolites and their associated benefits on plant growth promotion (e.g., secreted gibberellin (Khan et al., 2008)) and competitive antifungals (Wu et al., 2016). P. citrinum secondary metabolites are also being studied as potent molecules for drug development (Sahu et al., 2022). P. citrinum, among many extrolites, produces the lactone antifungal HMG-CoA reductase inhibitors mevastatin and citrinin (Ahmad et al., 2010a). Thus, testing these compounds’ biocontrol potential against the LWD pathogen would be very interesting and valuable.
Despite P. citrinum (S7) metabolites’ strong impact in the confront test (Figure 4), it unexpectedly failed to antagonize M. maydis growth in the metabolites assay (Figure 3). This result is probably affected by the assay protocol, which involved autoclave sterilization of a PDA medium consisting of P. citrinum growth medium instead of water. This procedure destroys the structure of proteins and other heat-sensitive compounds that may have antifungal potential. Yet, some antifungal compounds produced by the seeds’ endophytes are heat-stable. One such example is Fusarium sp. (ME2).
It is well known that Fusarium spp. produces toxins, the most prominent of which are fumonisins, aflatoxins, ochratoxin A, zearalenone, DON, and T-2 and HT-2 toxins, which are frequently co-contaminants in maize seed (Tarazona et al., 2020). One illustration of their importance in pathogen-pathogen interactions is a study dedicated to kernels and in vitro evaluation (Lanubile et al., 2021). The work indicated that F. verticillioides-Aspergillus flavus interactions in maize resulted in reduced fumonisins and aflatoxins biosynthetic gene expression profiles. Such interactions could exist here and should be explored more in future studies.
The most potent M. maydis antagonistic fungal species identified here (excelling in both the metabolites and the confrontation trials) are Aspergillus terreus (PE3) and Aspergillus flavus (ME1). Like Penicillium spp., Aspergillus spp. represents the most chemically examined fungal group with hundreds of biologically active secondary metabolites (Liu and Versicoamides, 2017). A. terreus is a significant saprophytic and endophytic filamentous fungus, producing a wide variety of bioactive secondary metabolites (Amr et al., 2023). It was proposed for use as biocontrol of Rhizoctonia solani, the causal agent of Phaseolus vulgaris and Vicia faba damping-off disease (Abdelaziz et al., 2023). This fungal species and some other endophytes identified here may also be dormant pathogens (opportunists) waiting for the proper condition to evoke their attack on the host (Samson et al., 2011). On the other hand, A. terreus was found to be a plant growth promoter after its inoculation in rice and maize plants in vivo and in vitro (Javed et al., 2020). Thus, as reported here, the A. terreus (PE3) inhibition ability against M. maydis makes it a good candidate for LWD bio-friendly control.
It is important to note that many endophytes use multiple methods to combat invasive pathogens. They can directly inhibit the pathogen’s growth and also trigger the plant’s systemic defense response (Gao et al., 2010). Research into the microbial communities, both pathogenic and non-pathogenic, associated with maize could enhance crop management and yield under these threats. All maize plants in natural environments contain seed-vectored endophytes, which may affect the resistance of maize cultivars to late wilt disease (LWD). Differences in resistance between susceptible and resistant LWD maize cultivars may stem from variations in their endophytic communities.
Finally, the seeding pathogenicity assay adjusted and used in the current study is a rapid research tool for evaluating the seeds’ microflora protective members’ ability to prevent pathogens’ penetration and establishment stages. This stage is essential to rule out inefficient antagonist microorganisms, focusing research efforts on more promising ones. The results of the current study are motivating us to expand our knowledge on this subject to uncover the full potential of the maize microbiome in helping the plant survive against pathogens. Understanding these interactions under natural conditions will help us grasp (and potentially influence) the long-term effects of excluding endophyte-based biocontrol methods.
