- 1Instituto de Investigaciones Quimicas, Universidad Mayor de San Andrés, La Paz, Bolivia
- 2Department of Biology, Lund University, Lund, Sweden
Symbiotic strains of fungi in the genus Trichoderma affect growth and pathogen resistance of many plant species, but the interaction is not known in molecular detail. Here we describe the transcriptomic response of two cultivars of the crop Chenopodium quinoa to axenic co-cultivation with Trichoderma harzianum BOL-12 and Trichoderma afroharzianum T22. The response of C. quinoa roots to BOL-12 and T22 in the early phases of interaction was studied by RNA sequencing and RT-qPCR verification. Interaction with the two fungal strains induced partially overlapping gene expression responses. Comparing the two plant genotypes, a broad spectrum of putative quinoa defense genes were found activated in the cultivar Kurmi but not in the Real cultivar. In cultivar Kurmi, relatively small effects were observed for classical pathogen response pathways but instead a C. quinoa-specific clade of germin-like genes were activated. Germin-like genes were found to be more rapidly induced in cultivar Kurmi as compared to Real. The same germin-like genes were found to also be upregulated systemically in the leaves. No strong correlation was observed between any of the known hormone-mediated defense response pathways and any of the quinoa-Trichoderma interactions. The differences in responses are relevant for the capabilities of applying Trichoderma agents for crop protection of different cultivars of C. quinoa.
Background
Trichoderma is a genus of ascomycete fungi widely studied for its versatile interactions with other organisms. Trichoderma can feed or parasitize on other fungi, bacteria, oomycetes and nematodes (Harman et al., 2004; Verma et al., 2007). Several species of Trichoderma are also symbionts with plants and can promote plant growth by several, yet so far only partially known mechanisms. Strains of several symbiotic species in the Trichoderma harzianum species complex (e.g., Trichoderma afroharzianum T22 (Chaverri et al., 2015), previously called T. harzianum) are used commercially because they can substantially improve yields of several species of crops (Harman et al., 1989; Monte, 2001; Hermosa et al., 2012). The strain T22 can improve the soil nutrient availability to plants (Altomare et al., 1999), and several species of Trichoderma have also been shown to enhance plant growth through volatile compound emission (Lee et al., 2016; Jalali et al., 2017) and stimulate plant systemic defense responses (Harman et al., 2004; Mukherjee et al., 2013). Nevertheless, plants do not always benefit from these interactions as described for some maize cultivar in field trials and lab experiments (Harman, 2006). Plant growth inhibition by T. harzianum has also been observed in quinoa seedlings after six but not two days of axenic co-culture (Rollano-Peñaloza et al., 2018).
Quinoa (Chenopodium quinoa Willd.) is an emerging crop of great interest due to its nutritional values (Vega-Gálvez et al., 2010) and its resistance to hostile environmental conditions, especially salinity and drought (Ruiz et al., 2014; Bazile et al., 2016). Quinoa seeds are gluten-free, contain all essential amino acids and its composition (vitamins, antioxidants, fatty acids and minerals) is highly suitable for human nutrition (Repo-Carrasco et al., 2003). Quinoa has a high genetic diversity, e.g., >4,000 accessions have been registered by the Food and Agriculture Organization (Zurita-Silva et al., 2014). The high genetic diversity of cultivars is the result of many years of selection by the indigenous people of the Andean Altiplano, where quinoa may have been domesticated 7,000 years ago by pre-Columbian cultures (Dillehay et al., 2007).
Quinoa agricultural yields can be boosted by Trichoderma application, as previously described (Ortuño et al., 2013). However, the outcome of plant-Trichoderma interactions is not always beneficial. Plant genotype-specific growth inhibition by commercially available Trichoderma strains have been reported for lentils (Prashar and Vandenberg, 2017), tomato (Tucci et al., 2011) sugarbeet (Schmidt et al., 2020) and maize (Harman, 2006). Thus, the incompatibility of particular plants with particular biocontrol strains can lead to undesired agricultural losses. Therefore, there is a need to understand the genotype-specific mechanisms that determine beneficial plant growth effects upon treatment with biocontrol agents like T22. Among the beneficial plant growth mechanisms triggered by biocontrol agents and mycorrhiza, the activation of plant defenses is one of the most studied topics. Plant defenses activated by mycorrhiza have been shown to include expression of Germin-like proteins (GLPs) (Fiorilli et al., 2018), and plant-fungi protein-protein interaction studies have shown that GLPs interact with Trichoderma cellulases (Saravanakumar et al., 2018). However, no studies about effects of Trichoderma on GLPs expression have been reported.
GLPs are plant proteins that have been especially well studied in grains like barley. These proteins are characterized by being associated with several enzymatic activities including oxalate oxidase (OXO), superoxide dismutase (SOD), ADP-glucose pyrophosphatase/ phosphodiesterase (AGPPase) and polyphenol oxidase (PPO) (Zimmermann et al., 2006; Dunwell et al., 2008). A potential function of germin-like proteins (GLPs) is found in its OXO and SOD activities, which may play a key role in production of hydrogen peroxide (H2O2) during plant defense (Ilyas et al., 2016). Because of a potential importance of GLP in protecting plant cells from superoxide toxicity produced under pathogen attacks, germin or GLP genes (i.e., HvOXO1) have been inserted into dicot plants like rapeseed or peanut to enhance their pathogen resistance (Livingstone et al., 2005; Dunwell et al., 2008; Liu et al., 2015).
In this work, we have studied the molecular response of two C. quinoa cultivars that have been shown to experience plant growth inhibition when treated over longer time with T. harzianum BOL-12 and T. afroharzianum T22 in axenic co-cultures. The response of quinoa to BOL-12 and T22 in the early phases of interaction was studied by transcriptomic analysis and RT-qPCR verification. Overall, we observed that upon interaction with the two fungal strains, a broad spectrum of putative quinoa defense genes were activated in Kurmi but not in the Real cultivar.
Methods
Biological Materials
Seeds of quinoa (Chenopodium quinoa Willd.) cultivars Maniqueña Real (Real) and Kurmi were kindly supplied by PROINPA (Quipaquipani, Bolivia). Trichoderma afroharzianum, Rifai, T22, anamorph ATCC 20847 (Chaverri et al., 2015) was purchased from the American Type Culture Collection (Manassas, VA, USA). Trichoderma harzianum BOL-12QD (BOL-12) was isolated and provided by the Instituto de Investigaciones Farmaco-bioquímicas (IIFB-UMSA, La Paz, Bolivia) (Rollano-Peñaloza et al., 2018). The study was performed under pertinent institutional, national and international guidelines and legislation.
Fungal Growth
T22 and BOL-12 were maintained on potato dextrose agar (BD-Difco, Detroit, USA) at 25°C. To isolate conidiospore suspensions, one ml of sterile water was added to two-week-old Trichoderma cultures on potato dextrose agar and collected spores were filtered through a sterile piece of cotton wool. The spores were washed twice with sterile H2O (Milli-Q, Merck Millipore, Burlington, MA, USA) and pelleted at 3700g for 5 min at 4 °C in an Allegra X-12R centrifuge (Beckman, Brea, CA, USA). Spores were resuspended in sterile H2O and kept at 4°C until experiments.
Germination of T22 and BOL-12 spores for C. quinoa treatment was performed as described by Yedidia, Benhamou (Yedidia et al., 1999) using 15 ml tubes shaken at 200 rpm for 18 h. The germinated spore suspension was washed twice by centrifugation as described above and finally resuspended in sterile H2O. The final spore concentration was adjusted to be 1 germinated spore/μl and verified by colony forming unit (CFU) counts in potato dextrose agar Petri dishes.
Disinfection of C. quinoa Seedlings and Germination
Seeds of C. quinoa were soaked in commercial bleach (NaClO; 27 g/kg) for 20 min., followed by six rinses in sterile H2O. Immediately thereafter, the seeds were placed on sterile water agar (8 g/L) in Petri dishes and incubated in darkness at 24°C for 14 h (Rollano-Peñaloza et al., 2018).
Co-culture of Quinoa and T. harzianum in Petri Dishes
Five germinated axenic seedlings of each cultivar Kurmi and Real with similar root length were aligned in a straight line on each 12 x 12 cm square Petri dishes containing 0.1X Murashige and Skoog Basal Salts Mixture (MS; Duchefa, Haarlem, The Netherlands), supplemented with 8 g/L agar. The Petri dishes were tilted 45° during growth with the agar/air interface facing upwards and seedlings having the roots pointing toward the bottom part of the Petri dish. The seedlings were incubated at 24°C for 4 h before treatment with T22 or BOL-12.
C. quinoa seedlings were treated by adding 10 μl [1 CFU/μl] of either T22 or BOL-12 germinated spore suspension on the neck of the primary root. Ten μl of sterile H2O was added to each seedling in the mock control group. After treatment, the seedlings were incubated at 24°C in a 16 h light /8 h dark photoperiod. Co-cultivation was done under fluorescent lights (Polylux XLr 30W, GE, Budapest, Hungary) at 50 μmol m−2 s−1 for 12 and 36 h.
