- 1Department of Agroecology and Crop Production, Faculty of Agrobiology, Food and Natural Resources, Czech University of Life Sciences Prague, Prague, Czechia
- 2Department of Biotechnology, The University of Burdwan, Bardhaman, West Bengal, India
- 3Department of Economic Theories, Faculty of Economics and Management, Czech University of Life Sciences Prague, Prague, Czechia
Climate change is severely impacting global forest ecosystems, stressing woody plants due to rising temperatures, shifting precipitation patterns, and extreme weather events. These pressures threaten biodiversity and disrupt the essential roles forests play in carbon sequestration, timber production, and ecosystem stability. Traditional forest management strategies, such as selective breeding, cannot keep up with the rapid pace of climate change, given the long juvenile phase of trees. Multiplex genome editing, particularly through CRISPR technologies, offers a promising solution to accelerate the development of climate-resilient traits in woody plants. By simultaneously targeting multiple genes, multiplex CRISPR enables efficient modification of polygenic traits that govern stress tolerance, disease resistance, and other crucial resilience factors. This mini-review examines the potential of multiplex CRISPR technologies in forest management, breeding, and agroecological practices, showing how they can improve tree resilience and support sustainable forestry in response to the growing challenges of climate change.
1 Introduction
Climate change is one of the biggest threats to global biodiversity and the health of ecosystems, particularly forests (Malhi et al., 2020). Trees, which provide essential services such as carbon sequestration, water cycle maintenance, and biodiversity conservation, are particularly vulnerable to the changing climate. Species that have evolved over millennia to thrive in specific climatic conditions are now facing unprecedented challenges in maintaining their growth, health, and productivity. Forest ecosystems are under increasing stress due to rising temperatures, shifting precipitation patterns, more frequent extreme weather events, and the spread of pests and diseases. This disruption not only threatens the survival of individual tree species but also jeopardizes the broader ecosystem services that forests provide to humanity. For example, trees’ roles in mitigating climate change through carbon storage could be diminished if they fail to adapt to new climatic conditions (Keenan, 2015; McDowell et al., 2022; Xu and Prescott, 2024).
While traditional forest management strategies, such as assisted migration and selective breeding, are being employed to help trees cope with climate change, these approaches have inherent limitations. Assisted migration, for instance, is hindered by slow natural adaptation processes and habitat fragmentation, which restricts the movement of species to more suitable environments (Charles and Stehlik, 2021). Selective breeding, despite its potential to enhance tree resilience and productivity, is constrained by several inherent challenges specific to woody perennials. These challenges stem from their outcrossing reproductive systems, extended juvenile phases, and massive genome sizes, which collectively slow down the breeding process and limit the efficiency of trait selection. Furthermore, predicting the future environmental conditions to which trees must adapt, such as the impacts of climate change, shifting pest populations, and fluctuating soil qualities, adds another layer of complexity (Cortés et al., 2020). Furthermore, these approaches may lead to genetic bottlenecks, reducing the genetic diversity needed for long-term adaptation. As a result, there is a growing recognition that more rapid and effective strategies are needed to enhance the resilience of trees to climate change (Cantarello et al., 2024).
In this context, the development of novel biotechnological tools, such as multiplex CRISPR genome editing, holds great promise for enhancing tree resilience (Ma and Liu, 2016; Cao et al., 2018; McCarty et al., 2020; Singh et al., 2024; Yao et al., 2024). CRISPR, a genome-editing technology derived from bacterial immune systems, employs programmable RNA molecules to accurately target and modify specific DNA sequences, enabling the correction, deletion, or addition of genetic material. When used with multiplexing strategies, CRISPR can simultaneously target multiple genes, significantly accelerating the development of resilience traits such as drought tolerance, pest resistance, and heat stress adaptation (McCarty et al., 2020). Traditional breeding methods, while foundational in forestry, often fail to keep pace with the urgent need for trees to adapt to escalating climate challenges, such as prolonged droughts, rising temperatures, and increased pest pressures. These methods are constrained by the long generation times and genetic complexity of trees, limiting their ability to address such specific environmental threats swiftly. In contrast, CRISPR offers a targeted and efficient solution, enabling precise edits to key genes that govern resilience traits like drought tolerance, heat resistance, and pest defense, thereby accelerating the development of climate-resilient tree species (Min et al., 2022; Singh et al., 2022; Bahariah et al., 2023).
