- 1Idaho Cooperative Fish and Wildlife Research Unit, University of Idaho, Moscow, ID, United States
- 2U.S. Geological Survey, Idaho Cooperative Fish and Wildlife Research Unit, University of Idaho, Moscow, ID, United States
- 3Idaho Department of Fish and Game, Coeur d'Alene, ID, United States
- 4Norwegian Institute for Nature Research, Trondheim, Norway
- 5Department of Wildlife, Fish, and Conservation Biology, University of California, Davis, Davis, CA, United States
Introduction: White Sturgeon Acipenser transmontanus in the Kootenai River basin is listed as endangered in the United States and Canada. Declines have been mainly attributed to poor recruitment exacerbated by the environmental effects of Libby Dam in Montana. Reduced primary production downstream of Libby Dam has been identified as one factor limiting growth of White Sturgeon, thereby limiting natural reproduction through delayed sexual maturity. However, estimating changes in growth over time without knowledge of fish location (i.e., Kootenai River vs. Kootenay Lake) is difficult. The objective of this project was to use microchemistry analysis to describe the movement of White Sturgeon within the Kootenai River basin.
Methods: Water samples (n = 27) and White Sturgeon fin rays (n = 162) collected in the Kootenai River basin were measured for strontium isotope (87Sr:86Sr) ratios using laser ablation multicollector inductively coupled plasma mass spectrometry. All water samples and a subset of fin rays (n = 8) were also analyzed for trace elements (e.g., Sr, Ba, Mg). Fin ray annuli were measured and tested using knowledge of known age; known locations from physical capture events were assigned to age at capture.
Results: Strontium isotope analysis was unable to detect differences in the Kootenai River and Kootenay Lake using water or fin ray samples. The Kootenai River and Kootenay Lake were distinguishable using trace element data from water samples, but not fin rays. The discrepancy with trace elements appears to be associated with the physiology of fin ray growth.
Discussion: Although this study did not meet its original objective of describing the movement of White Sturgeon in the Kootenai River basin from fin ray microchemistry, our results provide insight into the potential influence of physiology on microchemistry analysis. In particular, fin ray microchemistry of slow-growing fishes may be possible in freshwater systems with further investigation into the physiological processes associated with growth and the incorporation of elements into calcified structures.
1 Introduction
Sturgeons (Acipenseridae) across the world are imperiled due to a variety of anthropogenic disturbances such as modification to flow, nutrient, and substrate dynamics (Dynesius and Nilsson, 1994; Birstein et al., 1997; Pikitch et al., 2005; Haxton et al., 2016). Sturgeons are particularly sensitive to changes in habitat because of their specific flow and habitat requirements (Birstein et al., 1997; Cech and Doroshov, 2004). The requirements for successful spawning span from environmental cues for migration (e.g., peak flows, temperature) to requirements for developing juveniles post-hatch (e.g., suitable prey, interstitial spaces; Haxton et al., 2016). In addition to vulnerabilities associated with habitat modification, sturgeons are globally exploited for caviar and meat. Sturgeons are late maturing and spawn intermittently, putting them at high risk for recruitment overfishing (Boreman, 1997). The combination of environmental changes and increased anthropogenic pressures have led to the listing of multiple species of sturgeon in the United States, including the Kootenai River population of White Sturgeon Acipenser transmontanus, as endangered under the Endangered Species Act (Haxton et al., 2016).
White Sturgeon is native to the Pacific Coast of North America from northern Mexico to the Aleutian Islands in Alaska (Hildebrand et al., 2016). White Sturgeon populations are declining across the species' distribution due to their sensitivity to habitat degradation, overharvesting, and climate change (Paragamian et al., 2002; van Poorten and McAdam, 2010; Hildebrand et al., 2016). White Sturgeon also display multiple life history types, all of which include a freshwater component (Hildebrand et al., 2016). White Sturgeon are long-lived fish (>80 years) that typically mature between 10 and 20 years of age, with males maturing at younger ages than females (Hildebrand et al., 2016). Individuals spawn periodically (every 2–10 years) during high flows associated with spring runoff (Parsley et al., 1993; Paragamian et al., 2002; Paragamian, 2012). White Sturgeon eggs require large substrate for incubation and post-hatch larvae require free-flowing water to drift into protective habitat (Parsley et al., 1993; Paragamian et al., 2002; Hildebrand et al., 2016; Counihan and Chapman, 2018). Because of the spawning ecology and early life history requirements of White Sturgeon, populations in human-altered rivers experience challenges to reproduction and recruitment (Hildebrand et al., 2016). Recruitment failure is considered the cause of decline for several White Sturgeon populations (Anders et al., 2002; Hildebrand et al., 2016). A White Sturgeon population of particular concern is the Kootenai River population of British Columbia, Idaho, and Montana (Paragamian, 2012).
White Sturgeon in the Kootenai River basin was listed as endangered under the Endangered Species Act in 1994 by the United States and under the Species at Risk Act by Canada in 2006 (Paragamian, 2012). Declines have been attributed to poor recruitment exacerbated by the environmental effects of Libby Dam in Montana (Hildebrand et al., 2016). Libby Dam was constructed in 1972 on the Kootenai River to provide flood control, hydroelectric power, and recreational opportunity. Changes to temperature and discharge regimes downstream of Libby Dam have affected environmental cues for White Sturgeon spawning, and dampened spring flows have led to the sedimentation of downstream spawning habitat (Paragamian et al., 2002; Paragamian and Wakkinen, 2002). Evidence of White Sturgeon recruitment failure was documented within the first decade after construction of Libby Dam (Partridge, 1983). One of the most concerning trends that may be related to the absence of natural reproduction is the especially slow growth of White Sturgeon in the Kootenai River. White Sturgeon in the Kootenai River are estimated to exceed 30 years of age without reaching sexual maturity, which is thought to have negatively influenced natural recruitment (Anders et al., 2002; Paragamian and Beamesderfer, 2003; Paragamian et al., 2005). Slow growth of White Sturgeon in the Kootenai River is likely a combination of environmental effects, such as cold water temperatures and low nutrient concentrations (Beamesderfer, 1993; Paragamian and Beamesderfer, 2003; Watkins et al., 2017). Hatchery supplementation of White Sturgeon may also play an important role in reduced growth rates of White Sturgeon (i.e., density dependence; Justice et al., 2009). The effects of hatchery supplementation on growth have been observed in other White Sturgeon populations. For example, growth of White Sturgeon in the upper Columbia River basin declined as density increased from hatchery supplementation (Crossman et al., 2023). A better understanding of which factors regulate growth of White Sturgeon in the Kootenai River would help guide conservation efforts in the system.
The causes for slow growth of various fishes in the Kootenai River have been studied extensively (Snyder and Minshall, 1996; Watkins et al., 2017; Hardy et al., 2022). Libby Dam traps sediment and nutrients in Koocanusa Reservoir which has led to a decrease in primary production in downstream habitats. Nutrient addition began in 2005 to address the loss in primary production and is currently the largest riverine nutrient addition project in the world (Hardy et al., 2020, 2022). Nutrient addition has successfully increased primary production in the Kootenai River near the treatment site and has had a positive effect on growth of Largescale Suckers Catostomus macrocheilus (Watkins et al., 2017; Hardy et al., 2022). Conversely, the density of Mountain Whitefish Prosopium williamsoni increased with nutrient addition which resulted in decreased growth. Inferences regarding the slow growth of White Sturgeon in the Kootenai River basin have been limited by a lack of knowledge about White Sturgeon movements between the Kootenai River and Kootenay Lake. White Sturgeon hatch and rear in the Kootenai River but may use both lake and river habitats during adulthood (Paragamian and Wakkinen, 2002; Paragamian et al., 2002; Justice et al., 2009). Managers hypothesize that growth is faster in Kootenay Lake than in the Kootenai River. Because White Sturgeon are long-lived, tracking an entire lifetime of movement is difficult (Nelson et al., 2013). White Sturgeon outlive the batteries of telemetry tags and adequate technology to monitor passive integrated transponder (PIT) tags using instream antennae in large rivers such as the Kootenai River has been absent until recently. Challenges in documenting the movement of White Sturgeon have left managers uncertain about how much time White Sturgeon spend in the Kootenai River or Kootenay Lake as they grow. Microchemistry analysis is an alternative method that has been used to evaluate movement of fishes in various habitats (Pracheil et al., 2014; Tzadik et al., 2017). As such, microchemistry analysis was identified as a potential solution to the challenges of characterizing White Sturgeon movement in the Kootenai River basin.
Microchemistry analysis is the measurement of elements that are incorporated into calcified fin rays over a fish's life (Pracheil et al., 2014). Elements and their isotopes are released into water and subsequently integrated into fin rays via respiration and diet. Some elements and isotopes are incorporated into calcified structures at values that reflect what is available in the environment (Wolff et al., 2013; Willmes et al., 2016; Sellheim et al., 2017; Hegg et al., 2019). As fish grow and move, isotopic ratios change to reflect the new environment, thereby tracking migrations throughout a fish's life. Additionally, age and growth measurements from calcified structures can be paired with movements described by microchemistry analysis to describe the life history of the fish (e.g., Hale and Swearer, 2008; Allen et al., 2009). Various elemental and isotopic ratios have been used in microchemistry analysis, but in freshwater systems, isotopes of strontium (87Sr:86Sr) are often most useful in distinguishing among stream systems with diverse geologies (e.g., Brown and Severin, 2009; Hegg et al., 2013; Brennan et al., 2016). Strontium is isostructural to calcium (Ca) and replaces Ca in calcified structures more predictably than other elements (Pracheil et al., 2014). The most reliable isotopic ratio for analysis is 87Sr:86Sr because Sr is stable in calcified structures and at specific locations over time (Kennedy et al., 2000; Pracheil et al., 2014). Although 87Sr:86Sr analysis is most common in freshwater, trace element (TE) analysis is another method that can be paired with 87Sr:86Sr analysis to improve the ability to assign and discriminate among locations (Hegg et al., 2019).
Measurement of the TE value in a calcified structure is typically reported as the ratio of the element of interest to total Ca (Campana, 1999). Analysis of TE is most often used to detect anadromy, but some examples of successful freshwater TE studies exist (e.g., Howland et al., 2001; Clarke et al., 2015). Many elements have been used to describe movement, but results can be unpredictable as certain elements (e.g., Sr) can demonstrate phenomena such as life-long accumulation (Mugiya and Satoh, 1997; Howland et al., 2001). Some studies indicate that physiological processes may be dominant over environmental influences for particular elements (Sturrock et al., 2014). However, experimental studies of calcified structures in fishes indicate that Ca, Sr, and barium (Ba) are most useful in TE analysis (Hale and Swearer, 2008; Jarić et al., 2011; Veinott et al., 2014; Luque et al., 2017; Vignon et al., 2023). In this study, analyses of TE focused on Sr, Ba, and magnesium (Mg) because the incorporation of these elements into calcified structures of fishes has been well studied, they are commonly found throughout aquatic environments, and have been shown to reflect environmental concentrations (Doubleday et al., 2013; Pracheil et al., 2014). Additionally, Sr and Ba are considered metabolically inert and easily measured in calcified structures.