Seedling Growth Analysis
Hypocotyl length was analyzed from images taken with a Digital Camera Canon EOS Rebel T3. Measurements from the photographs were done with the segmented line tool of ImageJ 1.49 (Abramoff et al., 2004).
Sample Collection and RNA Extraction
For RNA extraction quinoa seedling were sampled 12 and 36 h after Trichoderma treatment. Each treatment enclosed five plates containing five seedlings. To reduce inter-plate variability, one root from each of the five plates was pooled for a biological replicate into pre-weighed aluminum foil envelopes. Roots were excised at the root-hypocotyl interface with a scalpel. The envelopes were weighed on a precision balance and shock-frozen in liquid nitrogen. Frozen samples were either processed immediately or stored at −80°C until RNA extraction. Roots and shoots were pooled separately.
Total RNA was extracted using the RNeasy Plant Mini Kit (Qiagen, Valencia, CA, USA), with the following modification: Root tissue samples preserved in liquid nitrogen were placed in a precooled mortar containing liquid nitrogen followed by thoroughly grinding without letting the samples thaw. Then 450 μL of Buffer RLT (Qiagen, Valencia, CA, USA) supplemented with B-mercaptoethanol (1%) was added. Grinding continued until samples thawed and were transferred to a 1.5 ml microcentrifuge tube. The rest of the procedure was followed according to Qiagen instructions. Total RNA quantity and quality was determined with a NanoDrop spectrophotometer. DNase treatment was performed with the DNA-free kit (Ambion, Carlsbad, CA, USA), following the instructions of the manufacturer. The integrity and quality of the RNA was determined as follows: 500 ng of DNase-treated RNA were dissolved in 8 μl of sterile water and split in two aliquots, one placed on ice and the other placed at 37°. After 20 min incubation, 2 μl of loading buffer was added to each sample and both aliquots were loaded on an agarose gel (2%) stained with ethidium bromide. The gel was run at 80 V for 30 min and visualized in an UV-transilluminator. Samples with sharp 18S and 28S rRNA and showing no evidence of degradation were retained.
RNA-Seq Library Construction and Sequencing
Total RNA treated with DNase was sent to IGA technology services (IGA, Udine, Italy; http://www.igatechnology.com) for poly(A)+ mRNA purification, strand-specific cDNA synthesis, library construction (Truseq stranded mRNA-seq) and sequencing using a HiSeq2500 (Illumina Inc., San Diego, CA, USA) in paired-end mode with a read length of 125 bp. Raw sequences have been deposited at the National Center of Biotechnology Information (NCBI) under project accession number: PRJNA720675.
Transcriptomic Analysis
RNA-seq reads were checked for quality by FastQC (v.0.9.0) and mapped on the quinoa genome “Kd” (Yasui et al., 2016a) and to the QQ74 coastal genome (Jarvis et al., 2017) by Tophat2 (v.2.2.9). Transcript abundances were assessed with HTSeq (v.0.9.1) with “intersection-nonempty” mode. Genes that had a minimum of 1 read mapped in each of the samples considered for analysis were included. Gene expression levels were measured as counts per million (CPM) (Anders et al., 2013). Library size normalization was performed using the trimmed mean of M-values (TMM) within the R package edgeR (v.3.14.0) (Robinson et al., 2010; Dillies et al., 2013). CPM were TMM-normalized in order to compensate for library size differences. Differential gene expression analysis comparing mock-treated samples with samples treated with Trichoderma was performed using edgeR with TMM normalized libraries (Anders et al., 2013) with a false discovery rate (FDR) of 5% (q < 0.05) (Benjamini and Hochberg, 1995).
Functional Annotation of Differentially Expressed Genes
Gene ontology (GO) term enrichment for sets of differentially expressed genes were estimated with Argot2 through sequence function prediction (Falda et al., 2012). Singular enrichment analysis (SEA) for biological processes was performed with AgriGO v2.0 (Du et al., 2010). The statistical test for SEA was Fisher's exact test and for false discovery rate the Yekutieli method was applied (Tian et al., 2017).
cDNA Synthesis and Gene Expression by qRT-PCR Analysis
Synthesis of cDNA was carried out with 500 ng of total RNA added to each 20 μl reaction of the RevertAid H Minus Reverse Transcription Kit (Thermo Scientific). The cDNA samples were stored at −20°C for downstream analysis. qRT-PCR of plant RNA was performed in a CFX384 Touch Real-Time PCR system (Bio-Rad, Hercules, CA, United States) using Maxima SYBR Green qPCR Master Mix (Thermo Scientific) supplemented with 0.25 μM of each specific primer and 10 ng of cDNA as template in a total volume of 10 μl/reaction. The PCR program had the following conditions: 1 cycle of: 95°C, 20 s; 30 cycles of: (95° C, 15 s; 60°C, 20s; 72 °C, 20s). The specificity of each PCR amplification was determined by melting curve analysis and by analysis in 2% agarose gels. The primer sequences can be found in Supplementary Table 7. The relative transcript expression was calculated by the Pfaffl algorithm, using CqACT2A and CqMON1 as endogenous genes for normalization. CqACT2A and CqMON1 were selected based on their stable expression as reference genes in Arabidopsis (Wallström et al., 2012). Ten-fold dilutions of cDNA template were used to determine the amplification efficiency for each gene (Pfaffl, 2001).
Primer pairs were designed using Perlprimer (Marshall, 2004) so that one of the primers in each pair spanned an exon-exon border, and the primer pairs were additionally checked using Netprimer (premierbiosoft.com) to avoid primer-primer interactions.
Evolutionary Analysis of GLP
Protein alignments were made using Muscle (Edgar, 2004). Aminoacid substitution models were evaluated by MEGA X (Kumar et al., 2018). The protein evolutionary tree was performed by maximum likelihood using LG model (Le and Gascuel, 2008) with gamma distribution (LG + G) and 95% limit for partial gaps. Total positions in the final dataset were 191. Bootstrap testing was conducted with 1000 replicates.
Results
Transcriptome Sequencing of C. quinoa in Axenic Co-culture With Trichoderma
Two cultivars of quinoa were grown axenically and subjected to 36 h treatments with the Trichoderma strains T22 and BOL-12 for gene expression analyses to detect molecular responses. During this short-term treatment, the effect of Trichoderma on seedlings was not measurable, consistent with previous observations (Rollano-Peñaloza et al., 2018).
Five RNA samples from quinoa roots treated with Trichoderma for 12 and 36 h were collected. The three RNA samples at 12 h post inoculation (hpi) showing best quantity and quality parameters were selected for RNA-seq. Similarly, three RNA samples at 36 hpi were selected to be analyzed with qRT-PCR. Sequencing was carried out in paired-end mode, and the final number of reads that passed the quality control varied between 10.2 and 23.1 million paired-end reads of 125 bp per sample (Table 1). Reads were mapped to the draft quinoa genome of cultivar Kd as well as to the chromosome-level assembly of the quinoa genome cultivar QQ74 (Table 1). On average, the proportion of mapped reads was substantially increased when reads were mapped to the QQ74 quinoa genome (93.9 %), as compared to the draft Kd quinoa genome (71.8%). Therefore, all downstream analyses were performed with data mapped to the QQ74 genome.
Differential Gene Expression in Quinoa in Response to Trichoderma Treatment
The differential gene expression analysis considered only reads that mapped to unique locations in the QQ74 genome. The average number of reads that were mapped to unique locations in the QQ74 genome was 89.8% (Table 1). The remaining reads (10.2%) producing multiple alignments were discarded. Further, only quinoa genes with at least one read in each of the samples analyzed were considered (Table 2).
Quinoa roots in general induced more genes than they repressed upon interaction with Trichoderma, with the exception of Kurmi interacting with T22 where more genes were repressed than induced (Table 2). Kurmi treated with T22 showed 16 times more differentially expressed genes than in the treatment with BOL-12. Similarly, quinoa cv. Real treated with T22, compared to the mock-controls, had 5.5 times more differentially expressed genes than Kurmi in the treatment with BOL-12 (Table 2).
Regarding communal effects by both Trichoderma strains, we observed more genes differentially expressed in cv. Real (141 genes) than in cv. Kurmi (75 genes) (Supplementary Tables 1–3). Among the quinoa genes up- or downregulated under one or several conditions, only 19 were communally differentially expressed in all experimental combinations, and all were induced (Figure 1, Table 3). That is, they were significantly induced during the interaction of each quinoa cultivar with each Trichoderma strain. The group of 19 differentially expressed genes were not significantly associated with any functional GO term upon analysis by SEA. However, the structural GO analysis suggests that 13 of the 19 gene products are localized outside the cytoplasm. This suggests an association of the response pattern to functions of the plasma membrane, the cell wall and the extracellular compartment, indicating functions relating to interactions with external stimuli (Table 3).
