- 1International Centre for Ecohydraulics Research, Faculty of Engineering and Physical Sciences, University of Southampton, Southampton, United Kingdom
- 2Faculty of Engineering and Physical Science, Institute of Sound and Vibration Research, University of Southampton, Southampton, United Kingdom
Introduction: Deterrents that use acoustics to guide fish away from dangerous areas (e.g., water intakes) depend on the elicitation of avoidance in the target species. Background noise is often neglected when testing acoustic deterrents, but it is important to account for its effects as freshwater environments present a wide variety of ambient soundscapes.
Methods: Using the widely studied goldfish (Carassius auratus) as a suitable experimental model, this study adopted a reductionist approach to investigate the relationship between the startle response to a pure tone signal and background noise. Under laboratory conditions, the startle responses of individual goldfish exposed to 120 ms tones at 250 Hz and four Sound Pressure Levels (SPLs: 115, 125, 135, 145 dB re 1 μPa) were quantified in the presence (treatment) and absence (ambient - control) of band-limited random noise (105 dB re 1 μPa).
Results and discussion: When observing the dose-response relationship, the proportion of fish that startled to the signal increased with SPL in both the treatment and control, although there was no difference between them, suggesting that the signal-to-noise ratio was not influential under the conditions tested. However, further analysis using Signal Detection Theory indicated that the was higher in the noisy treatment than the control when responding to both false alarms (startle during a pre-signal period) and hits (startle to an external stimulus during the pre-signal period). Furthermore, fish were better able to discriminate (d?) external stimuli over time (during the pre-signal period) in the treatment than control. There is a need to consider the role of background noise when designing acoustic fish deterrents that depend on the exhibition of avoidance behaviors.
Introduction
Globally, many populations of fish have suffered dramatic declines over recent decades [e.g., −36% in marine ecosystems between 1970 and 2012 (Reid et al., 2018) and −83% for fresh waters between 1970 and 2014 (Darwall et al., 2018)]. Potential explanations include climate change (Poff et al., 2012; Plagányi, 2019) and associated ocean acidification (Munday et al., 2014); overfishing (Le Pape et al., 2017); novel pollution such as microplastics (Birkenhead et al., 2020), noise (Currie et al., 2021), and artificial light at night (Vowles and Kemp, 2021); invasive species (Daniels and Kemp, 2022); and the cumulative effects of multiple stressors (Reid et al., 2018; Bayramoglu et al., 2019). Limiting the impacts of such factors in both the marine and freshwater environment forms a central tenet of a plethora of environmental legislation. Examples include laws that prohibit the discharge of oil or hazardous substances (e.g., the Clean Water Act 1972 in the United States of America), release of invasive species (e.g., Fisheries Management Act 2007 in Australia), or the construction of infrastructure, such as dams and weirs, without appropriate mitigation (e.g., Salmon and Freshwater Fisheries Act 1975 in the United Kingdom).
Over many decades, a range of environmental impact mitigation technologies have been developed to meet regulatory requirements aimed to conserve fish populations (Kemp, 2015). For example, fish passes have been designed to connect habitats that would otherwise be fragmented by dams, enabling life-history strategies to be fulfilled and sufficient gene flow to maintain population viability (Mallen-Cooper and Brand, 2007; Wilkes et al., 2018). Similarly, physical fish screens have been employed at the intakes of cooling water systems or hydropower turbines are intended to reduce entrainment and potential loss of fish (Baumgartner and Boys, 2012). The effectiveness of such environmental impact mitigation solutions, however, can be highly variable between site, context, and species (Roscoe and Hinch, 2010; Noonan et al., 2011). This can result in unintended negative consequences such as promoting the spread of invasive species (McLaughlin et al., 2012), while physical screens themselves may cause injury and death due to mechanical abrasion and impingement (Swanson et al., 2005; Black and Perry, 2014). To improve the performance of environmental impact mitigation technology, behavioral devices may have a role to play when used in combination. This was illustrated experimentally by Deleau et al. (2019) and Miller et al. (2022) who employed acoustic and electric fields, respectively, in the application of the marginal gains concept to fish screening.
Behavioral devices typically exploit a deterrent effect induced by a stimulus to modify the trajectory of a moving fish. A variety of abiotic stimuli have been used, including strobe lights (Kim and Mandrak, 2017), electricity (Miller et al., 2021), bubbles (Flores-Martin et al., 2021) and acoustics experimentally (Currie et al., 2020, 2021) and in situ (Piper et al., 2019). Concentrating on acoustics, underwater sound should provide an effective deterrent because it is omnidirectional (for the frequencies and source sizes commonly used) and so can reach multiple individuals simultaneously. It is also unaffected by variation in illumination (e.g., due to time of the day), unlike stimuli that are mediated through vision. As such, acoustic deterrents have been employed to protect fish at cooling water intakes (Maes et al., 2004) and abstraction points (Piper et al., 2019), as well as to control invasive species such as silver carp (Hypophthalmichthys molitrix) in the Great Lakes, USA (Vetter et al., 2015). However, the efficacy of acoustic deterrents can be highly variable (see Wamboldt, 2019; Bzonek et al., 2021), with none being completely effective (Putland and Mensinger, 2019).
There is considerable variability in responses of individuals to acoustic deterrents. For instance, deterrence efficiency can differ between European spratt (Sprattus sprattus) from 88% (Maes et al., 2004) to 11% (Turnpenny and Nedwell, 2003). Within studies, variation in responses may occur due to differences in sound parameters and has, for example, resulted in efficacies of 31% to 88% (Jesus et al., 2018) for Northern straight-mouth nase (Pseudochondrostoma duriense). Differences between studies may be a result of the experimental methodology employed, for example the stimulus may have been played at a frequency outside the hearing range (Putland and Mensinger, 2019). Additionally, the process of trial and error seen throughout the literature is unlikely to increase the effectiveness of acoustic deterrents (Deleau et al., 2019, 2020). There is, hence, a requirement to return to fundamental first principles to better understand the mechanisms that determine deterrence efficacy.
Background noise is also commonly disregarded in acoustic deterrence studies (Putland and Mensinger, 2019). It is important to measure the ambient background noise when quantifying the effectiveness of acoustic deterrents because this provides information on the signal-to-noise ratio (SNR) at which an avoidance response is elicited. As the SNR increases, the probability of an organism discriminating or discerning the signal is expected to rise whereas, at a low SNR, the signal may be masked by the ambient noise (Currie et al., 2019). However, a limited number of studies have demonstrated that fish may exhibit avoidance behaviors more frequently in noisy environments (Purser and Radford, 2011; Voellmy et al., 2014; Shafiei Sabet et al., 2015). In this scenario, the noise used did not act as a masker to a signal but evoked an avoidance response more frequently compared to the ambient equivalent.
To examine the relationship between ambient noise levels and avoidance displayed by fish in response to an acoustic signal, this experiment investigated how the elicitation of a startle response to pure tones differed to those in presence of band-limited random noise. Goldfish (Carassius auratus), an otophysan with specialized hearing capabilities, was selected as the model species due to the large amount of physiologically derived (e.g., Auditory Evoked Potential, AEP) information available (Popper and Clarke, 1976; Fay, 1984; Kenyon et al., 1998; Ladich and Fay, 2013) and their ease of maintenance under laboratory conditions. Using the presence (treatment) and absence (ambient - control) of band-limited random noise (105 dB re 1 μPa), this study compared the startle response exhibited by goldfish to four signals of differing amplitude [Sound Pressure Level (SPL): 115, 125, 134, 145 dB re 1 uPa]: (1) within each of the two noise conditions; (2) between each of the two noise conditions, involving (2a) coarse scale (dose-response relationship) and (2b) fine-scale analysis. In addition to a coarse-scale quantification using the avoidance responses (startle responses) to determine the dose-response relationship, for Objective 2b a fine-scale analysis was conducted using Signal Detection Theory (SDT) (Kemp et al., 2012).
