- 1Departamento de Ingeniería Genética, Centro de Investigación y de Estudios Avanzados, Irapuato, Mexico
- 2Unidad de Genómica Avanzada, Centro de Investigación y de Estudios Avanzados, Irapuato, Mexico
- 3Centro de Investigación en Alimentación y Desarrollo, Culiacán, Mexico
Mucoralean fungi from the genus Rhizopus are common inhabitants of terrestrial ecosystems, being some pathogens of animals and plants. In this study, we analyzed the symbiotic and toxinogenic potential of Rhizopus species derived from agricultural soils dedicated to the production of papaya (Carica papaya L.) in Mexico. Four representative strains of soil-derived Rhizopus spp. were analyzed employing molecular, microscopic, and metabolic methods. The ITS phylogenies identified the fungi as Rhizopus microsporus HP499, Rhizopus delemar HP475 and HP479, and Rhizopus homothallicus HP487. We discovered that R. microsporus HP499 and R. delemar HP475 harbor similar endofungal bacterial symbionts that belong to the genus Mycetohabitans (Burkholderia sensu lato) and that none of the four fungi were associated with Narnavirus RmNV-20S and RmNV-23S. Intriguingly, the interaction between R. delemar - Mycetohabitans showed different phenotypes from known R. microsporus - Mycetohabitans symbioses. Elimination of bacteria in R. delemar HP475 did not cause a detrimental effect on fungal growth or asexual reproduction. Moreover, metabolic and molecular analyses confirmed that, unlike symbiotic R. microsporus HP499, R. delemar HP475 does not produce rhizoxin, one of the best-characterized toxins produced by Mycetohabitans spp. The rhizoxin (rhi) biosynthetic gene cluster seems absent in this symbiotic bacterium. Our study highlights that the symbioses between Rhizopus and Mycetohabitans are more diverse than anticipated. Our findings contribute to expanding our understanding of the role bacterial symbionts have in the pathogenicity, biology and evolution of Mucorales.
Introduction
Mexico is the major exporter of papaya, producing on average 1,117,437.20 metric tons of this tropical fruit per year, harvesting 18,982.79 hectares (SIAP, 2020). Recent studies have shown that Rhizopus and other Mucorales are prevalent in papaya producing soils, and some of these fungi, including Rhizopus delemar, are also involved in the soft rot of the fruits (Cruz-Lachica et al., 2017; Cruz-Lachica et al., 2018).
Strains of Rhizopus microsporus have been reported as the causal agents of rice seedling blight (Furuya et al., 1974), a disease caused by the secreted toxin rhizoxin (Gho et al., 1978; Iwasaki et al., 1984). However, this toxin is not directly synthesized by R. microsporus, but by its intracellular bacterial symbionts (Partida-Martinez and Hertweck, 2005). To date, all investigated rhizoxin-producing strains of Rhizopus microsporus live in close association with endosymbiotic bacteria from the genus Mycetohabitans (Burkholderia sensu lato) (Lackner et al., 2009; Partida-Martinez, 2013; Dolatabadi et al., 2016). This novel genus currently recognizes two type species: M. rhizoxinica and M. endofungorum (Partida-Martinez et al., 2007a; Estrada-de Los Santos et al., 2018). These bacterial symbionts possess the well characterized hybrid PKS-NRPS biosynthetic gene cluster rhi for the production of the potent toxin rhizoxin (Partida-Martinez and Hertweck, 2007). This toxin inhibits mitosis in most eukaryotic cells, including plants, fungi, animals and even human cancer cells (Iwasaki et al., 1984; Jordan et al., 1998; Schmitt et al., 2008). The rhi biosynthetic gene cluster has been only found and characterized in Mycetohabitans spp. and in the plant commensal Pseudomonas fluorescens Pf-5 (Brendel et al., 2007). Besides the production of rhizoxin for their fungal host, Mycetohabitants symbionts are in full control of the asexual reproduction of R. microsporus (Partida-Martinez et al., 2007b) and also influence their sexuality (Mondo et al., 2017). Interestingly, some of these fungi, although apparently less frequently, live also in symbioses with two ssRNA viruses from the genus Narnavirus (RmNV-20S and RmNV-23S) (Espino-Vázquez et al., 2020). These narnaviruses, together with Mycetohabitans, have been shown to be important for the sexual success in this species (Mondo et al., 2017; Espino-Vázquez et al., 2020). These bacterial and viral symbionts are vertically inherited through the asexual sporangiospores and sexual zygospores produced by R. microsporus (Partida-Martinez et al., 2007b; Mondo et al., 2017; Espino-Vázquez et al., 2020).
Here we characterized four representative strains of Rhizopus species recovered from papaya producing soils from the states of Colima, Veracruz and Oaxaca in Mexico (Cruz-Lachica et al., 2018). By using molecular, microscopic and metabolic analyses, we identified that two of these strains harbor Mycetohabitans, but none of them narnaviruses. Remarkably, we found a novel association between R. delemar and Mycetohabitans that does not follow the phenotypes known from well-studied and globally distributed R. microsporus – Mycetohabitans pairs (Lackner et al., 2009; Partida-Martinez, 2013; Dolatabadi et al., 2016). This finding highlights the diversity of the symbioses within these fungal and bacterial genera and expands our possibilities to deepen our understanding of the ecological and evolutionary role of endosymbionts for fungal biology.
Materials and methods
Fungal strains and culturing conditions
Wild-type strain Rhizopus microsporus HP499, R. delemar HP475, R. delemar HP479 and R. homothallicus HP487 were isolated from soils of papaya producing regions in Colima, Veracruz and Oaxaca in Mexico and provided by García-Estrada in 2019 (Cruz-Lachica et al., 2018). Fungal strains R. microsporus ATCC 52813, ATCC 52814 and ATCC 11559 were obtained from the American Type Culture Collection and used as controls for symbiotic/rhizoxin-producing (ATCC 52813 and ATCC 52814) and asymbiotic/non-rhizoxin producing Rhizopus species (ATCC 11559). All strains were grown at 30°C in PDA (4 g/L potato extract, 10 g/L dextrose, and 15 g/L agar).