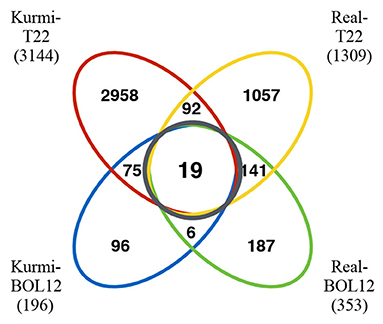
Figure 1. Venn diagram of quinoa genes differentially expressed in response to Trichoderma. Quinoa genes differentially expressed were grouped according to the cultivars and Trichoderma strains studied. The black circle indicates genes differentially expressed in both quinoa cultivars by each of the Trichoderma strains tested. The numbers in parenthesis indicate the number of genes differentially expressed in each of the quinoa-Trichoderma interactions studied.
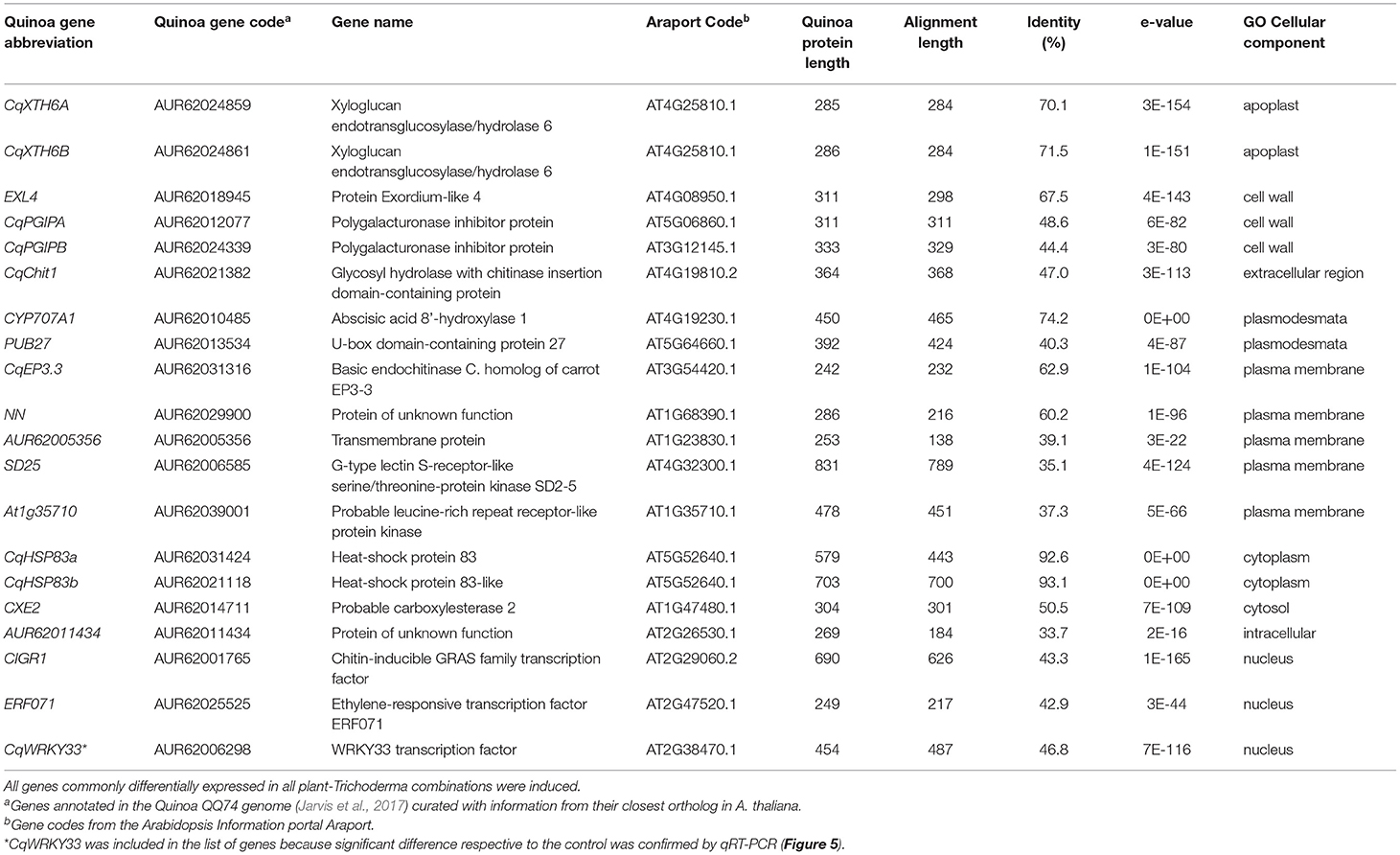
Table 3. Quinoa genes differentially expressed in both Kurmi and Real in response to both Trichoderma strains.
Quinoa Genes Differentially Expressed Unique to Each Cultivar
We decided to analyze genes that were induced by Trichoderma and were uniquely expressed in each cultivar. The Kurmi cultivar upon interaction with either BOL-12 or T22, expressed 75 genes that were communally differentially expressed (DE) but were not differentially expressed upon either Trichoderma interaction in cv. Real (Figures 1, 2, Supplementary Table 1). The expression profiles of these genes were clustered by Euclidean distance and are shown in Figure 2. From the 75 DE genes in cv. Kurmi by both strains of Trichoderma, 59 genes were induced (Supplementary Table 1), whereas 16 DE genes were repressed (Supplementary Table 2). The 75 DE genes expressed in cv. Kurmi are expressed in cv. Real but are not responsive to the treatment with either of the Trichoderma strains (Figure 2).
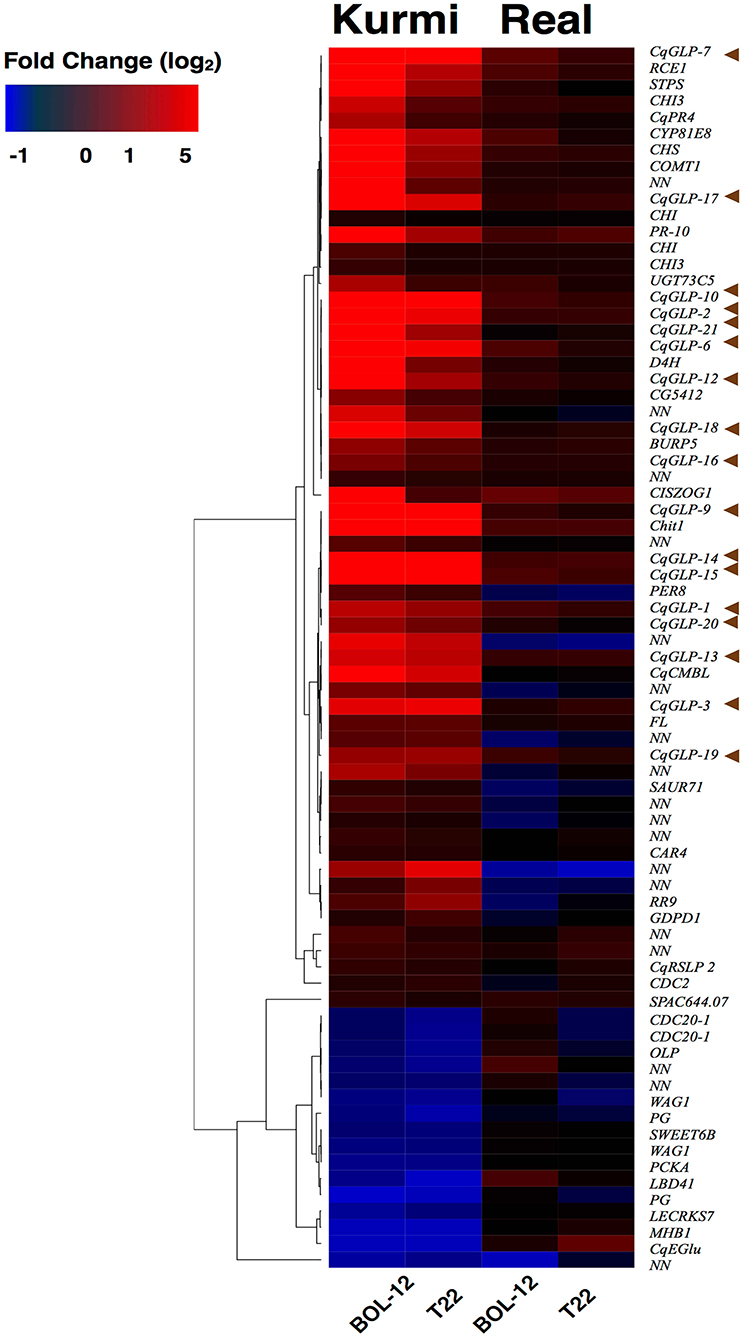
Figure 2. Heatmap profile of genes that respond to to Trichoderma BOL-12 and T22 in cv. Kurmi but not in C. quinoa cv. Real. Genes differentially expressed in cv. Kurmi in response to either Trichoderma strain, but not significantly responsive in cv. Real, were analyzed. Clustering by Euclidean distance shows the similarity in expressional change upon Trichoderma treatment. Brown arrows indicate CqGLPs.