In both 87Sr:86Sr and TE analysis, otoliths are the preferred and most studied calcified structure in fishes (Veinott and Evans, 1999; Hobbs et al., 2019). However, otoliths are relatively small in acipenserids and composed primarily of vaterite (Brennan and Cailliet, 1989; Baremore and Rosati, 2014; Pracheil et al., 2017). The structure of vaterite is poorly understood and often produces misleading results in microchemistry analyses (Pracheil et al., 2019a). Collection of otoliths is lethal, and many sturgeon populations have imperiled status. For these reasons, fin rays are often used for microchemistry studies of sturgeons (Sellheim et al., 2022). Calcification of fin rays in White Sturgeon begins 11–36 days after hatch, providing a nearly complete life history of 87Sr:86Sr and TE values (Sweeney et al., 2020). Fin rays are composed of hydroxyapatite, a calcium phosphate mineral, as opposed to the calcium carbonate matrices found in otoliths (Pracheil et al., 2014; Whitledge, 2017). When analyzed using laser ablation (LA) multicollector (MC) inductively coupled plasma mass spectrometry (ICPMS), fin rays demonstrate a CaPO polyatomic interference because of their hydroxyapatite composition (Woodhead et al., 2005; Vroon et al., 2008; Willmes et al., 2016). By increasing the plasma sampling depth in LA-MC-ICPMS, oxide levels can be reduced to eliminate interference. This technique makes fin ray microchemistry a reliable alternative to otolith microchemistry. Given the imperiled status of White Sturgeon and a lack of information about their life histories, the objective of this study was to use fin ray microchemistry analysis to describe the movement of White Sturgeon throughout the Kootenai River basin.
2 Materials and methods
2.1 Study area
The headwaters of the Kootenai River originate in eastern British Columbia (Figure 1). The river flows south into Montana and turns northwest near Libby, Montana. From there, the Kootenai River continues northwest through Idaho and into British Columbia's Kootenay Lake. From the west arm of Kootenay Lake, the river continues west to its confluence with the Columbia River at Castlegar, British Columbia. Natural barriers at Bonnington Falls, British Columbia, and Kootenai Falls, Montana, isolated fishes over 10,000 years ago (Northcote, 1973); Kootenay Lake was also formed during this period (Hildebrand et al., 1999). The Kootenai River basin has been subjected to substantial anthropogenic development (e.g., mining, timber harvest, levees) including eight dams. Corra Linn, Duncan, and Libby dams define the distribution of White Sturgeon in the Kootenai River. Corra Linn Dam is located at the outlet of Kootenay Lake. Upstream, Duncan Dam prevents fish movement to the Duncan River basin at the north end of Kootenay Lake. Libby Dam is 50 km upriver from a natural barrier, Kootenai Falls, and is the most upstream dam in the Kootenai River. Construction of Libby Dam created Lake Koocanusa.
Geologic heterogeneity drives the ability of microchemistry to discriminate between aquatic habitats (Kennedy et al., 2000; Hegg et al., 2013). Four main geologic features influence drainage into the study area (Horton, 2017): the Purcell Mountains to the north, Salish Mountains to the east, and Cabinet Mountains to the south. Geologic history of these mountain ranges began during Mesoproterozoic, about 1,000–1,600 Ma. To the west are the Selkirk Mountains which began forming during the Cretaceous Period between 145 and 66 Ma. Kootenay Lake is highly influenced by inflow from the Kootenai River, but Kootenay Lake also has many other tributaries that drain diverse geology (Cui et al., 2017). The western shore of Kootenay Lake is composed mainly of Paleozoic (541–252 Ma) and Mesozoic (252–66 Ma) geologic assemblages. The eastern and southern portions of the lake are primarily Neoproterozoic (900–550 Ma) and Mesozoic geologic units. In general, the geology surrounding Kootenay Lake and its tributaries is composed of younger formations that would be expected to have higher 87Sr:86Sr values than the Mesoproterozoic formations dominating the Kootenai River drainage upstream of Kootenay Lake (Kennedy et al., 2000; Dunnigan et al., 2023).
2.2 Water sample collection and processing
Water samples were collected from the Kootenai River (n = 6) and Kootenay Lake (n = 6) in 2011 by Hobbs (2013) and indicated that discrimination between the two systems was possible using 87Sr:86Sr analysis. However, 87Sr:86Sr values from water samples collected from the Kootenai River (n = 6) in September 2015 were not consistent with Hobbs (2013). As such, additional water samples were collected from the Kootenai River (n = 9) and Kootenay Lake (n = 12) in 2023. In February 2023, samples were collected from the surface of the Kootenai River during a period of low flow to ensure samples were minimally affected by runoff from tributaries. In March 2023, samples were collected from 30 m depth in Kootenay Lake during a period of mixing to best approximate an average annual 87Sr:86Sr value. Prior to sample collection, all 50 mL vials, lids, and 10 mL syringes were acid washed, rinsed with ultrapure water, air dried, and stored in Whirl Pak bags (Nasco, Fort Atkinson, Wisconsin; Shiller, 2003). Samples were collected using 0.2 μm pore filters (GE, Pittsburgh, Pennsylvania). Water samples were transported to the Interdisciplinary Center for Plasma Mass Spectrometry at the University of California-Davis (UC Davis) where they were analyzed to obtain 87Sr:86Sr values using a Nu Plasma HR (Nu032) MCICP mass spectrometer with a desolvating nebulizer inlet system (Nu Instruments DNS-100; North Wales, United Kingdom). The MCICP instrument was calibrated throughout sampling as described by Sellheim et al. (2017).
2.3 Fin ray collection
White Sturgeon were sampled in the Kootenai River from March 13 to October 26, 2015, by Idaho Department of Fish and Game (IDFG) and British Columbia Ministry of Water, Land, and Resource Stewardship. Fish were sampled using gill nets and setlines as part of annual monitoring (Hardy et al., 2020). Weighted multifilament gill nets had stretch mesh sizes of 2.5, 5.1, and 7.6 cm. Setlines were 23 m long with six baited hooks of various sizes (12/0, 14/0, 16/0). Fork length (FL; cm) and weight (kg) were measured from all captured White Sturgeon. All White Sturgeon were scanned for PIT tags and data were entered into an interagency database as each fish was processed. If a PIT tag identified the fish as known-age and hatchery-origin, a fin ray sample was collected. A section from the most anterior pectoral fin ray was collected using side-cutting pliers. The section was cut just distal to the origin of the fin ray and then 25 mm distally from the first cut (Koch et al., 2008; Nguyen et al., 2016). Fin ray samples were stored in coin envelopes and allowed to air dry.
2.4 Fin ray 87Sr:86Sr analysis
Fin rays from White Sturgeon were mounted in epoxy and sectioned using a Buehler low-speed saw (Buehler, Lake Bluff, Illinois) following standard techniques (Koch and Quist, 2007). Sections of fin rays (0.5 mm) were sanded until annuli were clearly visible. Cross sections were mounted onto petrographic slides using Crystalbond 509-3 (Aremco, Valley Vintage, New York) and cleaned with ultrapure water. Slides contained between six and ten cross sections of White Sturgeon fin rays. All samples were taken to UC-Davis for strontium isotope analysis using LA-MC-ICPMS with a New Wave Research UP213 (Thermo Scientific, Fremont, California) laser ablation system coupled with a Nu Plasma HR (Nu Instruments Nu032; North Wales, United Kingdom) multiple-collection, high-resolution, double-focusing plasma mass spectrometer system. Line scans of fin rays from White Sturgeon proceeded from the lumen to posterior lobe edge at a beam width of 80 μm, scanning speed of 10 μm/s, laser pulse frequency of 20 Hz, and 90–100% laser power. In addition to measuring 87Sr:86Sr, isotopic masses were measured for 87Sr:86Sr, 88Sr, and 85Rb:88Sr. At the beginning and end of each slide containing fin ray samples, instrumental accuracy was evaluated by measuring a White Seabass Atractoscion nobilis otolith, Green Sturgeon Acipenser medirostris fin ray, and White Sturgeon fin ray with accepted standard Sr ratio values. Data collection and reduction followed methods detailed by Willmes et al. (2018) using the IsoFishR app in Program R (R Core Team, 2024). Reduced data were then smoothed using a thin-plate regression spline (k = 100) and generalized cross validation using the mgcv package in R (Wood, 2017). After processing fin rays for microchemistry, fin ray cross sections were viewed with a dissecting microscope using transmitted light. Using knowledge of known ages, a single reader measured the distance between annuli on the ablation transect with Image-Pro Plus software (Media Cybernetics, Inc., Bethesda, Maryland).
2.5 Fin ray trace element analysis
Preliminary results from 87Sr:86Sr analysis of water and fin ray samples suggested low discriminatory power between the Kootenai River and Kootenay Lake. Therefore, we conducted TE analysis in an attempt to differentiate between habitats. Water samples collected for 87Sr:86Sr analysis in 2023 (n = 21) were analyzed for TE concentrations at UC Davis Interdisciplinary Center for Plasma Mass Spectrometry using an Agilent 8900 QQQ-ICPMS (Agilent Technologies Inc., Palo Alto, California) via a peristaltic pump and a 0.4 mL/min MicroMist nebulizer. Prior to analysis, the QQQ-ICPMS instrument was tuned and calibrated following methods detailed in Sweeney et al. (2020).
White Sturgeon fin rays from the Kootenai River basin were analyzed for trace element concentrations at UC Davis. To efficiently use allotted time and resources, we chose a small subsample (n = 8) of fin rays for TE analysis. The subsample reflected the distribution of ages (2–23 years) of the larger sample. In addition, subsampled fish had recapture histories from both the Kootenai River and Kootenay Lake. Fin rays were ablated using a Photon Machines 193 nm ArF Excimer laser (Photon Machines Inc., Redmond, Washington) with a HelEx dual-volume LA cell (Teledyne CETAC Technologies, Omaha, Nebraska) coupled to an Agilent 7700x Quadrupole ICPMS (Agilent, Santa Clara, California). Line scans of fin rays from White Sturgeon proceeded from the lumen to the lateral edge at a beam width of 40 μm, scanning speed of 5 μm/s, and laser pulse frequency of 10 Hz. Prior to data collection, a transect of a larger spot size (150 μm) was ablated at a higher speed (100 μm/s) as a cleaning run. Data collection and reduction followed methods detailed by Sweeney et al. (2020). Isotopic masses were measured for 88Sr, 137Ba, 44Ca, magnesium (24Mg), manganese (55Mn), iron (57Fe), zinc (66Zn), and lead (208Pb). A single reader measured the distance between annuli on the ablation transect using known ages from the inter-agency database and Image-Pro Plus software.
2.6 Data summary and analysis
Mean 87Sr:86Sr, Sr:Ca, Ba:Ca, and Mg:Ca values were obtained from ICPMS analysis for each water sample collected in 2015 and 2023. Values of 87Sr:86Sr, Sr:Ca, Ba:Ca, and Mg:Ca were collected from fin rays at annuli with recapture data (see Figure 2 for example). We estimated the range of 87Sr:86Sr profile for each fish as the maximum value observed minus the minimum value observed to compare the range in 87Sr:86Sr data from fish to that observed in water chemistry. Microchemistry data between the nearest 10 μm on either side of annuli were obtained for each recapture and assigned to either Kootenay Lake or the Kootenai River based on capture location. Only recaptures from age-5 and older (n = 34) White Sturgeon were used for 87Sr:86Sr analysis in an effort to minimize error from elevated rubidium (Rb) measurements observed in young fish. Because of the small sample size (14 observations from eight White Sturgeon) and no apparent influence of Rb in the TE profiles, all observations for TEs were included. Water and fin ray data were tested for normality and homogeneity of variance using the Shapiro-Wilk and Levene tests (Allen et al., 2009; Avigliano et al., 2019). Data did not meet parametric assumptions and were compared using the Wilcoxon rank sum test (Blair and Higgins, 1980). A type-I error rate of α = 0.05 was used for all statistical tests.