Isolation and microscopy of bacterial symbionts
Endosymbionts were isolated from their hosts as previously described (Partida-Martinez and Hertweck, 2005; Scherlach et al., 2006; Espino-Vázquez et al., 2020). Briefly, fungal mycelia was submerged on 0.5 mL 0.85% NaCl, mechanically damaged using a pipette tip and then centrifuged for 30 min at 13,200 rpm. Supernatant was plated on TSA petri dishes and incubated at 30°C until the appearance of bacterial colonies. Visualizations of endofungal bacteria were performed using 2-3 days-old grown mycelia (3 cm2) on 0.5 mL NaCl 0.85%. An aliquot of 10 µL fungal cell suspension was placed onto a microscope slide then 10 µL of 0.01 mM SYTO™ 9 were added, and the mix was incubated in the dark for 20 min. Fungal tissues were analyzed using a Leica DM600B and the GFP filter.
DNA isolation and molecular identification of Rhizopus spp. and their endosymbionts
Total DNA from wild-type fungal strains were isolated as described (Nicholson et al., 2001). For fungal molecular identification, the ITS region (Internal Transcribed Spacer) was amplified using ITS-1F (5´- CTTGGTCATTTAGAGGAAGTAA-3´) and ITS-4R (5´- TCCTCCGCTTATTGATATGC-3´) primers. For bacterial molecular identification, the 16S rRNA gene was amplified using 16S-F27 (5´- AGAGTTTGATCMTGGCTCAG-3´) and 16S-R1494 (5´- CTACGGRTACCTTGTTACGAC -3´) primers. Narnavirus RmNV-20S and RmNV-23S identification was done as before (Espino-Vázquez et al., 2020). All products were confirmed by Sanger Sequencing at the Genomic Services Laboratory from UGA-Langebio Cinvestav Irapuato, Mexico. Fungal and bacterial sequences have been deposited in Genbank under the following accessions OM677455-458 and OM634667-668, respectively.
Phylogenetic analyses
The fungal ITS and bacterial 16S rDNA gene sequences were aligned using the MUSCLE algorithm in the MEGA 11 software package (Table S1). These alignments were used for tree construction using the Maximum Likelihood method with 1000 bootstrap replicates and the Tamura-Nei model. Also, the same sequences were used to infer their evolutionary history using MrBayes. For this, two independent chains were used along with 100M Monte Carlo Markov chain generations.
Generation of cured (b-) and re-infected (b*) symbiotic Rhizopus strains
In order to generate cured, symbiont-free fungal strains (b-), the wild-type strains R. microsporus HP499 and R. delemar HP475 (b+) were constantly cultivated in the presence of ciprofloxacin (50-100 mg/ml) on PDB medium (Partida-Martinez and Hertweck, 2005) until the successful elimination of bacterial symbionts was confirmed by the absence of vegetative sporulation (Partida-Martinez et al., 2007b) and/or the lack of 16S rDNA gene amplification in the antibiotic treated fungi (Figure S7). Once cured fungal strains were confirmed and Mycetohabitans sp. HP499 and HP475 isolated and validated in axenic culture, co-infections experiments were performed. For this, a mycelial plug of cured fungal strains (b-) was placed on a PDA plate that contained several plugs of 2-days-old cultures of Mycetohabitans in TSA medium. Incubation at 30°C took place for ca. 72 hours until the appearance of sporangia was observed.
Rhizopus’ growth kinetics
All four wild-type (b+ or asymbiotic), bacteria-free (b-) and bacteria-reinfected (b*) Mexican Rhizopus strains were cultured on PDA, 30°C in quadruplicate. Radial growth was measured every 24 h. After 96 h, total fungal biomass was dried at 60°C and weighted. Data were analyzed by One-way ANOVA followed by the Tukey’s post-hoc test, P <0.05.
Fermentation, extraction, bioassays and HPLC-MS analyses for the identification of the toxin rhizoxin
To investigate the production of rhizoxin, liquid fermentations of Rhizopus and Mycetohabitans species were performed in 100 ml VK medium under agitation at 30°C for 4 days as previously described (Partida-Martinez and Hertweck, 2005). Raw extracts were obtained using 1:1 v/v ethyl acetate. The organic phase was filtered, dried and later dissolved in methanol. Raw extracts were first bioassayed using Trichoderma atroviridae, a rhizoxin-susceptible fungus (Schmitt et al., 2008). For this, 2x105 spores of T. atroviridae were mixed in 60 ml of PDA in a petri dish. Afterwards, raw extracts of Rhizopus spp. (50 µL, 8 mg/mL) and Mycetohabitans spp. (50 µL, 2 mg/mL) were poured in the plate. Plates were incubated at 28°C for 3 days and inhibition halos measured (Figure S8).
Rhizoxin detection by HPLC-MS was carried out as before (Partida-Martinez and Hertweck, 2005). HPLC analyses were done with the AZXDB-9 Zorbax Eclipse XDB-C18 4.6 x 150 mm column with 5 mm particle size. UV detection was done at 310 nm. HPLC fractions were analyzed by DIESI-MS into a 3D ion trap mass spectrometer LCQ Fleet (Thermo Fisher Scientific). Mass spectra were obtained in ESI positive mode with 4.5 kV, 105 V tube lens voltage, and 30 V capillary voltage at 280°C. The flow injection rate was 10 µL/min. For the MS/MS analysis, main peaks were fragmented with 28 eV collision energy.
Detection of the rhizoxin biosynthetic cluster (rhi) by PCR
Fragments of five ORFs of the rhizoxin gene cluster (rhi) were amplified by PCR using primers designed for M. rhizoxinica HKI 454 GenBank: FR687359.1 (Table S2). Four of them amplified fragments of the ORFs that build the PKS-NRPS thiotemplate system (rhiA, rhiB, rhiC and rhiD) and one the ORF that codes for the tandem trans-AT (rhiG, Table S2) (Partida-Martinez and Hertweck, 2007). The PCR reaction consisted of denaturation for 3 min at 94°C; 30 cycles of 94°C for 30 s, 64°C for 45 s, 72°C for 10 s; and a final extension at 72°C for 30 s. The PCR amplicons were cloned in vector pJET 1.2 (Thermo Fisher Scientific) and sequenced to confirm their identity.