Analysis of the 59 significantly induced genes revealed that 17 genes (CqGLPs) are highly expressed and share a high protein sequence identity (90%). These genes encode proteins that belong to the germin-like protein family (GLPs) (Figure 2; Supplementary Table 1). Further, several genes involved in flavonoid biosynthesis were specifically responsive in Kurmi. We identified 9 genes whose orthologs in Arabidopsis thaliana are described to be involved in the flavonoid biosynthesis pathway. These differentially expressed genes are orthologs to four out of five enzyme-coding genes necessary for production of flavonol glycosides from naringenin, also known as chalcone (Dao et al., 2011) (Figure 2, Supplementary Table 1).
The Real cultivar had 141 genes differentially expressed common to both Trichoderma strains tested (Figure 1, Supplementary Table 3). The cv. Real response to both Trichoderma strains showed mostly activation of transcription factors and enzymes without a significant match to a known pathway. Among the genes that were differentially expressed there are four ethylene-responsive transcription factors (ERFs), nine probable WRKY transcription factors and three chitinases (Supplementary Table 3).
Genes differentially expressed related to biotic interactions were observed in a larger proportion in quinoa cv. Kurmi than Real. Therefore, the focus of this study was on the response of the Kurmi cultivar.
Functional Annotation of Differentially Expressed Genes
To assess the function of the differentially expressed genes we annotated the differentially expressed genes of all combinations with Gene Ontology (GO) terms for biological processes. The quinoa genome has 44 776 annotated genes (Jarvis et al., 2017) but the annotation with Argot only assigned GO terms to 50.5 % of the genes (i.e., 22 650 genes annotated with GO terms). Despite the low percentage of GO terms assigned, GO annotation for the biological process category in Kurmi plants treated with BOL-12 revealed defense response (GO:0006952) and response to biotic stimulus (GO:0009607) as the main and only processes associated to Trichoderma BOL-12 treatment (Supplementary Table 4). In contrast, the interaction between Kurmi and T22 did not show any significant GO term for biological processes.
Quinoa plants of the Real cultivar had more genes associated to GO terms than Kurmi. However, no specific association to a cluster of similar GO terms were observed (Supplementary Table 4). Specifically, Real treated with T22 showed 38 genes that were annotated as stress response genes (GO:0006950) and 6 genes that were annotated to chitin catabolic processes (GO:0006032) and associated redundant GO terms (Supplementary Table 4). Further, Real treated with BOL-12 did not show any GO terms directly associated to defense response or response to stress, yet highest significance was observed to GO terms for cell wall related processes (Supplementary Table 4). Nonetheless, in the interaction between Real and each strain of Trichoderma we observed several genes related to defense being commonly activated. Among them were WRKY transcription factors (nine differentially expressed genes), ethylene-responsive genes (4 differentially expressed genes) and chitinases (three differentially expressed genes) (Supplementary Table 3).
Validation of RNA-Seq With qRT-PCR
Quinoa root transcriptomes have not previously been analyzed. We therefore validated the gene expression data obtained by RNA-seq by performing qRT-PCR for 10 selected genes, including induced, repressed and stably expressed genes (Figure 3, Supplementary Table 5). A log-log linear model analysis of the RNA-seq data and the qRT-PCR data showed a strong correlation (R2) of 0.848. The correlation was higher when the different quinoa-Trichoderma interaction pairs were assessed independently (Supplementary Table 5).
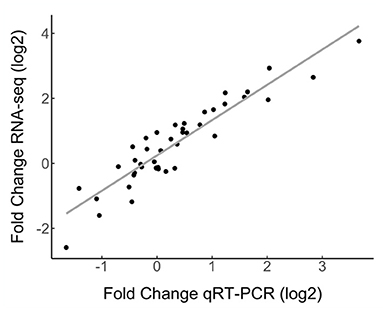
Figure 3. Correlation of RNA-seq and qRT-PCR gene expression data. Ten differentially expressed genes and two reference genes from the RNA-seq dataset were evaluated by qRT-PCR. Gene expression by qRT-PCR was normalized to the CqAct2 reference gene. Fold change was measured by comparing samples treated with Trichoderma against mock- treated. The selected genes were assessed in all quinoa-Trichoderma combinations as averages of triple biological replicates. The Pearson correlation coefficient between the RNA-seq and qRT-PCR data was 0.921 (For data see Supplementary Table 5).
Changes in Root Gene Expression at 36 hpi
Time changes in the expression of quinoa genes by Trichoderma treatment were assessed by qRT-PCR at 36 hpi. We followed time-dependent changes in two highly induced genes (CqGLP1 and CqGLP10) representing the GLP family and one gene that was induced in all quinoa-Trichoderma interactions (CqHSP83). The gene expression of CqGLP1 and CqGLP10 was reduced at 36 hpi compared to 12 hpi in the Kurmi cultivar but its expression was still higher than the mock-treatment. In contrast, the gene expression of CqGLP1 and CqGLP10 in the Real cultivar was higher at 36 hpi than at 12 hpi, being statistically significant in the Real - BOL-12 interaction (Figure 4). The CqHSP83 gene maintained its level of gene expression between 12 and 36 hpi by application of T22 in both cultivars. In contrast, the application of BOL-12 to both cultivars downregulated CqHSP83 gene expression at 36 hpi as compared to 12 hpi. However, the downregulation was only significant in the Kurmi cultivar (Figure 4). Overall, the results suggest that the induction of the analyzed genes is slower in cv. Real than in cv. Kurmi.
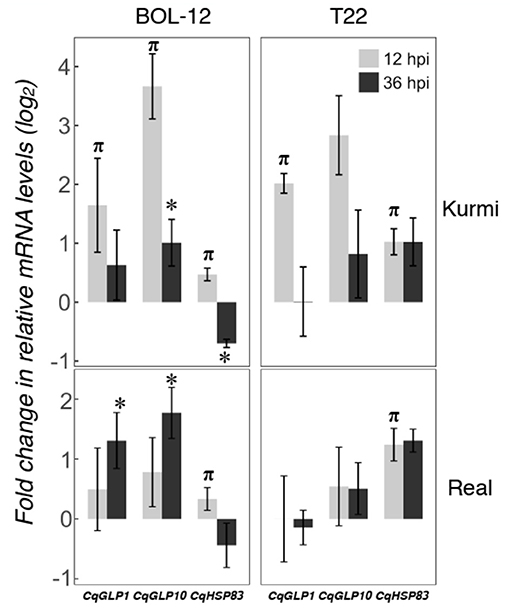
Figure 4. Gene expression at 12 and 36 hpi in representative CqGLPs and CqHSP83 in quinoa roots treated with Trichoderma. Quinoa cv. Kurmi and cv. Real root samples treated with Trichoderma BOL-12 and T22 strains were assessed at 36 hpi by qRT-PCR. mRNA levels were normalized to the CqAct2 reference gene. Fold changes (mean ± SE) were determined by comparing samples treated with Trichoderma against mock-treated ones. Asterisks denote significant changes in the gene expression as compared to the control treatment by qRT-PCR at 36 hpi (p < 0.05). Symbol π denotes significant changes in the gene expression as compared to the control treatment, using RNA-seq (p < 0.05) and confirmed by qRT-PCR (p < 0.05) at 12 hpi.
Shoot Gene Expression
We investigated changes in the quinoa shoot gene expression 36 h after Trichoderma application to the root neck (Figure 5). Ten out of 12 genes investigated were also expressed in the shoots, CqPER39 and CqPR1C gene expression was not detected in the shoots in any of the combinations studied (Supplementary Table 6). Trichoderma-induced gene expression changes in the shoots (Figure 5) showed a generally similar pattern of gene expression as observed in the roots at 36 hpi (Figure 4). CqGLP1 and CqGLP10 are significantly expressed in both cultivars upon interaction with BOL-12 but not with T22. Likewise, CqHSP83 is significantly expressed in both cultivars when interacting with T22 but not when interacting with BOL-12 (Figure 5). The other genes did not show a significant correlation in the shoot-root expression in any of the quinoa-Trichoderma interactions studied (Supplementary Table 6).
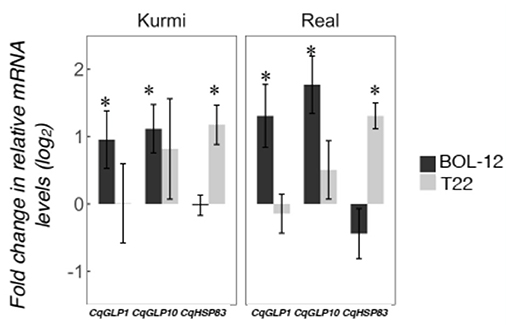
Figure 5. Gene expression changes in quinoa shoots after treatment of roots with Trichoderma. Quinoa shoot samples were assessed by qRT-PCR 36 h after treatment with Trichoderma to the roots. Gene expression was normalized to the CqAct2 reference gene and is shown as mean ± SE. Asterisks denote significant changes in the gene expression as compared to the control treatment (p < 0.05). Two CqGLP genes that were induced in Kurmi roots at 12 hpi as well as the CqHSP83 gene, which was induced in roots in all quinoa-Trichoderma interactions at 12 hpi are shown.