Given the accelerating pace of climate change, it is imperative to harness the potential of multiplex genome editing to develop climate-resilient forests. These tools not only offer a faster pathway to address the complexities of polygenic traits but also hold the promise of fundamentally transforming forest management and agroecological practices. This review aims to explore the latest advancements in multiplex CRISPR genome editing for trees, assess the scientific and technical challenges, highlight case studies from other species, and examine the broader implications of these technologies for sustainable forestry.
2 Multiplex genome editing: unlocking polygenic traits for climate-resilient forestry
Early genome editing focused on altering one gene at a time, which worked well for single-gene traits. However, many traits essential for climate resilience, such as stress tolerance and disease resistance, are controlled by multiple genes. These polygenic traits are influenced by complex interactions between genetic factors, making them hard to modify with single-gene approaches (Healy et al., 2018). Multiplex genome editing enables the simultaneous modification of multiple genes using advanced tools like CRISPR-Cas9, CRISPR-Cas12, or CRISPR-Cas13 (Armario Najera et al., 2019; Hillary and Ceasar, 2023). This approach is particularly advantageous for addressing complex traits, such as resilience, which are typically governed by multiple genes working together. This is because genes involved in resilience often have small effects, meaning changing just one gene may not have a noticeable impact. Additionally, genes interact with each other through epistasis (where one gene can affect the expression of another gene), and their expression can vary depending on the environment (Doust et al., 2014). Some genes might show stronger effects under drought, while others may be more active during temperature stresses. Examples of such complex gene interactions involved in tree resilience include a study on poplar, where overlapping heat- and drought-responsive genes form hierarchical co-expression networks in roots and leaves. In leaves, ERF1 and HSFA2 regulated heat-responsive subnetworks, while drought-responsive networks identified hub genes like RD26 and NST1, crucial for RNA regulation and hormone metabolism (Jia et al., 2017). These findings highlight the importance of tightly coordinated gene networks in managing complex stress responses, revealing shared and distinct regulatory mechanisms across drought and temperature stresses, interactions that add a layer of complexity beyond the reach of traditional breeding or single-gene edits.
Multiplex genome editing offers a powerful solution to the challenges posed by gene interactions and environmental factors, significantly accelerating the development of climate-resilient trees. In conventional breeding, the more target genes there are, the more crosses are required, leading to longer timeframes and a higher likelihood of introducing unintended genetic backgrounds, an especially impractical approach for long-lived tree species. By enabling the simultaneous modification of multiple genes that collectively contribute to desired traits, genome editing not only reduces these challenges but also enhances the precision and efficiency of achieving meaningful and durable genetic improvements. This precision is particularly critical in addressing the complex and multifaceted environmental stresses exacerbated by climate change, such as increased temperatures, prolonged droughts, and evolving pest pressures (Armario Najera et al., 2019; Wolter et al., 2019; Abdelrahman et al., 2021; Hassan et al., 2021). Below, we will explore some examples of polygenic traits relevant to forestry. Although successful applications of multiplex genome editing in forestry (multigenic traits) remain limited, we have included examples from other plant systems to highlight a few cases where the target traits are multigenic.