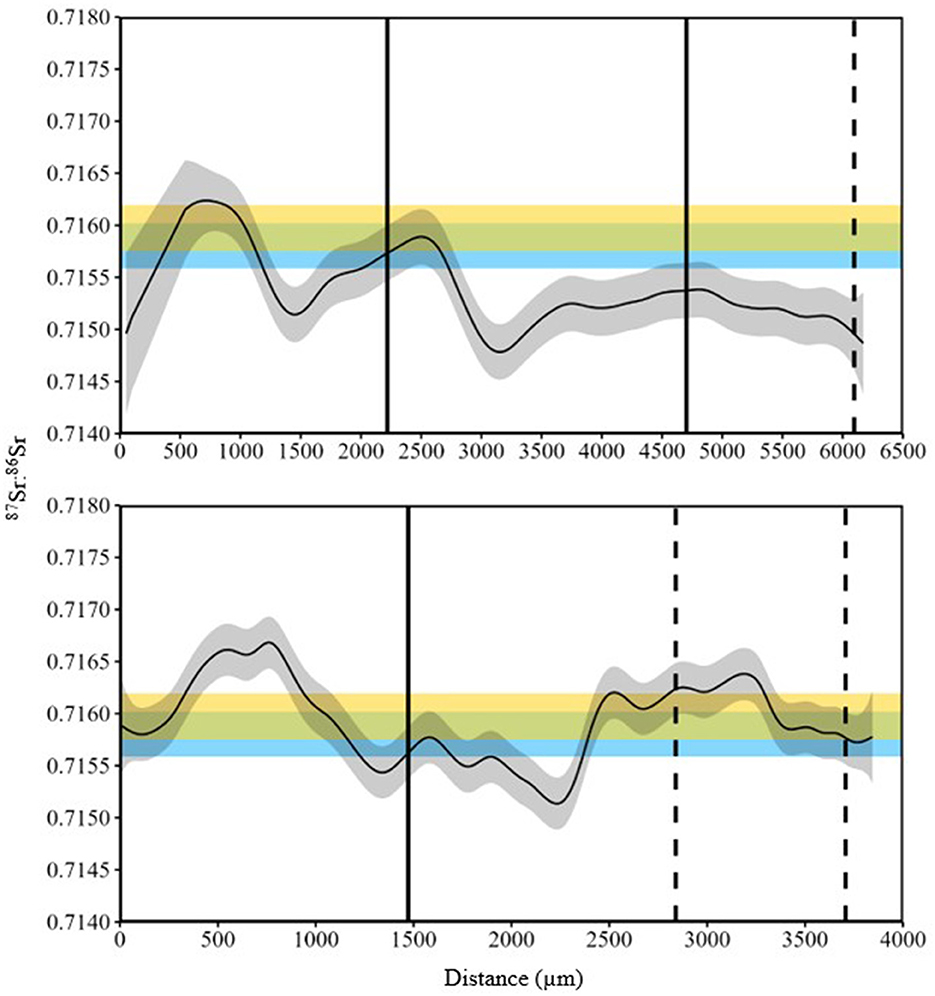
Figure 2. 87Sr:86Sr (solid line) data from line scans of two White Sturgeon (top panel age 16 yrs, 69.5 cm fork length; bottom panel age 20 yrs, 98.9 cm fork length) sampled in 2015 in the Kootenai River basin. Gray shading is the interquartile range of 87Sr:86Sr values. Distance was measured as micrometers from the lumen to the edge of the fin ray. Blue shading is the distribution of 87Sr:86Sr values for Kootenay Lake and yellow shading is the distribution of 87Sr:86Sr values for Kootenai River. Solid vertical lines represent capture in Kootenai River, dashed vertical lines represent capture in Kootenay Lake.
3 Results
Mean 87Sr:86Sr values from water samples were similar [Wilcoxon test statistic (W) = 99, P = 0.68; Table 1] between the Kootenai River and Kootenay Lake. Additionally, no spatial pattern existed in values observed throughout the basin that would indicate a gradient of values associated with environment (Figure 3). In total, 162 White Sturgeon fin rays were ablated using LA-MC-ICPMS to measure 87Sr:86Sr. White Sturgeon varied in length from 23 to 112 cm FL and in age from 2 to 23 years. The range of 87Sr:86Sr values detected by water samples was small compared to the range of values observed in White Sturgeon fin rays (Figure 4). The maximum difference between the highest 87Sr:86Sr and lowest 87Sr:86Sr values in water samples was 0.0024. In contrast, differences between the maximum and minimum values of 87Sr:86Sr on individual White Sturgeon fin rays varied from 0.00029 to 0.011. Age-5 and younger White Sturgeon typically had a higher range of 87Sr:86Sr values than older White Sturgeon (Figure 4). White Sturgeon with a highly vascularized lumen were often observed with elevated Rb and 87Sr:86Sr values near the lumen (Figures 5, 6). This pattern was particularly common in fin rays from age-5 and younger White Sturgeon.
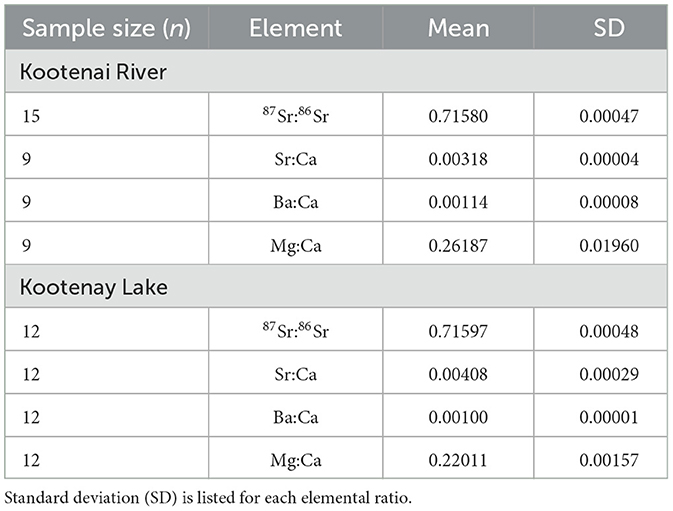
Table 1. Mean microchemistry values from water samples for all sites sampled in Kootenai River and Kootenay Lake in 2015 and 2023.
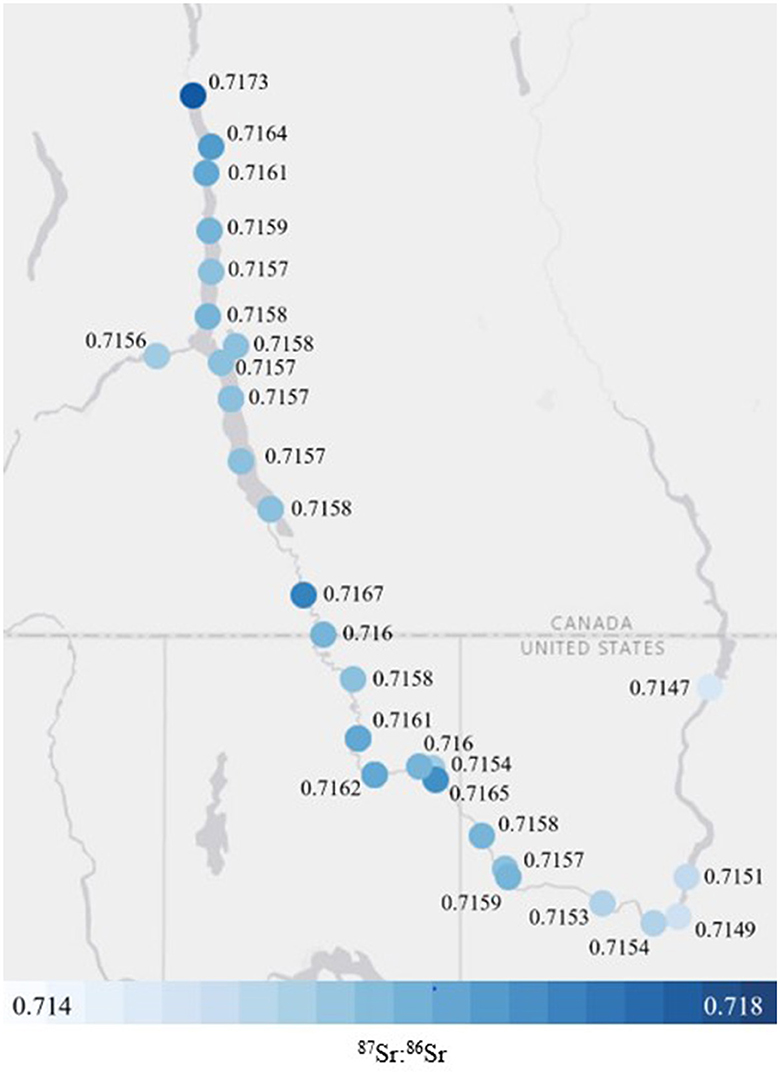
Figure 3. 87Sr:86Sr values for water samples collected in the Kootenai River basin in 2015 (n = 6) and 2023 (n = 21).
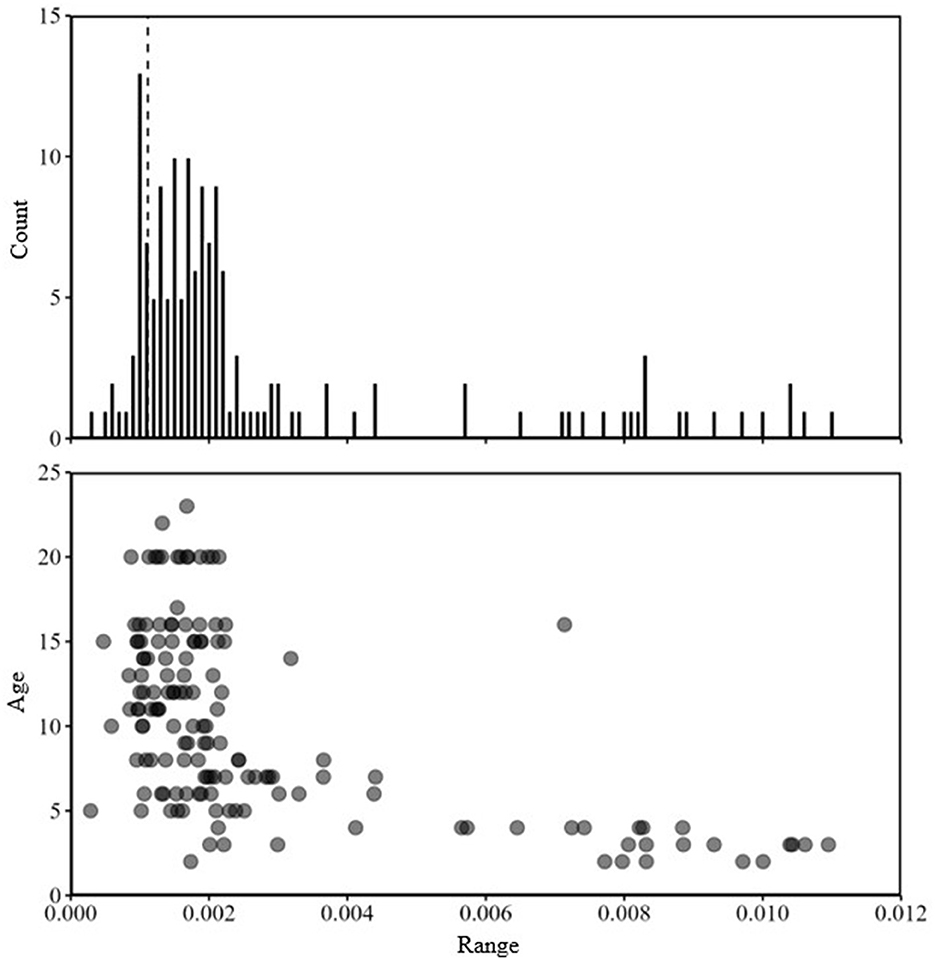
Figure 4. Frequency plot of the range (maximum minus minimum) of 87Sr:86Sr values from each line scan of White Sturgeon fin rays (n = 162) collected in 2015 from the Kootenai River (top panel). The vertical dashed line is the greatest estimated difference between Kootenai River and Kootenay Lake from water samples. Range of 87Sr:86Sr values from each line scan plotted by fish age (in yrs) at capture (bottom panel).