Methods
Fish husbandry
Goldfish [N = 160; mean standard length (SD): 65.7 (6.8) mm; mass: 10.6 (3.1) g] were transported in three batches from Hampshire Carp Hatcheries (UK) in oxygenated water to the International Centre for Ecohydraulics Research (ICER) facility, University of Southampton, in April 2021. They were maintained in husbandry tanks (1.50 m length × 1.00 m width × 0.80 m depth, filled to 0.68 m water depth) containing ≈1.2 m3 of aerated, filtered and dechlorinated water, under an artificial photoperiod (L:D 14:10) and fed once daily (Tetra goldfish flakes; protein: 42%). Water quality parameters comprising ammonia [0.06 (0.09) ppm], nitrites [0 (0) ppm], nitrates [40 (0) ppm], pH [pH 8.2 (0.04)] (API Freshwater Master Test Kit) and temperature [11.6 (1.1)°C] were monitored daily. The night before trials commenced, fish were transported to the experimental facility (A. B. Wood Laboratory, University of Southampton) and kept in holding tanks (0.5 m width × 0.84 m length × 0.64 m depth, filled to 0.54 m water depth) containing a total of ≈0.5 m3 of aerated, internally filtered and dechlorinated water [temperature: 11.6 (0.7)°C]. Fish were acclimated for at least 4 days in the husbandry tanks and 14 hours in the holding tanks before trials commenced. On completion of each trial, the subject fish (a single individual per trial) were placed in a post-test tank and returned to the ICER husbandry facility at the end of the day.
Experimental setup
Trials were conducted in a white medium density polyethylene cylindrical arena (modified 100 L Round Water Tank; 55.5 cm diameter × 45 cm depth, 4 mm thick; Direct Water Tanks, Retford, Nottinghamshire, UK) suspended in a large tank (8 m length × 8 m width × 5 m depth). The arena was suspended in water (Figure 1) using a bespoke metal frame to ameliorate the typical challenges faced by using a small tank in air (Holgate et al., 2023). By doing so, the impedance difference between the water in the tank and the surrounding air was reduced, creating a more homogeneous sound field in terms of SPL. The experimental arena was covered by a black polyethylene mesh (6 mm mesh width) to prevent escape of fish and filled to a depth of 30 cm. Water was replaced (≈20 L water change) after each trial to maintain good water quality. An underwater transducer (Electro-Voice UW-30; maximal output 153 dB re 1 μPa at 1 m for 150 Hz, Lubell Labs, Columbus, USA) was suspended 70 cm below the arena and a hydrophone (8105, manufacturer-calibrated sensitivity −205 dB re: 1 V/ μPa; Brüel and Kjær, Denmark) placed 20 cm from the tank to continuously monitor the sound throughout each trial. Trials were recorded via a webcam (C920; HD 1080p; 24 frames per second; Logitech Pro, Switzerland) installed directly above the experimental arena and footage subsequently analyzed. The laboratory was lit by fluorescent lighting which provided sufficient illumination for video recording.
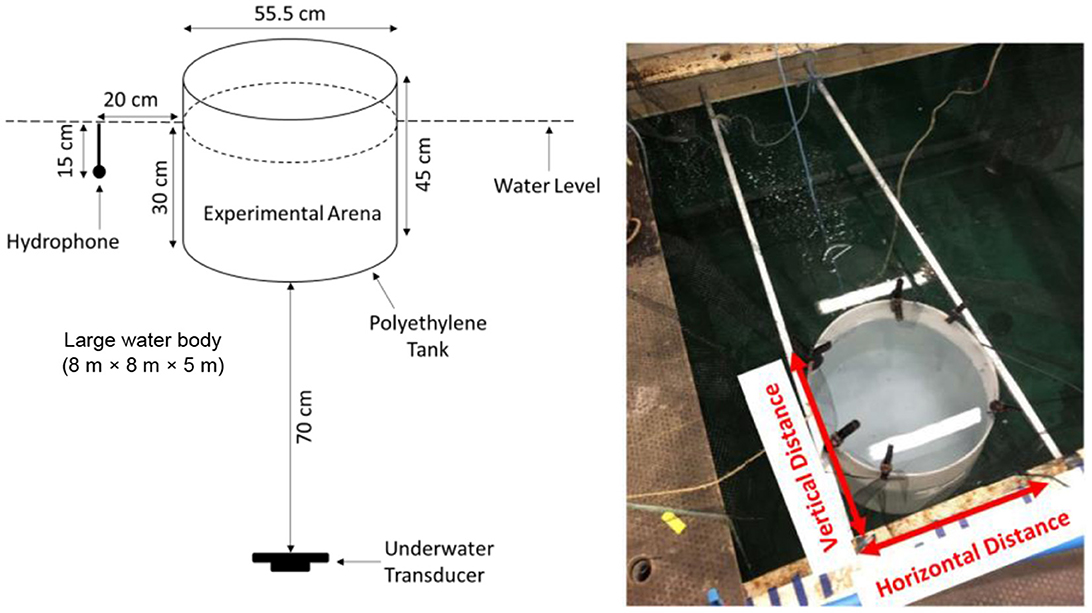
Figure 1. The experimental setup used to investigate the avoidance of goldfish as indicated by the exhibition of startle response to acoustics signals played with different levels of background noise (Holgate et al., 2023). The setup illustrates a cylindrical tank (experimental arena) installed within a large tank (8 m × 8 m × 5 m) to create a homogeneous sound field. The vertical and horizontal distance have been labeled and were used for the researcher to undertake acoustic mapping.
Experimental design
The study consisted of 20 replicates of 8 treatments (160 trials) based on a combination of a 250 Hz signal played at one of four SPLs (115, 125, 135 or 145 dB re 1 μPa) under one of two background noise conditions (treatment: 105 dB re 1 μPa band-limited 100–2500 Hz random noise; ambient control: ambient conditions). The frequency and SPLs of the signal were selected based on the results of a previous study (Holgate et al., 2023) in which 250 Hz was found to elicit an avoidance response more readily than any other frequency. The background noise was selected in light of the 3Rs (Prescott and Lidster, 2017) such that the SPL wasn't damaging to the ear but sufficiently above the ambient noise levels.
Prior to the start of each trial, conducted between 16 and 30 April 2021, a single fish (total number of individuals, N = 160) was acclimated in the experimental arena for 30 min. During the trials, the fish were randomly assigned a background noise level (ambient or 105 dB re 1 μPa band-limited noise). Following this, each individual experienced a total of four exposures of the same treatment (to determine if there was any tolerance of the signal). Each exposure consisted of a sinusoidal 120 ms tone ramped with a 20 ms Hanning taper (Holgate et al., 2023) and was followed by 4 min (pre-signal period) before the next exposure. Although the activation latency of the Mauthner cells (neurons located in the hindbrain responsible for mediating a rapid escape reflex) in goldfish is 5–10 ms, the tone was played at 120 ms to ensure sufficient time for the frequency spectrum to be well defined (Eaton et al., 1977; Zeddies and Fay, 2005). Fish behavior was continuously recorded during the trial and each fish was used once only.