CRISPR/Cas technologies, especially CRISPR-Cas9, have transformed genetic engineering by offering a precise and efficient way to modify specific DNA sequences. For example, in the study by Wolabu et al. (2024), CRISPR/Cas9 was used for multiplex genome editing to reduce lignin content in alfalfa by targeting the COUMARATE 3-HYDROXYLASE (MsC3H) gene. Four guide RNAs (gRNAs) were combined in a polycistronic system for multi-allele editing, generating 73 transgenic lines. Three homozygous MsC3H mutants showed reduced lignin content and improved forage quality, including better digestibility and nutrition. In another study, CRISPR/Cas9 enabled multiplex editing of seven closely linked Nucleoredoxin1 (NRX1) genes in Populus tremula × Populus alba. This was achieved using a single gRNA targeted the entire gene array, inducing diverse mutations such as small insertions, deletions, and large genomic rearrangements, including translocations and inversions. Target capture sequencing revealed a variety of repair outcomes and mutant alleles, demonstrating the versatility of CRISPR/Cas9 for simultaneously editing multiple genes and generating structural variations in tandem gene arrays (Chen Y. -H. et al., 2023). In a related study, multiplex editing targeted multiple genes in hybrid poplar (Populus tremula × P. alba). Researchers used a single gRNA to target a conserved site in MYB186 and its paralogs, MYB138 and MYB38, enabling simultaneous editing. This approach generated eight alleles, revealing a previously unrecognized role for non-glandular trichomes in triterpene production. Deep sequencing confirmed successful editing with no off-target effects. The CRISPR-Cas9 system, driven by the Medicago truncatula MtU6.6 promoter, was assembled using Gibson assembly and transformed via Agrobacterium-mediated methods, ensuring precise and efficient knockout mutants (Bewg et al., 2022). In another example, multiplex CRISPR/Cas9 editing was used to enhance lignin composition and wood properties in poplar trees for sustainable fiber production. From 69,123 strategies targeting 21 lignin biosynthesis genes, seven were selected, yielding 174 edited variants. These variants showed up to a 228% increase in the wood carbohydrate-to-lignin ratio, significantly improving pulping efficiency without affecting tree growth, highlighting the transformative potential of multiplex gene editing for complex traits in forestry (Sulis et al., 2023). Besides poplar trees, CRISPR/Cas9 has also been applied to apple trees for multiplex genome editing. Targeting the Phytoene Desaturase (PDS) and Terminal Flower 1 (TFL1) genes, researchers achieved high editing efficiency, with 85% of PDS-targeted lines showing albino phenotypes and 93% of TFL1-targeted lines exhibiting early flowering (Charrier et al., 2019). Sequencing revealed primarily insertion mutations with minimal off-target effects, and transient transformation produced T-DNA-free edited lines, highlighting CRISPR/Cas9 as a precise tool for crop improvement. A few other examples can be found in Pak et al. (2022). These advances also underscore the importance of promoter selection in optimizing editing efficiency. While Arabidopsis U6-26 promoters are commonly used in forest trees, species-specific promoters, such as the Pseudotsuga menziesii U6 promoter in pine and endogenous U6 promoters in Hevea brasiliensis, outperform generic promoters, achieving up to 67% efficiency (Poovaiah et al., 2021; Bruegmann et al., 2024). This highlights the advantage of endogenous promoters in enhancing genome editing outcomes (Fan et al., 2015; Bruegmann et al., 2024). Multiplex genome editing using CRISPR/Cas9 has proven effective in targeting polygenic traits like drought tolerance. For example, in wheat, simultaneous editing of five TaSal1 gene homologs using multiple sgRNAs led to plants with enhanced drought resilience traits, such as closed stomata and improved growth under stress conditions (Abdallah et al., 2025). CRISPR-Cas9 multiplex genome editing has also been successfully applied to target polygenic traits in tomatoes by precisely editing the tomato gene encoding hybrid proline-rich protein 1 (SlHyPRP1) gene. Functional domains, including the proline-rich domain (PRD) and eight cysteine-motif (8CM), were removed, resulting in multi-stress-tolerant variants. These edits improved salinity tolerance, heat resilience, drought endurance, and resistance to Pseudomonas syringae (Tran et al., 2023). More examples can be found in Prajapati and Tyagi (2024), highlighting the potential of multiplex editing to address complex, multigenic traits in crops.
