- 1Laboratory of Bioinformatics and Genomics of Microorganisms, Institute of Biological Sciences, Federal University of Pará, Belém, Brazil
- 2Laboratory of Simulation and Computational Biology, High Performance Computing Center, Federal University of Pará, Belém, Brazil
- 3Genomics and Bioinformatics Laboratory, Department of Research and Scientific Production, Universidad Tecnológica de Santiago, Santiago De Los Caballeros, Dominican Republic
- 4Laboratory of Applied Genetics, Socio-Environmental and Water Resources Institute, Federal Rural University of the Amazon, Belém, Brazil
The freshwater and saltwater aquatic food sector has experienced the most significant growth in recent years and is increasingly recognized as a sustainable alternative for fostering prosperous societies self-sufficiently and ecologically. One primary economic and health risk factor in aquaculture production is health control, with potentially more severe impacts observed in tropical and developing countries. While metagenomics holds great promise for application in agro-industrial fields like aquaculture, its adoption remains limited. Consequently, this study aimed to assess the prospects for developing and applying metagenomics in identifying pathogens in freshwater aquaculture. The WIPO database was used to search for patents developed using metagenomics to monitoring pathogens in freshwater aquaculture. Metagenomics methods have been extensively employed in different fields, such as, medicine, veterinary, biotechnology, agriculture, particularly in studies focusing on microbial communities in different ecosystems. In aquaculture, the utilization of metagenomics has predominantly revolved around investigating antibiotic resistance genes, primarily in saltwater farms. Despite this, freshwater aquaculture, particularly in fish and crustacean farming, aligns closely with sustainable development goals, notably (SDGs) 2, 3, 6, and 13. Countries such as the United States of America, South Korea, and Canada stand at the forefront of utilizing metagenomics for disease monitoring in freshwater aquaculture, evidenced by their active patent developments. The metagenomic analysis, coupled with bioinformatics tools and databases, represents a rapid, secure, and non-invasive approach to environmental monitoring for preventive purposes.
Systematic review registration: https://osf.io/srpyz/, identifier 10.17605/OSF.IO/SRPYZ.
1 Introduction
Fresh and saltwater fish and seafood are essential for global food security, strategically addressing food demand and nutritional deficiencies, particularly in developing countries (Cojocaru et al., 2022). In this context, aquaculture has experienced significant growth over the years, the long-term trend in total global capture fisheries has been relatively stable since the late-1980's, with catches generally fluctuating between 86 and 93 million tons per year. However, in 2018, total global capture fisheries production reached the highest level ever recorded at 96.4 million tons—an increase of 5.4% from the average of the previous 3 years (Bjørndal et al., 2024; FAO, 2020), emerging as a key player in sustainable animal protein production. The aquaculture production chain can sustain livelihoods, economies, and cultures while minimizing environmental impact, thus encompassing all three sustainability dimensions: social, economic, and environmental (Gephart et al., 2020; Verdegem et al., 2023). Consequently, the seafood sector is increasingly recognized as vital for fostering prosperous societies self-sufficiently and ecologically and sustainable development, particularly in countries with coastal and freshwater environments (Béné et al., 2016; Bennett et al., 2021; HLPE, 2014). The importance of aquatic foods was underscored in 2021 during the launch of the UN nutrition report, which declared, “There can be no food system transformation without aquatic foods” (Troell et al., 2023; WHO, 2020).
Health management plays a fundamental role in ensuring the sustainability and success of aquaculture production, necessitating robust monitoring techniques to identify and prevent the spread of diseases in fish stocks (Assefa and Abunna, 2018). Annual financial losses due to diseases amount to 6 million dollars and can be more severe in tropical and developing countries, where mitigation of losses is often constrained. The complete eradication of pathogens poses both technical and financial challenges. The adaptability of production systems relies on three main characteristics: (1) the ability to prevent the system from being affected by a disease, (2) the development of internal resistance following an infection, and (3) the management of additional risks associated with strategies to reduce vulnerability (Leung and Bates, 2013; Bush et al., 2010). Overall, based on the index of technological practices adoption, the implementation of technological practices in aquaculture production centers significantly reduces the likelihood of disease outbreaks and enhances the economic profitability of producers (Amaral et al., 2019).
However, molecular approaches can be used to identify pathogens previously to prevent losses in aquaculture production. In this field, metagenomics is a promising molecular biology approach for analyzing complex microbial communities. This method provides valuable information about microbial diversity in an area and allows us to infer their biological roles (New and Brito, 2020; Nielsen et al., 2014). Although metagenomics has found applications in infectious disease surveillance in public health (Miller et al., 2013) and food and pharmaceutical industries (Coughlan et al., 2015), its use remains uncommon in specific agro-industrial disciplines such as aquaculture, despite numerous studies focused on characterizing microbial communities in cattle rumen (Xie et al., 2022) and agricultural soil (Macedo et al., 2021).
The global rise in antibiotic resistance, driven by intense human activity, is altering microbial communities and consequently compromising the environmental health of aquatic ecosystems. Metagenomic techniques offer a detailed analysis of microbiome dynamics, facilitating the monitoring of water resources and aiding in the identification of enzymatic pathways that may uncover new gene sequences of interest. A significant aspect of pollution in aquatic environments is the accumulation of contaminants in riverbed sediments. When these sediments are disturbed, they can resuspend, negatively impacting water quality. The microbiome diversity within these sediments plays a crucial role in regulating metabolic activity and can be influenced by various factors, including seasonal variations and human actions (Behera et al., 2021; Rout et al., 2022).
These characteristics were observed in the Ganges River in India, which has a high level of pollution and where bacterial strains highly resistant to antibiotics have been discovered. Rapid urbanization and the disposal of toxic products have intensified this process. The situation is further exacerbated by the likelihood of Horizontal Gene Transfer (HGT) events, which facilitate the spread of antibiotic resistance genes (ARGs) among various microbial species (Rout et al., 2023). On the other hand, there is also the emergence of beneficial bacteria with potential for bioremediation of heavy metals, pesticides, and other pollutants (Behera et al., 2020).
The application of metagenomics in aquaculture has predominantly centered on assessing antibiotic-resistance genes (Hemamalini et al., 2022), while investigations into microbiome evaluation for pathogen monitoring are primarily conducted in saltwater environments (Munang'andu, 2016). This highlights a reliance on conventional disease monitoring methods involving isolating microorganisms from infected aquatic organisms (Munang'andu, 2016). However, these traditional detection methods have limitations, including the variety of microorganism species and the challenges associated with cultivating some species under laboratory conditions (Martínez-Porchas and Vargas-Albores, 2017; Streit and Schmitz, 2004; Wang et al., 2012).
Given the potential of metagenomics to enhance food safety and public health in freshwater aquaculture, this review examines the current status and possible applications of metagenomic analysis for monitoring pathogens as an alternative approach to achieving sustainable production, ensuring food safety for humans and maintaining animal health in aquaculture.
2 Methods
2.1 Search strategy
This systematic review was performed according to the PRISMA 2020 guidelines (Page et al., 2021). The articles search focused on published between 2015 and 2024 in the Scopus and Pubmed databases (Table 1). No search filters were applied to the article databases, considering that the PubMed and Scopus filters are different. In addition, articles were searched on websites using the integrative review method to ensure compatibility with the theme discussed. The WIPO database was used to search for patents developed using metagenomics to monitoring pathogens in freshwater aquaculture.
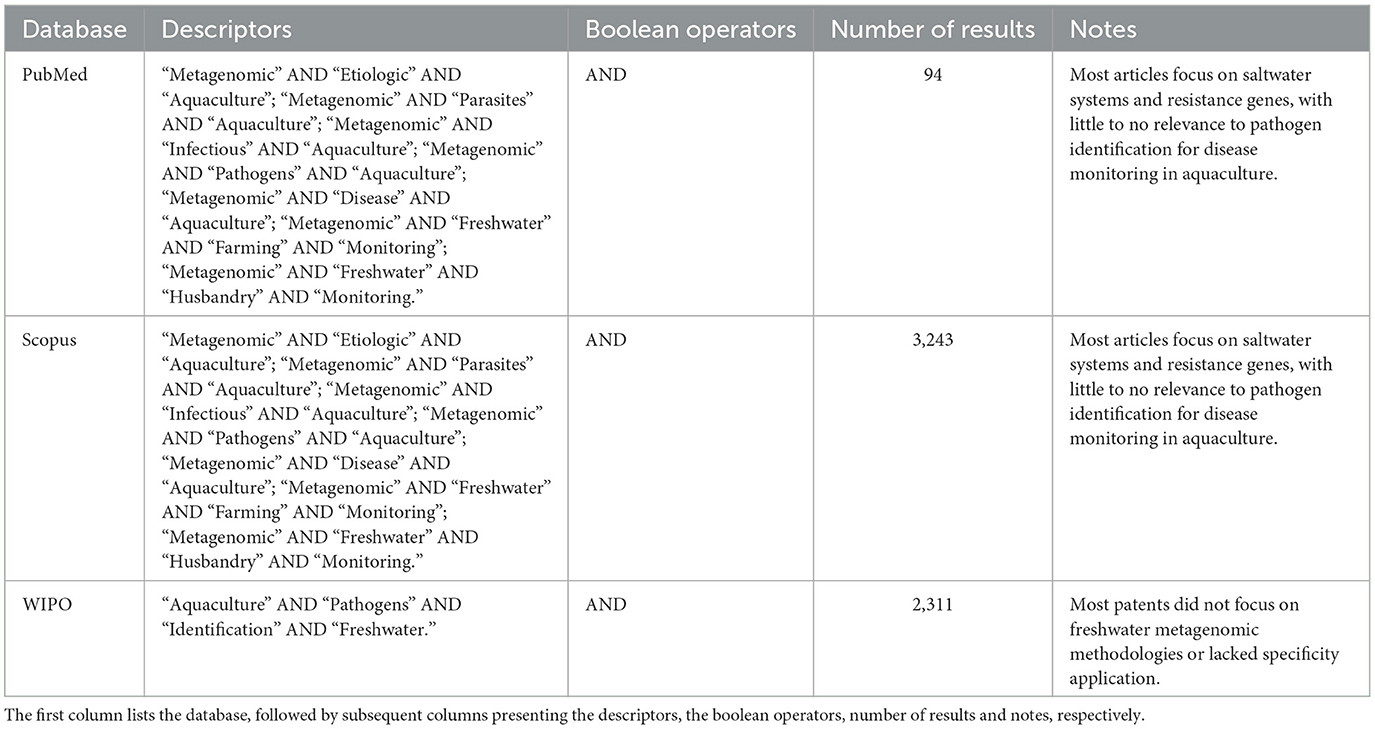
Table 1. Table of search terms employed in the literature review on metagenomics in aquaculture, detailing the databases accessed, boolean operators used, applied filters, number of results retrieved, and relevant observations.