5 Conclusion
This study focused on understanding the endophytes’ role in seeds’ acquired resistance to late wilt disease (LWD). Most microorganism species isolated from maize grains and identified here had an antagonistic effect against the LWD pathogen Magnaporthiopsis maydis in vitro (extrolites and confrontation assays). The bacteria B. subtilis (R2) was the best protective endophyte in terms of seeds’ germination (50%) and sprouts’ biomass (34%). The maize cultivar’s resistance/susceptibility to the disease may be related to the endophyte colonizing it. Indeed, the most successful species in the confrontation and seedlings pathogenicity assays, A. terreus (PE2, PE3), A. flavus (ME1) and B. subtilis, were isolated from the most LWD-susceptible maize cultivars. Still, the possible link between the maize cultivar LWD susceptibility and the bioprotective potential of its endophytes community members should be clarified in future studies. Also, studying the endophytes’ impact on M. maydis during the later growth stages is critical. The results of this study are encouraging us to deepen and widen our understanding of this subject matter to uncover the maize microbiome’s role in the plant survival struggle against pathogens. Such future research directions may include studying the endophytes’ action mechanism involved in pathogen repression, interactions between the seeds’ microflora colonizers, and host physiology and environmental factors that affect the seeds’ microbiome composition and function. Exploring these interactions under natural conditions could help us manipulate and influence the consequences of endophyte-based biocontrol.
Data availability statement
The original contributions presented in the study are publicly available. Data generated by DNA sequencing were deposited in the NCBI repository. GenBank accession numbers for the nucleotide sequences: HM2 Magnaporthiopsis maydis PP794674, Ht1 Fusarium dhileepanii PP794675, S2 Fusarium dhileepanii PP794676, S7 Penicillium citrinum PP794677, S9 Fusarium dhileepanii PP794678, R2 Bacillus subtilis PP811660, Vi2 Macrophomina phaseolina PP794679, D6 Rhizopus arrhizus PP794680, J1 Rhizopus arrhizus PP794681, P1 Trichoderma asperellum PP794682, M2 Chaetomium subaffine PP794683, M3 Chaetomium cochliodes PP794684, M4 Chaetomium cochliodes PP794685, R1 Alternaria alternata PP794686, ME1 Aspergillus flavus PP794687, ME2 Fusarium ananatum PP794688, PE1 Mucor circinelloides PP794689, PE2 Aspergillus terreus PP794690, PE3 Aspergillus terreusPP794691, RO1 Cladosporium cladosporioides PP794692. All other data generated or analyzed during this study are included in this published article (and its Supplementary Information Files).
Author contributions
OD: Writing – review & editing, Writing – original draft, Visualization, Validation, Supervision, Resources, Project administration, Methodology, Investigation, Funding acquisition, Formal analysis, Data curation, Conceptualization. AA: Data curation, Formal analysis, Writing – review & editing, Visualization, Validation, Methodology, Investigation. ED: Writing – review & editing, Visualization, Validation, Methodology, Investigation, Formal analysis, Data curation, Conceptualization. AG: Conceptualization, Data curation, Formal analysis, Investigation, Methodology, Validation, Visualization, Writing – review & editing.
Funding
The author(s) declare financial support was received for the research, authorship, and/or publication of this article. This work was supported by a one-year research grant (2023) from the Israel Field Crops Cultivation (FALCHA) Workers’ Organization.
Acknowledgments
We would like to thank Galia Shofman (MIGAL – Galilee Research Institute and Tel-Hai College, Israel) for her wise advice.
Conflict of interest
The authors declare that the research was conducted in the absence of any commercial or financial relationships that could be construed as a potential conflict of interest.
The author(s) declared that they were an editorial board member of Frontiers, at the time of submission. This had no impact on the peer review process and the final decision.
Publisher’s note
All claims expressed in this article are solely those of the authors and do not necessarily represent those of their affiliated organizations, or those of the publisher, the editors and the reviewers. Any product that may be evaluated in this article, or claim that may be made by its manufacturer, is not guaranteed or endorsed by the publisher.