Results
Morphological description and taxonomic determination of Rhizopus strains
Morphological observations during the vegetative development of the Rhizopus strains showed the characteristic rhizoids, grayish branched aerial mycelium, and elliptic shape spores (Figure 1A) (Schipper and Stalpers, 1984). According to the ITS phylogenetic analyses (Figures 1C, S3), the identities of the four Mexican fungal strains are R. microsporus HP499, R. delemar HP475, R. delemar HP479, and R. homothallicus HP487 for which sexual reproductive structures were observed as this fungus in not heterothallic (Figure 1Ad´, d´´) (Gryganskyi et al., 2018).
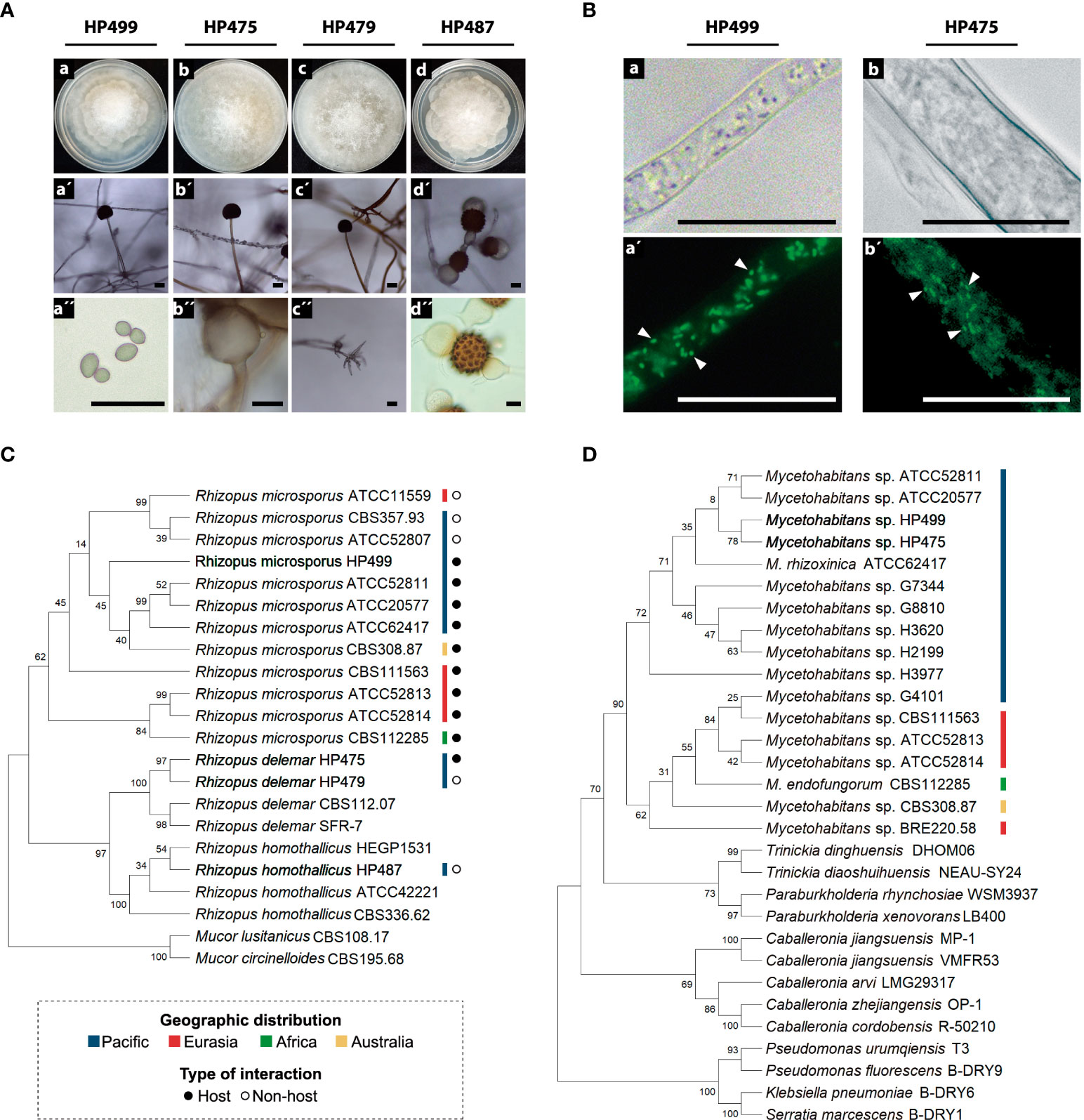
Figure 1 Identification of Rhizopus spp. and their bacterial symbionts. (A) Morphological structures of Rhizopus spp. (a-d) Colonies grown on PDA at 30°C for 72 h. (a´-c´) Sporangium and sporangiophore. (d´) and (d´´) Zygospores. (a´´) Sporangiospores. (b´´) Columella. (c´´) Rhizoids. Scale bars = 20 μm. (B) Bacterial endosymbionts thriving in the cytoplasm of the fungal strains HP499 and HP475. DNA from bacterial symbionts was stained with SYTO™ 9. (a-b) Micrographs taken under white light, and (a´-b´) under fluorescence. Arrows point out individual bacterial cells. Scale bars = 20 μm. (C) Fungal phylogeny based on the ITS marker. (D) Bacterial phylogeny based on the 16S rRNA gene. The evolutionary history was inferred for (C, D) by using the Maximum Likelihood method with 1000 bootstrap replicates and Tamura-Nei model.
Identification of bacterial and viral endosymbionts
We detected positive 16S rRNA gene amplification in R. microsporus HP499 and R. delemar HP475 (Figure S1). Thus, we searched and confirmed the presence of bacteria inside the fungal cytosol by SYTO™ 9 staining (Figures 1B and S2). Phylogenetic analyses grouped these bacteria closer to M. rhizoxinica ATCC 62417 and into the Pacific branch (Figures 1D, S4). Accordingly, we named these bacteria as Mycetohabitans sp. HP499 and Mycetohabitans sp. HP475. We also succeeded in isolating and cultivating both bacterial symbionts that showed to be gram-negative small bacilli (Figure S5). Additionally, we investigated the presence of the Narnavirus RmNV-20S and RmNV-23S by RT-PCR. However, none of the four fungal Mexican strains harbored these viruses (Figure S6).