2.2 Eligibility criteria
Initially, articles were selected using large criteria based on title, abstract and keywords. Thereafter, small criteria were used for selection: (1) published between 2015 and 2024; (2) articles and literature reviews; (3) english literature; (4) subject area. Articles were removed: (a) literature unrelated to the topic; (b) not significant monitoring pathogen focus; (c) non-English literature; (d) duplications. The patents are selected only according to subject area and relationship with the topic. The selection methodology flowchart can be seen (Figure 1).
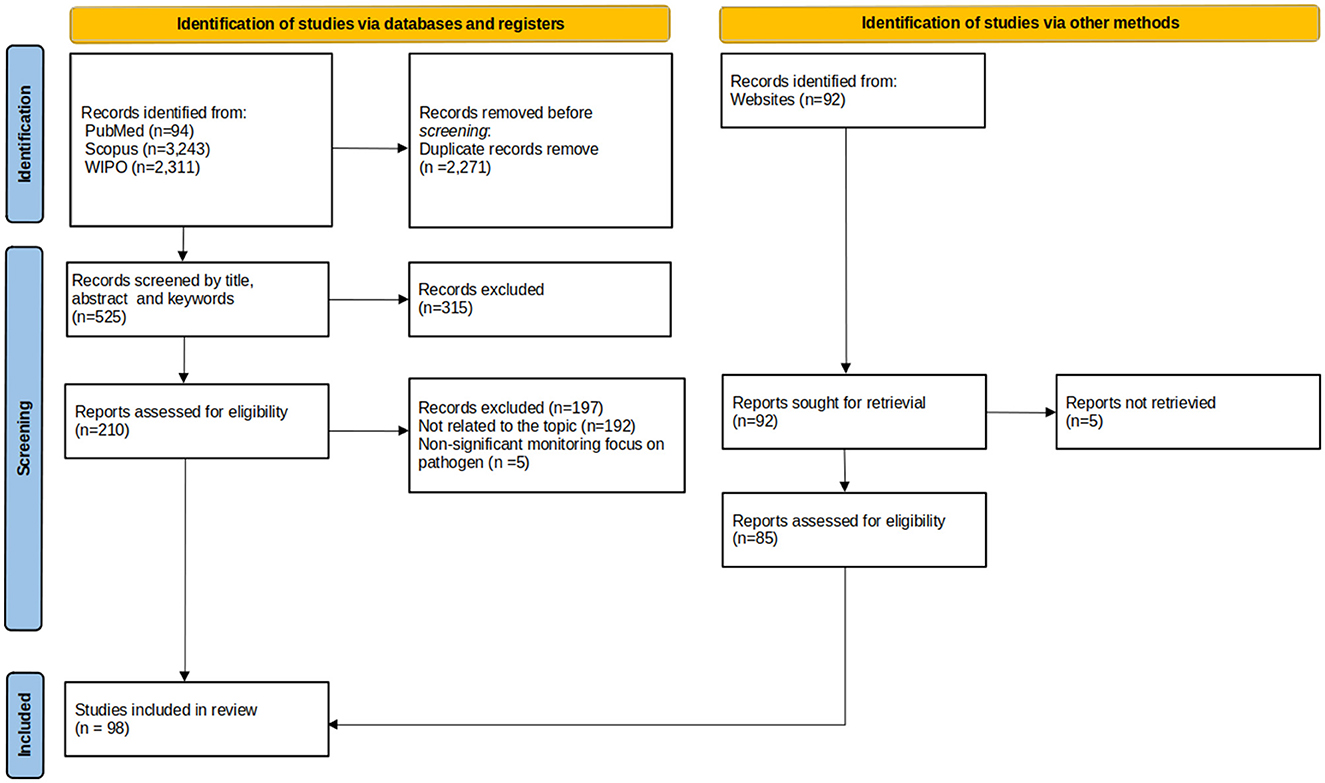
Figure 1. PRISMA workflow indicating the bibliography inclusion and exclusion criteria. The bibliographic material selection process was divided into two categories highlighted in yellow, with each category comprising three stages marked in blue: identification, screening, and inclusion.
2.3 Data analysis
The selected data were organized and stored in Microsoft Excel® spreadsheet software for data evaluation and accounting. The articles were analyzed with a focus on sustainable development considerations. The Vosviewer software (Van Eck and Waltman, 2010) was used for bibliometric analysis for the selection of key terms in research and their application related to the 17 Sustainable Development Goals (SDGs). The geographic indicators of technological production were evaluated based on the origin of the patents.
3 Results
3.1 Sustainability indicators
Since most global aquaculture production is concentrated in freshwater environments (FAO Fisheries and Aquaculture, 2016), these environments require increased emphasis on implementing Sustainable Development Goals. This is due to the potentially greater impact of aquaculture activities in freshwater areas compared to saltwater systems, which are less numerous. Despite estimates existing for aquaculture production, a substantial data gap remains regarding the actual volume of aquaculture production (Ottinger et al., 2018). Therefore, it is speculated that current production levels in freshwater regions are considerably higher.
The 17 SDGs, consisting of 169 targets and 232 indicators, were established in 2015 to promote global sustainable development by 2030. The goals are urgent for all countries in the political and social spheres. Targets consist of sets of actions of an urgent and delimited nature aimed at achieving a specific goal within a specified timeframe. Indicators, both quantitative and qualitative, are necessary for tracking progress and monitoring the SDGs (NU, 2015). The SDGs are universally applicable and take into account diverse realities, capabilities, and levels of development across countries. Aquaculture is aligned with the goals of Agenda 30 for life in water. According to FAO (2017), sustainable aquaculture can contribute to the success of other SDGs, including SDG 1 (ending poverty), SDG 2 (ending hunger, achieving food security, and promoting sustainable agriculture), SDG 3 (health), SDG 6 (water), SDG 13 (climate action), SDG 14 (marine ecosystems), and SDG 15 (terrestrial ecosystems, forests, and land). Farmers play an indispensable role in realizing a sustainable future. The adoption of the SDGs by rural producers emerges as a strategy for adapting to climate change and holds the potential to enhance productivity and production efficiency. Consequently, it contributes to all the aforementioned SDGs, with particular emphasis on food security, poverty eradication, and climate action (SDG 1, SDG 2, and SDG 13), which are target objectives of the UN. This is because a rural enterprise has the capacity to stimulate the local economy by generating jobs, income, and food (Khanal et al., 2021).
In the analysis of selected and published articles from 2017 to 2023 and patents, subsequently cited in Sections 3.3, 3.5, respectively, exhibit characteristics that align with their shared objectives in the Figure 2. Freshwater aquaculture, with a focus on the cultivation of fish and crustaceans, is in line with sustainable development goals and disease control initiatives aimed at ensuring public safety, particularly addressing the emergence of novel viruses and infectious diseases. Climate change is also a notable concern. These indicators are directly relevant to SDGs 2, 3, 6, and 13.