Acoustic stimuli and sound mapping
Acoustic samples were generated in MATLAB (Release 2019b, The Mathworks, Inc., Natick, USA) using a laptop connected to a DAQ (NI USB-6212; National Instruments, USA), transmitting the signal through an amplifier (Prosound Power AMP 200; frequency response: 20–20 kHz) and emitted via the UW30 underwater transducer. Acoustic signals were standardized such that the desired SPL was reached in the center of the experimental arena. Use of artificially generated signals allowed for control of the specific acoustic components tested.
Prior to conducting trials, the sound pressure field within the experimental arena was mapped at regular points (Figure 2) using a calibrated hydrophone (Brüel and Kjær 8105) attached to a manual slider (82 measurements, each 5 cm apart) at three depths (5 cm, 15 cm, 25 cm measured from the water surface). Data capture and stimulus generation were synchronized to facilitate computation of the Particle Acceleration (PA). Both SPL and PA were quantified to create maps of the sound field. The PA, a, was calculated as:
where ρ is the ambient density and ∇P is the pressure gradient (Kinsler et al., 1982).
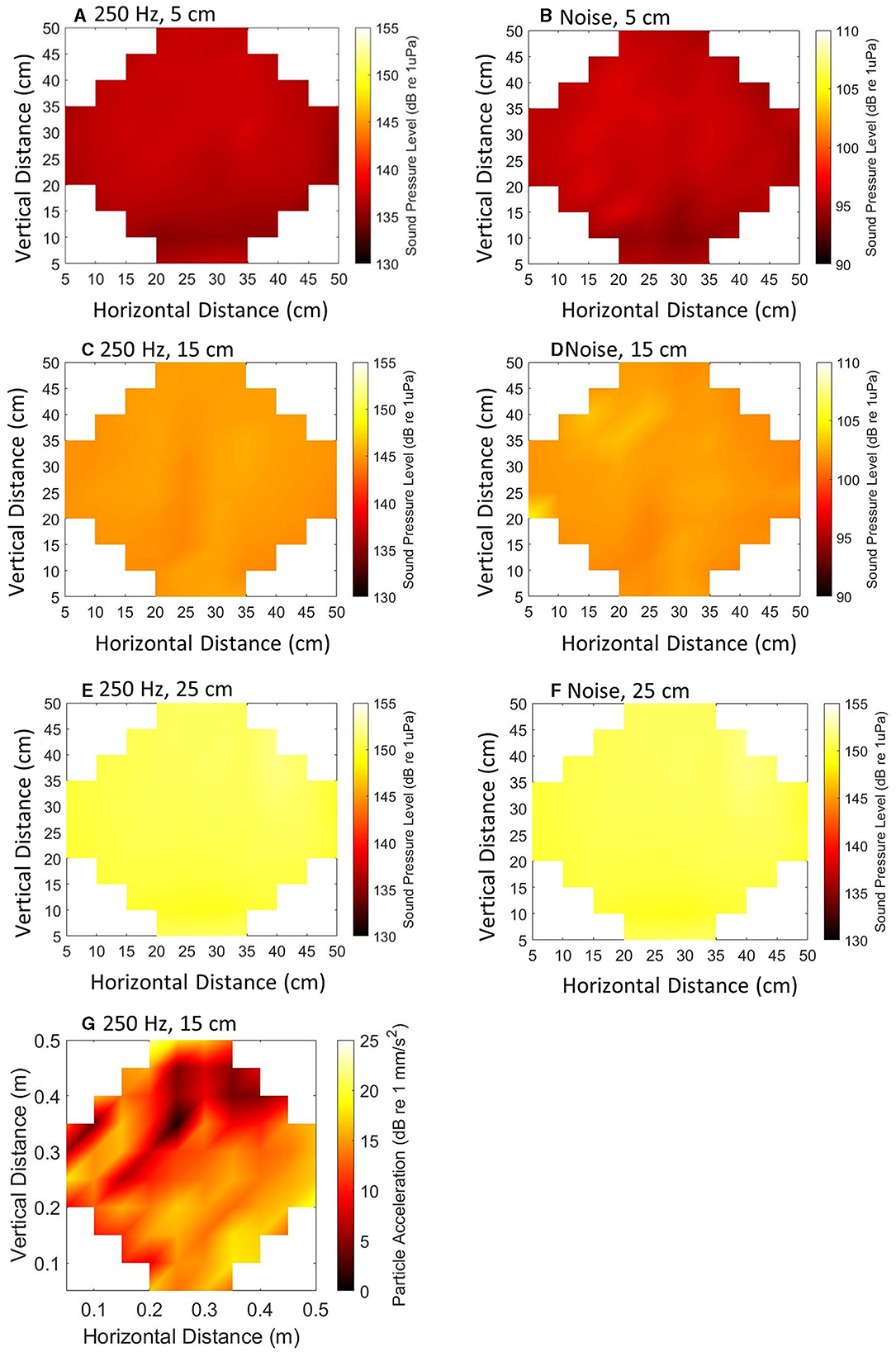
Figure 2. Maps illustrating Sound Pressure Level (SPL) (A–F) and Particle Acceleration (PA) (G) of a 120 ms, 250 Hz stimulus (A, C, E, G) at 145 dB re 1 μPa in the center of the arena and 100–2500 Hz band-limited random noise (B, D, F) at 110 dB re 1 μPa in the center of the arena. Point measurements were taken at three depths (5, 15, and 25 cm measured from the water surface).
The pressure gradient was calculated using the measurements of the pressure signal. The root mean square (RMS) of the pressure difference was calculated independently in three directions (x, y, and z). The pressure gradient was obtained as the difference in sound pressure between measurement points. The RMS particle acceleration, in each direction, was calculated as the pressure gradient (dP/dx in Pa m−1) and water density (kg m−3). The total RMS particle acceleration was obtained by combining the values in all three directions, with the results expressed in decibels (dB re 1 mm s−2). Subsequently, the PA was represented in maps (Figure 2).
The measured ambient sound was on average < 88 dB re 1 μPa, which was the electrical noise floor of the measurement system used (TC4032, manufacturer-calibrated sensitivity −170 dB re 1 V/μPa; Teledyne Reson, USA). The SPL was uniform across the horizontal plane and the pure tone SPL differed by ≈14 dB between the top and the bottom of the tank, whilst the noise differed vertically by ≈ 8.8 dB (Table 1). This provided an area of refuge for the fish, following the 3Rs guidance (Prescott and Lidster, 2017).

Table 1. The mean ± standard deviation of the 145 dB re 1 μPa, 250 Hz tone and 105 dB re 1 μPa, 100–2500 Hz band-limited random noise across a cylindrical experimental tank (0.555 m diameter) taken at 3 different depths (5, 15, and 25 cm).
Analysis
Recordings of fish behavior obtained during each trial were reviewed blind and in a random order. Presence or absence of a startle response, defined as a change in body tortuosity with erratic swimming such as a sudden increase in speed or a change in direction (Kastelein et al., 2008), were recorded for each trial. This did not include any freezing behavior and the startle responses were not categorized as C or S startles due to limitations with the recording devices. Whilst there are a number of avoidance behaviors such as C-starts, S-starts, single bends and double bends (Domenici and Hale, 2019), this study focuses on the startle response in the broader context, i.e. using it as a proxy for an avoidance response.