The absence of Mycetohabitans alters R. microsporus HP499 phenotype, but not that of R. delemar HP475
To address the importance of the endofungal bacteria in R. microsporus HP499 and R. delemar HP475, we generated symbiont-free (b-) and re-infected fungi (b*) (Figure S7). R. microsporus HP499 significantly decreased its growth in the absence of its Mycetohabitans endobacteria, being the wild-type (wt) phenotype recovered when the fungus was re-infected. Remarkably, none of the manipulated strains of R. delemar HP475 (b-, b*) changed its growth rate with respect to the wild-type (wt) fungus throughout 96h (Figures 2A, B). The asymbiotic R. delemar HP479 and R. homothallicus HP487 showed similar growth rates as the symbiotic R. delemar HP475 and R. microsporus HP499, respectively (Figure 2A). Encouraged by the strong effect of Mycetohabitans sp. HP499 on the growth of its host, we searched for changes in the asexual reproduction of the fungus (Partida-Martinez et al., 2007b). As expected, absence of the endofungal bacteria nullifies the production of sporangiospores in R. microsporus HP499, however, in R. delemar HP475 no changes in the number of sporangiospores produced were quantified in the absence of the bacterial symbionts (Figure 2C).
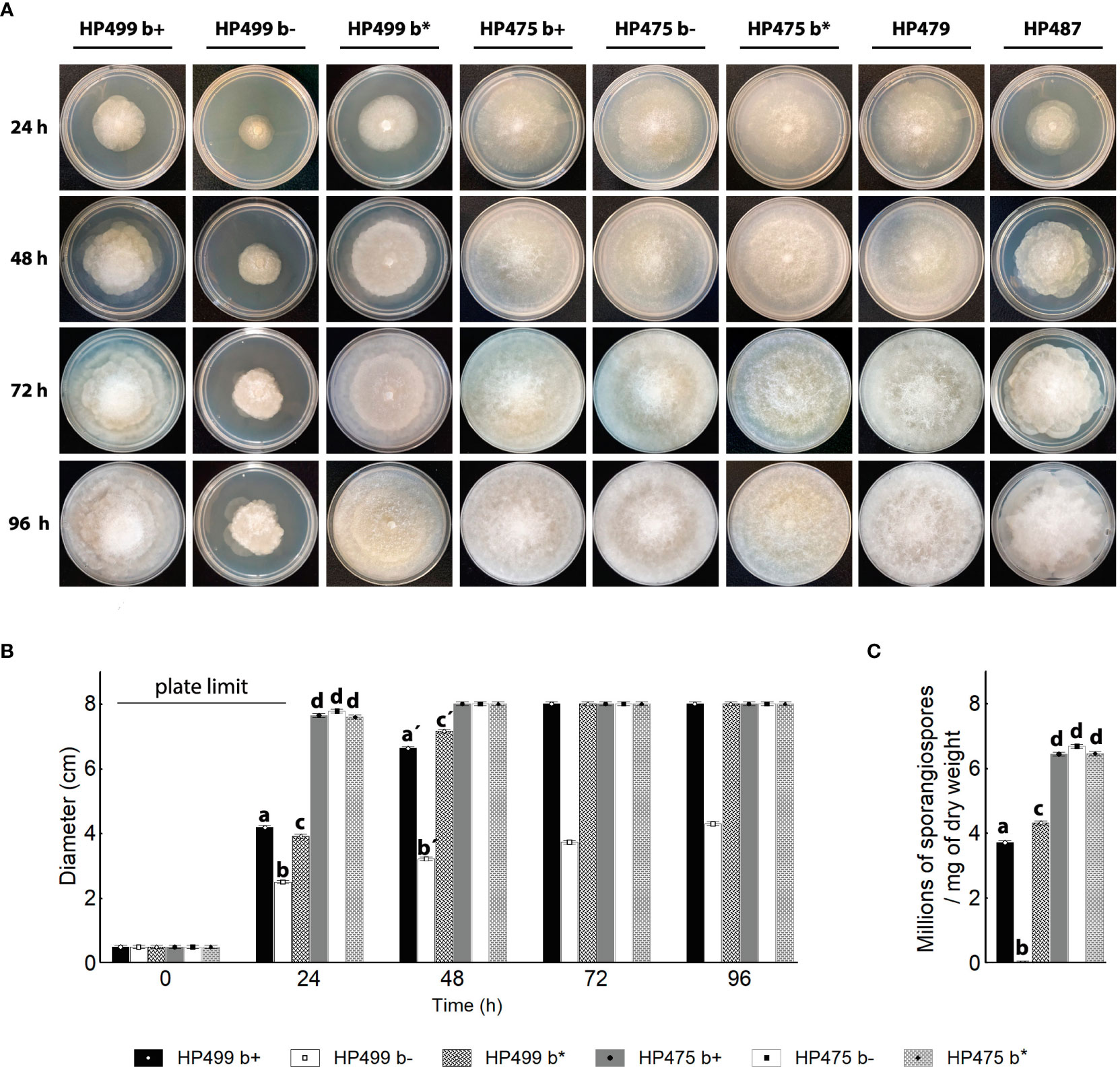
Figure 2 Development of symbiotic and asymbiotic Rhizopus strains. (A) Development (up to 96 h) of colonies of Rhizopus microsporus HP499, R. delemar HP475 and HP479, and R. homothallicus HP487 grown on PDA at 30°C. b+ natural symbiotic strain. b- cured strain. b* re-infected strain with their natural bacteria. (B) Growth kinetics of wild-type, cured, and re-infected fungal hosts for 96 h on PDA plates. Error bars represent the standard error. One-way ANOVA followed by Tukey’s post-hoc test, P <0.05, n = 4 in each group. Letters indicate statistical significance. (C) Sporangiospores produced per mg of dry weight after 96h of growth. One-way ANOVA followed by Tukey’s post-hoc test, P <0.05, n = 4. Error bars represent the standard error.