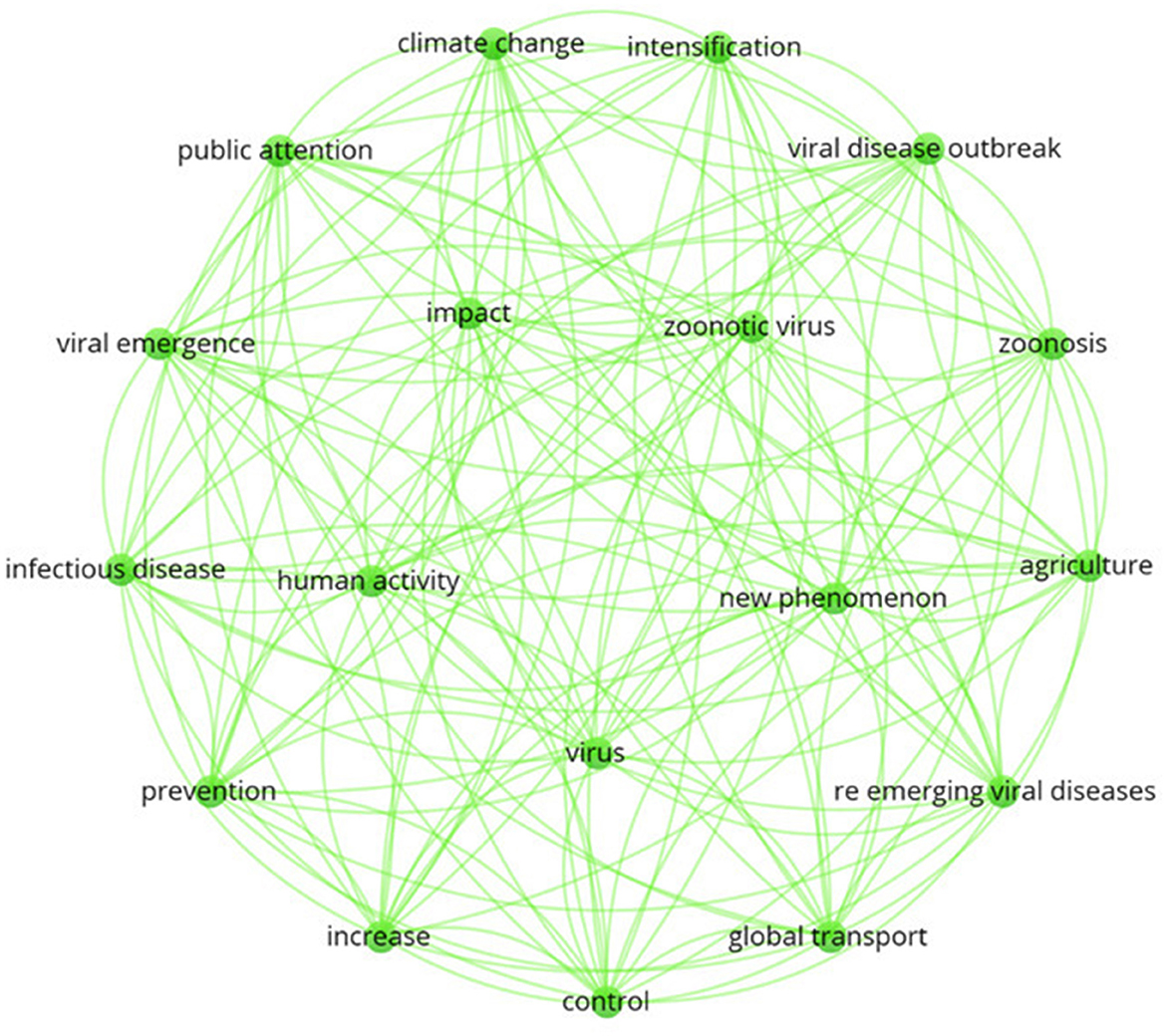
Figure 2. Network visualization performed through binary counting of shared characteristics among articles published between 2017 and 2023 on the PubMed and ScienceDirect platforms. The exclusive presence of a single color signifies the association with a distinct group, elucidating the interconnectedness of the words and highlighting their inherent relationship.
3.2 Aquaculture systems and molecular surveillance
Emerging diseases in terrestrial and marine ecosystems represent significant focal points for syntheses aimed at advancing our understanding of host-pathogen dynamics in a changing world. However, freshwater environments currently lack a comprehensive and generalized perspective on disease development (Okamura and Feist, 2011). The impacts of human-animal-environment interactions have historically been more pronounced in freshwater systems due to the preference for human settlements near freshwater bodies, resulting in animal aggregations near, excessive fishing, and environmental degradation. Consequently, new factors of human impact warrant investigation regarding their influence on pathogen dynamics in freshwater environments, including climate change (El-Sayed and Kamel, 2020) and novel practices in aquaculture production (Murray and Peeler, 2005). Characteristics such as high population density, the importation of fish products for feed, equipment movement, and pathogen exchange with wild populations contribute to the emergence and prevalence of new diseases in aquaculture (Bouwmeester et al., 2021). Moreover, the type of aquaculture system significantly influences pathogen establishment and impact on farms. In recirculating aquaculture systems (RAS), pathogen loads can escalate rapidly, with the challenge dose directly correlated to the onset of clinical conditions (Noble and Summerfelt, 1996; Bowden et al., 2002).
The specific pathogen free (SPF) strategy was experimentally introduced in aquaculture during the 1980's with Litopenaeus vannamei shrimp (Alday-Sanz, 2018). This approach mitigates disease risks in aquaculture, enabling greater farming intensification, production, and profitability. While the L. vannamei and Salmon industries in Asia have successfully adopted the SPF strategy, other farms, such as those cultivating Lates calcarifer and Oreochromis niloticus, are increasingly implementing this breeding approach (Subasinghe et al., 2023). The foundation for SPF breeding involves acquiring stocks whose original population has demonstrated resistance to specific pathogens, such as White Spot Syndrome Virus (WSSV), for at least 2 consecutive years. These stocks must be reared in highly biosecure facilities and fed pathogen-free diets. Consequently, a surveillance program utilizing molecular and histological tools is imperative (Alday-Sanz et al., 2020).
This scenario has paved the way for the development of proactive diagnostic tools for screening broodstock to ensure SPF stocks, which can also be applied to biosecurity management across various aquaculture farms (Munang'andu et al., 2017). Among the molecular tools, some methods used are nested PCR, quantitative real-time PCR, fluorescent quantitative PCR, pre-amplification PCR, insulated isothermal PCR assays, one-step PCR assay, duplex real-time PCR, optimized PCR assay, and fluorescence in situ hybridization (Islam et al., 2023).
Metagenomics remains relatively underexplored for this purpose in aquaculture, yet it holds significant potential for facilitating effective, low-cost, and comprehensive monitoring. Furthermore, it can contribute to the surveillance of new and emerging diseases, as the method does not require prior knowledge of the genomic sequence of the pathogen due to being a cultivation-independent method (Peters et al., 2018).
3.3 Metagenomics as a recent disease monitoring method in freshwater aquaculture
In recent years, identifying relevant parasitic, etiological, and infectious agents in freshwater aquaculture through metagenomic approaches has yielded new insights into disease and diagnosis. Evidence has surfaced regarding proliferative gill disease (PGD) and infections involving mixed myxozoan species alongside Henneguya ictaluri, the primary causative agent of PGD and the most significant parasite in the fish farming of Ictalurus punctatus and hybrid catfish (female channel catfish Ictalurus punctatus × male blue catfish I. furcatus) in North America (Stilwell et al., 2022). These mixed PGD infections were subsequently confirmed by detecting pre-sporogonic stages of other myxozoans (Stilwell et al., 2023). It was observed that populations of anaerobic microorganisms increased and at the same time, ascomycetes decreased in the intestinal flora of the Chinese mitten crab, Eriocheir sinensis, after infection by the fungus Metschnikowia bicuspidata. This finding contributes to a better understanding of host-pathogen interactions associated with “milky disease,” which can impact fish and crustaceans, often leading to medium to high mortality rates (Jiang et al., 2022).
Developing pathogen control strategies in aquaculture is typically a time-consuming process, commencing with the manifestation of the disease in clinical reports and extending until the identification of the etiological agent. For example, metagenomics has reduced diagnostic time preventing virus disease outbreaks and mitigating significant economic losses in aquaculture production. Moreover, it enables the discovery of new viruses from one or a few samples (Gangnonngiw et al., 2023), acknowledging that these environments are susceptible to the emergence of novel viruses infecting diverse organisms (Munang'andu et al., 2017). Examining the microbiota of an aquaculture system can help forestall the emergence of opportunistic pathogens, such as Aeromonas spp., through the early identification of other infectious agents like the WSSV. Furthermore, these investigations are integral to understanding their associations with metabolism, diseases, environmental information processing, organic systems, and cellular processes (Xue et al., 2022), also enables the acquisition of complete genomes of viruses, such as Decapod iridescent virus 1, which threatens the cultivation of Macrobrachium rosenbergii and other crustaceans (Qian et al., 2023).
Metagenomic analyses of freshwater can serve as valuable tools in aquaculture sanitary management. They facilitate not only the monitoring of potential pathogens but also the conducting of hygienic-sanitary assessments, including the inference of levels of nitrogenous compounds in water that may be toxic to aquaculture species. Fang et al. (2019) identified the prevalence of Proteobacteria in water and sediment samples from freshwater crab aquaculture environments, reaching levels equivalent to those found in water bodies influenced by wastewater treatment plants.
3.4 Bioinformatic tools for metagenomic analyze
Many microbial communities within freshwater environments remain largely unexplored, resulting in a scarcity of dedicated reference databases for metagenomic annotation (Tyagi et al., 2019). Conversely, marine environments have seen significant advancements in metagenomic database development, exemplified by resources like MarRef, MarDB, and MarCat (Klemetsen et al., 2018), due to having a more established inventory of microbial diversity, supported by extensive projects such as the International Census of Marine Microbes (ICoMM; http://icomm.mbl.edu). Nevertheless, in this section, we will outline bioinformatics tools (Table 2) applicable to metagenomic analyses within freshwater systems, aiming to delve deeper into these ecosystems, including their relevance to aquaculture. Given the current significant focus of aquaculture on studies of resistance genes, we also compiled databases currently utilized in investigations within this area. We emphasize that while this information is not the main focus of the review, we believe it to be relevant for aquaculture and can be used in conjunction with diversity analysis and metagenomic gene prediction to monitor pathogens for health control.