All statistical analyses were performed in R (version 3.6.3: https://rstudio.com/). Logistic regression was performed using a generalized linear mixed model (GLMM) with a binomial error structure and a “logit” link function. Akaike's Information Criterion (AIC) was used to support each model by giving a parsimonious quantification of model fit (Spake et al., 2015). To determine whether external factors may have confounded the results by influencing the probability of startling, a reductive model was developed. Factors included in the model were: tank days (minimum number of days in the husbandry tank); time of day (relative the beginning of the trial to the nearest hour); difference between holding and experimental tank temperature (°C); and mass (g). The initial GLMM contained all predictor variables with exposure (order of stimulus exposure), trial, and “exposure:trial” included as random effects. Manual backwards selection using variable significance (significance at p < 0.05) was undertaken as model simplification (Table 2). Trial was considered to be a random effect since the model produced the lowest AIC. Exposure (the nth stimulus played, 1–4) was included in a GLMM as a fixed effect alongside the other external factors, however, none were recognized to predict startle responses and the null model had the optimum AIC (Table 3). Since there was no effect of tolerance (exposure 1–4), the data were combined and treated as a single set.
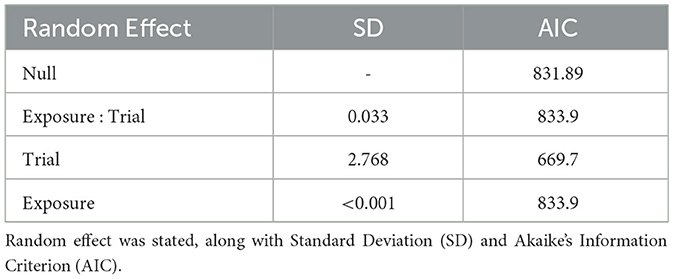
Table 2. Random effects included in generalized linear mixed models developed to determine if external factors affect the presence of a startle response to 120 ms pure tones (treatments of 250, 400, 600, 800, 1000, 2000 Hz and 115, 125, 135, 145 dB re 1 μPa) in goldfish.
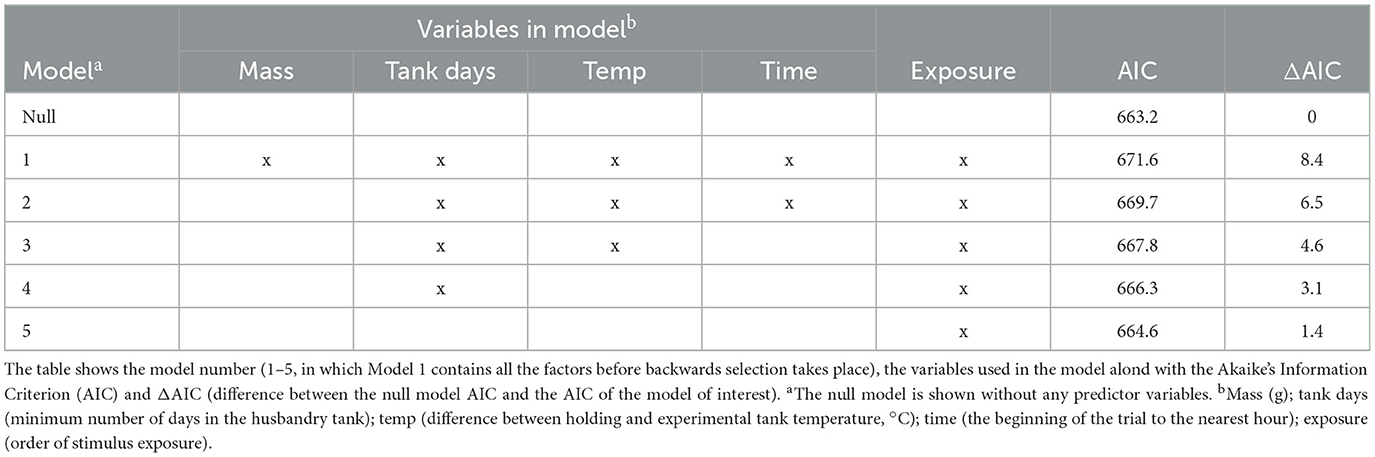
Table 3. Variables included in general linear mixed models developed to determine if external factors affect the presence of a startle response to 250 Hz 120 ms pure tones (SPLs of 115, 125, 135, 145 dB re 1 μPa) in goldfish in two acoustic environments (ambient and 105 dB re 1 μPa, 100–2500 Hz band limited random noise).
Objective 1: influence of SPL on startle response within each noise condition (dose-response)
Logistic regression with binomial error structure was used to show the dose-response relationship. This determined the influence of SPL on the presence of a startle response for both the treatment and ambient control noise conditions. Dose-response curves were then represented on plots with SPL against probability of startle for each background noise condition and the significance of the relationship recorded.
Objective 2a: coarse-scale dose-response assessment of the influence of noise treatment on startle response
Logistic regression with binomial error structure was used to show the dose-response relationship for startle responses between the ambient control and the treatment noise conditions. The distributions were compared according to the null hypothesis that there was no difference between the startle responses in the ambient control and the treatment.
Objective 2b: fine-scale assessment using Signal Detection Theory
The startle responses in the 4 min pre-signal period for both the ambient control and treatment noise conditions were considered. In the pre-signal periods, there were some occasional external acoustic cues that were uncontrolled. These acoustic cues came from sources such as the slamming of a car door nearby. Additionally, in the pre-signal period there were startles from individuals in absence of any acoustic stimulus. Audio files viewed in MATLAB and video footage were used to identify whether the startling goldfish in the pre-signal period were responding to an Acoustic Cue (AC) that was identified (AC identified) or not (no AC).
Three GLMMs with Poisson error structure and a “log” link function determined whether the number of startles in the pre-signal periods to AC identified, no AC, and for both affected the response to the 120 ms pure tone signal. Similarly, the number of startles to AC identified, no AC, and for both cases were compared between noisy and quiet environments using a GLM with Poisson error structure. The GLM with Poisson error structure and a “log” link function determined that the number of startles in the pre-signal period did not affect the response to the 120 ms pure tone for any of the signal treatments (AC identified: z = −0.794–1.818, p = 0.069–0.783; no AC: z = −0.851–0.879, p = 0.169–0.996; both: z = −0.565–1.962, p = 0.050–0.838). Therefore, the number of startles in the quiet and noisy pre-signal periods were compared and tested using a GLM with Poisson error structure.
Evaluation of fine-scale behavior of individuals between the ambient control and treatment noise conditions in the 4 min pre-signal period was conducted using SDT. The advantage of using SDT to assess fish behavior is that it considers response bias, which is the tendency for the individual to react as if the signal is present even if it is absent (Kemp et al., 2012; Kerr and Kemp, 2018). If a peak in the audio file (AC identified) matched with a startle in the video footage, it was characterized as a “hit”; if there was a startle and no peak identified (no AC) it was deemed a “false alarm”; if there was a peak (AC identified) and no startle response it was a “miss” (Figure 3). A “non-response” was categorized as a 0.5 s interval in which there was neither an abnormal peak in the audio file nor a startle in the video footage. The SDT discriminability, d′, and response criterion, c′, are measures of how easily the stimulus is detected by an individual and the level that an internal response results in the exhibition of a behavioral response, respectively. The d′ and c′ are described as:
where ZH and ZFA are the standard deviation units (Z scores of the unit normal Gaussian distribution) of the probability of hit and false alarm assuming the data is normally distributed with similar variance (Kemp et al., 2012; Kerr and Kemp, 2018).
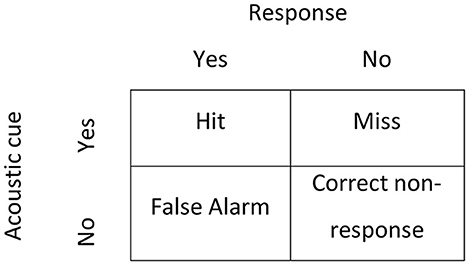
Figure 3. Four potential outcomes considered in Signal Detection Theory. In this study, the acoustic cue was either identified (yes) or not (no) in a 4 min control period in presence or absence of noise. The response was the presence (yes) or absence (no) of a startle response.