Mycetohabitans sp. HP475 does not produce the potent toxin rhizoxin
We evaluated the capacity to produce rhizoxin and its derivatives by the four Mexican Rhizopus strains, as well as by the two new isolated bacterial symbionts Mycetohabitans sp. HP499 and HP475. First, we evaluated the antifungal capacity of raw extracts vs. Trichoderma atroviridae, a rhizoxin-susceptible fungus (Schmitt et al., 2008). This bioassay showed that only the raw extracts of R. microsporus HP499 and its symbiotic bacterium Mycetohabitans sp. HP499 inhibited the growth of T. atroviridae (Figure S8). Our HPLC-MS analyses confirmed that R. microsporus HP499 and Mycetohabitans sp. HP499 did produce rhizoxin and its derivatives (Figures 3A, B). These rhizoxin-like peaks were MS/MS analyzed and compared to those produced by the well characterized rhizoxin-producing strain R. microsporus ATCC 52814 wt (Figure S9), confirming the same chemical nature. However, symbiotic R. delemar HP475 nor its Mycetohabitans bacteria inhibited the growth of T. atroviridae nor produced rhizoxin (Figures 3A, S8), while asymbiotic R. delemar HP479 and R. homothallicus HP487 did not inhibit growth of T. atroviridae and did not produce rhizoxins, as expected.
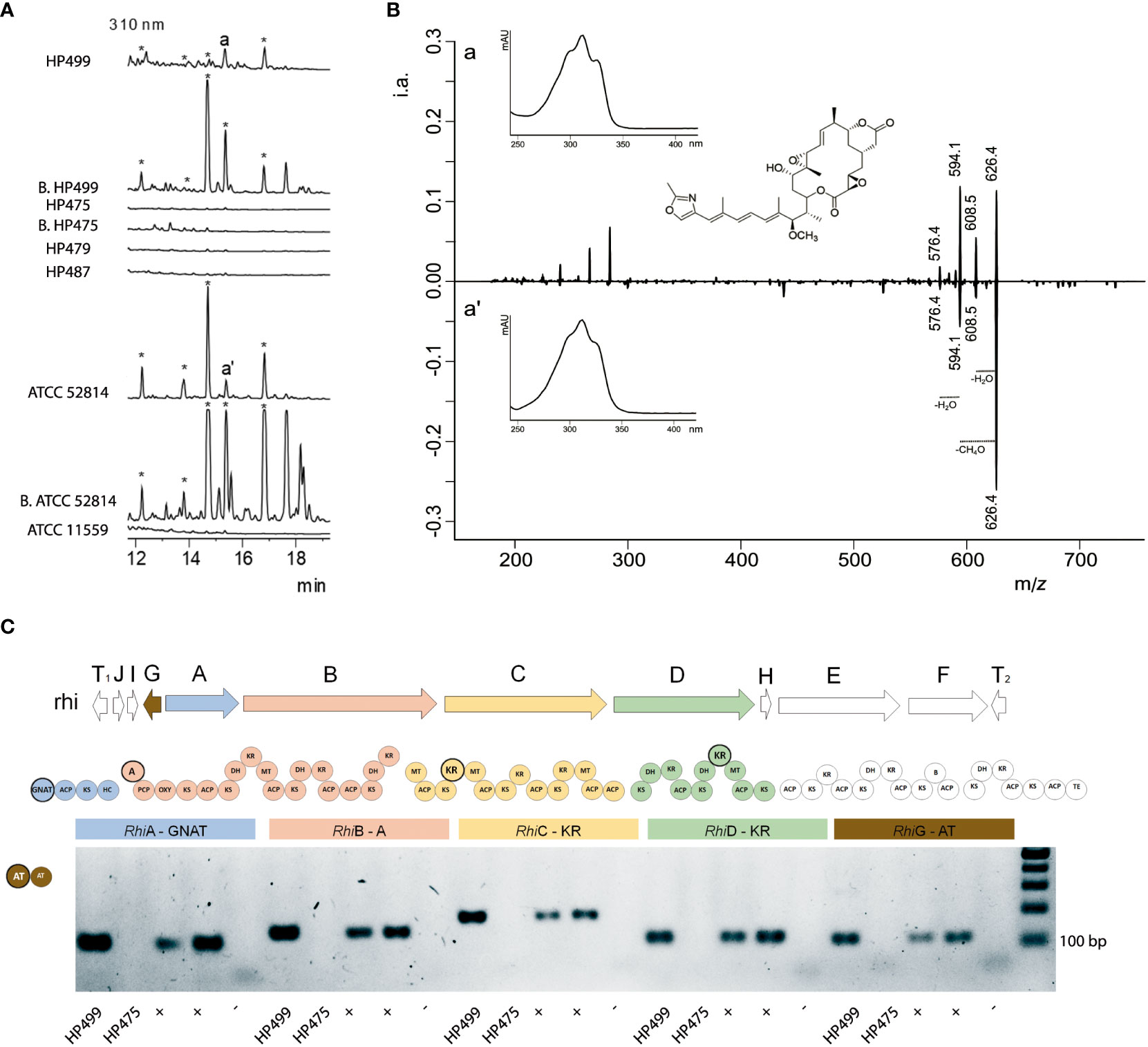
Figure 3 Metabolic and molecular identification of the production of rhizoxin. (A) HPLC chromatograms of raw extracts obtained from the liquid fermentation of Rhizopus and Mycetohabitans species. R. delemar HP479, R. homathallicus HP487 and R. microsporus ATCC 11559 are asymbiotic fungal strains. R. microsporus HP499, R. delemar HP475 and R. microsporus ATCC 52814 are symbiotic fungal strains, and Mycetohabitans sp. HP499 [B. HP499], Mycetohabitans sp. HP475 [B. HP475]) and Mycetohabitans sp. ATCC 52814 [B. ATCC 52814] are their respective endofungal bacteria. Strains ATCC 52814 and ATCC 52814 [B. ATCC 52814] were used as positive, and ATCC 11559 as negative controls, respectively. Peaks labeled with a, a’, and * represent rhizoxin derivatives. (B) Characteristic spectra of rhizoxin (Maximum at 310 nm) and its MS/MS (626 m/z) fragmentation pattern in HP499 (a) and ATCC 52814 (a’) strains. Collision energy was 28 eV. (C) PCR amplification of the rhi cluster (rhzoxin). R. microsporus HP499 does have gene fragments from the rhizoxin cluster, while R. delemar HP475 does not show any. Reference fungal strains ATCC 52813 and ATCC 52814 harboring Mycetohabitans were used as positive controls (positives for rhizoxin production), while asymbiotic strain ATCC 11559 served as negative control. Highlighted circles represent the amplified motifs within the rhi BGC. GNAT N-acetyltransferase, ACP acyl carrier protein, KS ketosynthase, HC condensation/heterocyclization, A adenylation, PCP peptidyl carrier protein, OXY oxygenase, DH dehydratase, KR ketoreductase, MT C-methyltransferase, B unknown domain possibly involved in β-branching, TE thioesterase, RhiA-F thiotemplate, RhiI O-methyltransferase, RhiG acyltransferase, RhiH cytochrome P450 monooxygenase, and T1, T2 transposase genes.