CRISPR-Cas12 offers a significant advancement in multiplex genome editing, particularly with its ability to create staggered DNA cuts that enable more efficient edits, especially in larger genomic regions. The CRISPR/Cas12a system differs from CRISPR/Cas9 in several ways. Cas12a targets T-rich protospacer adjacent motif (PAM) sequences, such as 5′-TTTV-3′, located upstream of the protospacer sequence. Unlike Cas9, Cas12a is guided by a single crRNA, eliminating the need for a trans-acting crRNA. It also exhibits both DNA and RNA endonuclease activities. Furthermore, Cas12a contains a RuvC-like domain that cleaves the DNA 18–23 base pairs downstream of the PAM, creating a 5-base pair sticky end. These differences result in Cas12a inducing mutations with higher efficiency than Cas9. By generating staggered double-strand breaks away from the seed region, Cas12a promotes repeated cleavage and extensive end processing, which enhances the effectiveness of CRISPR/Cpf1 homology-directed repair (HDR)-mediated mutations. This capability is crucial for modifying genes associated with complex traits, enabling more robust genetic improvements in trees (Yan et al., 2019; Bandyopadhyay et al., 2020; Senthilnathan et al., 2023; Zhang et al., 2024). For example, CRISPR/Cas12a has shown great potential for multiplex genome editing in poplar (Populus alba × Populus glandulosa). Researchers tested three Cas12a nucleases—AsCas12a, LbCas12a, and FnCas12a—targeting the phytoene desaturase gene for multiple knockouts. AsCas12a was the most efficient, achieving a 70% mutation rate. This system enabled large-fragment deletions, broadening the possibilities for genetic modifications in forest trees. Increasing the co-cultivation temperature during Agrobacterium-mediated transformation from 22°C to 28°C further improved editing efficiency, showcasing CRISPR/Cas12a’s versatility in advancing tree biotechnology (An et al., 2020).
In addition to Cas9 and Cas12, CRISPR/Cas13 provides another powerful tool for multiplex genome editing, expanding the possibilities for genetic modification. While Cas12 targets DNA, Cas13 is unique in that it targets RNA, offering distinct advantages for precision editing without altering the DNA sequence itself. The Cas13 protein contains two higher eukaryotes and prokaryotes nucleotide-binding (HEPN) domains that facilitate the processing of pre-crRNA into functional crRNA and the cleavage of target RNA, enabling precise RNA-guided activity. These HEPN domains are located at different positions depending on the Cas13 subtype, contributing to its functional diversity. Cas13 is classified into six subtypes: VI-A (Cas13a, C2c2), VI-B (Cas13b, C2c4), VI-C (Cas13c, C2c7), VI-D (Cas13d), and the recently identified VI-X (Cas13X) and VI-Y (Cas13Y). Among these, Cas13d stands out for its compact size and efficient activity, making it particularly suitable for precise RNA editing. Additionally, Cas13 exhibits collateral cleavage activity, degrading non-target RNA near the complex, which can be exploited for applications such as RNA detection. This RNA-targeting capability allows Cas13 to dynamically regulate gene expression, modulate RNA splicing, and knock down harmful transcripts without altering the DNA sequence. Its reversible and finely tuned functionality, combined with its classification diversity, makes Cas13 a valuable tool for tackling complex challenges in plant genome editing and adapting to environmental changes (Yan et al., 2019; Kavuri et al., 2022).
Cas13 has been successfully utilized in various applications, such as controlling viral infections and modulating gene expression in plant systems (Mahas et al., 2019; Sharma et al., 2022; Yu et al., 2022; Ribeiro et al., 2024). Its ability to manipulate RNA makes it an ideal candidate for fine-tuning gene expression in forest trees, allowing for precise control over traits like stress tolerance, disease resistance, and growth regulation. Since Cas13 operates at the RNA level, it does not involve direct modifications to the genome, distinguishing it from traditional genome editing approaches. However, because Cas13 targets RNA, the changes are temporary unless maintained continuously. This means organisms treated with Cas13 are not considered GMOs unless the Cas13 gene is integrated into their genome. By pairing Cas13 with multiplexing strategies, researchers can simultaneously target and modify multiple RNA molecules, enhancing the efficiency and versatility of genetic interventions in forest tree biotechnology. Nevertheless, challenges such as off-target effects, delivery efficiency in complex plant systems, and the potential for unintended impacts on non-target RNAs must be addressed to fully realize its potential.