The discriminability and response criterion were calculated using the percentage of fish that responded or did not respond to an AC in each 4 min pre-signal period. If c′ is unbiased, it has a value of 0 and negative values of c′ signify a bias toward responding whereas positive values signify a bias toward not responding. Values of d′ of 1 and 2 are equivalent to one and two standard deviations, with higher values representing higher levels of signal discriminability. The discriminability and response criterion were calculated for all the pre-signal periods in the ambient control and the noise treatment and plotted on a Receiver Operating Characteristic (ROC) curve. Since there were four signal exposures per trial, it was possible to observe the responses in the pre-signal periods prior to each exposure, hence, gaining an idea of the responses over time.
Results
Objective 1: influence of SPL on startle response within each noise condition
Startle responses were observed at each of the SPLs in each of the background noise conditions. The proportion of goldfish that startled (Figure 4) increased with SPL in both the ambient control (z = 7.975, p ≤ 0.001) and treatment (z = 9.289, p ≤ 0.001).
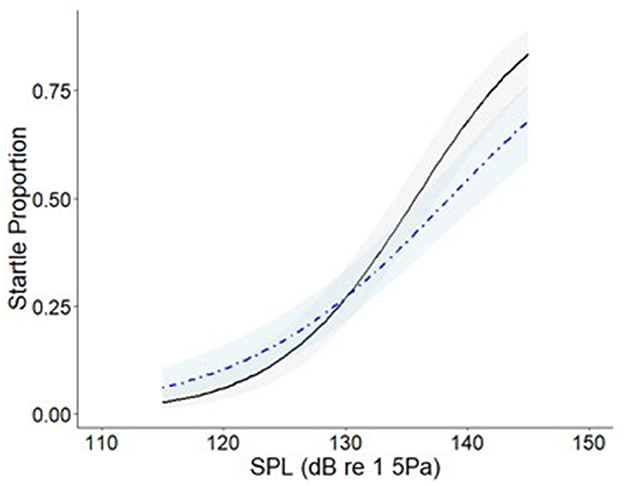
Figure 4. Logistic regression curves showing the proportion of goldfish (N = 140, with 20 replicates per treatment) that startled when exposed to a 120 ms, 250 Hz pure tone at 115, 125, 135, 145 dB re 1 μPa in two distinct acoustic environments: ambient control background noise (blue dot dash line) and (treatment) 105 dB re 1 μPa, 100–2500 Hz band-limited random noise (black solid line). The shaded regions indicate 95% confidence intervals.
Objective 2a: coarse-scale assessment of the influence of noise treatment on startle response
There was no difference in the proportion of the tested population exhibiting startle responses between the ambient control and the noise treatment (z = 0.996, p = 0.319). However, on visual inspection of the dose-response curves (Figure 4), a larger proportion of the population startled in the quiet compared to the noisy condition when the SPL of the signal was 115 dB re 1 μPa and 125 re 1 μPa. Conversely, when the signal was 135 dB re 1 μPa and 145 dB re 1 μPa, a larger proportion of startles occurred in the noisy treatment compared to the ambient control.
Objective 2b: fine-scale assessment using Signal Detection Theory
The number of startles in the pre-signal periods for both AC identified (z = −2.303, p = 0.021) and no AC (z = 7.717, p ≤ 0.001) differed between the ambient control and the background noise treatment.
Using the SDT analytical framework (Figure 5), there was a difference in d′ (t = −6.662, p ≤ 0.001) and c′ (t = 13.57, p ≤ 0.001) between the ambient control and noise treatment for all the pre-signal periods. The response criterion suggests that the individuals were more likely to respond in the noise treatment than the ambient control, irrespective of whether the individual was responding to AC identified or no AC. Similarly, d′ suggests that the goldfish were better able to discriminate external stimuli in the noise treatment than in the ambient control.
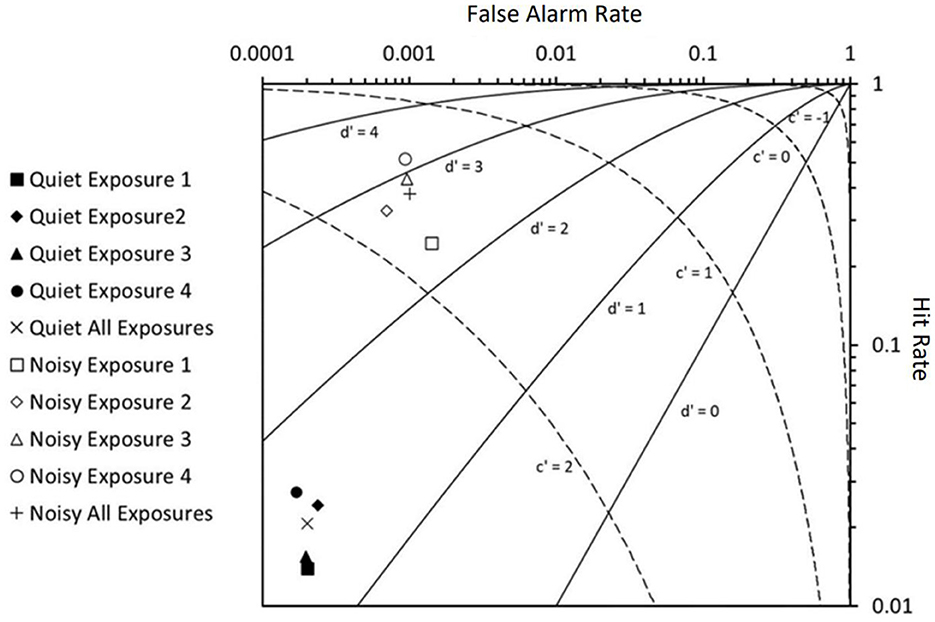
Figure 5. Reciever Operating Characteristic (ROC) curve calculated as part of the use of Signal Detection Theory as a framework to investigate the response of goldfish to identified acoustic cues and no acoustic cues in a control (quiet: ambient noise) and treatment noise (noisy: 105 dB re 1 μPa, 100–2500 Hz band-limited random noise) condition. Ten contexts were considered showing responses over time (exposures 1–4) and overall. Values are shown on a logarithmic scale.
For the startle responses in the ambient control, both d′ and c′ did not differ between the four pre-signal periods (the 4 min period before each of the four signal exposures). However, for those exhibited in the noise treatment, d′ increased and c′ decreased with each of the four pre-signal periods, i.e. over time (Table 4). Individual fish were also more likely to respond to AC identified over the duration of the trial in the noise treatment than in the ambient control. Hence, not only were fish better primed to respond in the presence of background noise, but this effect also increased over time.
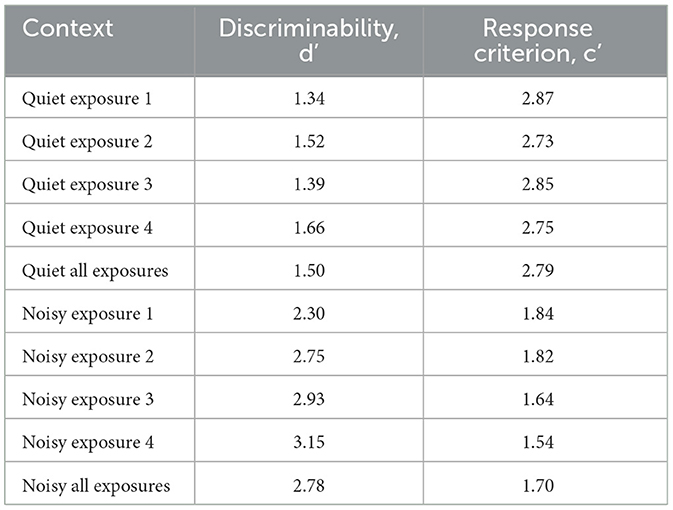
Table 4. Discriminability, d', and response criterion, c', calculated in an investigation to quantify the response of goldfish to identified acoustic cues and no acoustic cues in a control (quiet: ambient noise) and treatment noise (noisy: 105 dB re 1 μPa, 100–2500 Hz band-limited random noise) condition.