Finally, we investigated if the lack of rhizoxin and its derivatives in symbiotic R. delemar HP475 and its endobacteria was due to the absence of the rhi cluster. As depicted in Figure 3C, fragments of the rhiG, rhiA, rhiB, rhiC and rhiD ORFs were readily amplified from the genomic DNA from R. microsporus HP499 and the rhizoxin-positive R. microsporus ATCC 52813 and 52814, but not from R. delemar HP475.
Discussion
Papaya is a highly valued agricultural product of Mexico. In this study, we investigated the symbiotic and toxinogenic potential of four Rhizopus strains that were recovered from soils from the states of Colima, Veracruz and Oaxaca in which papaya is produced (Cruz-Lachica et al., 2018). Our results revealed that two of these strains harbor bacterial symbionts from the genus Mycetohabitans, but none of them the narnaviruses RmNV-20S and RmNV-23S. These symbiotic fungal strains were identified as R. microsporus HP499 and R. delemar HP475 (Figure 1C). Microscopic observations and molecular identification of these bacterial symbionts showed that they thrive in the fungal cytoplasm (Figure 1B), are most similar to each other, and close to the type strain Mycetohabitans rhizoxinica ATCC 62417 that belongs to the Pacific clade (Figure 1D). Significantly, in this Pacific clade are now strains from Japan, USA and Mexico (Figures 1C, D; S3 and S4; Table S1). These results support the biogeographical distribution of symbiotic fungi and their endobacteria (Lackner et al., 2009). Notably, our report that R. delemar HP475 harbor Mycetohabitans symbionts is, as far as we are aware, unique. Other recent studies searching for endofungal bacteria in the phylum Mucoromycota, or specifically in the genus Rhizopus, have revealed that Mycetohabitans is the most common symbiont of Rhizopus microsporus (Okrasińska et al., 2021). In fact, only until recently, all Mycetohabitans strains known were associated with several strains of this fungal species (Lackner et al., 2009; Partida-Martinez, 2013; Dolatabadi et al., 2016), as the origin of Mycetohabitans species obtained from clinical specimens could not be clearly tracked (Gee et al., 2011). Recently, novel Mycetohabitans symbionts living in four different strains of Mortierella verticillata have been discovered, although their capacity to produce rhizoxin has not been reported nor their effects on the biology of Mortierella fungi (Büttner et al., 2021). Lastly, a clinical isolate of R. microsporus stably associates with Ralstonia pickettii. This bacterial symbiont helps its fungal host to evade amoeba and cause opportunistic infections in animals (Itabangi et al., 2022). All these reports and our evidence support the notion that interactions of Rhizopus species with endofungal bacteria are more diverse and plastic than initially thought.
Moreover, our findings that the Mycetohabitans symbiont of R. delemar HP475 does not influence fungal growth nor is in control of its asexual sporulation (Figure 2) suggest that this symbiont might be of more recent acquisition. Horizontal transfer of Mycetohabitans symbionts in R. microsporus has been postulated as possible for the following reasons: a) Mycetohabitans spp. can readily colonize cured R. microsporus hosts (Partida-Martinez and Hertweck, 2005), as they possess a functional type 2 secretion system that enables the release of chitinolytic enzymes, specially chitinase, that allow bacterial entry into the fungal hyphae (Moebius et al., 2014); b) Mucoralean fungi tend to be rhizoxin-resistant, as their amino acid in the 100 position of their β-tubulin is not arginine (N), but serine (S) or alanine (A) (Schmitt et al., 2008). These changes in this amino acid prevent β-tubulin binding with rhizoxin, and further allows microtubule polymerization during mitosis; and c) Phylogenies of symbiotic Rhizopus microsporus and their Mycetohabitans symbionts revealed a high degree of co-speciation, but also suggested the possibility of occasional horizontal transfers (Lackner et al., 2009). All these make us hypothesize that R. delemar HP475 might have acquired its Mycetohabitans symbionts by contact with symbiotic R. microsporus in the papaya producing soils sampled. It is also likely that the horizontal transfer of Mycetohabitans symbionts to a different species of Rhizopus could have imposed genomic re-arrangements in the endobacteria to adapt to the new host. One such adaptation could possibly be the full or partial loss of the rhi cluster. This hypothesis warrants further and deeper investigation.
In addition, our experiments and evidence to date have not revealed yet any further contribution of Mycetohabitans to the fitness/adaptation of R. delemar HP475. However, we cannot discard that Mycetohabitans sp. HP475 could confer other ecological advantages to its fungal host in its natural environment, as members from this genus have the highest metabolic potential in the genus Burkholderia sensu lato, despite their relatively reduced genomes (Mullins and Mahenthiralingam, 2021). This has been shown for Mycetohabitans sp. CBS 308.87 (Figure 1D; Figure S4 and Table S1), a symbiotic bacterium of R. microsporus that produces low amounts of rhizoxin and its derivatives, but which has the biosynthetic gene cluster nec to produce cytotoxic benzolactones called necroximes A, B, C and D (Niehs et al., 2020). Remarkably, the nec BGC was also found in the endofungal bacterial symbiont Candidatus Mycoavidus necroximicus that thrives inside M. verticillata NRRL 6337 and produces necroximes C and D. These metabolites protect its fungal host from nematode attacks, increasing fungal survival in the soil (Büttner et al., 2021).
From the agricultural perspective, our study points out to the necessity of studying the prevalence of symbiotic and toxinogenic Rhizopus species and other Mucorales in Mexico to prevent agricultural losses, and also the consumption of toxic fruits that could promote disease in humans and animals.
In sum, the two novel symbiotic relationships identified here will help expand our understanding of the distribution, ecology and evolution of fungal-bacterial-viral symbioses in early-diverging fungi.