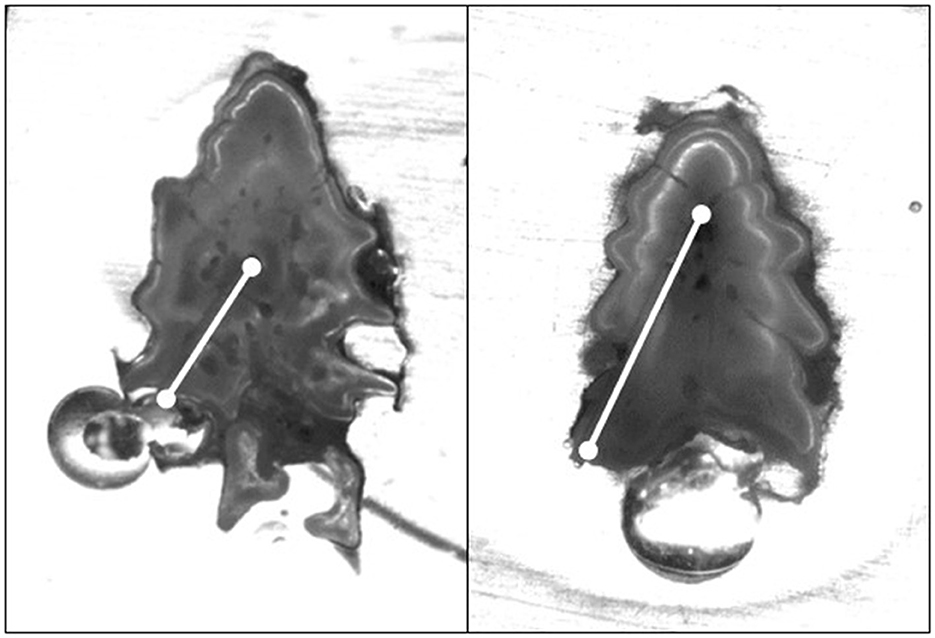
Figure 5. Images of White Sturgeon fin rays sampled in 2015 from the Kootenai River basin. Both are age-2 fish that displayed high rubidium values and abnormally high 87Sr:86Sr values (see Figure 6). Line scan path is highlighted by the white line.
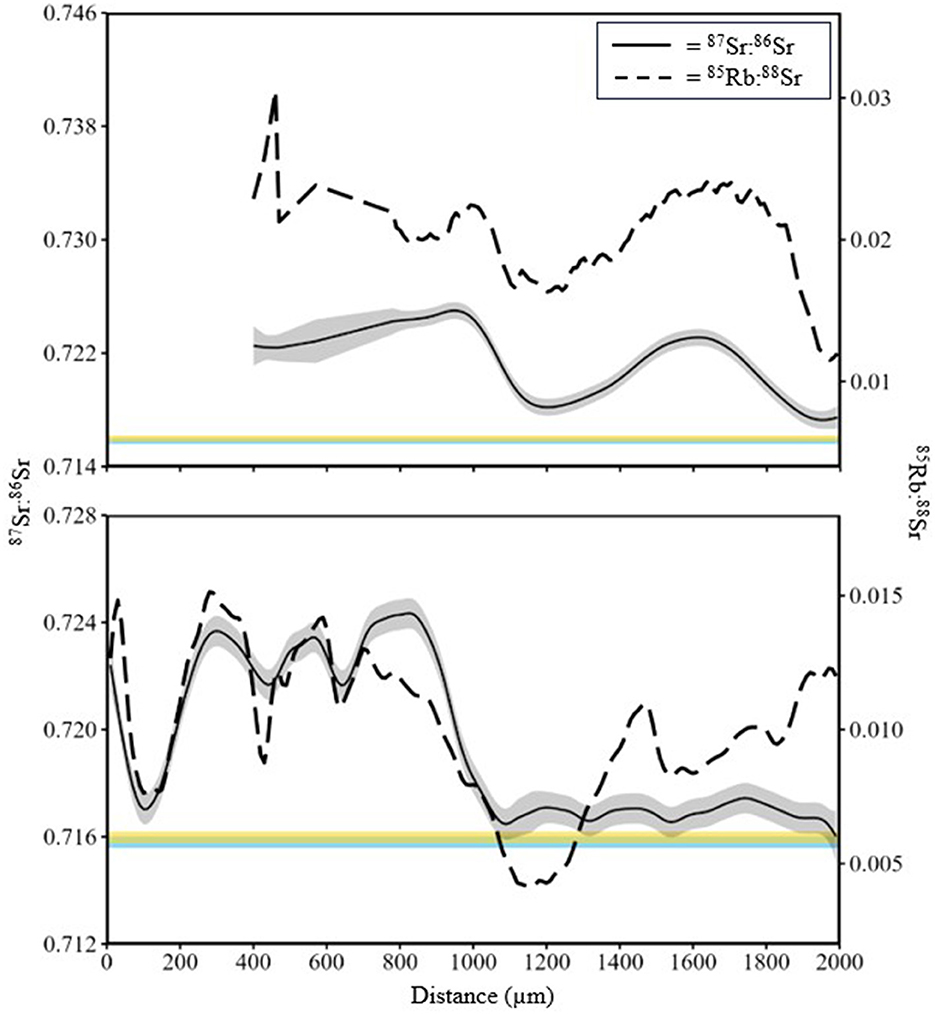
Figure 6. 87Sr:86Sr and 85Rb:88Sr data from line scans of two White Sturgeon (top panel age 2 yr, 31.5 cm fork length; bottom panel age 2 yr, 31.6 cm fork length) sampled in 2015 in the Kootenai River basin (see Figure 5). Gray shading is the interquartile range of 87Sr:86Sr values. Distance was measured as micrometers from the lumen to the edge of the fin ray. Blue shading is the distribution of 87Sr:86Sr values for Kootenay Lake and yellow shading is the distribution of 87Sr:86Sr values for Kootenai River.
Summary statistics for 87Sr:86Sr were calculated at locations on the fin ray with a known location (i.e., based on recapture; see Figure 2 for an example). 87Sr:86Sr values from fin rays when fish were in Kootenay Lake were significantly different from values measured when fish were in the Kootenai River (W = 1,310, P = 0.02). Despite significant test results, shifts in the distribution of these data could not reliably describe differences in location (Figure 7). Collectively, results from the analysis of 87Sr:86Sr from water samples and White Sturgeon fin rays suggested that 87Sr:86Sr was not useful for describing whether White Sturgeon inhabited the Kootenai River or Kootenay Lake. Although 87Sr:86Sr exhibited low variability across fin rays, high variability in total strontium (88Sr) from fin rays indicated that TE may provide insight not available with 87Sr:86Sr (Figure 8).
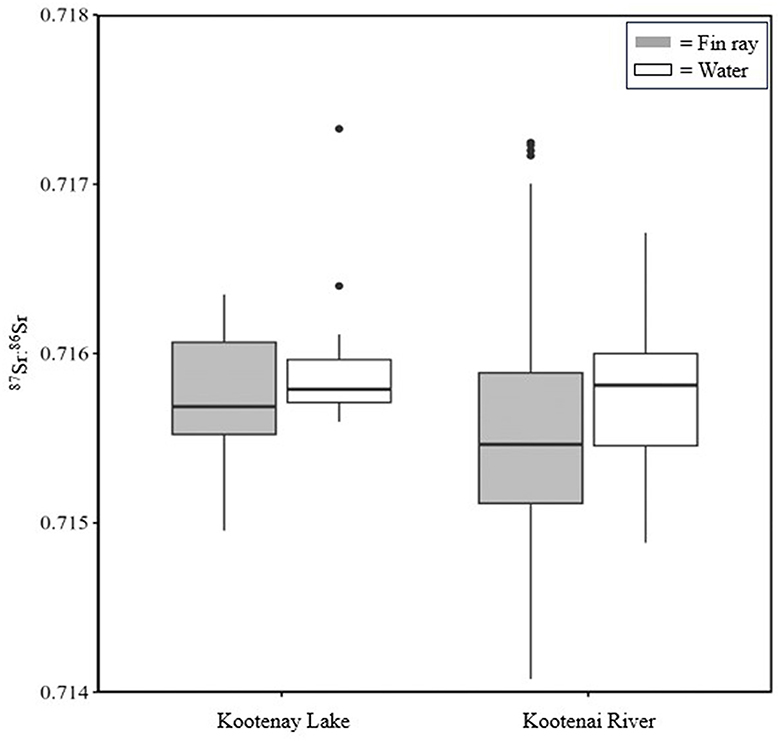
Figure 7. Comparison of 87Sr:86Sr data from the Kootenai River and Kootenay Lake. Fin ray data were collected from White Sturgeon captured in Kootenai River in 2015. Microchemistry data were assigned to either Kootenay Lake or the Kootenai River based on capture location and measured between the nearest 10 μm on either side of annuli for each recapture event. Water samples were collected in the Kootenai River basin in 2015 (n = 6) and 2023 (n = 21).