Discussion
This study explored the influence of background noise on the ability of fish to discern acoustic signals that may induce avoidance, here indicated by a startle response. Individual goldfish were exposed to four signals of differing SPLs (115, 125, 134, 145 dB re 1 uPa) either in the presence (treatment) or absence (ambient control) of band-limited random noise (105 dB re 1 μPa). The probability of exhibiting a startle response was positively related to the signal SPL. The background noise did not mask the signal to inhibit a response, as indicated by no difference in the probability of startling between the control and treatment. Consequently, fish were more likely to startle during the pre-signal period in the presence of the treatment background noise than during the equivalent period in the ambient control. Accounting for the internal status of the subject fish, possibly influenced by previous experience, motivation, or some other intrinsic characteristics, understanding the influence of background noise on their response to AC identified and no AC will help determine the effectiveness of acoustic deterrents.
The observation that the probability of startling increased with signal strength is consistent with the results of other studies (Currie et al., 2019; Holgate et al., 2023). Response to the signals, however, did not differ between the background noise treatment and the ambient control. This suggests that the band-limited random noise presented was below some threshold level required for masking. Further work is needed to determine the relationship between the SNR and an avoidance response in the subject species, particularly since other studies have demonstrated that fish may exhibit avoidance behaviors more frequently in noisy environments (Purser and Radford, 2011; Voellmy et al., 2014; Shafiei Sabet et al., 2015), as previously mentioned. Certainly, the effects of noise on fishes is a commonly reported phenomenon in a range of fish and can have negative impacts such as elevated stress (Schreck et al., 2016; Guh et al., 2021), inhibited communication (Radford et al., 2014; Pine et al., 2021) and hearing loss (Smith et al., 2022). From the perspective of developing an acoustic deterrent, understanding the role of SNR in eliciting a behavioral response is important in setting design criteria and recommendations for deployment, reducing the probability of variable results depending on site-specific contexts.
Using SDT as a framework that considers the internal status of the fish, we observed a difference in the fine-scale response between the treatment and control background noise when considering the presence (AC identified) and absence (no AC) of ACs during the pre-signal period. In this study, the coarse-scale analysis suggested no difference in response between treatment and control. However, when using the fine-scale approach to quantifying the fish response using SDT, rather than simply using a binary score or response/non-response to the stimulus, additional information of value was provided. The discriminability, d′, and response criterion, c′, indicated that the individuals in the background noise treatment were, respectively, more able to discern the signal and were more responsive (i.e. likely to exhibit either a hit or false alarm) than those in the ambient control. While the reason for this is unclear, there may be several explanations. First, the AC identified was not controlled for, meaning the data varied in frequency and SPL. Sounds with varied amplitude and frequencies have been found to deter fishes to a greater degree than the regular equivalent (Jong et al., 2020). Second, AC identified occurred randomly throughout the pre-signal period rather than at some pre-determined and consistent interval, potentially increasing the probability of eliciting a response (e.g., sounds with irregular pulse intervals increased the frequency of startle responses in zebrafish, Danio rerio, more than those with regular pulse intervals; Shafiei Sabet et al., 2015). Finally, it is possible that the noisy environment may have been more likely to cause startling in the subject fish, as suggested by the SDT analysis, resulting in a heightened response in those individuals. Similar observations have been reported for European minnow (Danio rerio) and three-spined stickleback (Gasterosteus aculeatus), where startles were elicited more frequently during playback of noise than during ambient conditions (Purser and Radford, 2011; Voellmy et al., 2014). In such conditions, the influence of priming, and other related phenomena, on the modification of fish behavior in the development of behavioral deterrents may provide an interesting and useful avenue of future research.
Focusing on the wider implications of this study, the development of acoustic fish deterrents should account for the influence of background noise conditions that are likely to vary with site-specific characteristics. The influence of background noise may provide an explanation, at least in part, for why the response of fish to acoustic cues may vary between studies. Further work is needed to ascertain the role that SNR may play in modifying fish response, and potentially enhancing it. As such, multiple background noise levels per signal SPL could be played to establish a relationship between SNR and behavioral response. Furthermore, this study demonstrated the value of using finer-scale analysis, such as that provided by SDT, to account for the internal status of the subject fish when investigating the response to stimuli of interest, an element that is seldom considered in most other studies. It is recommended that for future experiments, other behaviors may be quantified, and that the exact position of the fish could be measured using stereo cameras such that the exact received level and swimming depth can be calculated. We also recommend longer term studies considering habituation effects on individual behavior. Finally, the laboratory study here should not be used to directly inform acoustic deterrents in situ; however, the results obtained can be used to aid field studies testing similar objectives.
Data availability statement
The original contributions presented in the study are included in the article/supplementary material, further inquiries can be directed to the corresponding author.
Ethics statement
The animal study was approved by the University of Southampton Ethics and Research Governance Committee. The study was conducted in accordance with the local legislation and institutional requirements.
Author contributions
AH: Data collection and analyzing, Writing—original draft, Writing—review & editing. PW: Writing—review & editing. TL: Writing—review & editing. PK: Writing—review & editing.
Funding
The author(s) declare financial support was received for the research, authorship, and/or publication of this article. This study was funded by the EPSRC, University of Southampton and the Center for Doctoral Training (CDT-SIS).
Acknowledgments
The authors thank Hampshire Carp Hatcheries for supplying the goldfish, members of ICER and the University of Southampton who provided cover. Additional thanks to EPSRC, University of Southampton and the Center for Doctoral Training (CDT-SIS) for funding this study. Data supporting this study are openly available from the University of Southampton repository (University of Southampton, 2020) at: https://doi.org/10.5258/SOTON/D2820.
Conflict of interest
The authors declare that the research was conducted in the absence of any commercial or financial relationships that could be construed as a potential conflict of interest.
The author(s) declared that they were an editorial board member of Frontiers, at the time of submission. This had no impact on the peer review process and the final decision.
Publisher's note
All claims expressed in this article are solely those of the authors and do not necessarily represent those of their affiliated organizations, or those of the publisher, the editors and the reviewers. Any product that may be evaluated in this article, or claim that may be made by its manufacturer, is not guaranteed or endorsed by the publisher.