3.5 Perspectives on technological development
This review identified a limited number of patents related to metagenomic analysis in freshwater aquaculture. Notably, technological advancements in production are relatively recent within this industry and coincide with the launch period of the United Nations 2030 agenda. Achieving sustainable growth in freshwater aquaculture necessitates technological advancements to enhance productivity and expand the market for aquatic products (Mizik, 2023). This imperative arises from the need to address contemporary challenges such as climate change, contamination risks, and environmental impacts (Maulu et al., 2021). Consequently, strategies aimed at optimizing aquaculture systems and enhancing health management are paramount for sustaining and fostering the growth of the freshwater aquaculture industry. Within the realm of health management, continual monitoring, disease surveillance, and prompt diagnosis emerge as indispensable long-term solutions for safeguarding the health of aquaculture facilities (Ragasa et al., 2022). Metagenomics can serve as a valuable tool in this regard.
The application of metagenomic techniques has resulted in significant advancements in freshwater aquaculture and has fundamentally altered the approach to exploring the genetic diversity of environmental resources. Consequently, numerous endeavors have been undertaken to seek technological solutions and surmount obstacles in developing and applying metagenomics, aiming to broaden the exploration of diverse ecosystems (Yadav et al., 2020; Offiong et al., 2023). The advancement of the aquaculture system has been propelled by the development of patents for disease monitoring and food safety, including patents applied to metagenomic analysis (Table 3), thereby ensuring both production and consumer safety and safeguarding the exclusivity and commercial value of its products. Moreover, climate changes, characterized by temperature variations, can impact the dynamics of microorganisms, influencing factors such as pathogenicity, presence, and abundance (Majdi et al., 2020).
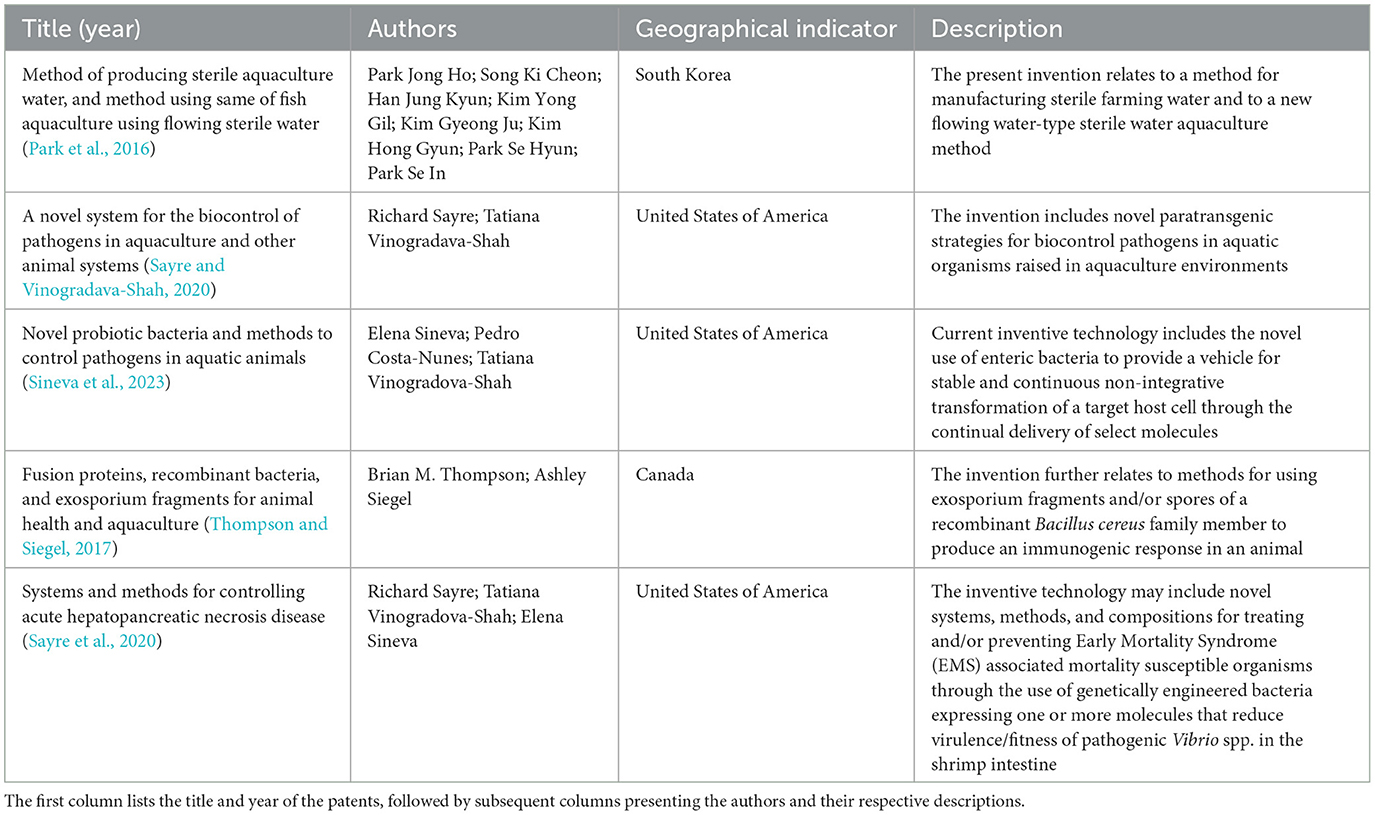
Table 3. Patents identified within the World Intellectual Property Organization between 2016 and 2023 are detailed below.
The United States of America, South Korea, and Canada countries typically lead in the development of patents, benefiting from a well-established scientific foundation alongside substantial investments, infrastructure, and a streamlined registration system, in contrast to countries like Brazil (Yamashita, 2021; Zhou et al., 2019). A common thread among these regions is the scarcity of water resources, stemming from factors such as pollution, climate change, or limited availability, necessitating investments in water treatment technologies, adoption of sustainable agricultural practices, implementation of water monitoring measures, and promotion of resource efficiency across all sectors (Huang et al., 2021; Dolan et al., 2021). Conversely, these regions are also notable for their status as significant fish producers and exporters in recent years (FAO, 2022).
4 Discussion
4.1 Metagenomics and its potential applications in freshwater aquaculture
This is the first review on the application of metagenomics in freshwater aquaculture systems. The physicochemical parameters of water play a pivotal role in shaping the composition of bacterial groups, with salinity emerging as a crucial determinant. Salinity exerts a profound influence on microbial communities by diminishing activity and biomass and altering the physiological properties of microbial cells, thus serving as a key indicator with significant ramifications for aquatic ecosystems (Rath et al., 2019). Specific bacterial subclasses, such as Beta-proteobacteria, exhibit a notable preference for freshwater ecosystems (Lew et al., 2022). Consequently, it is imperative to distinguish and juxtapose research findings between freshwater and saltwater systems, even within artificial aquaculture environments, and to make this differentiation in bibliographical research, as done in this review.
This review underscores the recent emergence of metagenomics application within freshwater aquaculture, primarily confined to investigations into antibiotic-resistance genes, while its utilization for pathogen monitoring remains relatively limited. In contrast, research in saltwater aquaculture has seen broader engagement (Haro-Moreno et al., 2020; Xie et al., 2022). Marine microbial communities have long been the focus of phylogenetic diversity studies and have constituted a significant area of early research in applied metagenomics (Handelsman, 2004). Moreover, they have been the subject of international ocean discovery initiatives (Bickle et al., 2011). This dynamic underscores the pressing need to incorporate climate change into ocean conservation strategies, given the vulnerability of marine socio-ecological systems (Santos et al., 2020), alongside the expansion of marine aquaculture driven by increased demand for seafood products in recent years (Galparsoro et al., 2020), which has propelled advancements in studies concerning marine microbial communities in aquaculture.
Despite the discrepancy in the advancement of studies concerning microbial communities in marine and freshwater environments, freshwater aquaculture necessitates a more comprehensive understanding of microbiological dynamics. This understanding is crucial for elucidating the intricate symbiotic and antagonistic relationships between cultivable aquatic organisms and microbial agents. Currently, inland or freshwater aquaculture stands as the primary contributor to global fish production, accounting for 64% of the total output in 2016. Although crustaceans are produced in smaller quantities within the inland aquaculture sector (Goddard and Delghandi, 2020), freshwater aquaculture still represents a significant portion of global aquaculture production. Consequently, there is a pressing need for further research into water management and treatment, encompassing the exploration of microbial diversity within the aquatic environment.