Data availability statement
The datasets presented in this study can be found in online repositories. The names of the repository/repositories and accession number(s) can be found in the article/Supplementary Material.
Author contributions
JC-R, JM-S & LP-M: Planned and designed research. JC-R, JM-S, GC-L & RA-V: Performed experiments and analyzed the data. RG-E: Collected soil samples and isolated Rhizopus spp. RA-V, RW & LP-M: Generated and analyzed MSn data. LPP-M: Secured funding. JC-R, JM-S, GC-L, RA-V & LP-M: Wrote the paper. All authors contributed to the article and approved the submitted version.
Funding
LP-M acknowledges Consejo Nacional de Ciencia y Tecnología in Mexico (CONACyT), which supported this project with grants: FOINS-2015-01-006 and A1-S-9889.
Acknowledgments
We are thankful to María Nélida Vázquez Sánchez, Laboratorio de Cromatografía/Dra. Danae Carrillo, Laboratorio de Microscopía/Dr. Lino Sánchez, and Antonio Cisneros, all from Cinvestav Unidad Irapuato, for their technical support and help with chromatography, microscopy and photography, respectively.
Conflict of interest
The authors declare that the research was conducted in the absence of any commercial or financial relationships that could be construed as a potential conflict of interest.
Publisher’s note
All claims expressed in this article are solely those of the authors and do not necessarily represent those of their affiliated organizations, or those of the publisher, the editors and the reviewers. Any product that may be evaluated in this article, or claim that may be made by its manufacturer, is not guaranteed or endorsed by the publisher.
Supplementary material
The Supplementary Material for this article can be found online at: https://www.frontiersin.org/articles/10.3389/ffunb.2022.893700/full#supplementary-material
References
Brendel N., Partida-Martinez L. P., Scherlach K., Hertweck C. (2007). A cryptic PKS–NRPS gene locus in the plant commensal Pseudomonas fluorescens pf-5 codes for the biosynthesis of an antimitotic rhizoxin complex. Org. Biomol. Chem. 5, 2211–2213. doi: 10.1039/B707762A
Büttner H., Niehs S. P., Vandelannoote K., Cseresnyés Z., Dose B., Richter I., et al. (2021). Bacterial endosymbionts protect beneficial soil fungus from nematode attack. Proc. Natl. Acad. Sci. 118, e2110669118. doi: 10.1073/pnas.2110669118
Cruz-Lachica I., Marquez-Zequera I., Allende-Molar R., Sañudo-Barajas J. A., Leon-Felix J., Ley-Lopez N., et al. (2018). Diversity of mucoralean fungi in soils of papaya (Carica papaya l.) producing regions in Mexico. Fungal Biol. 122, 810–816. doi: 10.1016/j.funbio.2018.04.008
Cruz-Lachica I., Márquez-Zequera I., García-Estrada R. S., Carrillo-Fasio J. A., León-Félix J., Allende-Molar R., et al. (2017). Identification of mucoralean fungi causing soft rot in papaya (Carica papaya l.) fruit in Mexico. Rev. Mex. Fitopatol. 35, 397–417. doi: 10.18781/r.mex.fit.1611-3
Dolatabadi S., Scherlach K., Figge M., Hertweck C., Dijksterhuis J., Menken S. B. J., et al. (2016). Food preparation with mucoralean fungi: A potential biosafety issue? Fungal Biol. 120, 393–401. doi: 10.1016/j.funbio.2015.12.001
Espino-Vázquez A. N., Bermúdez-Barrientos J. R., Cabrera-Rangel J. F., Córdova-López G., Cardoso-Martínez F., Martínez-Vázquez A., et al. (2020). Narnaviruses: novel players in fungal-bacterial symbioses. ISME J. 14, 1743–1754. doi: 10.1038/s41396-020-0638-y
Estrada-de Los Santos P., Palmer M., Chávez-Ramírez B., Beukes C., Steenkamp E. T., Briscoe L., et al. (2018). Whole genome analyses suggests that Burkholderia sensu lato contains two additional novel genera (Mycetohabitans gen. nov., and Trinickia gen. nov.): Implications for the evolution of diazotrophy and nodulation in the burkholderiaceae. Genes 9, 389. doi: 10.3390/genes9080389
Furuya S., Kurata M., Saito T. (1974). Studies on Rhizopus sp. causing growth injury of young rice seedlings and its chemical control. Proc. Assoc. Plant Prot. 9, 49–55.
Gee J. E., Glass M. B., Lackner G., Helsel L. O., Daneshvar M., Hollis D. G., et al. (2011). Characterization of Burkholderia rhizoxinica and B. endofungorum isolated from clinical specimens. PloS One 6, e15731. doi: 10.1371/journal.pone.0015731
Gho N., Sato Z., Yaoita T., Aoyagi K. (1978). Studies on the control of Rhizopus in the nursery cases of rice seedlings. 5. influence of a phytotoxic substance produced by Rhizopus on growht of rice. Proc. Assoc. Plant Prot. 26, 90–94.