Supplementary material
The Supplementary Material for this article can be found online at: https://www.frontiersin.org/articles/10.3389/ffunb.2024.1436759/full#supplementary-material
References
Abdelaziz A. M., El-Wakil D. A., Hashem A. H., Al-Askar A. A., AbdElgawad H., Attia M. S. (2023). Efficient role of endophytic aspergillus terreus in biocontrol of Rhizoctonia solani causing damping-off disease of Phaseolus vulgaris and Vicia faba. Microorganisms 11, 1487. doi: 10.3390/microorganisms11061487
Ahmad A., Mujeeb M., Panda B. (2010a). An HPTLC method for the simultaneous analysis of compactin and citrinin in Penicillium citrinum fermentation broth. J. Planar Chromatography-Modern TLC 23, 282–285. doi: 10.1556/JPC.23.2010.4.8
Ahmad A., Panda B. P., Mujeeb M. (2010b). Screening of nutrient parameters for mevastatin production by Penicillium citrinum MTCC 1256 under submerged fermentation using the Plackett-Burman design. J. Pharm. Bioallied Sci. 2, 44. doi: 10.4103/0975-7406.62709
Amr K., Ibrahim N., Elissawy A. M., Singab A. N. B. (2023). Unearthing the fungal endophyte Aspergillus terreus for chemodiversity and medicinal prospects: a comprehensive review. Fungal Biol. Biotechnol. 10, 6. doi: 10.1186/s40694-023-00153-2
Avila-Adame C., Koller W. (2003). Characterization of spontaneous mutants of Magnaporthe grisea expressing stable resistance to the Qo-inhibiting fungicide azoxystrobin. Curr. Genet. 42, 332–338. doi: 10.1007/s00294-002-0356-1
Castroagudín V. L., Ceresini P. C., de Oliveira S. C., Reges J. T., Maciel J. L., Bonato A. L., et al. (2015). Resistance to QoI fungicides is widespread in Brazilian populations of the wheat blast pathogen Magnaporthe oryzae. Phytopathology 105, 284–294. doi: 10.1094/PHYTO-06-14-0184-R
Chagas R. S., Martins L. F. B., Souza A. L. L., Luz M. C., Gomes L. L., Junior F. L., et al. (2022). Trichoderma in the phytopathogenic biocontrol. Bulg. J. Agric. Sci. 28 (4).
Compant S., Samad A., Faist H., Sessitsch A. (2019). A review on the plant microbiome: ecology, functions, and emerging trends in microbial application. J. Adv. Res. 19, 29–37. doi: 10.1016/j.jare.2019.03.004
Corkley I., Fraaije B., Hawkins N. (2022). Fungicide resistance management: maximizing the effective life of plant protection products. Plant Pathol. 71, 150–169. doi: 10.1111/ppa.13467
Darwesh O. M., Elshahawy I. E. (2023). Management of sunflower charcoal-rot and maize late-wilt diseases using the aqueous extract of vermicompost (vermitea) and environmental-safe biochar derivative (wood vinegar). Sci. Rep. 13, 17387. doi: 10.1038/s41598-023-43974-2
Degani O. (2021). A review: late wilt of maize—the pathogen, the disease, current status and future perspective. J. Fungi 7, 989. doi: 10.3390/jof7110989
Degani O., Becher P., Gordani A. (2022a). Pathogenic interactions between Macrophomina phaseolina and Magnaporthiopsis maydis in mutually infected cotton sprouts. Agriculture 12, 255. doi: 10.3390/agriculture12020255
Degani O., Danielle R., Dor S. (2021). The microflora of maize grains as a biological barrier against the late wilt causal agent. Magnaporthiopsis maydis. Agron. 11, 965. doi: 10.3390/agronomy11050965
Degani O., Dor S. (2021). Trichoderma biological control to protect sensitive maize hybrids against late wilt disease in the field. J. Fungi 7, 315. doi: 10.3390/jof7040315
Degani O., Dor S., Fraidman E., Rabinovitz O., Graph S. (2020). Molecular tracking and remote sensing to evaluate new chemical treatments against the maize late wilt disease causal agent, Magnaporthiopsis maydis. J. Fungi 6, 54. doi: 10.3390/jof6020054