Microbial activities within aquaculture systems, particularly in open or semi-closed systems that use river water, remain inadequately understood in practice. The adoption of metagenomics within freshwater aquaculture holds promise for furnishing comprehensive microbiological data, encompassing insights into metabolic processes. This approach could significantly enhance our comprehension and manipulation of the microcosm within aquaculture systems, particularly regarding nutrient biogeochemical processes, antibiotic resistance, biofloc-forming microbial communities, probiotic utilization, effluent bioremediation, pathogen detection, and disease outbreak mechanisms (Martínez-Porchas and Vargas-Albores, 2017). The widespread integration of metagenomics into freshwater aquaculture practices could catalyze sustainable transformations in traditional aquaculture methodologies, facilitating enhanced health control and water quality monitoring within these systems.
Here we highlight the indicators “climate change,” “zoonotic viruses,” and “prevention.” Climate change is associated with losses in aquaculture production across various regions, impacting factors such as alterations in feeding behavior, physiology, metabolism, and growth performance due to thermal stress, as well as changes in primary and secondary productivity of systems reliant on this food source for animals (Maulu et al., 2021). For more technically advanced or intensive production systems—such as those involving covered or indoor structures, genetic improvement programs, and the application of molecular biology tools—the anticipated impacts are expected to be smaller and may even lead to beneficial outcomes in this scenario. Concerning diseases, it is evident that alterations in temperature regimes can render fish and shellfish vulnerable to pathogenic agents, potentially leading to the emergence of new diseases and, at elevated temperatures, an escalation in virulence, replication rates, and the life cycle of pathogens (Marcogliese, 2008; Sae-Lim et al., 2017).
Zoonotic viruses were one of the main focuses of the articles. It is noteworthy that, from a public health perspective, zoonotic diseases in aquaculture are predominantly investigated concerning bacterial and parasitic agents. The primary zoonotic agents in fish belong to bacterial genera, including Mycobacterium spp., Streptococcus spp., Erysipelothrix spp., Vibrio spp., Aeromonas spp., and Pseudomonas spp. (Ziarati et al., 2022). Consequently, a new trend in research on aquatic organisms is emerging, with a growing emphasis on the investigation of viruses and the discovery of new ones. This trend may be attributed to current aquaculture practices, which may favor the emergence of novel viruses (Munang'andu et al., 2017).
Prevention, as the primary objective of articles applying metagenomics in freshwater aquaculture, underscores an active surveillance approach aimed at gathering data that directly assesses the health status of a population concerning a specific disease. This form of surveillance is characterized by its provision of higher-quality information, as well as its swiftness and cost-effectiveness compared to passive surveillance methods typically employed in aquaculture. In passive surveillance, data collected for other purposes, such as biometrics for performance monitoring, are repurposed to assess the health status of animals (Assefa and Abunna, 2018).
Viral diseases have gained notable importance in public health, especially those derived from food, including fish, crustaceans, and fresh or frozen shellfish. Fish products have been associated with diseases such as acute gastroenteritis and hepatitis A, leading to major economic losses and serious human health problems. In the case of hepatitis A, symptoms can range from vomiting and abdominal cramps to liver failure and death, particularly in high-risk groups such as the elderly (Ziarati et al., 2022).
4.2 Advantages of implementing metagenomic analysis in sustainable freshwater aquaculture: optimization of production and disease prevention
Metagenomics offers significant control over aquaculture production by enabling precise environmental monitoring, thereby aligning with the concept of Precision Fish Farming. The principles underlying precision farming in aquaculture emphasize enhancing the accuracy, precision, and repeatability of operations, facilitating continuous monitoring, enabling more reliable decision-making, and reducing reliance on manual operations and subjective assessments (Føre et al., 2018). This management approach involves the acquisition, processing, and analysis of temporal, spatial, and individual data to optimize resource utilization. In highly intensive production systems, the adoption of technological advancements is paramount for optimizing various aspects of production, including survival, growth, and overall performance. This optimization facilitates higher stocking densities and enables modifications to daily operations to maintain a controlled environment (Mizik, 2023). Consequently, the integration of metagenomics technology within freshwater aquaculture enterprises allows for increased intensification of activities, leading to enhanced economic profitability (Amaral et al., 2019). The intensification of aquaculture systems represents the pathway toward fostering a more sustainable aquaculture industry, given that aquaculture does not necessitate expanding spatial footprints to augment production volume (Costa-Pierce and Chopin, 2021).
Disease control represents a primary challenge in aquaculture production, with the predominant method being the utilization of antibiotics or pharmacologically active compounds, even as a preventive measure in the absence of evident pathogens (Chen et al., 2020). However, this practice has led to the indiscriminate use of antibiotics in aquaculture, resulting in the emergence of antibiotic-resistant microorganisms and the bioaccumulation of antibiotics in aquaculture products intended for consumption. Consequently, this has had adverse effects on international trade, including the rejection of imported aquaculture products.
While the overall risks of antibiotic exposure through the consumption of aquaculture products are deemed non-threatening to human health (Chen et al., 2018), numerous studies have linked the escalation in antimicrobial resistance within aquaculture environments to the resistance observed in human pathogens (Chen et al., 2022; Li et al., 2020; Salgueiro et al., 2020). This situation underscores the imperative for alternatives to traditional antibiotic usage in disease prevention and control within aquaculture, facilitating the development of a sustainable and prosperous industry. Hence, metagenomics emerges as a viable option within aquaculture production, offering a safe and environmentally less aggressive methodology in disease control and prevention. This approach ensures a proactive assessment of disease establishment and prevalence in freshwater aquaculture systems.
5 Conclusion
Climate changes and common practices within aquaculture systems contribute to altering the dynamics of pathogens in freshwater environments, potentially facilitating the emergence of new pathogens or establishing the prevalence of specific microorganisms relative to others. Consequently, there is an imperative to establish sustainable management practices aimed at monitoring diseases beyond traditional production methods, that can be done by metagenomic analysis, coupled with bioinformatics tools and databases, to enable comprehensive understanding of the microbiota within both natural and artificial freshwater systems. From this perspective, the development of new technologies for metagenomic analysis in freshwater aquaculture has been the focus of developed countries, yet the number of technologies remains limited. Furthermore, among the main targets of pathogen monitoring research in recent years, viruses have been a category subject to increasing investigation, with evidence suggesting a constant emergence of new viruses in freshwater aquaculture. In this context, metagenomic analysis has emerged as a facilitating tool for identifying and monitoring new diseases, which represents a fast, safe, and non-invasive approach to environmental monitoring aimed at disease prevention in freshwater aquaculture, and it can add significant value to aquaculture products.
Data availability statement
The datasets analyzed in this study can be found in online repositories. The names of the repository/repositories and accession number(s) can be found in the article/supplementary material.
Author contributions
DM: Conceptualization, Data curation, Formal analysis, Investigation, Methodology, Writing – original draft. TA: Conceptualization, Data curation, Writing – original draft. ED: Formal analysis, Validation, Writing – review & editing. MR: Formal analysis, Validation, Writing – review & editing. OA: Formal analysis, Validation, Writing – review & editing. RR: Conceptualization, Formal analysis, Methodology, Supervision, Writing – review & editing.
Funding
The author(s) declare financial support was received for the research, authorship, and/or publication of this article. The authors would like to thank the Dean's Office for Research and Graduate Studies/Federal University of Pará-PROPESP/UFPA (PAPQ).
Conflict of interest
The authors declare that the research was conducted in the absence of any commercial or financial relationships that could be construed as a potential conflict of interest.
Publisher's note
All claims expressed in this article are solely those of the authors and do not necessarily represent those of their affiliated organizations, or those of the publisher, the editors and the reviewers. Any product that may be evaluated in this article, or claim that may be made by its manufacturer, is not guaranteed or endorsed by the publisher.
References
Alday-Sanz, V. (2018). Specific pathogen free (SPF), specific pathogen resistant (SPR) and specific pathogen tolerant (SPT) as part of the biosecurity strategy for whiteleg shrimp (Penaeus vannamei Boone 1931). Asian Fish Sci. 31S, 112–120. doi: 10.33997/j.afs.2018.31.S1.008
Alday-Sanz, V., Brock, J., Flegel, T. W., McIntosh, R., Bondad-Reantaso, M., Salazar, M., et al. (2020). Facts, truths and myths about SPF shrimp. Rev. Aquac. 12, 76–84. doi: 10.1111/raq.12305
Amaral, M. C. F., Medeiros, N. B. C., Rodrigues, M., Sousa, L. L., Jesus, E. C., Hamoy, I. G., et al. (2019). Management and technological practices in Amazonian fish farms: a case study in the Southeast of Pará. Aquaculture 507, 183–189. doi: 10.1016/j.aquaculture.2019.03.076
Assefa, A., and Abunna, F. (2018). Maintenance of fish health in aquaculture: review of epidemiological approaches for prevention and control of infectious disease of fish. Vet. Med. Int. 2018:5432497. doi: 10.1155/2018/5432497
Behera, B. K., Chakraborty, H. J., Patra, B., Rout, A. K., Dehury, B., Das, B. K., et al. (2020). Metagenomic analysis reveals bacterial and fungal diversity and their bioremediation potential from sediments of river Ganga and Yamuna in India. Front. Microbiol. 11:556136. doi: 10.3389/fmicb.2020.556136
Behera, B. K., Dehury, B., Rout, A. K., Patra, B., Mantri, N., Chakraborty, H. J., et al. (2021). Metagenomics study in aquatic resource management: recent trends, applied methodologies and future needs. Gene Rep. 25:101372. doi: 10.1016/j.genrep.2021.101372
Béné, C., Arthur, R., Norbury, H., Allison, E. H., Beveridge, M. C. M., Bush, S., et al. (2016). Contribution of fisheries and aquaculture to food security and poverty reduction: assessing the current evidence. World Dev. 79, 177–196. doi: 10.1016/j.worlddev.2015.11.007
Bennett, A., Basurto, X., Virdin, J., Lin, X., Betances, S. J., Smith, M. D., et al. (2021). Recognize fish as food in policy discourse and development funding. Ambio 50, 981–989. doi: 10.1007/s13280-020-01451-4
Bickle, M., Arculus, R., Barrett, P., DeConto, R., Camoin, G., Edwards, K., et al. (2011). Illuminating Earth's Past, Present, and Future: The Science Plan for the International Ocean Discovery Program 2013–2023. Washington, DC: I.O.D.P.M. International. Available at: https://www.iodp.org/112-high-resolution-pdf-version/file (acessed May 8, 2024).