Gryganskyi A. P., Golan J., Dolatabadi S., Mondo S., Robb S., Idnurm A., et al. (2018). Phylogenetic and phylogenomic definition of Rhizopus species. G3 Genes.Genom.Genet. 8, 2007–2018. doi: 10.1534/g3.118.200235
Itabangi H., Sephton-Clark P. C. S., Tamayo D. P., Zhou X., Starling G. P., Mahamoud Z., et al. (2022). A bacterial endosymbiont of the fungus Rhizopus microsporus drives phagocyte evasion and opportunistic virulence. Curr. Biol. 32, 1115–1130.e6. doi: 10.1016/j.cub.2022.01.028
Iwasaki S., Kobayashi H., Furukawa J., Namikoshi M., Okuda S., Sato Z., et al. (1984). Studies on macrocyclic lactone antibiotics. VII. structure of a phytotoxin “rhizoxin“ produced by rhizopus chinensis. J. Antibiot. (Tokyo) 37, 354–362. doi: 10.7164/antibiotics.37.354
Jordan A., Hadfield J. A., Lawrence N. J., McGown A. T. (1998). Tubulin as a target for anticancer drugs: Agents which interact with the mitotic spindle. Med. Res. Rev. 18, 259–296. doi: 10.1002/(SICI)1098-1128(199807)18:4<259::AID-MED3>3.0.CO;2-U
Lackner G., Möbius N., Scherlach K., Partida-Martinez L. P., Winkler R., Schmitt I., et al. (2009). Global distribution and evolution of a toxinogenic Burkholderia-Rhizopus symbiosis. Appl. Environ. Microbiol. 75, 2982–2986. doi: 10.1128/AEM.01765-08
Moebius N., Üzüm Z., Dijksterhuis J., Lackner G., Hertweck C. (2014). Active invasion of bacteria into living fungal cells. eLife 3, e03007. doi: 10.7554/eLife.03007
Mondo S. J., Lastovetsky O. A., Gaspar M. L., Schwardt N. H., Barber C. C., Riley R., et al. (2017). Bacterial endosymbionts influence host sexuality and reveal reproductive genes of early divergent fungi. Nat. Commun. 8, 1843. doi: 10.1038/s41467-017-02052-8
Mullins A. J., Mahenthiralingam E. (2021). The hidden genomic diversity, specialized metabolite capacity, and revised taxonomy of Burkholderia sensu lato. Front. Microbiol. 12 doi: 10.3389/fmicb.2021.726847
Nicholson T. P., Rudd B. A., Dawson M., Lazarus C. M., Simpson T. J., Cox R. J. (2001). Design and utility of oligonucleotide gene probes for fungal polyketide synthases. Chem. Biol. 8, 157–178. doi: 10.1016/s1074-5521(00)90064-4
Niehs S. P., Dose B., Richter S., Pidot S. J., Dahse H.-M., Stinear T. P., et al. (2020). Mining symbionts of a spider-transmitted fungus illuminates uncharted biosynthetic pathways to cytotoxic benzolactones. Angew. Chem. Int. Ed. 59, 7766–7771. doi: 10.1002/anie.201916007
Okrasińska A., Bokus A., Duk K., Gęsiorska A., Sokołowska B., Miłobędzka A., et al. (2021). New endohyphal relationships between mucoromycota and burkholderiaceae representatives. Appl. Environ. Microbiol. 87, e02707-20. doi: 10.1128/AEM.02707-20
Partida-Martinez L. P. (2013). A model for bacterial-fungal interactions (Saarbrücken, Germany: LAP LAMBERT Academic Publishing).
Partida-Martinez L. P., Groth I., Schmitt I., Richter W., Roth M., Hertweck C. (2007a). Burkholderia rhizoxinica sp. nov. and Burkholderia endofungorum sp. nov., bacterial endosymbionts of the plant-pathogenic fungus Rhizopus microsporus. Int. J. Syst. Evol. Microbiol. 57, 2583–2590. doi: 10.1099/ijs.0.64660-0
Partida-Martinez L. P., Hertweck C. (2005). Pathogenic fungus harbours endosymbiotic bacteria for toxin production. Nature 437, 884–888. doi: 10.1038/nature03997
Partida-Martinez L. P., Hertweck C. (2007). A gene cluster encoding rhizoxin biosynthesis in “Burkholderia rhizoxina”, the bacterial endosymbiont of the fungus Rhizopus microsporus. Chem. Bio Chem. 8, 41–45. doi: 10.1002/cbic.200600393
Partida-Martinez L. P., Monajembashi S., Greulich K.-O., Hertweck C. (2007b). Endosymbiont-dependent host reproduction maintains bacterial-fungal mutualism. Curr. Biol. 17, 773–777. doi: 10.1016/j.cub.2007.03.039
Scherlach K., Partida-Martinez L. P., Dahse H.-M., Hertweck C. (2006). Antimitotic rhizoxin derivatives from a cultured bacterial endosymbiont of the rice pathogenic fungus Rhizopus microsporus. J. Am. Chem. Soc 128, 11529–11536. doi: 10.1021/ja062953o
Schipper M. A. A., Stalpers J. A. (1984). A revision of the genus Rhizopus. II. the Rhizopus microsporus-group. Stud. Mycol. 25, 20–34.
Schmitt I., Partida-Martinez L. P., Winkler R., Voigt K., Einax E., Dölz F., et al. (2008). Evolution of host resistance in a toxin-producing bacterial–fungal alliance. ISME J. 2, 632–641. doi: 10.1038/ismej.2008.19
SIAP (2020) Servicio de información agroalimentaria y pesquera. Available at: https://nube.siap.gob.mx/cierreagricola/.
Keywords: endobacteria, endohyphal bacteria, Rhizopus, Mycetohabitans, rhizoxin, fungal-bacterial Interactions, Mucorales
Citation: Cabrera-Rangel JF, Mendoza-Servín JV, Córdova-López G, Alcalde-Vázquez R, García-Estrada RS, Winkler R and Partida-Martínez LP (2022) Symbiotic and toxinogenic Rhizopus spp. isolated from soils of different papaya producing regions in Mexico. Front. Fungal Biol. 3:893700. doi: 10.3389/ffunb.2022.893700
Received: 10 March 2022; Accepted: 10 October 2022;
Published: 24 October 2022.
Edited by:
Raffaella Balestrini, Institute for Sustainable Plant Protection, National Research Council (CNR), ItalyReviewed by:
Laith Khalil Tawfeeq Al-Ani, Universiti Sains Malaysia, MalaysiaLourdes Villa Tanaca, Instituto Politécnico Nacional, Mexico
Jose Manuel Villalobos-Escobedo, Centro de Investigaciones y Estudios Avanzados, Instituto Politécnico Nacional de México Cinvestav, Mexico
Copyright © 2022 Cabrera-Rangel, Mendoza-Servín, Córdova-López, Alcalde-Vázquez, García-Estrada, Winkler and Partida-Martínez. This is an open-access article distributed under the terms of the Creative Commons Attribution License (CC BY). The use, distribution or reproduction in other forums is permitted, provided the original author(s) and the copyright owner(s) are credited and that the original publication in this journal is cited, in accordance with accepted academic practice. No use, distribution or reproduction is permitted which does not comply with these terms.
*Correspondence: Laila P. Partida-Martínez, bGFpbGEucGFydGlkYUBjaW52ZXN0YXYubXg=
†These authors have contributed equally to this work and share first authorship