Degani O., Dor S., Movshowitz D., Fraidman E., Rabinovitz O., Graph S. (2018b). Effective chemical protection against the maize late wilt causal agent, Harpophora maydis, in the field. PloS One 13, e0208353. doi: 10.1371/journal.pone.0208353
Degani O., Movshowitz D., Dor S., Meerson A., Goldblat Y., Rabinovitz O. (2019). Evaluating Azoxystrobin seed coating against maize late wilt disease using a sensitive qPCR-based method. Plant Dis. 103, 238–248. doi: 10.1094/PDIS-05-18-0759-RE
Degani O., Gordani A. (2022). New antifungal compound, 6-pentyl-α-pyrone, against the maize late wilt pathogen. Magnaporthiopsis maydis. Agron. 12, 2339. doi: 10.3390/agronomy12102339
Degani O., Regev D., Dor S., Rabinovitz O. (2020). Soil bioassay for detecting Magnaporthiopsis maydis infestation using a hyper susceptible maize hybrid. J. Fungi 6, 107. doi: 10.3390/jof6030107
Degani O., Weinberg T., Graph S. (2014). Chemical control of maize late wilt in the field. Phytoparasitica 42, 559–570. doi: 10.1007/s12600-014-0394-5
Degani O., Yifa R., Gordani A., Becher P., Chen A. (2022b). Cultivars resistance assay for maize late wilt disease. Biology 11, 1854. doi: 10.3390/biology11121854
Deng Y., Chen H., Li C., Xu J., Qi Q., Xu Y., et al. (2019). Endophyte Bacillus subtilis evade plant defense by producing lantibiotic subtilomycin to mask self-produced flagellin. Commun. Biol. 2, 368. doi: 10.1038/s42003-019-0614-0
Dor S., Degani O. (2019). Uncovering the host range for maize pathogen Magnaporthiopsis maydis. Plants 8, 259. doi: 10.3390/plants8080259
El-Mehalowy A. A., Hassanein N. M., Khater H. M., Daram El-Din E. A., Youssef Y. A. (2004). Influence of maize root colonization by rhizosphere actinomycetes and yeast fungi on plant growth and on the biological control of late wilt disease. Inter. J. Agric. Biol. 6, 599–605.
El-Naggar A.-A. A. A., Yassin M. A. (2024). Screening of some biotic and abiotic agents for controlling maize late wilt disease caused by Magnaporthiopsis maydis. Plant 12, 11–18. doi: 10.11648/j.plant.20241201.13
El-Naggarr A. A. A., Sabryr A. M., Yassin M. A. (2015). Impact of late wilt disease caused by Harpophora maydis on maize yield. J. Biol. Chem. Environ. Sc 10, 577–595.
El-Shabrawy E.-S., Shehata H. (2018). Controlling maize late-wilt and enhancing plant salinity tolerance by some rhizobacterial strains. Egypt. J. Phytopathol. 46, 235–255. doi: 10.21608/ejp.2018.87796
El-Shehawy A., Ata A., El-Ghonemy M. (2014). Impact of late wilt caused by Cephalosporium maydis on maize grain yield and protein content. Egypt. J. Phytopathol. 42, 1–10. doi: 10.21608/ejp.2014.95629
Fayzalla E., Sadik E., Elwakil M., Gomah A. (1994). Soil solarization for controlling Cephalosporium maydis, the cause of late wilt disease of maize in Egypt. Egypt J. Phytopathol. 22, 171–178.
Fernández-Ortuño D., Torés J. A., De Vicente A., Pérez-García A. (2010). Mechanisms of resistance to QoI fungicides in phytopathogenic fungi. Int. Microbiol. 11, 1–9.
Francl L. J. (2001). The disease triangle: a plant pathological paradigm revisited. Plant Health Instruct. 10. doi: 10.1094/PHI-T-2001-0517-01
Gao F.-K., Dai C.-C., Liu X.-Z. (2010). Mechanisms of fungal endophytes in plant protection against pathogens. Afr. J. Microbiol. Res. 4, 1346–1351.
García-Carneros A., Girón I., Molinero-Ruiz L. (2011). Aggressiveness of Cephalosporium maydis causing late wilt of maize in Spain. Commun. Agric. Appl. Biol. Sci. 77, 173–179.