Bjørndal, T., Dey, M., and Tusvik, A. (2024). Economic analysis of the contributions of aquaculture to future food security. Aquaculture 578:740071. doi: 10.1016/j.aquaculture.2023.740071
Bolyen, E., Rideout, J. R., Dillon, M. R., Bokulich, N. A., Abnet, C. C., Al-Ghalith, G. A., et al. (2019). Reproducible, interactive, scalable and extensible microbiome data science using QIIME 2. Nat. Biotechnol. 37, 852–857. doi: 10.1038/s41587-019-0252-6
Bouwmeester, M. M., Goedknegt, M. A., Poulin, R., and Thieltges, D. W. (2021). Collateral diseases: aquaculture impacts on wildlife infections. J. Appl. Ecol. 58, 453–464. doi: 10.1111/1365-2664.13775
Bowden, T. J., Smail, D. A., and Ellis, A. E. (2002). Development of a reproducible infectious pancreatic necrosis challenge model for Atlantic salmon Salmo salar L. J. Fish Dis. 25, 255–563. doi: 10.1046/j.1365-2761.2002.00402.x
Bush, S. R., van Zwieten, P. A. M., Visser, L., van Dijk, H., Bosma, R., de Boer, W. F., et al. (2010). Scenarios for resilient shrimp aquaculture in tropical coastal areas. Ecol. Soc. 15:215. doi: 10.5751/ES-03331-150215
Chen, H., Liu, S., Xu, X. R., Diao, Z. H., Sun, K. F., Hao, Q. W., et al. (2018). Tissue distribution, bioaccumulation characteristics and health risk of antibiotics in cultured fish from a typical aquaculture area. J. Hazard Mater. 343, 140–148. doi: 10.1016/j.jhazmat.2017.09.017
Chen, J., Sun, R., Pan, C., Sun, Y., Mai, B., and Li, Q. (2020). Antibiotics and food safety in aquaculture. J. Agric. Food Chem. 2020:3996. doi: 10.1021/acs.jafc.0c03996
Chen, X., He, Z., Zhao, J., Minze, L., Xue, Y., Zhou, J., et al. (2022). Metagenomic analysis of bacterial communities and antibiotic resistance genes in Penaeus monodon biofloc-based aquaculture environments. Front. Mar. Sci. 8:762345. doi: 10.3389/fmars.2021.762345
Cojocaru, A. L., Liu, Y., Smith, M. D., Akpalu, W., Chávez, C., Dey, M. M., et al. (2022). The “seafood” system: aquatic foods, food security, and the global south. Rev. Environ. Econ. Pol. 2022:721032. doi: 10.1086/721032
Costa-Pierce, B. A., and Chopin, T. (2021). The hype, fantasies and realities of aquaculture development globally and in its new geographies. World Aquac. 2, 23–35.
Coughlan, L. M., Cotter, P. D., Hill, C., and Alvarez-Ordóñez, A. (2015). Biotechnological applications of functional metagenomics in the food and pharmaceutical industries. Front. Microbiol. 6:72. doi: 10.3389/fmicb.2015.00672
Dolan, F., Lamontagne, J., Link, R., Hejazi, M., Reed, P., Edmonds, J., et al. (2021). Evaluating the economic impact of water scarcity in a changing world. Nat. Commun. 12, 1–10. doi: 10.1038/s41467-021-22194-0
Edgar, R. C. (2010). Search and clustering orders of magnitude faster than BLAST. Bioinformatics 26, 2460–2461. doi: 10.1093/bioinformatics/btq461
El-Sayed, A., and Kamel, M. (2020). Climatic changes and their role in emergence and re-emergence of diseases. Environ. Sci. Pollut. Res. 27, 22336–22352. doi: 10.1007/s11356-020-08896-w
Fang, H., Huang, K., Yu, J., Ding, C., Wang, Z., Zhao, C., et al. (2019). Metagenomic analysis of bacterial communities and antibiotic resistance genes in the Eriocheir sinensis freshwater aquaculture environment. Chemosphere 224, 202–211. doi: 10.1016/j.chemosphere.2019.02.068
FAO (2017). Aquaculture, the Sustainable Development Goals (SDGs)/Agenda 2030 and FAO'S Common Vision for Sustainable Food and Agriculture. Available at: http://www.fao.org/cofi/30794-011acfda6d140b8ede06f0b184c8e5fd4.pdf (accessed January 10, 2023).
FAO (2020). The State of World Fisheries and Aquaculture 2020: Sustainability in Action. Food and Agriculture Organization of the United Nations. Available at: http://www.fao.org/3/ca9229en/ca9229en.pdf (accessed August 06, 2024).
FAO (2022). El estado mundial de la pesca y la acuicultura 2022. Hacia la transformación azul. Rome: FAO.
FAO Fisheries and Aquaculture (2016). FAO Yearbook—Fishery and Aquaculture Statistics. Available at: http://www.fao.org/documents/card/en/c/570fc84b-5d02-4345-a6b3-5876279e4a8d (acessed May 8, 2024).
Florensa, A. F., Kaas, R. S., Clausen, P. T. L. C., Aytan-Aktug, D., and Aarestrup, F. M. (2022). ResFinder—an open online resource for identification of antimicrobial resistance genes in next-generation sequencing data and prediction of phenotypes from genotypes. Microb. Genom. 1:000748. doi: 10.1099/mgen.0.000748
Føre, M., Frank, K., Norton, T., Svendsen, E., Alfredsen, J. A., Dempster, T., et al. (2018). Precision fish farming: a new framework to improve production in aquaculture. Biosyst. Eng. 173, 176–193. doi: 10.1016/j.biosystemseng.2017.10.014
Galparsoro, I., Murillas, A., Pinarbasi, K., Sequeira, A. M., Stelzenmüller, V., Borja, Á., et al. (2020). Global stakeholder vision for ecosystem-based marine aquaculture expansion from coastal to offshore areas. Rev. Aquac. 4, 2061–2079. doi: 10.1111/raq.12422
Gangnonngiw, W., Bunnontae, M., Kayansamruaj, P., Senapin, S., Srisala, J., Flegel, W., et al. (2023). A novel ssDNA Bidnavirus in the giant freshwater prawn Macrobrachium rosenbergii. Aquaculture 568:739340. doi: 10.1016/j.aquaculture.2023.739340
Gephart, J. A., Golden, C. D., Asche, F., Belton, B., Brugere, C., Froehlich, H. E., et al. (2020). Scenarios for global aquaculture and its role in human nutrition. Rev. Fish Sci. Aquac. 29, 122–138. doi: 10.1080/23308249.2020.1782342
Goddard, S., and Delghandi, M. (2020). Importance of the conservation and management of freshwater to aquaculture. Freshwater 5, 35–44. doi: 10.1016/B978-0-12-409548-9.11954-2
Handelsman, J. (2004). Metagenomics: application of genomics to uncultured microorganisms. Microbiol. Mol. Biol. Rev. 4, 669–685. doi: 10.1128/MMBR.68.4.669-685.2004
Haro-Moreno, J. M., Coutinho, F. H., Zaragoza-Solas, A., Picazo, A., Almagro-Moreno, S., and López-Pérez, M. (2020). Dysbiosis in marine aquaculture revealed through microbiome analysis: reverse ecology for environmental sustainability. FEMS Microbiol. Ecol. 12:fiaa218. doi: 10.1093/femsec/fiaa218
Hemamalini, N., Shanmugam, S. A., Kathirvelpandian, A., Deepak, A., Kaliyamurthi, V., and Suresh, E. (2022). A critical review on the antimicrobial resistance, antibiotic residue and metagenomics-assisted antimicrobial resistance gene detection in freshwater aquaculture environment. Aquac. Res. 53, 344–366. doi: 10.1111/are.15601
HLPE (2014). Sustainable Fisheries and Aquaculture for Food Security and Nutrition. A Report by the High Level Panel of Experts on Food Security and Nutrition of the Committee on World Food Security. Available at: https://www.fao.org/fileadmin/user_upload/hlpe/hlpe_documents/HLPE_S_and_R/HLPE_2014_Sustainable_Fisheries_and_Aquaculture_Summary_EN.pdf (accessed June 10, 2024).