Evolutionary Analysis of the Germin-Like Proteins
Plant germins were first investigated and have been characterized in most functional detail in cereals (Ilyas et al., 2016). To investigate the coincidental induction of germin-like proteins in cv. Kurmi (Supplementary Table 1), we carried out BLAST searches to identify all germin and germin-like homologs in C. quinoa, Beta vulgaris, A. thaliana and Hordeum vulgare and performed alignments and evolutionary analyses. We found that 16 of the 17 quinoa GLPs induced by Trichoderma (highlighted in green) in cv. Kurmi belong to a single (98% bootstrap) C. quinoa-specific clade of 29 homologs (Figure 6, Supplementary Table 8). The remaining GLP induced by Trichoderma (CqGLP20) is grouped in an unresolved putative clade, which contains four non-expressed quinoa GLPs and one sugarbeet GLP (Figure 6). Quinoa germin-like proteins were significantly associated with specific homologs in B. vulgaris. Species-specific gene groups were also observed for B. vulgaris and A. thaliana. The result suggests that recent expansions of gene groups have occurred independently in the amaranth family species C. quinoa and B. vulgaris.
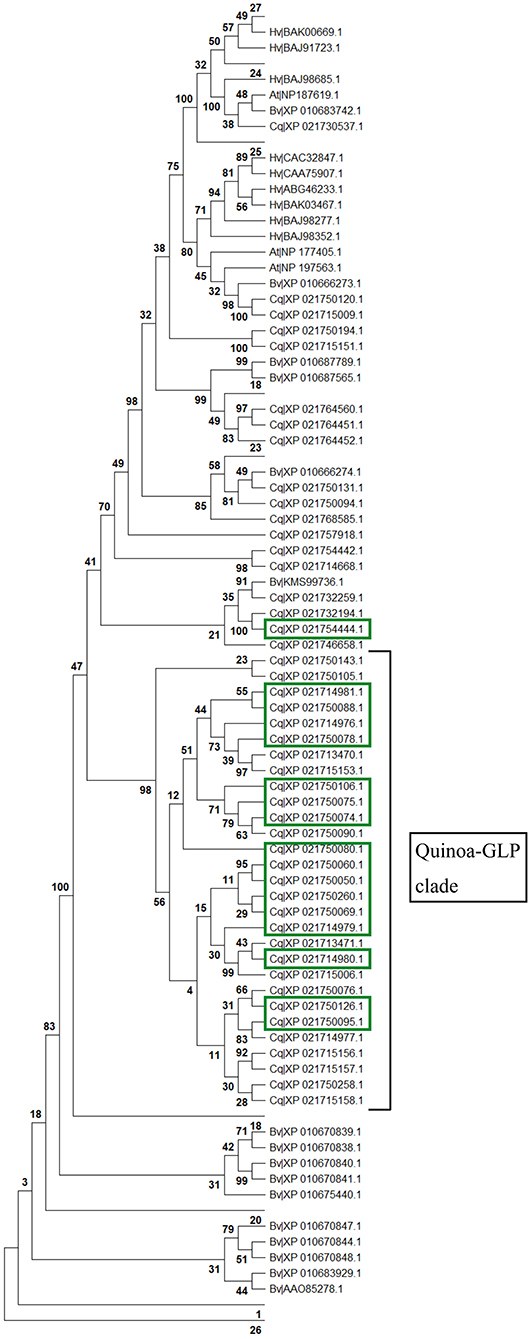
Figure 6. Protein evolutionary tree of germin and germin-like proteins. All identified germin-like proteins found in C. quinoa (Cq), B. vulgaris (Bv), A. thaliana (At) and H. vulgare (Hv) homologs were aligned by Muscle. The protein evolutionary tree was constructed by maximum likelihood using the LG + G model with 1,000 iterations. Bootstrap values are given in percentage (%). Values below 30% are not shown except for in the Trichoderma-responsive quinoa-specific GLP clade. The Trichoderma-induced homologs are marked in green.
Predicted structure of quinoa GLP1 shows a highly conserved structure compared to the crystal structure of Barley Germin (PDB: 1FI2), which has oxalate oxidase and manganese superoxide dismutase activities. The model suggest that the protein active site with aminoacid residues that bind to manganese is conserved and thus CqGLP1 might have a potential enzymatic activity (Figure 7).
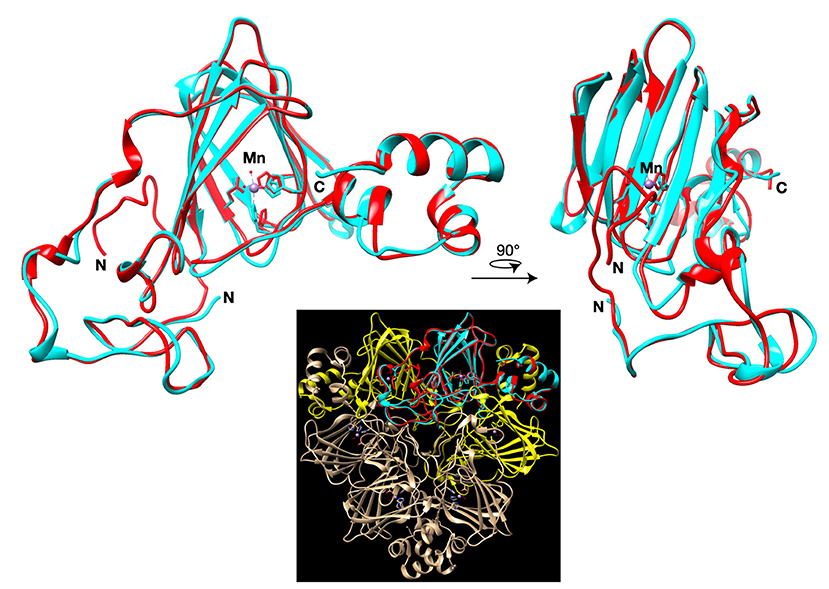
Figure 7. I-TASSER model of the C. quinoa GLP1. Whole quinoa germin-like protein (red) aligned to the template crystal structure of barley germin (oxalate oxidase; pdb:1FI2; cyan). The manganese ion of the active site is shown as a purple sphere. Inset shows the alignment integrated in the hexameric germin structure with neighboring germin monomers colored yellow.
Discussion
The outcome of plant-Trichoderma interactions with respect to both growth and physiological changes has been shown to be genotype-specific regarding both the plant and the Trichoderma biomaterial (Harman et al., 2004; Tucci et al., 2011). Here, we have observed a small set of quinoa genes being responsive in all combinations of Trichoderma strains and quinoa cultivars studied. However, we have found many more genes that are differentially expressed by a specific quinoa cultivar in response to either or both of two Trichoderma strains (Figure 1).
The set of 19 genes that showed significant responses in all cultivar-strain combinations mainly include genes connected to biotic stress response and cell wall modification (Table 3). Orthologs of these genes are known to be involved in defense response. For example, the polygalacturonase inhibitor protein AtPGIP1 (AT5G06860) in A. thaliana is thought to inhibit cell wall pectin degrading enzymes, commonly produced by fungal pathogens (Lorenzo and Ferrari, 2002). Xyloglucan endotransglucosylase/hydrolases are cell wall repairing enzymes, many of which are induced by fungal infection (Miedes and Lorences, 2007). Further, two highly similar (protein sequence identity of 94.3%) heat-shock proteins (CqHSP83A and CqHSP83B) annotated to be involved in general stress responses (GO:0006950) were upregulated in all quinoa-Trichoderma interactions. Large heat-shock proteins (70–90 kDa) are known to be involved in plant defense response through the stabilization of protective plant proteins (Hubert et al., 2003; Takahashi et al., 2003; Zhang et al., 2015). The heat-shock proteins expressed by quinoa might have been induced to contribute to the stabilization of defense proteins that would prevent or counteract damages induced by Trichoderma (Rollano-Peñaloza et al., 2018).
Several differences in the defense response patterns between the quinoa cultivars were observed. Especially, the Kurmi cultivar displays specific activation of several homologs to biotic stress-associated plant genes (Supplementary Tables 1, 2). In contrast, the responsive genes in cv. Real were mostly involved in general cellular processes (Supplementary Table 3), and to a lesser extent involving defense response genes. The defense response gene set induced in cv. Real was also completely different from the one activated in Kurmi (Figure 1, Table 2, and Supplementary Tables 1–3). Surprisingly, in neither case an obvious association to known major pathogen response pathways like jasmonic acid, salicylic acid or ethylene pathways (Pieterse et al., 2009; Salas-Marina et al., 2011; Mathys et al., 2012) could be observed in the GO analysis at 12 hpi. The low association of the quinoa differentially expressed genes with these known pathways could, however, be caused by the relatively low level of GO annotation observed for the quinoa genome, which limits the statistical discovery power of the GO analysis for quinoa genes.