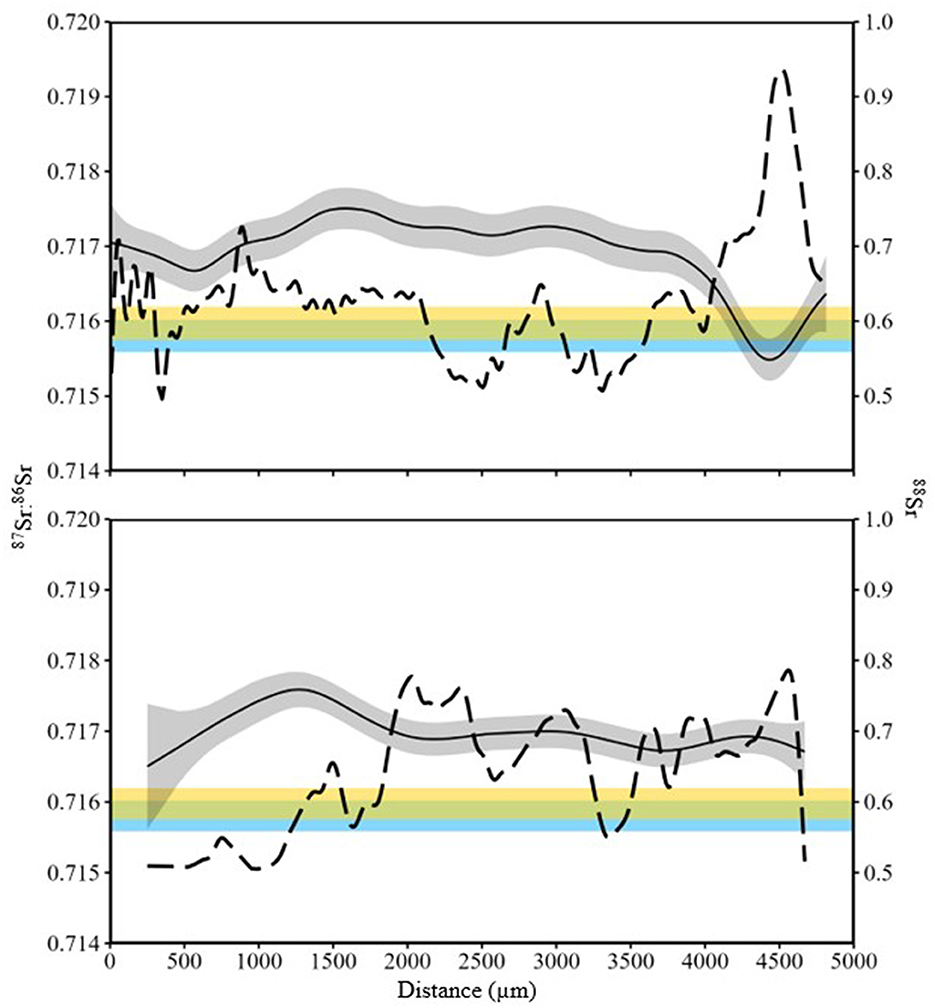
Figure 8. 87Sr:86Sr (solid line) and 88Sr (dashed line) from line scans of two White Sturgeon (top panel age 7 yr, 49.3 cm fork length; bottom panel age 8 yr, 73.3 cm fork length) sampled in 2015 in the Kootenai River basin. Gray shading is the interquartile range of 87Sr:86Sr values. Distance was measured as micrometers from the lumen to the edge of the fin ray. Blue shading is the distribution of 87Sr:86Sr values for Kootenay Lake and yellow shading is the distribution of 87Sr:86Sr values for Kootenai River.
Mean values of all TE values measured from water samples differed significantly between the Kootenai River and Kootenay Lake (Table 1). Mean Sr:Ca in water samples was lower in the Kootenai River than in Kootenay Lake, whereas Ba:Ca and Mg:Ca were highest in the Kootenai River. Patterns in TEs from eight White Sturgeon (29–106 cm FL; 2–23) fin rays were inconsistent with observations from water samples. For instance, Sr:Ca and Ba:Ca had a clear inverse relationship in water samples, but the same pattern was absent from all fin rays (Figure 9). All peaks in line scans that might be interpreted as movement were almost exclusively associated with annuli. Mg:Ca appeared to increase over time and, similar to Ba:Ca, failed to exhibit an inverse relationship with Sr:Ca (Figure 10). Sr:Ca and Ba:Ca from locations on fin rays where White Sturgeon were recaptured in Kootenay Lake were not significantly different than values measured when fish were in the Kootenai River (W = 13–26, P = 0.16–0.90). In contrast, Mg:Ca was significantly different (i.e., higher in Kootenay Lake) between known recapture locations (W = 45, P ≤ 0.05).
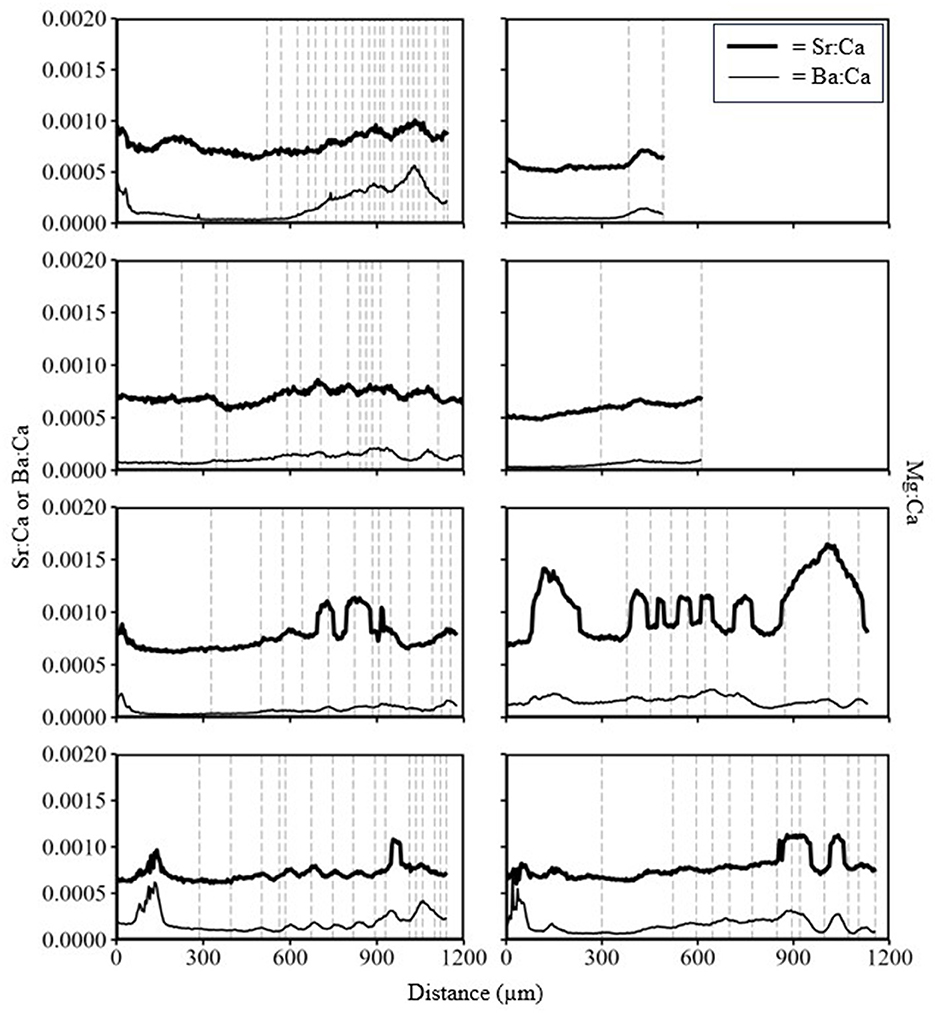
Figure 9. Trace element line scan of eight White Sturgeon fin rays collected from the Kootenai River basin in 2015. Vertical dashed lines are annuli. Distance was measured as micrometers of the line scan from the lumen to the edge of the fin ray.
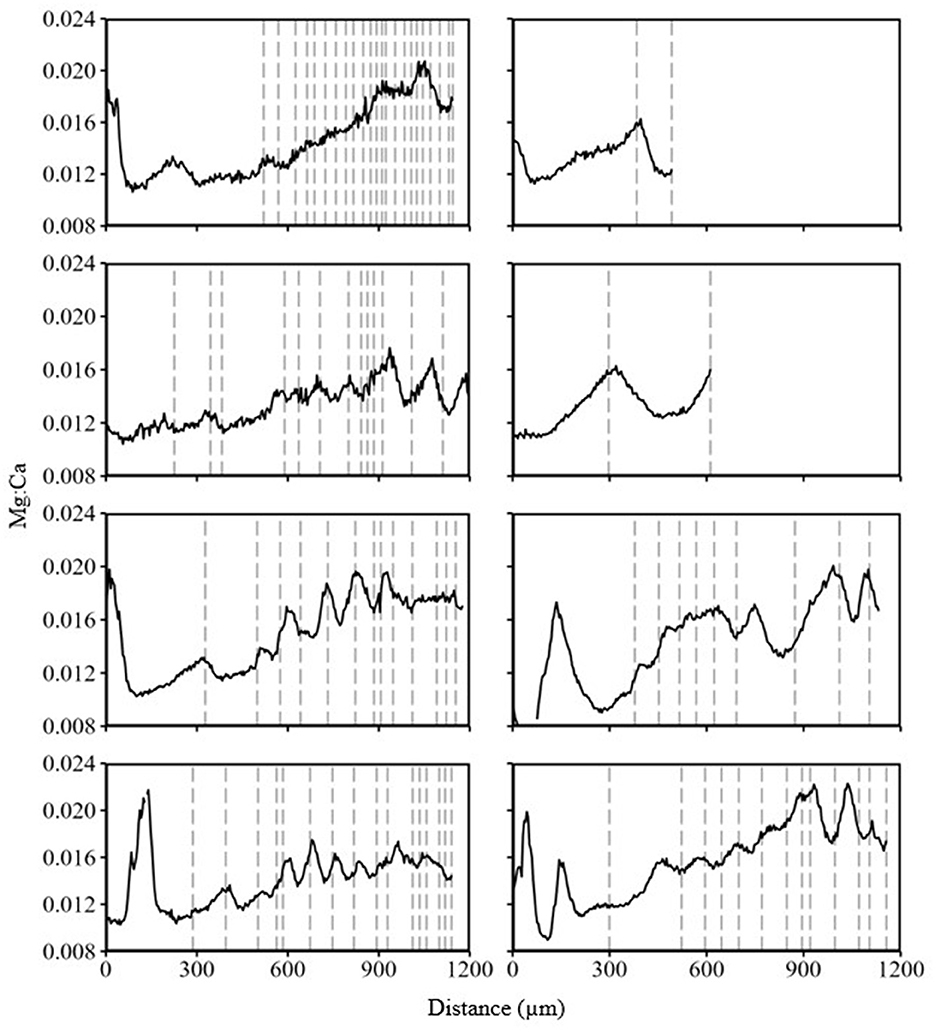
Figure 10. Trace element line scan of eight White Sturgeon fin rays collected from the Kootenai River basin in 2015. Black lines represent Mg:Ca values. Vertical dashed lines are annuli. Distance was measured as micrometers of the line scan from the lumen to the edge of the fin ray.
4 Discussions
Microchemistry has become an important tool in fish science. Describing movement of fishes with 87Sr:86Sr is accomplished by comparing the isotopic ratio found in calcified structures to that of water in the study area. Analyses of 87Sr:86Sr have been used to describe locations of fishes at many spatial scales, from small streams (Kennedy et al., 2000) to the ocean coastline (Fink-Jensen et al., 2022). In this study, 87Sr:86Sr analyses were unable to detect movement of White Sturgeon given the homogeneity of water chemistry in the Kootenai River basin. Using 87Sr:86Sr is best conducted in systems with high geologic heterogeneity since the age of geologic formations in the watershed is reflected in the water chemistry (Kennedy et al., 2000). Although the geology of the Kootenai River basin is heterogenous, the geography of the basin leads to mixing of water in the mainstem waterbodies. Both the Kootenai River and Kootenay Lake have major tributaries that drain from the Selkirk and Purcell mountain ranges. Upstream of Libby Dam, Koocanusa Reservoir has major tributaries that drain the Purcell and Salish mountain ranges. Mixed water from Koocanusa Reservoir forms the Kootenai River downstream of Libby Dam further homogenizing 87Sr:86Sr values. Multivariate analysis that pair TE analysis with 87Sr:86Sr analysis have been used in other systems to discriminate between water bodies (Hale and Swearer, 2008; Allen et al., 2018; Pracheil et al., 2019b). Because 87Sr:86Sr values alone were unable to discriminate between the Kootenai River and Kootenay Lake, TE analysis was completed for a subsample of White Sturgeon in this research.