References
Baumgartner, L., and Boys, C. (2012). Reducing the perversion of diversion: applying world-standard fish screening practices to the Murray-Darling Basin. Ecol. Manag. Restor. 13, 135–143. doi: 10.1111/j.1442-8903.2012.00655.x
Bayramoglu, B., Chakir, R., and Lungarska, A. (2019). Impacts of land use and climate change on freshwater ecosystems in France. Environ. Model. Assess. 25, 147–172. doi: 10.1007/s10666-019-09673-x
Birkenhead, J., Radford, F., Stead, J., Cundy, A., and Hudson, M. (2020). Validation of a method to quantify microfibres present in aquatic surface microlayers. Sci. Rep. 10, 635. doi: 10.1038/s41598-020-74635-3
Black, J., and Perry, E. (2014). Laboratory evaluation of the survival of fish impinged on modified traveling water screens. N. Am. J. Fish. Manag. 34, 359–372. doi: 10.1080/02755947.2013.862193
Bzonek, P. A., Van Nynatten, A., and Mandrak, N. E. (2021). Phylogenetic signal found in fish–community response to an acoustic common carp deterrent. Freshw. Biol. 66, 1698–1708. doi: 10.1111/fwb.13785
Currie, H., White, P. R., Leighton, T. G., and Kemp, P. S. (2021). Collective behaviour of the European minnow (Phoxinus phoxinus) is influenced by signals of differing acoustic complexity. Behav. Process. 189, 104416. doi: 10.1016/j.beproc.2021.104416
Currie, H. A., White, P. R., Leighton, T. G., and Kemp, P. S. (2020). Group behavior and tolerance of Eurasian minnow (phoxinus phoxinus) in response to tones of differing pulse repetition rate. J. Acoust. Soc. Am., 147, 1709–1718. doi: 10.1121/10.0000910
Currie, H. L., White, P., Leighton, T., and Kemp, P. S. (2019). Group behavioral responses of juvenile common carp (Cyprinus carpio) to pulsed tonal stimuli in the presence of masking noise. Proc. Meet. Acoust. 37, 1180. doi: 10.1121/2.0001180
Daniels, J., and Kemp, P. S. (2022). Personality-dependent passage behaviour of an aquatic invasive species at a barrier to dispersal. Anim. Behav. 192, 63–74. doi: 10.1016/j.anbehav.2022.07.005
Darwall, W., Bremerich, V., De Wever, A., Dell, A. I., Freyhof, J., Gessner, M. O., et al. (2018). The alliance for freshwater life: a global call to unite efforts for freshwater biodiversity science and conservation. Aquat. Conserv. Mar. Freshw. Ecosyst. 28, 1015–1022. doi: 10.1002/aqc.2958
Deleau, M., White, P., Peirson, G., Leighton, T., and Kemp, P. (2019). Use of acoustics to enhance the efficiency of physical screens designed to protect downstream moving European eel (Anguilla anguilla). Fish. Manag. Ecol. 27, 1–9. doi: 10.1111/fme.12362
Deleau, M., White, P., Peirson, G., Leighton, T., and Kemp, P. (2020). The response of anguilliform fish to underwater sound under an experimental setting. River Res. Appl. 36, 441–451. doi: 10.1002/rra.3583
Domenici, P., and Hale, M. E. (2019). Escape responses of fish: a review of the diversity in motor control, kinematics and behaviour. J. Exp. Biol. 222, 166009. doi: 10.1242/jeb.166009
Eaton, R. C., Bombardieri, R. A., and Meyer, D. L. (1977). The Mauthner-initiated startle response in teleost fish. J. Exp. Biol. 6, 65–81. doi: 10.1242/jeb.66.1.65
Fay, R. (1984). The goldfish ear codes the axis of acoustic particle motion in three dimensions. Science 225, 951–954. doi: 10.1126/science.6474161
Flores-Martin, N., Leighton, T. G., White, P. R., and Kemp, P. S. (2021). The response of common carp (Cyprinus carpio) to insonified bubble curtains. J. Acoust. Soc. Am. 150, 3874–3888. doi: 10.1121/10.0006972
Guh, Y., Tseng, Y., and Shao, Y. (2021). To cope with a changing aquatic soundscape: Neuroendocrine and antioxidant responses to chronic noise stress in fish. Gen. Comp. Endocrinol. 314, 113918. doi: 10.1016/j.ygcen.2021.113918
Holgate, A., White, P. R., Leighton, T. G., and Kemp, P. S. (2023). Applying appropriate frequency criteria to advance acoustic behavioural guidance systems for fish. Sci. Rep. 13, 423. doi: 10.1038/s41598-023-33423-5
Jesus, J., Amorim, M., Fonseca, P., Teixeira, A., Natário, S., Carrola, J., et al. (2018). Acoustic barriers as an acoustic deterrent for native potamodromous migratory fish species. J. Fish Biol. 95, 247–255. doi: 10.1111/jfb.13769
Jong, D., Forland, K., Amorim, T. N., Rieucau, M. C. P., Slabbekoorn, G. H., and Sivle, L. D. (2020). Predicting the effects of anthropogenic noise on fish reproduction. Rev. Fish Biol. Fish. 30, 245–268. doi: 10.1007/s11160-020-09598-9
Kastelein, R., Heul, S., Verboom, W., Jennings, N., Veen, J., Haan, D., et al. (2008). Startle response of captive North Sea fish species to underwater tones between 0.1 and 64kHz. Mar. Environ. Res. 65, 369–377. doi: 10.1016/j.marenvres.2008.01.001
Kemp, P. S. (2015). “Impoundments, barriers and abstractions: Impact on fishes and fisheries, mitigation and future directions,” in Freshwater Fisheries Ecology, ed. J. F. Craig (New York, NY: Wiley), 717–769.
Kemp, P. S., Anderson, J. J., and Vowles, A. S. (2012). Quantifying behaviour of migratory fish: Application of signal detection theory to fisheries engineering. Ecol. Eng. 41, 22–31. doi: 10.1016/j.ecoleng.2011.12.013
Kenyon, T., Ladich, F., and Yan, H. (1998). A comparative study of hearing ability in fishes: the auditory brainstem response approach. J. Comp. Physiol. A Neuroethol. Sens. Neural Behav. Physiol. 182 307–318. doi: 10.1007/s003590050181
Kerr, J. R., and Kemp, P. S. (2018). Masking a fish's detection of environmental stimuli: Application to improving downstream migration at River Infrastructure. J. Fish Biol. 95, 228–237. doi: 10.1111/jfb.13812
Kim, J., and Mandrak, N. E. (2017). Effects of strobe lights on the behaviour of freshwater fishes. Environ. Biol. Fishes 100, 1427–1434. doi: 10.1007/s10641-017-0653-7
Kinsler, L. E., Frey, A. R., Coppens, A. B., and Sanders, J. V. (1982). Fundamentals of Acoustics. New York, NY: Wiley.