The quinoa genes specifically induced in the Kurmi cultivar upon interaction with Trichoderma (Supplementary Table 1) resemble a set of defense response genes observed in plant-pathogenic interactions (Dixon, 2001). Several of these induced genes (chalcone synthase, chalcone isomerase, flavonol synthase, UDP glycosyl transferase and cis-zeatin O-glucosyltransferase) belong to the flavonoid biosynthetic pathway (Dao et al., 2011). Flavonoids have an important role in plant defense (Dixon, 2001; Treutter, 2006). For example, some A. thaliana mutants lacking the UDP glycosyl transferase gene (AtUGT74F1) are more susceptible to Pseudomonas syringae infection than the wild-type (Boachon et al., 2014). Further, a QTL analysis for pathogen resistance in soybean identified two UDP glycosyl transferase genes as the candidate genes responsible for resistance to Fusarium (Cheng et al., 2017). Thus, the Kurmi cultivar might be producing flavonol glycosides in order to prevent damage from Trichoderma overgrowth.
The Kurmi cultivar specifically induced several plant defensins that belongs to the germin and GLP family (Figure 6 and Supplementary Table 1). The Trichoderma-responsive GLPs form a majority (16 genes out of 29) of a recently expanded quinoa-specific clade (Figure 6, Supplementary Table 8), which is thus strongly connected to Trichoderma interaction. Two of the GLP genes were further tested, and were found to be also induced in leaves (Figure 5). The timing of the induction further indicated that GLPs are induced in both Kurmi and Real, albeit more slowly in Real (Figure 4). Given that several quinoa GLPs were expressed upon interaction with Trichoderma, it is very likely that these GLP defensins have an important role in the plant immune response of quinoa. Resistance to microbe attacks has been previously connected to the speed of response in different cultivars (Pritsch et al., 2000; Venisse et al., 2002). Thus, the rapid induction of a cluster of GLPs in both roots and leaves of Kurmi compared to Real (Supplementary Tables 2, 3; Figure 4) makes these genes potential candidates for breeding to increase the tolerance to microbial attacks in quinoa plants.
The set of defense-related genes (peroxidases, chitinases, ERF and WRKY transcription factors) induced in quinoa cv. Real upon interaction with Trichoderma (Supplementary Table 3) has been observed in L. japonicus roots upon 1 h of incubation with chitin oligosaccharides (Nakagawa et al., 2011). However, the levels of such defense-related genes returned to normal after 7 h in L. japonicus whereas in quinoa remained induced after 12 h, possibly due to the persistance of interaction with the living Trichoderma agent as compared to the transient nature of the elicitor. Similar to the L. japonicus system, the 24 h interaction of Trichoderma with A. thaliana resulted in the induction of the same defense-related genes as seen here in quinoa (Brotman et al., 2013). This set of genes could thus be a basal gene response of plants after recognition of beneficial fungi like Trichoderma through chitin. In contrast, the Kurmi cultivar might have a different set of receptors that helps the plant to perceive a possible negative effect from Trichoderma and thus rapidly activate a different set of defense-related genes.
The plant root transcriptomic response to Trichoderma has been poorly studied compared to aerial parts (Vos et al., 2015). Nevertheless, it has been observed that in tomato roots the recognition of Trichoderma at 24 hpi activates ROS signaling, SA responses, cell wall modifications (Palma et al., 2019), JA responses and induction of plant defenses (Brotman et al., 2013; Ho et al., 2016). In our study, we have observed a similar pattern for ROS signaling, cell wall modifications and induction of plant defenses (Table 3, Supplementary Tables 1–3), confirming that the first response of root plants to beneficial fungi like Trichoderma is to activate defenses. Further, our study reveals that the defense response against beneficial fungi is variable between cultivars (Figure 2). The variable molecular response between cultivars to Trichoderma could help to create molecular markers of compatibility between certain plant cultivars and certain strains of Trichoderma.
In conclusion, our study suggests that Trichoderma triggers a defense response in quinoa plants. Comparing the defense response of two quinoa cultivars we can observe that the Kurmi cultivar mainly induced a set of genes involved in plant defense. In contrast, the Real cultivar did not have a clear response because most of the changes mediated by Trichoderma were related to general stress and regulation of biological processes. The Kurmi cultivar might have higher tolerance to microbe attacks due to the expression of genes involved in the biosynthesis of flavonol glycosides and a clade of GLP-defensins unique to quinoa. These genes are thus candidates for selection of quinoa cultivars with higher resistance to microbe attacks.
Data Availability Statement
The datasets presented in this study can be found in online repositories. The names of the repository/repositories and accession number(s) can be found below: https://www.ncbi.nlm.nih.gov/bioproject/PRJNA720675.
Author Contributions
AR and OR-P designed the study, analyzed the data, and wrote the paper with input from all authors who reviewed and approved the final manuscript. OR-P performed the experiments. All authors contributed to the article and approved the submitted version.
Funding
This work was funded by the Swedish International Development Agency (SIDA) in a strategic collaboration between Universidad Mayor de San Andrés (UMSA) (Bolivia) and Lund University (Sweden), under the SIDA-UMSA Cooperation Agreement 75000554; the Lars Hiertas Minne Stiftelsen (OR) and the Jörgen Lindströms Stipendiefond (OR). Genome mapping, read count and blastn computations were performed on resources provided by SNIC (Swedish National Infrastructure for Computing) through Uppsala Multidisciplinary Center for Advanced Computational Science (UPPMAX, Uppsala, Sweden) under Project SNIC b2015011/b2016265 along with resources provided by UMSA (Universidad Mayor de Sán Andres) through Centro Nacional de Computación Avanzada para Bioinformática y Genómica (CnCaBo, La Paz, Bolivia).
Conflict of Interest
The authors declare that the research was conducted in the absence of any commercial or financial relationships that could be construed as a potential conflict of interest.
Publisher's Note
All claims expressed in this article are solely those of the authors and do not necessarily represent those of their affiliated organizations, or those of the publisher, the editors and the reviewers. Any product that may be evaluated in this article, or claim that may be made by its manufacturer, is not guaranteed or endorsed by the publisher.
Acknowledgments
We are grateful to Proinpa Institute (Quipaquipani, Bolivia) for the generous donation of quinoa seeds and the Instituto de Investigaciones Farmaco-bioquímicas–UMSA (La Paz, Bolivia) for providing Trichoderma harzianum BOL-12QD.
Supplementary Material
The Supplementary Material for this article can be found online at: https://www.frontiersin.org/articles/10.3389/ffunb.2021.768648/full#supplementary-material
References
Abramoff, M. D., Magalhães, P. J., and Ram, S. J. (2004). Image processing with imageJ. Biophotonics Int. 11, 36–42.