Analysis of TE describes the location of fishes by comparing the amount a specific element in a water body to that found in calcified structures. Different elements may be measured in microchemistry analysis, each with varying levels of influence from either diet or environment (Doubleday et al., 2013; Tzadik et al., 2017). Concentrations of TE may vary in response to geology, pollution, and other environmental inputs (Campana, 1999). Elements subject to physiological influence (e.g., Zn, Mg) are best paired with elements that have well-understood incorporation processes (e.g., Ba, Sr) for discrimination between water bodies (Clarke et al., 2007; Loewen et al., 2016; Thomas and Swearer, 2019). Systems with distinct chemical transitions (e.g., freshwater to salt water; Limburg, 1995; Allen et al., 2009; Sellheim et al., 2022) are well-studied, but fewer analyses of TE have been conducted in freshwater systems (Clarke et al., 2015; Avigliano et al., 2019). Studies using sturgeon fin rays found that TEs were able to detect natal origin of river sturgeons between the Mississippi and Missouri river basins (Phelps et al., 2012) and describe movement of Shovelnose Sturgeon Scaphirhynchus platorynchus in the Mississippi and Wisconsin rivers (Pracheil et al., 2019b). In the Kootenai River basin, TE analysis was able to distinguish between water samples from the Kootenai River and Kootenay Lake. Unfortunately, TEs in White Sturgeon fin rays provided no discriminatory power, thereby suggesting that biological processes may have influenced TEs in fin rays or that the small annuli size, coupled with the relatively large ablation spot size was unable to resolve movements.
The processes regulating elemental incorporation into otoliths are generally known, but processes of incorporation in fin rays are less understood (Reis-Santos et al., 2023). Research into the processes of elemental incorporation in otoliths is extensive and has increased confidence in the interpretation of microchemistry results (Farrell and Campana, 1996; Elsdon and Gillanders, 2005; Brown and Severin, 2009; Clarke et al., 2015). Some processes involved in the growth of fin rays are documented (e.g., annuli formation, vascularization), but the cellular and molecular processes are not (Tzadik et al., 2017). Studies into fin ray physiology have largely been experimental studies on environmental effects or comparison to otoliths (e.g., Clarke et al., 2007; Sellheim et al., 2017; Sweeney et al., 2020). Rude et al. (2014) found that dietary influences from marine-derived hatchery feed changed the microchemistry in fin rays of juvenile Muskellunge Esox masquinongy. The authors also reported that once Muskellunge consumed a wild diet, there was no influence on fin ray microchemistry because wild diets had a similar chemical composition to the environment. A review by Loewen et al. (2016) found that incorporation of elements into fin rays was highly influenced by growth, reproduction, and environmental conditions even for elements that are considered reliable geologic markers. Methodologically, the chemical structure of fin rays can also create challenges during ablation. In this study, both the hydroxyapatite structure and morphological characteristics of White Sturgeon fin rays influenced methods of ablation.
The morphology of sturgeon fin rays presents several challenges with regard to microchemistry analysis. Fin rays are flexible structures, and their morphology can be altered by growth or damage (Whitledge, 2017). A variety of techniques have been used to overcome morphological issues when conducting microchemistry analysis on fin rays. For example, spot analysis is sometimes used when morphology prevents effective line scans (Pracheil et al., 2014; Sellheim et al., 2017). White Sturgeon from the Kootenai River grow particularly slow, and most fin ray samples taken from the Kootenai River basin exhibited crowding of annuli. The hydroxyapatite structure of fin rays delivers a low signal when ablated, requiring a large spot size (40–80 μm) to ablate enough material to register a signal with both LA-MC-ICPMS and quadrupole ICPMS. The large spot size negates the benefits of using spot analysis (i.e., targeted ablation) because multiple annuli were often ablated simultaneously. Therefore, portions of our microchemistry results were a mixture of multiple years' worth of annuli data, further complicating inferences.
The original goal of this research was to provide insight on the population ecology of White Sturgeon that could be used to inform future conservation efforts in the Kootenai River system. Specifically, we sought to characterize movement of White Sturgeon with the aim of describing factors influencing growth across different habitats. Unfortunately, we were unable to answer precipitating research objectives but our process provided an important cautionary account and path for future research in fin ray microchemistry analysis and physiology. All study systems and fishes present unique conditions that influence methods used in microchemistry analysis. Water chemistry can change daily (e.g., tidal systems; Sellheim et al., 2022), seasonally (e.g., spring runoff; Hüssy et al., 2016), or over several years (e.g., pollution; Daros et al., 2021) making water chemistry one of the most important factors for recognizing the scope of inference in microchemistry study design. Considering data from fishes, understanding how elements are incorporated into the calcified structure of choice and how elemental incorporation may change over time is important. Lastly, additional attention given to determining the appropriate method for collection of microchemistry data may improve use of these techniques as microchemistry analysis can be conducted using different equipment and ablation methods (Pracheil et al., 2014). In many scientific fields, the majority of “null results” go unpublished or even unreported (e.g., Franco et al., 2014). Non-reporting of null results can lead to wasted effort in future research. Observations from this study support previous research findings from otolith microchemistry that elements commonly measured in otolith TE analysis can be more influenced by physiology than environment (Sturrock et al., 2014). Research of physiological processes in fin ray formation is necessary to improve interpretations of microchemistry analysis in fin rays.
Data availability statement
The datasets presented in this article are the property of the Idaho Department of Fish and Game but can be made available upon reasonable request to the corresponding author.
Ethics statement
The animal study was approved by all fish handling associated with the creation of this data set was performed in accordance with Idaho Department of Fish and Game and American Fisheries Society guidelines. The study was conducted in accordance with the local legislation and institutional requirements.
Author contributions
CG: Writing – original draft, Writing – review & editing, Data curation, Formal analysis, Visualization. MQ: Writing – original draft, Writing – review & editing, Conceptualization, Formal analysis, Funding acquisition, Investigation. RH: Writing – original draft, Writing – review & editing, Conceptualization, Funding acquisition, Investigation. MW: Writing – original draft, Writing – review & editing, Methodology, Software. LL: Writing – original draft, Writing – review & editing, Methodology. SW: Writing – original draft, Writing – review & editing. TS: Writing – original draft, Writing – review & editing.
Funding
The author(s) declare financial support was received for the research, authorship, and/or publication of this article. Funding for this project was provided by the Idaho Cooperative Fish and Wildlife Research Unit, the Idaho Department of Fish and Game, the Kootenai Tribe of Idaho, and Bonneville Power Administration.
Acknowledgments
We thank the Idaho Department of Fish and Game and the British Columbia Ministry of Water, Land, and Resource Stewardship for their collaborative effort in collecting fin rays for the study and providing us with recapture history data. We also thank J. Glessner for his invaluable help during our long hours in the lab. We especially thank P. Rust, W. Ewing, and S. Stevenson for their contributions to this project. We thank the Kootenai Tribe of Idaho, without whom this research would not be possible, for the production and tagging of White Sturgeon used in this study. Lastly, we thank M. Corsi, T. Johnson, K. Sellheim, J. Sweeney, K. Vierling, and two reviewers for helpful comments on a previous version of the manuscript. The U.S. Geological Survey, Idaho Cooperative Fish and Wildlife Research Unit, is jointly sponsored by the University of Idaho, U.S. Geological Survey, Idaho Department of Fish and Game, and Wildlife Management Institute. Any use of trade, firm, or product names is for descriptive purposes only and does not imply endorsement by the U.S. Government.
Conflict of interest
The authors declare that the research was conducted in the absence of any commercial or financial relationships that could be construed as a potential conflict of interest.
The author(s) declared that they were an editorial board member of Frontiers, at the time of submission. This had no impact on the peer review process and the final decision.
Publisher's note
All claims expressed in this article are solely those of the authors and do not necessarily represent those of their affiliated organizations, or those of the publisher, the editors and the reviewers. Any product that may be evaluated in this article, or claim that may be made by its manufacturer, is not guaranteed or endorsed by the publisher.
References
Allen, P. J., DeVries, R. J., Fox, D. A., Gabitov, R. I., and Anderson, W. G. (2018). Trace element and strontium isotopic analysis of Gulf Sturgeon fin rays to assess habitat use. Environ. Biol. Fishes. 101, 469–488. doi: 10.1007/s10641-018-0713-7
Allen, P. J., Hobbs, J. A., Cech, J.r. J. J, Van Eenennaam, J. P., and Doroshov, S.I. (2009). Using trace elements in pectoral fin rays to assess life history movements in sturgeon: estimating age at initial seawater entry in Klamath River Green Sturgeon. Transact. Am. Fish. Soc. 138, 240–250. doi: 10.1577/T08-061.1
Anders, P., Richards, D. L., and Powell, M. (2002). The first endangered White Sturgeon population: repercussions in an altered large river-floodplain ecosystem. Am. Fish. Soc. Symp. 2002, 67–82.
Avigliano, E., de Carvalho, B. M., Miller, N., Gironde, S. C., Tombari, A., Limburg, K., et al. (2019). Fin spine chemistry as a non-lethal alternative to otoliths for stock discrimination in an endangered catfish. Mar. Ecol. Progr. Ser. 614, 147–157. doi: 10.3354/meps12895
Baremore, I. E., and Rosati, J. D. (2014). A validated, minimally deleterious method for aging sturgeon. Fish. Bull. 112, 274–282. doi: 10.7755/FB.112.4.4
Beamesderfer, R. C. (1993). A standard weight (Ws) equation for White Sturgeon. Calif. Fish Game 79, 63–69.
Birstein, V., Bemis, W., and Waldman, J. (1997). The threatened status of acipenseriform species: a summary. Environ. Biol. Fishes. 48, 427–435. doi: 10.1023/A:1007382724251
Blair, R. C., and Higgins, J. J. (1980). A comparison of the power of Wilcoxon's rank-sum statistic to that of Student's t statistic under various nonnormal distributions. J. Educ. Stat. 5, 309–335. doi: 10.2307/1164905
Boreman, J. (1997). “Sensitivity of North American sturgeons and paddlefish to fishing mortality,” in Sturgeon biodiversity and conservation, eds Birstein, V.J., Waldman, J.R., and Bemis, W.E. (Springer, Dordrecht, Netherlands). 399–405. doi: 10.1007/0-306-46854-9_28
Brennan, J. S., and Cailliet, G. M. (1989). Comparative age-determination techniques for White Sturgeon in California. Transact. Am. Fish. Soc. 118, 296–310. doi: 10.1577/1548-8659(1989)118<0296:CATFWS>2.3.CO;2
Brennan, S. R., Torgersen, C. E., Hollenbeck, J. P., Fernandez, D. P., Jensen, C. K., and Schindler, D. E. (2016). Dendritic network models: improving isoscapes and quantifying influence of landscape and in-stream processes on strontium isotopes in rivers. Geophys. Res. Lett. 43, 5043–5051. doi: 10.1002/2016GL068904
Brown, R. J., and Severin, K. P. (2009). Otolith chemistry analyses indicate that water Sr:Ca is the primary factor influencing otolith Sr:Ca for freshwater and diadromous fish but not for marine fish. Can. J. Fish. Aquat. Sci. 66, 1790–1808. doi: 10.1139/F09-112
Campana, S. (1999). Chemistry and composition of fish otoliths: pathways, mechanisms and applications. Mar. Ecol. Progr. Ser. 188, 263–297. doi: 10.3354/meps188263
Cech, J. J., and Doroshov, S. I. (2004). “Environmental requirements, preferences, and tolerance limits of North American sturgeons,” in Sturgeons and Paddlefish of North America. Fish & Fisheries Series, Vol 27., eds. G. T. O. LeBreton, F. W. H. Beamish, and R. S. McKinley (Dordrecht: Springer), 73–86.