Ladich, F., and Fay, R. R. (2013). Auditory evoked potential audiometry in fish. Rev. Fish Biol. Fish. 23, 317–364. doi: 10.1007/s11160-012-9297-z
Le Pape, O., Bonhommeau, S., Nieblas, A. E., and Fromentin, J. M. (2017). Overfishing causes frequent fish population collapses but rare extinctions. PNAS 31, 114. doi: 10.1073/pnas.1706893114
Maes, J., Turnpenny, A. W. H., Lambert, D. R., Nedwell, J. R., Parmentier, A., and Ollevier, F. (2004). Field evaluation of a sound system to reduce estuarine fish intake rates at a power plant cooling water inlet. J. Fish Biol. 64, 938–946. doi: 10.1111/j.1095-8649.2004.00360.x
Mallen-Cooper, M., and Brand, D. (2007). Non-salmonids in a salmonid fishway: what do 50 years of data tell us about past and future fish passage? Fish. Manage. Ecol. 14, 319–332. doi: 10.1111/j.1365-2400.2007.00557.x
McLaughlin, R. L., Smyth, E. R., Castro-Santos, T., Jones, M. L., Koops, M. A., Pratt, T. C., et al. (2012). Unintended consequences and trade-offs of Fish Passage. Fish Fish. 14, 580–604. doi: 10.1111/faf.12003
Miller, M., De Bie, D., Sharkh, J. S., and Kemp, P. (2021). Behavioural response of downstream migrating European eel (Anguilla anguilla) to electric fields under static and flowing water conditions. Ecol. Eng. 172, 106397. doi: 10.1016/j.ecoleng.2021.106397
Miller, M., Sharkh, S. M., and Kemp, P. S. (2022). Response of upstream migrating juvenile European eel (Anguilla Anguilla) to electric fields: application of the marginal gains concept to fish screening. PLoS ONE 17, e0270573. doi: 10.1371/journal.pone.0270573
Munday, P. L., Cheal, A. J., Dixson, D. L., and Rummer, J. L. (2014). Behavioural impairment in reef fishes caused by ocean acidification at CO2 seeps. Nat. Clim. Change 4, 487–492. doi: 10.1038/nclimate2195
Noonan, M., Grant, J., and Jackson, C. (2011). A quantitative assessment of fish passage efficiency. Fish Fish. 13, 450–464. doi: 10.1111/j.1467-2979.2011.00445.x
Pine, M. K., Wilson, L., Jeffs, A. G., McWhinnie, L., Juanes, F., Scuderi, A., et al. (2021). A Gulf in lockdown: how an enforced ban on recreational vessels increased dolphin and fish communication ranges. Glob. Change Biol. 27, 4839–4848. doi: 10.1111/gcb.15798
Piper, A. T., White, P. R., Wright, R. M., Leighton, T. G., and Kemp, P. S. (2019). Response of seaward-migrating European eel (Anguilla anguilla) to an infrasound deterrent. Ecol. Eng. 127, 480–486. doi: 10.1016/j.ecoleng.2018.12.001
Plagányi, E. (2019). Climate change impacts on fisheries. Science 363, 930–931. doi: 10.1126/science.aaw5824
Poff, N., Olden, J., and Strayer, D. (2012). “Climate change and freshwater fauna extinction risk,” in Saving a Million Species, ed L. Hannah (London: Island Press/Center for Resource Economics).
Popper, A., and Clarke, N. (1976). The auditory system of the goldfish (Carassius auratus): effects of intense acoustic stimulation. Comp. Biochem. Physiol. 53, 11–18. doi: 10.1016/S0300-9629(76)80003-5
Prescott, M. J., and Lidster, K. (2017). Improving quality of science through better animal welfare: the NC3Rs strategy. Lab Anim. 46, 152–156. doi: 10.1038/laban.1217
Purser, J., and Radford, A. (2011). Acoustic noise induces attention shifts and reduces foraging performance in three-spined sticklebacks (Gasterosteus aculeatus). PloS ONE 6, e17478. doi: 10.1371/journal.pone.0017478
Putland, R., and Mensinger, A. (2019). Acoustic deterrents to manage fish populations. Rev. Fish Biol. Fish. 29, 789–807. doi: 10.1007/s11160-019-09583-x
Radford, A. N., Kerridge, E., and Simpson, S. D. (2014). Acoustic communication in a noisy world: can fish compete with anthropogenic noise? Behav. Ecol. 25, 1022–1030. doi: 10.1093/beheco/aru029
Reid, A. J., Carlson, A. K., Creed, I. F., Eliason, E. J., Gell, P. A., Johnson, P. T., et al. (2018). Emerging threats and persistent conservation challenges for freshwater biodiversity. Biol. Rev. 94, 849–873. doi: 10.1111/brv.12480
Roscoe, D., and Hinch, S. (2010). Effectiveness monitoring of fish passage facilities: Historical trends, geographic patterns and future directions. Fish Fish. 11, 12–33. doi: 10.1111/j.1467-2979.2009.00333.x
Schreck, C. B., Tort, L., Farrell, A., and Brauner, C. (2016). Biology of stress in Fish. Cambridge, MA: Academic Press.
Shafiei Sabet, S., Neo, Y. Y., and Slabbekoorn, H. (2015). The effect of temporal variation in sound exposure on swimming and foraging behaviour of captive zebrafish. Anim. Behav. 107, 49–60. doi: 10.1016/j.anbehav.2015.05.022
Smith, M. E., Accomando, A. W., Bowman, V., Casper, B. M., Dahl, P. H., Jenkins, A. K., et al. (2022). Physical effects of sound exposure from underwater explosions on Pacific mackerel (Scomber japonicus): effects on the inner ear. J. Acoust. Soc. Am. 152, 733–744. doi: 10.1121/10.0012991
Spake, R., Ezard, T., Martin, P., Newton, A., and Doncaster, C. (2015). A meta-analysis of functional group responses to forest recovery outside of the tropics. Conserv. Biol. 29, 1695–1703. doi: 10.1111/cobi.12548
Swanson, C., Young, P. S., and Cech, J. J. (2005). Close encounters with a fish screen: Integrating physiologicaland behavioral results to protect endangered species inexploited ecosystems. Trans. Am. Fish. Soc. 134, 1111–1123. doi: 10.1577/T04-121.1
Turnpenny, A., and Nedwell, J. (2003). “Development and operation of acoustic fish deterrent systems at estuarine power stations,” in Symposium on Cooling Water Intake Technologies to Protect Aquatic Organisms, Arlington, VI, USA, 6-7 May 2003, 187–200.
Vetter, B. J., Cupp, A. R., Fredricks, K. T., Gailowski, M. P., and Mesinger, A. F. (2015). Acoustical deterrence of silver carp (Hypophthalmichthys molitrix). Biol. Invasions. 17, 3383–3392. doi: 10.1007/s10530-015-0964-6
Voellmy, I., Purser, J., Flynn, D., Kennedy, P., Simpson, S., Radford, A., et al. (2014). Acoustic noise reduces foraging success in two sympatric fish species via different mechanisms. Anim. Behav. 89, 191–198. doi: 10.1016/j.anbehav.2013.12.029
Vowles, A., and Kemp, P. (2021). Artificial light at night (ALAN) affects the downstream movement behaviour of the critically endangered European eel, Anguilla anguilla. Environ. Pollut. 274, 116585. doi: 10.1016/j.envpol.2021.116585
Wamboldt, J. (2019). Evaluation of an acoustic fish deterrent system in shallow water application at the Emiquon Preserve, Lewistown, IL. Manag. Biol. Invasions 10, 536–558. doi: 10.3391/mbi.2019.10.3.09
Wilkes, M. A., Webb, J. A., Pompeu, P. S., Silva, L. G., Vowles, A. S., Baker, C. F., et al. (2018). Not just a migration problem: metapopulations, habitat shifts, and gene flow are also important for fishway science and management. River Res. Appl. 35, 1688–1696. doi: 10.1002/rra.3320
Keywords: dose-response, acoustics, fish, acoustic deterrents, fish conservation, bioacoustics, Signal Detection Theory
Citation: Holgate A, White PR, Leighton TG and Kemp P (2024) Adopting a reductionist approach to advance acoustic deterrents in fish conservation. Front. Freshw. Sci. 2:1320582. doi: 10.3389/ffwsc.2024.1320582
Received: 12 October 2023; Accepted: 09 February 2024;
Published: 07 March 2024.
Edited by:
Leandro E. Miranda, US Geological Survey and Mississippi State University, United StatesReviewed by:
Marybeth Brey, United States Geological Survey, United StatesAdam Brysiewicz, Institute of Technology and Life Sciences, Poland
Allen Mensinger, University of Minnesota Duluth, United States
Copyright © 2024 Holgate, White, Leighton and Kemp. This is an open-access article distributed under the terms of the Creative Commons Attribution License (CC BY). The use, distribution or reproduction in other forums is permitted, provided the original author(s) and the copyright owner(s) are credited and that the original publication in this journal is cited, in accordance with accepted academic practice. No use, distribution or reproduction is permitted which does not comply with these terms.
*Correspondence: Paul Kemp, cC5rZW1wQHNvdG9uLmFjLnVr