Ghazy N., El-Gremi S., Belal E.-S. (2017). Chemical and histological differences of corn (Zea mays L.) responsive to Harpophora maydis infection. Environ. Biodivers. Soil Secur. 1, 3–7. doi: 10.21608/jenvbs.2017.2142.1017
Ghazy N., El-Nahrawy S. (2020). Siderophore production by Bacillus subtilis MF497446 and Pseudomonas koreensis MG209738 and their efficacy in controlling Cephalosporium maydis in maize plant. Arch. Microbiol. 203, 1195–1209. doi: 10.1007/s00203-020-02113-5
Goko M., Murimwa J., Gasura E., Rugare J., Ngadze E. (2021). Identification and characterisation of seed-borne fungal pathogens associated with maize (Zea mays L.). Int. J. Microbiol. 2021, 6702856. doi: 10.1155/2021/6702856
Gond S. K., Bergen M. S., Torres M. S., White J. F. Jr. (2015). Endophytic Bacillus spp. produce antifungal lipopeptides and induce host defence gene expression in maize. Microbiol. Res. 172, 79–87. doi: 10.1016/j.micres.2014.11.004
Gouy M., Guindon S., Gascuel O. (2010). SeaView version 4: a multiplatform graphical user interface for sequence alignment and phylogenetic tree building. Mol. Biol. Evol. 27, 221–224. doi: 10.1093/molbev/msp259
Hamza A. M., El-Kot G., El-Moghazy S. (2013). Non-traditional methods for controlling maize late wilt disease caused by Cephalosporium maydis. Egypt. J. Biol. Pest Control 23, 87–93.
Javed A., Shah A. H., Hussain A., Shinwari Z. K., Khan S. A., Khan W., et al. (2020). Potential of endophytic fungus Aspergillus terreus as potent plant growth promoter. Pak. J. Bot. 52, 1083–1086. doi: 10.30848/PAK.J.BOT
Johal L., Huber D. M., Martyn R. (2004). “Late wilt of corn (maize) pathway analysis: intentional introduction of Cephalosporium maydis,” in Pathways Analysis for the Introduction to the U.S. of Plant Pathogens of Economic Importance (West Lafayette, IN, USA: Purdue University).
Khan S. A., Hamayun M., Yoon H., Kim H. Y., Suh S. J., Hwang S. K., et al. (2008). Plant growth promotion and Penicillium citrinum. BMC Microbiol. 8, 231. doi: 10.1186/1471-2180-8-231
Krause K., Jung E. M., Lindner J., Hardiman I., Poetschner J., Madhavan S., et al. (2020). Response of the wood-decay fungus Schizophyllum commune to co-occurring microorganisms. PloS One 15, e0232145. doi: 10.1371/journal.pone.0232145
Kumar K. A., Gangappa E., Ramesh S., Swamy D. (2021). Identification of high yielding inbred lines resistant to late wilt disease caused by Harpophora maydis in maize. Electron. J. Plant Breed. 12, 151–158. doi: 10.37992/EJPB
Lanubile A., Giorni P., Bertuzzi T., Marocco A., Battilani P. (2021). Fusarium verticillioides and Aspergillus flavus co-occurrence influences plant and fungal transcriptional profiles in maize kernels and in vitro. Toxins 13, 680. doi: 10.3390/toxins13100680
Liu L., Versicoamides F.-H. (2017). prenylated indole alkaloids from Aspergillus tennesseensis. Organ. Lett. 19, 942–945. doi: 10.1021/acs.orglett.7b00145
Luo J., Zhang N. (2013). Magnaporthiopsis, a new genus in Magnaporthaceae (Ascomycota). Mycologia 105, 1019–1029. doi: 10.3852/12-359
Mallett K., Hiratsuka Y. (1986). Nature of the “black line” produced between different biological species of the Armillaria mellea complex. Can. J. Bot. 64, 2588–2590. doi: 10.1139/b86-342
Michail S. H., Abou-Elseoud M. S., Nour Eldin M. S. (1999). Seed health testing of corn for Cephalosporium maydis. Acta Phytopathol. Entomol. Hungarica 34, 35–42.