Huang, Z., Yuan, X., and Liu, X. (2021). The key drivers for the changes in global water scarcity: water withdrawal versus water availability. J. Hydrol. 601:126658. doi: 10.1016/j.jhydrol.2021.126658
Islam, S. I., Mou, M. J., Sanjida, S., and Mahfuj, S. (2023). A review on molecular detection techniques of white spot syndrome virus: perspectives of problems and solutions in shrimp farming. Vet. Med. Sci. 9, 778–801. doi: 10.1002/vms3.979
Jiang, H., Bao, J., Xing, Y., Cao, G., Li, X., and Chen, Q. (2022). Metabolomic and metagenomic analyses of the Chinese mitten crab Eriocheir sinensis after challenge with Metschnikowia bicuspidata. Front. Microbiol. 13:990737. doi: 10.3389/fmicb.2022.990737
Khanal, U., Wilson, C., Rahman, S., Lee, B. L., and Hoang, V. N. (2021). Smallholder farmers' adaptation to climate change and its potential contribution to UN's sustainable development goals of zero hunger and no poverty. J. Clean Prod. 281:124999. doi: 10.1016/j.jclepro.2020.124999
Klemetsen, T., Raknes, I. A., Fu, J., Agafonov, A., Balasundaram, S. V., Tartari, G., et al. (2018). The MAR databases: development and implementation of databases specific for marine metagenomics. Nucl. Acids Res. 46, D692–D699. doi: 10.1093/nar/gkx1036
Lakin, S. M., Dean, C., Noyes, N. R., Dettenwanger, A., Ross, A. S., Doster, E., et al. (2017). MEGARes: an antimicrobial resistance database for high throughput sequencing. Nucl. Acids Res. 45, D574–D580. doi: 10.1093/nar/gkw1009
Langille, M. G., Zaneveld, J., Caporaso, J. G., McDonald, D., Knights, D., Reyes, J. A., et al. (2013). Predictive functional profiling of microbial communities using 16S rRNA marker gene sequences. Nat. Biotechnol. 31, 814–821. doi: 10.1038/nbt.2676
Leung, T. L. F., and Bates, A. E. (2013). More rapid and severe disease outbreaks for aquaculture at the tropics: implications for food security. J. Appl. Ecol. 50, 215–222. doi: 10.1111/1365-2644.12017
Lew, S., Glińska-Lewczuk, K., Burandt, P., Kulesza, K., Kobus, S., and Obolewski, K. (2022). Salinity as a determinant structuring microbial communities in coastal lakes. Int. J. Environ. Res. Publ. Health 19:4592. doi: 10.3390/ijerph19084592
Li, J., Wen, J., Chen, Y., Wang, Q., and Yin, J. (2020). Antibiotics in cultured freshwater products in Eastern China: occurrence, human health risks, sources, and bioaccumulation potential. Chemosphere 1:128441. doi: 10.1016/j.chemosphere.2020.128441
Macedo, G., van Veelen, H. P. J., Hernandez-Leal, L., van der Maas, P., Heederik, D., Mevius, D., et al. (2021). Targeted metagenomics reveals inferior resilience of farm soil resistome compared to soil microbiome after manure application. Sci. Tot. Environ. 770:145399. doi: 10.1016/j.scitotenv.2021.145399
Majdi, N., Uthoff, J., Traunspurger, W., Laffaille, P., and Maire, A. (2020). Effect of water warming on the structure of biofilm-dwelling communities. Ecol. Indic. 117:106622. doi: 10.1016/j.ecolind.2020.106622
Marcogliese, D. J. (2008). The impact of climate change on the parasites and infectious diseases of aquatic animals. Rev. Sci. Tech. Off. Int. Epiz. 27, 467–484. doi: 10.20506/rst.27.2.1820
Martínez-Porchas, M., and Vargas-Albores, F. (2017). Microbial metagenomics in aquaculture: a potential tool for a deeper insight into the activity. Rev. Aquac. 9, 42–56. doi: 10.1111/raq.12102
Maulu, S., Hasimuna, O. J., Haambiya, L. H., Monde, C., Musuka, C. G., Makorwa, T. H., et al. (2021). Climate change effects on aquaculture production: sustainability implications, mitigation, and adaptations. Front. Sustain. Food Syst. 5:609097. doi: 10.3389/fsufs.2021.609097
McArthur, A. G., Waglechner, N., Nizam, F., Yan, A., Azad, M. A., Baylay, A. J., et al. (2013). The comprehensive antibiotic resistance database. Antimicrob. Agents Chemother. 57, 3348–3357. doi: 10.1128/AAC.00419-13
Menzel, P., Kim, L. N., and Krogh, A. (2016). Fast and sensitive taxonomic classification for metagenomics with Kaiju. Nat. Commun. 7:11257. doi: 10.1038/ncomms11257
Miller, R. R., Montoya, V., Gardy, J. L., Patrick, D. M., and Tang, P. (2013). Metagenomics for pathogen detection in public health. Genome Med. 5:gm485. doi: 10.1186/gm485
Mizik, T. (2023). How can precision farming work on a small scale? A systematic literature review. Precision Agric. 24, 384–406. doi: 10.1007/s11119-022-09934-y
Munang'andu, H. M. (2016). Environmental viral metagenomics analyses in aquaculture: applications in epidemiology and disease control. Front. Microbiol. 7:1986. doi: 10.3389/fmicb.2016.01986
Munang'andu, H. M., Mugimba, K. K., Byarugaba, D. K., Mutoloki, S., and Evensen, Ø. (2017). Current advances on virus discovery and diagnostic role of viral metagenomics in aquatic organisms. Front. Microbiol. 8:406. doi: 10.3389/fmicb.2017.00406
Murray, A. G., and Peeler, E. J. (2005). A framework for understanding the potential for emerging diseases in aquaculture. Prev. Vet. Med. 67, 223–235. doi: 10.1016/j.prevetmed.2004.10.012
New, F. N., and Brito, I. L. (2020). Annual review of microbiology what is metagenomics teaching us, and what is missed? Annu. Rev. Microbiol. 2020, 117–135. doi: 10.1146/annurev-micro-012520-072314
Nielsen, H. B., Almeida, M., Juncker, A. S., Rasmussen, S., Li, J., Sunagawa, S., et al. (2014). Identification and assembly of genomes and genetic elements in complex metagenomic samples without using reference genomes. Nat. Biotechnol. 32, 822–828. doi: 10.1038/nbt.2939
Noble, A. C., and Summerfelt, S. T. (1996). Diseases encountered in rainbow trout cultured in recirculating systems. Annu. Rev. Fish Dis. 6, 199665–199692. doi: 10.1016/S0959-8030(96)90006-X
NU (2015). Transforming Our World: the 2030 Agenda for Sustainable Development. New York, NY: United Nations.