Altomare, C., Norvell, W. A., Björkman, T., and Harman, G. E. (1999). Solubilization of phosphates and micronutrients by the plant-growth-promoting and biocontrol fungus Trichoderma harzianum Rifai 1295-22. Appl. Environ. Microbiol. 65, 2926–33. doi: 10.1128/AEM.65.7.2926-2933.1999
Anders, S., McCarthy, D. J., Chen, Y., Okoniewski, M., Smyth, G. K., Huber, W., et al. (2013). Count-based differential expression analysis of RNA sequencing data using R and Bioconductor. Nat Protoc. 8, 1765–86. doi: 10.1038/nprot.2013.099
Bazile, D., Jacobsen, S. E., and Verniau, A. (2016). The Global expansion of quinoa: trends and limits. Front. Plant Sci. 7, 622. doi: 10.3389/fpls.2016.00622
Benjamini, Y., and Hochberg, Y. (1995). Controlling the false discovery rate: a practical and powerful approach to multiple testing. J R Stat. Soc. Series B Stat. Methodol. 57, 289–300. doi: 10.1111/j.2517-6161.1995.tb02031.x
Boachon, B., Gamir, J., Pastor, V., Erb, M., Dean, J. V., and Flors, V. (2014). Role of two UDP-Glycosyltransferases from the L group of arabidopsis in resistance against Pseudomonas syringae. Eur. J. Plant Pathol. 139, 707–20. doi: 10.1007/s10658-014-0424-7
Brotman, Y., Landau, U., Cuadros-Inostroza, Á., Takayuki, T., Fernie, A. R., and Chet, I. (2013). Trichoderma-plant root colonization: escaping early plant defense responses and activation of the antioxidant machinery for saline stress tolerance. PLoS Pathog. 9, e1003221. doi: 10.1371/journal.ppat.1003221
Chaverri, P., Branco-Rocha, F., Jaklitsch, W., Gazis, R., Degenkolb, T., and Samuels, G. J. (2015). Systematics of the Trichoderma harzianum species complex and the re-identification of commercial biocontrol strains. Mycologia. 107, 558–90. doi: 10.3852/14-147
Cheng, P., Gedling, C. R., Patil, G., Vuong, T. D., Shannon, J. G., and Dorrance, A. E. (2017). Genetic mapping and haplotype analysis of a locus for quantitative resistance to Fusarium graminearum in soybean accession PI 567516C. Theor. Appl. Genet. 130, 999–1010. doi: 10.1007/s00122-017-2866-8
Dao, T. T., Linthorst, H. J., and Verpoorte, R. (2011). Chalcone synthase and its functions in plant resistance. Phytochem Rev. 10, 397–412. doi: 10.1007/s11101-011-9211-7
Dillehay, T. D., Rossen, J., Andres, T. C., and Williams, D. E. (2007). Preceramic adoption of peanut, squash, and cotton in northern Peru. Science. 316, 1890–3. doi: 10.1126/science.1141395
Dillies, M. A., Rau, A., Aubert, J., Hennequet-Antier, C., Jeanmougin, M., and Servant, N. (2013). A comprehensive evaluation of normalization methods for Illumina high-throughput RNA sequencing data analysis. Brief Bioinform. 14, 671–83. doi: 10.1093/bib/bbs046
Dixon, R. A. (2001). Natural products and plant disease resistance. Nature. 411, 843–7. doi: 10.1038/35081178
Du, Z., Zhou, X., Ling, Y., Zhang, Z., and Su, Z. (2010). agriGO: a GO analysis toolkit for the agricultural community. Nucleic Acids Res. 38, W64–70. doi: 10.1093/nar/gkq310
Dunwell, J. M., Gibbings, J. G., Mahmood, T., and Saqlan Naqvi, S. M. (2008). Germin and germin-like proteins: evolution, structure, and function. CRC Crit. Rev. Plant Sci. 27, 342–75. doi: 10.1080/07352680802333938
Edgar, R. C. (2004). MUSCLE: multiple sequence alignment with high accuracy and high throughput. Nucleic Acids Research. 32, 1792–7. doi: 10.1093/nar/gkh340
Falda, M., Toppo, S., Pescarolo, A., Lavezzo, E., Camillo, D., Facchinetti, B., et al. (2012). A Argot2: a large scale function prediction tool relying on semantic similarity of weighted Gene Ontology terms. BMC Bioinformatics. 13, S14. doi: 10.1186/1471-2105-13-S4-S14
Fiorilli, V., Vannini, C., Ortolani, F., Garcia-Seco, D., Chiapello, M., and Novero, M. (2018). Omics approaches revealed how arbuscular mycorrhizal symbiosis enhances yield and resistance to leaf pathogen in wheat. Sci. Rep. 8, 9625. doi: 10.1038/s41598-018-27622-8
Harman, G. E. (2006). Overview of mechanisms and uses of Trichoderma spp. Phytopathology. 96, 190–4. doi: 10.1094/PHYTO-96-0190
Harman, G. E., Howell, C. R., Viterbo, A., Chet, I., and Lorito, M. (2004). Trichoderma species — opportunistic, avirulent plant symbionts. Nat. Rev. Microbiol. 2, 43–56. doi: 10.1038/nrmicro797
Harman, G. E., Taylor, A. G., and Stasz, T. E. (1989). Combining effective strains of Trichoderma harzianum and solid matrix priming to improve biological seed treatments. Plant Dis. 73, 631–7. doi: 10.1094/PD-73-0631
Hermosa, R., Viterbo, A., Chet, I., and Monte, E. (2012). Plant-beneficial effects of Trichoderma and of its genes. Microbiology. 158, 17–25. doi: 10.1099/mic.0.052274-0
Ho, C-. L., Tan, Y-. C., Yeoh, K-. A., Ghazali, A-. K., Yee, W-. Y., and Hoh, C-. C. D. (2016). novo transcriptome analyses of host-fungal interactions in oil palm (Elaeis guineensis Jacq.). BMC Genom. 17, 66. doi: 10.1186/s12864-016-2368-0
Hubert, D. A., Tornero, P., Belkhadir, Y., Krishna, P., Takahashi, A., and Shirasu, K. (2003). Cytosolic HSP90 associates with and modulates the Arabidopsis RPM1 disease resistance protein. EMBO J. 22, 5679–89. doi: 10.1093/emboj/cdg547
Ilyas, M., Rasheed, A., and Mahmood, T. (2016). Functional characterization of germin and germin-like protein genes in various plant species using transgenic approaches. Biotechnol. Letters. 38, 1405–21. doi: 10.1007/s10529-016-2129-9
Jalali, F., Zafari, D., and Salari, H. (2017). Volatile organic compounds of some Trichoderma spp. increase growth and induce salt tolerance in Arabidopsis thaliana. Fungal Ecol. 29, 67–75. doi: 10.1016/j.funeco.2017.06.007
Jarvis, D. E., Ho, Y. S., Lightfoot, D. J., Schmöckel, S. M., and Borm, L. i. B. (2017). The genome of Chenopodium quinoa. Nature. 542, 307–12. doi: 10.1038/nature21370
Kumar, S., Stecher, G., Knyaz, L. M., and Tamura, C. (2018). K. MEGA X: molecular evolutionary genetics analysis across computing platforms. Mol. Biol. Evol. 35, 1547–9. doi: 10.1093/molbev/msy096
Le, S. Q., and Gascuel, O. (2008). An improved general amino acid replacement matrix. Mol. Biol. Evol. 25, 1307–20. doi: 10.1093/molbev/msn067
Lee, S., Yap, M., Behringer, G., Hung, R., and Bennett, J. W. (2016). Volatile organic compounds emitted by Trichoderma species mediate plant growth. Fungal Biol. Biotechnol. 3, 7. doi: 10.1186/s40694-016-0025-7
Liu, F., Wang, M., Wen, J., Yi, B., Shen, J., Ma, J., et al. (2015). Overexpression of barley oxalate oxidase gene induces partial leaf resistance to Sclerotinia sclerotiorum in transgenic oilseed rape. Plant Pathol. 64, 1407–16. doi: 10.1111/ppa.12374
Livingstone, D. M., Hampton, J. L., Phipps, P. M., and Grabau, E. A. (2005). Enhancing resistance to Sclerotinia minor in peanut by expressing a barley oxalate oxidase gene. Plant Physiol. 137, 1354–62. doi: 10.1104/pp.104.057232
Lorenzo, D. G., and Ferrari, S. (2002). Polygalacturonase-inhibiting proteins in defense against phytopathogenic fungi. Curr. Opin. Plant Biol. 5, 295–9. doi: 10.1016/S1369-5266(02)00271-6
Marshall, O. J. (2004). PerlPrimer: cross-platform, graphical primer design for standard, bisulphite and real-time PCR. Bioinformatics. 20, 2471–2. doi: 10.1093/bioinformatics/bth254
Mathys, J., Cremer, D. e., Timmermans, K., Van Kerkhove, P., Lievens, S., Vanhaecke, B. M, et al (2012). Genome-wide characterization of ISR induced in Arabidopsis thaliana by Trichoderma hamatum T382 against Botrytis cinerea infection. Front. Plant Sci. 3, 108. doi: 10.3389/fpls.2012.00108
Miedes, E., and Lorences, E. P. (2007). The implication of xyloglucan endotransglucosylase/hydrolase (XTHs) in tomato fruit infection by Penicillium expansum Link. J. Agric. Food Chem. 55, 9021–6. doi: 10.1021/jf0718244
Monte, E. (2001). Understanding Trichoderma: between biotechnology and microbial ecology. Int. Microbiol. 4, 1–4. doi: 10.1007/s101230100001
Mukherjee, P. K., Horwitz, B. A., Herrera-Estrella, A., Schmoll, M., and Kenerley, C. M. (2013). Trichoderma Research in the Genome Era. Annu. Rev. Phytopathol. 51, 105–29. doi: 10.1146/annurev-phyto-082712-102353
Nakagawa, T., Kaku, H., Shimoda, Y., Sugiyama, A., Shimamura, M., and Takanashi, K. (2011). From defense to symbiosis: limited alterations in the kinase domain of LysM receptor-like kinases are crucial for evolution of legume–Rhizobium symbiosis. Plant J. 65, 169–80. doi: 10.1111/j.1365-313X.2010.04411.x