The combination of Cas12 and Cas13 technologies, each with its distinct advantages, presents a powerful toolkit for advancing genetic research and improvement in complex organisms like forest trees. However, the use of CRISPR/Cas13 systems in forestry applications has yet to be explored, although it holds significant potential for forest trees. Similarly, CRISPR/Cas12 systems remain underexplored in this context. These approaches could offer valuable insights into the regulation of key traits in woody plants, such as stress tolerance, disease resistance, and growth (Figure 1A). By utilizing these systems for multiplex genome editing, scientists can accelerate the development of climate-resilient trees with enhanced traits, ultimately contributing to sustainable forest management and ecosystem health.
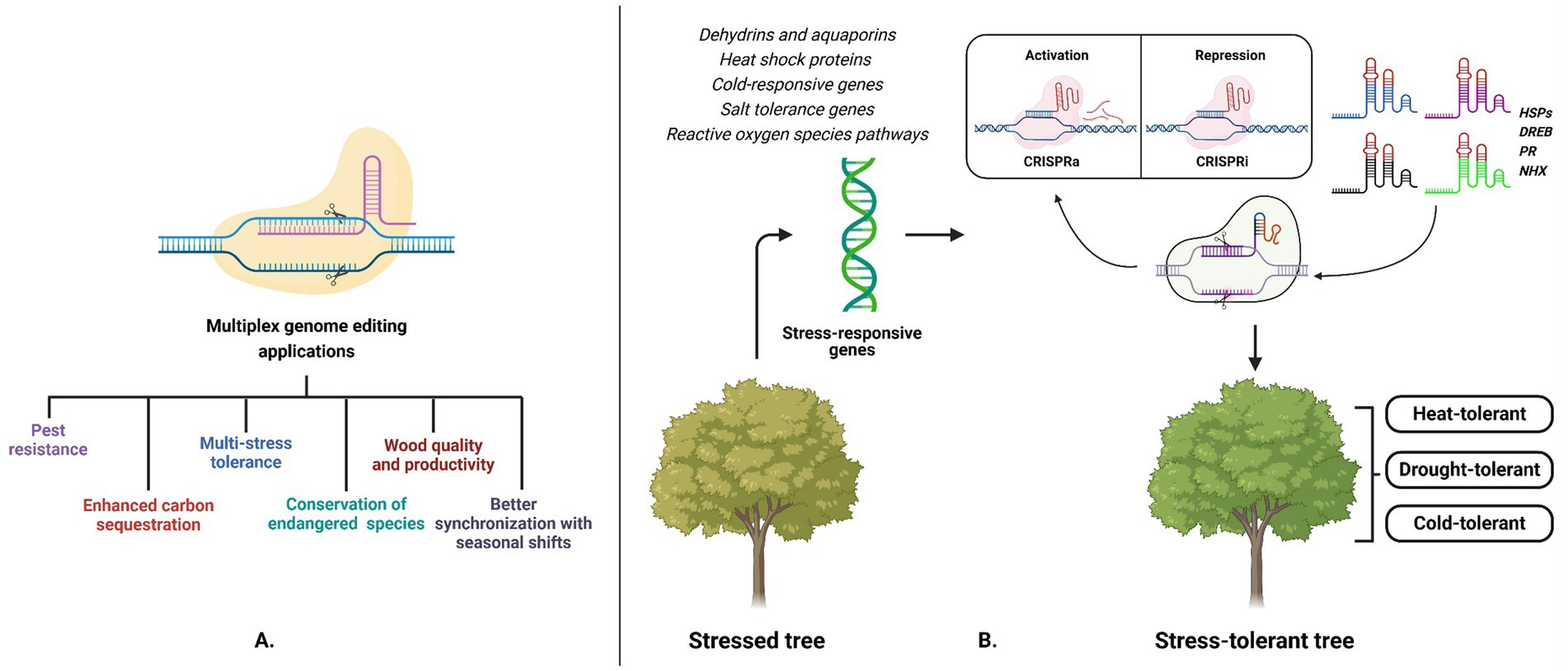
Figure 1. Multiplex genome editing for stress-resilient forestry. This figure illustrates the use of advanced genome editing tools and their applications to develop stress-resilient forest trees capable of thriving in the face of climate change. (A) Applications of multiplex genome editing: the section demonstrates potential forestry applications of genome editing targeting multiple genes simultaneously. Key outcomes include pest resistance, improved tolerance to multiple abiotic stresses (drought, heat, and cold), enhanced wood quality and productivity, increased carbon sequestration, conservation of endangered tree species, and synchronization of phenological traits with seasonal changes. (B) Stress-responsive genes and stress-tolerant trees: stress-responsive genes, such as dehydrins, aquaporins, heat shock proteins (HSPs), dehydration-responsive element-binding proteins (DREB), pathogenesis-related (PR) proteins, and salt tolerance genes (e.g., NHX), are shown to be critical in stress adaptation. Using CRISPR-mediated technologies like CRISPRa (activation) and CRISPRi (inhibition), precise modulation of these genes can be achieved. This results in trees with improved tolerance to heat, drought, and cold stresses, paving the way for climate-resilient forestry.
3 Critical assessment: challenges and future outlook on “super trees”
The idea of “super trees” (Figure 1B) has captured the imagination of scientists and environmentalists as a potential solution to climate change challenges. Genetically engineered trees, designed to exhibit traits like faster growth, pest resistance, tolerance to extreme weather, and enhanced carbon sequestration, could indeed revolutionize sustainable forestry. However, while the concept holds promise, significant obstacles remain in transforming it into a reality. In the following subsections, we will critically examine the technical, ecological, ethical, and regulatory challenges, along with the future advancements needed to realize the full potential of super trees.