Ortiz-Bustos C., López-Bernal A., Testi L., Molinero-Ruiz L. (2019). Environmental and irrigation conditions can mask the effect of Magnaporthiopsis maydis on growth and productivity of maize. Plant Pathol. 68, 1555–1564. doi: 10.1111/ppa.13070
Ortiz-Bustos C. M., Testi L., García-Carneros A. B., Molinero-Ruiz L. (2015). Geographic distribution and aggressiveness of Harpophora maydis in the Iberian peninsula, and thermal detection of maize late wilt. Eur. J. Plant Pathol. 144, 383–397. doi: 10.1007/s10658-015-0775-8
Patanita M., Campos M. D., Félix M. D. R., Carvalho M., Brito I. (2020). Effect of tillage system and cover crop on maize mycorrhization and presence of Magnaporthiopsis maydis. Biology 9, 46. doi: 10.3390/biology9030046
Pecsi S., Nemeth L. (1998). Appearance of Cephalosporium maydis Samra Sabet and Hingorani in Hungary. Meded. Fac. Landbouwkd. En Toegep. Biol. Wet. Univ. Gent. 63, 873–877.
Rakesh B., Gangappa E., Gandhi S., Gowda R. V., Swamy S. D., Ramesh S., et al. (2022). Mapping genomic regions controlling resistance to late wilt disease caused by Harpophora maydis in maize (Zea mays L.). Euphytica 218, 101. doi: 10.1007/s10681-022-03052-6
Rodriguez R., White J. Jr., Arnold A. E., Redman A. R. A. (2009). Fungal endophytes: diversity and functional roles. New Phytol. 182, 314–330. doi: 10.1111/j.1469-8137.2009.02773.x
Sabet K. A., Samra A. S., Hingorani M. K., Mansour I. M. (1961). Stalk and root rots of maize in the United Arab Republic. FAO Plant Prot. Bull. 9, 121–125.
Sabet K., Samra A., Mansour I. (1966). Interaction between Fusarium oxysporum F. vasinfectum and Cephalosporium maydis on cotton and maize. Ann. Appl. Biol. 58, 93–101. doi: 10.1111/j.1744-7348.1966.tb05074.x
Sabet K. A., Samra A. S., Mansour I. M. (1970). Saprophytic behaviour of Cephalosporium maydis and C. acremonium. Ann. Appl. Biol. 66, 265–271. doi: 10.1111/j.1744-7348.1970.tb06433.x
Sahab A. F., Osman A. R., Soleman N. K., Mikhail M. S. (1985). Studies on root-rot of lupin in Egypt and its control. Egypt. J. Phytopathol. 17, 23–35.
Sahu M. K., Singh D., Ghosh S. C., Das A., Jha H. (2022). Bioactive potential of secondary metabolites of rhizospheric fungus Penicillium citrinum isolate-ABRF3. J. BioSci. Biotechnol. 11, 1–14.
Saleh A. A., Leslie J. F. (2004). Cephalosporium maydis is a distinct species in the Gaeumannomyces-Harpophora species complex. Mycologia 96, 1294–1305. doi: 10.1080/15572536.2005.11832879
Samra A. S., Sabet K. A., Abdel-Rahim M. F. (1966). Effect of soil conditions and cultural practices on infection with stalk rots (Cairo, Egypt: U.A.R. Ministry of Agriculture, Government Printing Offices), 117–164.
Samra A. S., Sabet K. A., Hingorani M. K. (1962). A new wilt disease of maize in Egypt. Plant Dis. Rep. 46, 481–483.
Samra A. S., Sabet K. A., Hingorani M. K. (1963). Late wilt disease of maize caused by. Cephalosporium maydis. Phytopathol. 53, 402–406.
Samson R., Peterson S., Frisvad J. C., Varga J. (2011). New species in Aspergillus section Terrei. Stud. Mycol. 69, 39–55. doi: 10.3114/sim.2011.69.04
Shofman G., Bahouth M., Degani O. (2022). Aggressive strains of the late wilt fungus of corn exist in Israel in mixed populations and can specialize in disrupting growth or plant health. Fungal Biol. 126, 793–808. doi: 10.1016/j.funbio.2022.10.003
Singh S. D., Siradhana B. S. (1987a). Influence of some environmental conditions on the development of late wilt of maize induced by Cephalosporium maydis. Indian J. Mycol. Pl. Pathol. 17, 1–5.