Ortuño, N., Castillo, J., Claros, M., Navia, O., Angulo, M., and Barja, D. (2013). Enhancing the sustainability of quinoa production and soil resilience by using bioproducts made with native microorganisms. Agronomy. 3, 732–46. doi: 10.3390/agronomy3040732
Palma, D. e., Salzano, M., Villano, M., Aversano, C., Lorito, R., Ruocco, M. M, et al. (2019). Transcriptome reprogramming, epigenetic modifications and alternative splicing orchestrate the tomato root response to the beneficial fungus Trichoderma harzianum. Hortic. Res. 6, 5. doi: 10.1038/s41438-018-0079-1
Pfaffl, M. W. A. (2001). New mathematical model for relative quantification in real-time RT-PCR. Nucleic Acids Res. 29, e45. doi: 10.1093/nar/29.9.e45
Pieterse, C. M. J., Leon-Reyes, A., Van der Ent, S., and Van Wees, S. C. M. (2009). Networking by small-molecule hormones in plant immunity. Nat. Chem. Biol. 5, 308–16. doi: 10.1038/nchembio.164
Prashar, P., and Vandenberg, A. (2017). Genotype-specific responses to the effects of commercial Trichoderma formulations in lentil (Lens culinaris ssp. culinaris) in the presence and absence of the oomycete pathogen Aphanomyces euteiches. Biocontrol Sci. Technol. 27, 1123–44. doi: 10.1080/09583157.2017.1376035
Pritsch, C., Muehlbauer, G. J., Bushnell, W. R., Somers, D. A., and Vance, C. P. (2000). Fungal development and induction of defense response genes during early infection of wheat spikes by Fusarium graminearum. Mol. Plant-Microbe Interact. 13, 159–69. doi: 10.1094/MPMI.2000.13.2.159
Repo-Carrasco, R., Espinoza, C., and Jacobsen, S. E. (2003). Nutritional value and use of the andean crops quinoa (Chenopodium quinoa) and Kañiwa (Chenopodium pallidicaule). Food Rev. Int. 19, 179–89. doi: 10.1081/FRI-120018884
Robinson, M. D., McCarthy, D. J., and Smyth, G. K. (2010). edgeR: a Bioconductor package for differential expression analysis of digital gene expression data. Bioinformatics. 26, 139–40. doi: 10.1093/bioinformatics/btp616
Rollano-Peñaloza, O. M., Widell, S., Mollinedo, P., and Rasmusson, A. G. (2018). Trichoderma harzianum T-22 and BOL-12QD inhibit lateral root development of Chenopodium quinoa in axenic co-culture. Cogent Biol. 4, 1–12. doi: 10.1080/23312025.2018.1530493
Ruiz, K. B., Biondi, S., Oses, R., Acuña-Rodríguez, I. S., Antognoni, F., and Martinez-Mosqueira, E. A. (2014). Quinoa biodiversity and sustainability for food security under climate change. A review. Agron. Sustain. Dev. 34, 349–59. doi: 10.1007/s13593-013-0195-0
Salas-Marina, M. A., Silva-Flores, M. A., Uresti-Rivera, E. E., Castro-Longoria, E., Herrera-Estrella, A., and Casas-Flores, S. (2011). Colonization of Arabidopsis roots by Trichoderma atroviride promotes growth and enhances systemic disease resistance through jasmonic acid/ethylene and salicylic acid pathways. Eur. J. Plant Pathol. 131, 15–26. doi: 10.1007/s10658-011-9782-6
Saravanakumar, K., Wang, S., Dou, K., Lu, Z., and Chen, J. (2018). Yeast two-hybrid and label-free proteomics based screening of maize root receptor to cellulase of Trichoderma harzianum. Physiol. Mol. Plant Pathol. 104, 86–94. doi: 10.1016/j.pmpp.2018.10.002
Schmidt, J., Dotson, B. R., Schmiderer, L., van Tour, A., Kumar, B., and Marttila, S. (2020). Substrate and plant genotype strongly influence the growth and gene expression response to Trichoderma afroharzianum T22 in sugar Beet. Plants. 9, 1005. doi: 10.3390/plants9081005
Takahashi, A., Casais, C., Ichimura, K., and Shirasu, K. (2003). HSP90 interacts with RAR1 and SGT1 and is essential for RPS2-mediated disease resistance in Arabidopsis. Proc. Natl. Acad. Sci. U.S.A. 100, 11777–82. doi: 10.1073/pnas.2033934100
Tian, T., Liu, Y., Yan, H., You, Q., and Du, Y. i. X. (2017). agriGO v2.0: a GO analysis toolkit for the agricultural community, 2017 update. Nucleic Acids Res. 45, W122–W9. doi: 10.1093/nar/gkx382
Treutter, D. (2006). Significance of flavonoids in plant resistance: a review. Environ. Chem. Lett. 4, 147–57. doi: 10.1007/s10311-006-0068-8
Tucci, M., Ruocco, M., Masi, D. e., De Palma, L., and Lorito, M. (2011). The beneficial effect of Trichoderma spp. on tomato is modulated by the plant genotype. Mol. Plant Pathol. 12, 341–54. doi: 10.1111/j.1364-3703.2010.00674.x
Vega-Gálvez, A., Miranda, M., Vergara, J., Uribe, E., Puente, L., and Martínez, E. A. (2010). Nutrition facts and functional potential of quinoa (Chenopodium quinoa Willd.), an ancient Andean grain: a review. J. Sci. Food Agric. 90, 2541–7. doi: 10.1002/jsfa.4158
Venisse, J-. S., Malnoy, M., Faize, M., Paulin, J-. P., and Brisset, M-. N. (2002). Modulation of defense responses of Malus spp. during compatible and incompatible interactions with Erwinia amylovora. Mol. Plant-Microbe Interact. 15, 1204–12. doi: 10.1094/MPMI.2002.15.12.1204
Verma, M., Brar, S. K., Tyagi, R. D., Surampalli, R. Y., and Valéro, J. R. (2007). Antagonistic fungi, Trichoderma spp.: Panoply of biological control. Biochem. Eng. J. 37, 1–20. doi: 10.1016/j.bej.2007.05.012
Vos, C. M. F., Cremer, D. e., Cammue, K., and De Coninck, B. P. A. (2015). The B. toolbox of Trichoderma spp. in the biocontrol of Botrytis cinerea disease. Mol. Plant Pathol. 16, 400–12. doi: 10.1111/mpp.12189
Wallström, S. V., Aidemark, M., Escobar, M. A., and Rasmusson, A. G. (2012). An alternatively spliced domain of the NDC1 NAD(P)H dehydrogenase gene strongly influences the expression of the ACTIN2 reference gene in Arabidopsis thaliana. Plant Sci. 183, 190–6. doi: 10.1016/j.plantsci.2011.08.011
Yasui, Y., Hirakawa, H., Oikawa, T., Toyoshima, M., Matsuzaki, C., and Ueno, M. (2016a). Draft genome sequence of an inbred line of Chenopodium quinoa, an allotetraploid crop with great environmental adaptability and outstanding nutritional properties. DNA Res. 23, 535–46. doi: 10.1093/dnares/dsw037
Yasui, Y., Hirakawa, H., Oikawa, T., Toyoshima, M., Matsuzaki, C., and Ueno, M. (2016b). Draft genome sequence of an inbred line of Chenopodium quinoa an allotetraploid crop with great environmental adaptability and outstanding nutritional properties. DNA Res. 2016, dsw037. doi: 10.1093./dnares/dsw037
Yedidia, I., Benhamou, N., and Chet, I. (1999). Induction of defense responses in cucumber plants (Cucumis sativus L.) by the biocontrol agent Trichoderma harzianum. Appl. Environ. Microbiol. 65, 1061–70. doi: 10.1128/AEM.65.3.1061-1070.1999
Zhang, X-. C., Millet, Y. A., Cheng, Z., Bush, J., and Ausubel, F. M. (2015). Jasmonate signalling in Arabidopsis involves SGT1b–HSP70–HSP90 chaperone complexes. Nature Plants. 1, 15049. doi: 10.1038/nplants.2015.49
Zimmermann, G., Bäumlein, H., Mock, H-. P., Himmelbach, A., and Schweizer, P. (2006). The multigene family encoding germin-like proteins of barley. Regulation and function in basal host resistance. Plant Physiol. 142, 181–92. doi: 10.1104/pp.106.083824
Keywords: Chenopodium quinoa, transcriptomics, germin-like proteins, Trichoderma, plant-fungi interactions
Citation: Rollano-Peñaloza OM, Mollinedo PA, Widell S and Rasmusson AG (2021) Transcriptomic Analysis of Quinoa Reveals a Group of Germin-Like Proteins Induced by Trichoderma. Front. Fungal Biol. 2:768648. doi: 10.3389/ffunb.2021.768648
Received: 01 September 2021; Accepted: 04 November 2021;
Published: 01 December 2021.
Edited by:
Benjamin A. Horwitz, Technion Israel Institute of Technology, IsraelReviewed by:
Jaka Razinger, Agricultural institute of Slovenia, SloveniaLourdes Villa Tanaca, Instituto Politécnico Nacional, Mexico
Copyright © 2021 Rollano-Peñaloza, Mollinedo, Widell and Rasmusson. This is an open-access article distributed under the terms of the Creative Commons Attribution License (CC BY). The use, distribution or reproduction in other forums is permitted, provided the original author(s) and the copyright owner(s) are credited and that the original publication in this journal is cited, in accordance with accepted academic practice. No use, distribution or reproduction is permitted which does not comply with these terms.
*Correspondence: Allan G. Rasmusson, YWxsYW4ucmFzbXVzc29uQGJpb2wubHUuc2U=