Clarke, A. D., Telmer, K. H., and Shrimpton, J. M. (2007). Habitat use and movement patterns for a fluvial species, the Arctic grayling, in a watershed impacted by a large reservoir: evidence from otolith microchemistry. J. Appl. Ecol. 44, 1156–1165. doi: 10.1111/j.1365-2664.2007.01350.x
Clarke, A. D., Telmer, K. H., and Shrimpton, J. M. (2015). Movement patterns of fish revealed by otolith microchemistry: a comparison of putative migratory and resident species. Environ. Biol. Fishes. 98, 1583–1597. doi: 10.1007/s10641-015-0384-6
Counihan, T. D., and Chapman, C. G. (2018). Relating river discharge and water temperature to the recruitment of age-0 White Sturgeon Acipenser transmontanus in the Columbia River using over-dispersed catch data. J. Appl. Ichthyol. 34, 279–289. doi: 10.1111/jai.13570
Crossman, J. A., Korman, J., McLellan, J. G., Howell, M. D., and Miller, A. L. (2023). Competition overwhelms environment and genetic effects on growth rates of endangered White Sturgeon from a conservation aquaculture program. Can. J. Fish. Aquat. Sci. 80, 958–977. doi: 10.1139/cjfas-2022-0113
Cui, Y., Miller, D., Schiarizza, P., and Diakow, L. J. (2017). British Columbia Digital Geology. British Columbia Ministry of Energy, Mines and Petroleum Resources, British Columbia Geological Survey Open File 2017-8, 9p. Data version 2019-12-19.
Daros, F. A., Condini, M. V., Altafin, J. P., Ferreira, F. O., and Hostim-Silva, M. (2021). Fish otolith microchemistry as a biomarker of the world's largest mining disaster. Sci. Total Environ. 807:151780. doi: 10.1016/j.scitotenv.2021.151780
Doubleday, Z., Izzo, C., Woodcock, S., and Gillanders, B. (2013). Relative contribution of water and diet to otolith chemistry in freshwater fish. Aquat. Biol. 18, 271–280. doi: 10.3354/ab00511
Dunnigan, J. L., Linley, T. J., Janak, J. M., Nims, M. K., Garavelli, L., and McMichael, G. A. (2023). Otolith 87Sr/86Sr identifies natal origin, movement and life history of Burbot Lota lota in the Kootenai River following 45 years of impoundment. Ecol. Freshw. Fish. 32, 618–632. doi: 10.1111/eff.12712
Dynesius, M., and Nilsson, C. (1994). Fragmentation and flow regulation of river systems in the northern third of the world. Science 266, 753–762. doi: 10.1126/science.266.5186.753
Elsdon, T., and Gillanders, B. (2005). Strontium incorporation into calcified structures: separating the effects of ambient water concentration and exposure time. Mar. Ecol. Progr. Ser. 285, 233–243. doi: 10.3354/meps285233
Farrell, J., and Campana, S. E. (1996). Regulation of calcium and strontium deposition on the otoliths of juvenile tilapia, Oreochromis niloticus. Comp. Biochem. Physiol. Part A Physiol. 115, 103–109. doi: 10.1016/0300-9629(96)00015-1
Fink-Jensen, P., Hüssy, K., Thomsen, T. B., Serre, S. H., Søndergaard, J., and Jansen, T. (2022). Lifetime residency of Capelin Mallotus villosus in West Greenland revealed by temporal patterns in otolith microchemistry. Fish. Res. 247:106172. doi: 10.1016/j.fishres.2021.106172
Franco, A., Malhotra, N., and Simonovits, G. (2014). Publication bias in the social sciences: unlocking the file drawer. Science 345, 1502–1505. doi: 10.1126/science.1255484
Hale, R., and Swearer, S. (2008). Otolith microstructural and microchemical changes associated with settlement in the diadromous fish Galaxias maculatus. Mar. Ecol. Progr. Ser. 354, 229–234. doi: 10.3354/meps07251
Hardy, R. S., Ross, T. J., McDonnell, K. N., and McCormick, J. L. (2020). Kootenai River Resident Fish Mitigation Program: White Sturgeon, Burbot, Native Salmonid Monitoring and Evaluation. Annual Progress Report Number 1988-065-00. May 1, 2017-April 30, 2019. Annual Report, 9888-065-00. Boise: Idaho Department of Fish and Game.
Hardy, R. S., Ross, T. J., McDonnell, K. N., Quist, M. C., Holderman, C., and Stevens, B. S. (2022). Nutrient restoration of a large, impounded, ultra-oligotrophic western river to recover declining native fishes. North Am. J. Fish. Manag. 42, 977–993. doi: 10.1002/nafm.10792
Haxton, T. J., Sulak, K., and Hildebrand, L. (2016). Status of scientific knowledge of North American sturgeon. J. Appl. Ichthyol. 32, 5–10. doi: 10.1111/jai.13235
Hegg, J. C., Kennedy, B. P., and Chittaro, P. (2019). What did you say about my mother? The complexities of maternally derived chemical signatures in otoliths. Can. J. Fish. Aquat. Sci. 76, 81–94. doi: 10.1139/cjfas-2017-0341
Hegg, J. C., Kennedy, B. P., and Fremier, A. K. (2013). Predicting strontium isotope variation and fish location with bedrock geology: understanding the effects of geologic heterogeneity. Chem. Geol. 360–361, 89–98. doi: 10.1016/j.chemgeo.2013.10.010
Hildebrand, L., McLeod, C., and McKenzie, S. (1999). Status and management of White Sturgeon in the Columbia River in British Columbia, Canada: an overview. J. Appl. Ichthyol. 15, 164–172. doi: 10.1111/j.1439-0426.1999.tb00227.x
Hildebrand, L. R., Drauch Schreier, A., Lepla, K., McAdam, S. O., McLellan, J., Parsley, M. J., et al. (2016). Status of White Sturgeon Acipenser transmontanus throughout the species range, threats to survival, and prognosis for the future. J. Appl. Ichthyol. 32, 261–312. doi: 10.1111/jai.13243
Hobbs, J. A. (2013). Using Fin Ray Geochemistry to Assess Historic White Sturgeon Life History Movements in the Kootenai River. Final Report to U.S. Army Corps of Engineers. Washington, DC.
Hobbs, J. A., Lewis, L. S., Willmes, M., Denney, C., and Bush, E. (2019). Complex life histories discovered in a critically endangered fish. Sci. Rep. 9:16772. doi: 10.1038/s41598-019-52273-8
Horton, J. D. (2017). The State Geologic Map Compilation (SGMC) Geodatabase of the Conterminous United States (ver. 1.1, August 2017): U.S. Geological Survey Data Release.
Howland, K. L., Tonn, W. M., Babaluk, J. A., and Tallman, R. F. (2001). Identification of freshwater and anadromous Inconnu in the Mackenzie River system by analysis of otolith strontium. Transact. Am. Fish. Soc. 130, 725–741. doi: 10.1577/1548-8659(2001)130<0725:IOFAAI>2.0.CO;2
Hüssy, K., Gröger, J., Heidemann, F., Hinrichsen, H.-H., and Marohn, L. (2016). Slave to the rhythm: seasonal signals in otolith microchemistry reveal age of eastern Baltic cod Gadus morhua. ICES J. Mar. Sci. 73, 1019–1032. doi: 10.1093/icesjms/fsv247
Jarić, I., Lenhardt, M., Pallon, J., Elfman, M., Kalauzi, A., Suciu, R., et al. (2011). Insight into Danube Sturgeon life history: trace element assessment in pectoral fin rays. Environ. Biol. Fish. 90, 171–181. doi: 10.1007/s10641-010-9728-4
Justice, C., Pyper, B. J., Beamesderfer, R. C. P., Paragamian, V. L., Rust, P. J., Neufeld, M. D., et al. (2009). Evidence of density- and size-dependent mortality in hatchery-reared juvenile White Sturgeon Acipenser transmontanus in the Kootenai River. Can. J. Fish. Aquat. Sci. 66, 802–815. doi: 10.1139/F09-034
Kennedy, B. P., Blum, J. D., Folt, C. L., and Nislow, K. H. (2000). Using natural strontium isotopic signatures as fish markers: methodology and application. Can. J. Fish. Aquat. Sci. 57, 2280–2292. doi: 10.1139/f00-206
Koch, J. D., and Quist, M. C. (2007). A technique for preparing fin rays and spines for age and growth analysis. N. Am. J. Fish. Manag. 27, 782–784. doi: 10.1577/M06-224.1
Koch, J. D., Schreck, W. J., and Quist, M. C. (2008). Standardised removal and sectioning locations for Shovelnose Sturgeon fin rays. Fish. Manag. Ecol. 15, 139–145. doi: 10.1111/j.1365-2400.2008.00594.x
Limburg, K. E. (1995). Otolith strontium traces environmental history of subyearling American shad Alosa sapidissima. Mar. Ecol. Progr. Ser. 119, 25–35. doi: 10.3354/meps119025
Loewen, T. N., Carriere, B., Reist, J. D., Halden, N. M., and Anderson, W. G. (2016). Linking physiology and biomineralization processes to ecological inferences on the life history of fishes. Comp. Biochem. Physiol. Part A. 202, 123–140. doi: 10.1016/j.cbpa.2016.06.017
Luque, P. L., Zhang, S., Rooker, J. R., Bidegain, G., and Rodríguez-Marín, E. (2017). Dorsal fin spines as a non-invasive alternative calcified structure for microelemental studies in Atlantic bluefin tuna. J. Exp. Mar. Biol. Ecol. 486, 127–133. doi: 10.1016/j.jembe.2016.09.016
Mugiya, Y., and Satoh, C. (1997). Strontium accumulation in slow-growing otoliths in the Goldfish Carassius auratus. Fish. Sci. 63, 361–364. doi: 10.2331/fishsci.63.361
Nelson, T. C., Doukakis, P., Lindley, S. T., Schreier, A. D., Hightower, J. E., Hildebrand, L. R., et al. (2013). Research tools to investigate movements, migrations, and life history of sturgeons (Acipenseridae), with an emphasis on marine-oriented populations. PLoS ONE. 8:e71552. doi: 10.1371/journal.pone.0071552
Nguyen, P. L., Jackson, Z. J., and Peterson, D. L. (2016). Comparison of fin ray sampling methods on White Sturgeon Acipenser transmontanus growth and swimming performance. J. Fish Biol. 88, 655–667. doi: 10.1111/jfb.12866
Northcote, T. (1973). Some Impacts of Man on Kootenay Lake and Its Salmonoids. Ann Arbor, MI: Great Lakes Fishery Commission.