Singh S. D., Siradhana B. S. (1989). Chemical control of late wilt of maize induced by Cephalosporium maydis. Indian J. Mycol. Pl. Path. 19, 121–122.
Singh S., Siradhana B. (1990). Effect of macro and micronutrients on the development of late wilt of maize induced by Cephalosporium maydis. Summa Phytopathol. 16, 140–145.
Sunitha N., Gangappa E., Gowda R. V., Ramesh S., Biradar S., Swamy D, et al. (2020). Assessment of impact of late wilt caused by Harpophora maydis (Samra, Sabet and Hing) on grain yield and its attributing traits in maize (Zea mays L.). Mysore J. Agric. Sci. 54, 30–36.
Tarazona A., Gómez J. V., Mateo F., Jimenez M., Romera D., Mateo E. M. (2020). Study on mycotoxin contamination of maize kernels in Spain. Food Control 118, 107370. doi: 10.1016/j.foodcont.2020.107370
Tej R., Rodríguez-Mallol C., Rodríguez-Arcos R., Karray-Bouraoui N., Molinero-Ruiz L. (2018). Inhibitory effect of Lycium europaeum extracts on phytopathogenic soil-borne fungi and the reduction of late wilt in maize. Eur. J. Plant Pathol. 152, 249–265. doi: 10.1007/s10658-018-1469-9
Wu C.-W., Wu X., Wen C., Peng B., Peng X. X., Chen X., et al. (2016). Fructose promotes growth and antifungal activity of Penicillium citrinum. Protein Cell 7, 527–532. doi: 10.1007/s13238-016-0280-7
Wu L., Wu H.-J., Qiao J., Gao X., Borriss R. (2015). Novel routes for improving biocontrol activity of Bacillus based bioinoculants. Front. Microbiol. 6, 1395. doi: 10.3389/fmicb.2015.01395
Zeller K. A., Ismael A. S. M., El-Assiuty E. M., Fahmy Z. M., Bekheet F. M., Leslie J. F., et al. (2002). Relative competitiveness and virulence of four clonal lineages of Cephalosporium maydis from Egypt toward greenhouse-grown maize. Plant Dis. 86, 373–378. doi: 10.1094/PDIS.2002.86.4.373
Zhang D., Yu S., Yang Y., Zhang J., Zhao D., Pan Y., et al. (2020). Antifungal effects of volatiles produced by Bacillus subtilis against Alternaria solani in potato. Front. Microbiol. 11. doi: 10.3389/fmicb.2020.01196
Keywords: seedings pathogenicity assay, Cephalosporium maydis, crop protection, endophytes, fungus, Harpophora maydis, microflora, phylogenetic analysis
Citation: Degani O, Ayoub A, Dimant E and Gordani A (2024) Antagonistic interactions between maize seeds microbiome species and the late wilt disease agent, Magnaporthiopsis maydis. Front. Fungal Biol. 5:1436759. doi: 10.3389/ffunb.2024.1436759
Received: 22 May 2024; Accepted: 15 July 2024;
Published: 07 August 2024.
Edited by:
Grzegorz Janusz, Maria Curie-Skłodowska University, PolandReviewed by:
Tariq Mukhtar, Pir Mehr Ali Shah Arid Agriculture University, PakistanFrancisco José Sautua, University of Buenos Aires, Argentina
Copyright © 2024 Degani, Ayoub, Dimant and Gordani. This is an open-access article distributed under the terms of the Creative Commons Attribution License (CC BY). The use, distribution or reproduction in other forums is permitted, provided the original author(s) and the copyright owner(s) are credited and that the original publication in this journal is cited, in accordance with accepted academic practice. No use, distribution or reproduction is permitted which does not comply with these terms.
*Correspondence: Ofir Degani, ZC1vZmlyQG1pZ2FsLm9yZy5pbA==; b2ZpcmRAdGVsaGFpLmFjLmls