Offiong, N. O., Edet, J. B., Shaibu, S. E., Akan, N. E., Atakpa, E. O., Sanganyado, E., et al. (2023). Metagenomics: an emerging tool for the chemistry of environmental remediation. Front. Environ. Chem. 4:1052697. doi: 10.3389/fenvc.2023.1052697
Okamura, B., and Feist, S. W. (2011). Emerging diseases in freshwater systems. Freshw. Biol. 56, 627–637. doi: 10.1111/j.1365-2427.2011.02578.x
Ottinger, M., Clauss, K., and Kuenzer, C. (2018). Opportunities and challenges for the estimation of aquaculture production based on earth observation data. Remote Sens. 10:1076. doi: 10.3390/rs10071076
Page, M. J., Moher, D., Bossuyt, P. M., Boutron, I., Hoffmann, T. C., Mulrow, C. D., et al. (2021). PRISMA 2020 explanation and elaboration: updated guidance and exemplars for reporting systematic reviews. Br. Med. J. 372:n160. doi: 10.1136/bmj.n160
Park, J. H., Song, K. C., Han Jung, K., Kim, Y. G, Kim, G. J., Kim, H. G., et al. (2016). Method of Producing Sterile Aquaculture Water, and Method Using Same of Fish Aquaculture Using Flowing Sterile Water. EP N. EP3017696. Application: 31.03.2015. Publication: 11.05.2016. Available at: https://patentscope.wipo.int/search/en/detail.jsf?docId=EP173338437&_cid=P22-M1HVPR-12947-1
Peters, L., Spatharis, S., Dario, M. A., Dwyer, T., Roca, I. J. T., Kintner, A., et al. (2018). Environmental DNA: A new low-cost monitoring tool for pathogens in salmonid aquaculture. Front. Microbiol. 9:9. doi: 10.3389/fmicb.2018.03009
Qian, Q., Zhou, Y., Chen, Z., Zhu, Y., Xu, J., Gao, X., et al. (2023). Pathogenesis and complete genome sequence of Decapod iridescent virus 1 (DIV1) associated with mass mortality in Macrobrachium rosenbergii. Aquaculture 566:739220. doi: 10.1016/j.aquaculture.2022.739220
Ragasa, C., Charo-Karisa, H., Rurangwa, E., Tran, N., and Mashiasia, K. (2022). Sustainable aquaculture development in sub-Saharan Africa. Nat. Food 3, 92–94. doi: 10.1038/s43016-022-00467-1
Rath, K. M., Fierer, N., Murphy, D. V., and Rousk, J. (2019). Linking bacterial community composition to soil salinity along environmental gradients. ISME J. 13, 836–846. doi: 10.1038/s41396-018-0313-8
Rout, A. K., Dehury, B., Parida, P. K., Sarkar, D. J., Behera, B., Das, B. K., et al. (2022). Taxonomic profiling and functional gene annotation of microbial communities in sediment of river Ganga at Kanpur, India: insights from whole-genome metagenomics study. Environ. Sci. Pollut. Res. 29, 82309–82323. doi: 10.1007/s11356-022-21644-6
Rout, A. K., Tripathy, P. S., Dixit, S., Behera, D. U., Behera, B., Das, B. K., et al. (2023). Unveiling the microbiome landscape: a metagenomic study of bacterial diversity, antibiotic resistance, and virulence factors in the sediments of the river ganga, India. Antibiotics 12:1735. doi: 10.3390/antibiotics12121735
Sae-Lim, P., Kause, A. H, Mulder, A., and Olesen, I. (2017). Breeding and genetics symposium: climate change and selective breeding in aquaculture. J. Anim. Sci. 95, 1801–1812. doi: 10.2527/jas.2016.1066
Salgueiro, V., Manageiro, V., Bandarra, N. M., Reis, L., Ferreira, E., and Caniça, M. (2020). Bacterial diversity and antibiotic susceptibility of Sparus aurata from aquaculture. Microorganisms 9:1343. doi: 10.3390/microorganisms8091343
Santos, C. F., Agardy, T., Andrade, F., Calado, H., Crowder, L. B., Ehler, C. N., et al. (2020). Integrating climate change in ocean planning. Nat. Sustain. 3, 505–516. doi: 10.1038/s41893-020-0513-x
Sayre, R., and Vinogradava-Shah, T. (2020). Novel System for the Biocontrol of Pathogens in Aquaculture and Other Animal Systems. EP N. EP3601557. Application: 02.04.2018. Publication: 05.02.2020. Available at: https://patentscope.wipo.int/search/en/detail.jsf?docId=EP283374369&_fid=IN283300932
Sayre, R., Vinogradova-Shah, T., and Sineva, E. (2020). Systems and Methods for the Control of Acute Hepatopancreatic Necrosis Disease. US N. US20200178566. Application: 07.08.2018. Publication: 11.06.2020. Available at: https://patentscope.wipo.int/search/en/detail.jsf?docId=US296006564&_cid=P22-M1HW2N-23321-2
Schloss, P. D., Gevers, D., and Westcott, S. L. (2011). Reducing the effects of PCR amplification and sequencing artifacts on 16S rRNA-based studies. PLoS ONE 6:e27310. doi: 10.1371/journal.pone.0027310
Schloss, P. D., Westcott, S. L., Ryabin, T., Hall, J. R., Hartmann, M., Hollister, E. B., et al. (2009). Introducing mothur: open-source, platform-independent, community-supported software for describing and comparing microbial communities. Appl. Environ. Microbiol. 75, 7537–7541. doi: 10.1128/AEM.01541-09
Sineva, E., Costa-Nunes, P., and Vinogradova-Shah, T. (2023). Novel Probiotic Bacteria and Methods to Control Pathogens in Aquatic Animals. US N. US20230233621. Application: 11.06.2021. Publication: 27.07.2023.
Stilwell, J. M., Camus, A. C., Woodyard, E. T., Ware, C., Rosser, T. G., Gunn, M. A., et al. (2023). Species-specific in situ hybridization confirms arrested development of Henneguya ictaluri in hybrid catfish (Channel Catfish × Blue Catfish) under experimental conditions, with notes on mixed-species infections in clinical cases of proliferative gill disease from Mississippi catfish aquaculture. J. Aquat. Anim. Health 35, 223–237. doi: 10.1002/aah.10196
Stilwell, J. M., Griffin, M. J., Waldbieser, G. C., Stanton, J. B., Leary, J. H., Khoo, L. H., et al. (2022). Myxozoan community composition and diversity in clinical cases of proliferative gill disease in mississippi catfish aquaculture. J. Parasitol. 108, 132–140. doi: 10.1645/21-57
Streit, W. R., and Schmitz, R. A. (2004). Metagenomics—the key to the uncultured microbes. Curr. Opin. Microbiol. 7, 492–498. doi: 10.1016/j.mib.2004.08.002
Subasinghe, R., Alday-Sanz, V., Bondad-Reantaso, M. G., Jie, H., Shinn, A. P., and Sorgeloos, P. (2023). Biosecurity: reducing the burden of disease. J. World Aquac. Soc. 54, 397–426. doi: 10.1111/jwas.12966
Thompson, B. M., and Siegel, A. (2017). Fusion Proteins, Recombinant Bacteria, and Exosporium Fragments for Animal Health and Aquaculture. CA N. CA3016922. Application: 16.03.2017. Publication: 21.09.2017. Available at: https://patentscope.wipo.int/search/en/detail.jsf?docId=CA232201214&_fid=WO2017161181
Troell, M., Costa-Pierce, B., Stead, S., Cottrell, R. S., Brugere, C., Farmery, A. K., et al. (2023). Perspectives on aquaculture's contribution to the Sustainable Development Goals for improved human and planetary health. J. World Aquac. Soc. 54, 251–342. doi: 10.1111/jwas.12946
Tyagi, A., Singh, B., Thammegowda, N. K. B., and Singh, N. K. (2019). Shotgun metagenomics offers novel insights into taxonomic compositions, metabolic pathways and antibiotic resistance genes in fish gut microbiome. Arch. Microbiol. 201, 295–303. doi: 10.1007/s00203-018-1615-y
Van Eck, N., and Waltman, L. (2010). Software survey: VOSviewer, a computer program for bibliometric mapping. Scientometrics 84, 523–538. doi: 10.1007/s11192-009-0146-3
Verdegem, M., Buschmann, A. H., Latt, U. W., Dalsgaard, A. J. T., and Lovatelli, A. (2023). The contribution of aquaculture systems to global aquaculture production. World Aquac. Soc. 54, 206–250. doi: 10.1111/jwas.12963
Wang, X., Olsen, L. M., Reitan, K. I., and Olsen, Y. (2012). Discharge of nutrient wastes from salmon farms: environmental effects, and potential for integrated multi-trophic aquaculture. Aquac. Environ. Interact. 2, 267–283. doi: 10.3354/aei00044
WHO (2020). The State of Food Security and Nutrition in the World 2020. Transforming Food Systems for Affordable Healthy Diets. Rome: FAO.
Wood, D. E., and Salzberg, S. L. (2014). Kraken: ultrafast metagenomic sequence classification using exact alignments. Genome Biol. 15:R46. doi: 10.1186/gb-2014-15-3-r46
Xie, Y., Sun, H., Xue, M., and Liu, J. (2022). Metagenomics reveals differences in microbial composition and metabolic functions in the rumen of dairy cows with different residual feed intake. Anim. Microbiome 4:3. doi: 10.1186/s42523-022-00170-3
Xue, M., Jiang, N., Fan, Y., Yang, T., Li, M., Liu, W., et al. (2022). White spot syndrome virus (WSSV) infection alters gut histopathology and microbiota composition in crayfish (Procambarus clarkii). Aquac. Rep. 22:101006. doi: 10.1016/j.aqrep.2022.101006
Yadav, R., Kohli, A. S., and Prakash, J. (2020). Metagenomics current research, application and computational analysis. J. Med. Pharm. Allied Sci. 11:4901–4908. doi: 10.55522/jmpas.V11I3.2931
Yamashita, N. (2021). Economic crisis and innovation capacity of Japan: evidence from cross-country patent citations. Technovation 101:102208. doi: 10.1016/j.technovation.2020.102208
Zhou, Y., Kang, S., and Tan, G. (2019). Community evolution in international patent application Top1 networks. Recent Trends Decision Sci. Manag. 1142, 649–657. doi: 10.1007/978-981-15-3588-8_75
Zhu, W., Lomsadze, A., and Borodovsky, M. (2010). Ab initio gene identification in metagenomic sequences. Nucl. Acids Res. 38:e132. doi: 10.1093/nar/gkq275
Keywords: aquatic systems, fish, crustaceans, disease control, microbial community, methods
Citation: Macedo DB, dos Anjos TMC, De Los Santos EFF, Rodrigues MDN, Alegria OVC and Ramos RTJ (2024) New perspectives on metagenomic analysis for pathogen monitoring in sustainable freshwater aquaculture production: a systematic review. Front. Freshw. Sci. 2:1459233. doi: 10.3389/ffwsc.2024.1459233
Received: 04 July 2024; Accepted: 17 September 2024;
Published: 02 October 2024.
Edited by:
Ioannis A. Giantsis, Aristotle University of Thessaloniki, GreeceReviewed by:
Ilenia Azzena, University of Sassari, ItalyAjaya Kumar Rout, Rani Lakshmi Bai Central Agricultural University, India
Jitendra Kumar Sundaray, Indian Council of Agricultural Research, India
Copyright © 2024 Macedo, dos Anjos, De Los Santos, Rodrigues, Alegria and Ramos. This is an open-access article distributed under the terms of the Creative Commons Attribution License (CC BY). The use, distribution or reproduction in other forums is permitted, provided the original author(s) and the copyright owner(s) are credited and that the original publication in this journal is cited, in accordance with accepted academic practice. No use, distribution or reproduction is permitted which does not comply with these terms.
*Correspondence: Rommel Thiago Jucá Ramos, cm9tbWVscmFtb3NAdWZwYS5icg==