Paragamian, V. (2012). Kootenai River White Sturgeon: synthesis of two decades of research. Endang. Spec. Res. 17, 157–167. doi: 10.3354/esr00407
Paragamian, V. L., and Beamesderfer, R. C. P. (2003). Growth estimates from tagged White Sturgeon suggest that ages from fin rays underestimate true age in the Kootenai River, USA and Canada. Transact. Am. Fish. Soc. 132, 895–903. doi: 10.1577/T02-120
Paragamian, V. L., Beamesderfer, R. C. P., and Ireland, S. C. (2005). Status, population dynamics, and future prospects of the endangered Kootenai River White Sturgeon population with and without hatchery intervention. Transact. Am. Fisher. Soc. 134, 518–532. doi: 10.1577/T03-011.1
Paragamian, V. L., and Wakkinen, V. D. (2002). Temporal distribution of Kootenai River White Sturgeon spawning events and the effect of flow and temperature. J. Appl. Ichthyol. 18, 542–549. doi: 10.1046/j.1439-0426.2002.00391.x
Paragamian, V. L., Wakkinen, V. D., and Kruse, G. (2002). Spawning locations and movement of Kootenai River White Sturgeon. J. Appl. Ichthyol. 18, 608–616. doi: 10.1046/j.1439-0426.2002.00397.x
Parsley, M. J., Beckman, L. G., and McCabe, J.r. G.T. (1993). Spawning and rearing habitat use by White Sturgeons in the Columbia River downstream from McNary Dam. Transact. Am. Fish. Soc. 122, 217–227. doi: 10.1577/1548-8659(1993)122<0217:SARHUB>2.3.CO;2
Partridge, F. (1983). Kootenai River fisheries investigations. Idaho Department Fish and Game, Federal Aid to Fish and Wildlife Restoration, Project F73-R-5, Subproject IV, Study VI. Boise: Idaho Department of Fish and Game.
Phelps, Q. E., Whitledge, G. W., Tripp, S. J., Smith, K. T., Garvey, J. E., Herzog, D. P., et al. (2012). Identifying river of origin for age-0 Scaphirhynchus sturgeons in the Missouri and Mississippi rivers using fin ray microchemistry. Can. J. Fish. Aquat. Sci. 69, 930–941. doi: 10.1139/f2012-038
Pikitch, E. K., Doukakis, P., Lauck, L., Chakrabarty, P., and Erickson, D. L. (2005). Status, trends and management of sturgeon and paddlefish fisheries. Fish Fish. 6, 233–265. doi: 10.1111/j.1467-2979.2005.00190.x
Pracheil, B. M., Chakoumakos, B. C., Feygenson, M., Whitledge, G. W., Koenigs, R. P., and Bruch, R. M. (2017). Sturgeon and paddlefish (Acipenseridae) sagittal otoliths are composed of the calcium carbonate polymorphs vaterite and calcite. J. Fish Biol. 90, 549–558. doi: 10.1111/jfb.13085
Pracheil, B. M., George, R., and Chakoumakos, B. C. (2019a). Significance of otolith calcium carbonate crystal structure diversity to microchemistry studies. Rev. Fish Biol. Fish. 29, 569–588. doi: 10.1007/s11160-019-09561-3
Pracheil, B. M., Hogan, J. D., Lyons, J., and McIntyre, P. B. (2014). Using hard-part microchemistry to advance conservation and management of North American freshwater fishes. Fisheries. 39, 451–465. doi: 10.1080/03632415.2014.937858
Pracheil, B. M., Lyons, J., Hamann, E. J., Short, P. H., and McIntyre, P. B. (2019b). Lifelong population connectivity between large rivers and their tributaries: a case study of shovelnose sturgeon from the Mississippi and Wisconsin rivers. Ecol. Freshw. Fish. 28, 20–32. doi: 10.1111/eff,.12423
R Core Team (2024). R: A Language and Environment for Statistical Computing. Vienna: R Foundation for Statistical Computing. Available at: http://www.R-project.org/ (accessed May 1, 2024).
Reis-Santos, P., Gillanders, B. M., Sturrock, A. M., Izzo, C., Oxman, D. S., Lueders-Dumont, J. A., et al. (2023). Reading the biomineralized book of life: expanding otolith biogeochemical research and applications for fisheries and ecosystem-based management. Rev. Fish Biol. Fish. 33, 411–449. doi: 10.1007/s11160-022-09720-z
Rude, N. P., Smith, K. T., and Whitledge, G. W. (2014). Identification of stocked muskellunge and potential for distinguishing hatchery-origin and wild fish using pelvic fin ray microchemistry. Fish. Manag. Ecol. 21, 312–321. doi: 10.1111/fme.12081
Sellheim, K., Willmes, M., Hobbs, J. A., Glessner, J. J. G., Jackson, Z. J., and Merz, J. E. (2017). Validating fin ray microchemistry as a tool to reconstruct the migratory history of White Sturgeon. Transact. Am. Fish. Soc. 146, 844–857. doi: 10.1080/00028487.2017.1320305
Sellheim, K., Willmes, M., Lewis, L. S., Sweeney, J., Merz, J., and Hobbs, J. A. (2022). Diversity in habitat use by White Sturgeon revealed using fin ray geochemistry. Front. Mar. Sci. 9:859038. doi: 10.3389/fmars.2022.859038
Shiller, A. M. (2003). Syringe filtration methods for examining dissolved and colloidal trace element distributions in remote field locations. Environ. Sci. Technol. 37, 3953–3957. doi: 10.1021/es0341182
Snyder, E. B., and Minshall, G. W. (1996). Ecosystem Metabolism and Nutrient Dynamics in the Kootenai River in Relation to Impoundment and Flow Enhancement for Fisheries Management. Pocatello: Idaho State University, Stream Ecology Center, Annual Report,.
Sturrock, A., Trueman, C., Milton, J., Waring, C., Cooper, M., and Hunter, E. (2014). Physiological influences can outweigh environmental signals in otolith microchemistry research. Mar. Ecol. Progr. Ser. 500, 245–264. doi: 10.3354/meps10699
Sweeney, J. K., Willmes, M., Sellheim, K., Lewis, L. S., Hobbs, J. A., Fangue, N. A., et al. (2020). Ontogenetic patterns in the calcification and element incorporation in fin rays of age-0 White Sturgeon. Environ. Biol. Fish. 103, 1401–1418. doi: 10.1007/s10641-020-01031-1
Thomas, O. R. B., and Swearer, S. E. (2019). Otolith biochemistry—a review. Rev. Fish. Sci. Aquac. 27, 458–489. doi: 10.1080/23308249.2019.1627285
Tzadik, O. E., Curtis, J. S., Granneman, J. E., Kurth, B. N., Pusack, T. J., Wallace, A. A., et al. (2017). Chemical archives in fishes beyond otoliths: a review on the use of other body parts as chronological recorders of microchemical constituents for expanding interpretations of environmental, ecological, and life-history changes. Limnol. Oceanogr. 15, 238–263. doi: 10.1002/lom3.10153
van Poorten, B. T., and McAdam, S. O. (2010). Estimating differences in growth and metabolism in two spatially segregated groups of Columbia River White Sturgeon using a field-based bioenergetics model. Open Fish Sci. J. 3, 132–141. doi: 10.2174/1874401X01003010132
Veinott, G., Westley, P. A. H., Purchase, C. F., and Warner, L. (2014). Experimental evidence simultaneously confirms and contests assumptions implicit to otolith microchemistry research. Can. J. Fish. Aquat. Sci. 71, 356–365. doi: 10.1139/cjfas-2013-0224
Veinott, G. I., and Evans, R. D. (1999). An examination of elemental stability in the fin ray of the White Sturgeon with laser ablation sampling–inductively coupled plasma–mass spectrometry (LAS-ICP-MS). Transact. Am. Fish. Soc. 128, 352–361. doi: 10.1577/1548-8659(1999)128<0352:AEOESI>2.0.CO;2
Vignon, M., Tabouret, H., Aymes, J.-C., Pecheyran, C., Rives, J., Coste-Heinrich, P., et al. (2023). Effect of metabolic rate on time-lags change in otolith microchemistry - an experimental approach using Salmo trutta. J. Exp. Biol. 226:jeb245265. doi: 10.1242/jeb.245265
Vroon, P. Z., van der Wagt, B., Koornneef, J. M., and Davies, G. R. (2008). Problems in obtaining precise and accurate Sr isotope analysis from geological materials using laser ablation MC-ICPMS. Anal. Bioanal. Chem. 390, 465–476. doi: 10.1007/s00216-007-1742-9
Watkins, C. J., Ross, T. J., Quist, M. C., and Hardy, R. S. (2017). Response of fish population dynamics to mitigation activities in a large regulated river. Transact. Am. Fish. Soc. 146, 703–715. doi: 10.1080/00028487.2017.1308882
Whitledge, G. W. (2017). “Morphology, composition, and growth of structures used for age estimation,” in Age and Growth of Fishes: Principles and Techniques, eds. M. C. Quist, and D. A. Isermann (Bethesda, MD: American Fisheries Society), 9–32.
Willmes, M., Kinsley, L., Moncel, M.-H., Armstrong, R. A., Aubert, M., Eggins, S., et al. (2016). Improvement of laser ablation in situ micro-analysis to identify diagenetic alteration and measure strontium isotope ratios in fossil human teeth. J. Archaeol. Sci. 70,102–116. doi: 10.1016/j.jas.2016.04.017
Willmes, M., Ransom, K. M., Lewis, L. S., Denney, C. T., Glessner, J. J. G., and Hobbs, J. A. (2018). IsoFishR: an application for reproducible data reduction and analysis of strontium isotope ratios (87Sr/86Sr) obtained via laser-ablation MC-ICP-MS. PLOS ONE 13:e0204519. doi: 10.1371/journal.pone.0204519
Wolff, B. A., Johnson, B. M., and Landress, C. M. (2013). Classification of hatchery and wild fish using natural geochemical signatures in otoliths, fin rays, and scales of an endangered catostomid. Can. J. Fish. Aquat. Sci. 70, 1775–1784. doi: 10.1139/cjfas-2013-0116
Wood, S. N. (2017). Generalized Additive Models: An Introduction wIth R, 2nd Edn. Boca Raton, FL: CRC Press.
Keywords: sturgeon, microchemistry, movement dynamics, endangered species, strontium isotopes, Trace elements
Citation: Ghere CL, Quist MC, Hardy RS, Willmes M, Lewis LS, Wilson SM and Smith TW (2024) An evaluation of fin ray microchemistry to describe movement of White Sturgeon in the Kootenai River basin: insights and limitations. Front. Freshw. Sci. 2:1475115. doi: 10.3389/ffwsc.2024.1475115
Received: 02 August 2024; Accepted: 10 September 2024;
Published: 27 September 2024.
Edited by:
James E. Garvey, Southern Illinois University Carbondale, United StatesReviewed by:
Tanya Fendler, Southern Illinois University Carbondale, United StatesTodd Slack, USACE Engineer Research and Development Center, United States
Copyright © 2024 Ghere, Quist, Hardy, Willmes, Lewis, Wilson and Smith. This is an open-access article distributed under the terms of the Creative Commons Attribution License (CC BY). The use, distribution or reproduction in other forums is permitted, provided the original author(s) and the copyright owner(s) are credited and that the original publication in this journal is cited, in accordance with accepted academic practice. No use, distribution or reproduction is permitted which does not comply with these terms.
*Correspondence: Courtnie L. Ghere, Y2doZXJlQHVpZGFoby5lZHU=