3.1 Technical challenges: a slow road ahead
Developing genetically modified “super trees” is a challenging task due to the unique biology and ecological role of trees. Unlike annual crops, trees grow slowly and live for many years. This slow growth delays the expression of genetic changes, making it difficult to assess their long-term impacts (Kijowska-Oberc et al., 2020). Modifications to improve traits such as drought resistance may unintentionally affect nutrient uptake, soil interactions, or other essential functions. Trees play vital roles in ecosystems, and changes to their genomes could have cascading effects on biodiversity, soil health, and ecosystem services. Therefore, precision is critical to ensure that genetic modifications achieve desired outcomes without negative consequences.
This need for precision is further complicated by the complexity of tree genomes. Tree genomes are highly heterozygous and much larger than those of model plants like Arabidopsis or maize (Neale and Kremer, 2011). Such complexity makes it challenging to achieve precise targeting of specific genes. Gene-editing tools like CRISPR/Cas9 have made significant advancements in plant biotechnology, but they might face limitations when applied to trees. Multiplex genome editing, which allows simultaneous modification of multiple genes, offers great potential but comes with risks, such as off-target effects (Gkazi, 2019; Guo et al., 2023; Gurel et al., 2023). These unintended changes could lead to significant ecological consequences, especially in long-lived organisms like trees. Advancing the precision and stability of CRISPR is essential to address these risks.
Despite these challenges, the high genetic diversity of most forest tree species provides a valuable opportunity. Since many tree species remain undomesticated or are in the early stages of domestication, this genetic diversity can support tree breeding, “plus tree” selection, and the development of highly targeted genome-editing strategies. Utilizing this diversity can help ensure that genetic modifications are both effective and adaptive, reducing the risk of unintended ecological impacts (Cao et al., 2022). However, leveraging this diversity requires robust and efficient methods for genetic transformation.
In vitro regeneration of forest trees remains a major bottleneck in the application of genetic modification. Protocols for somatic embryogenesis and regeneration vary widely among species and genotypes, directly affecting the success of genetic transformations (Giri et al., 2004). Current delivery methods, such as Agrobacterium-mediated transformation or biolistics, often face challenges in achieving high efficiency and precision in trees with large, heterozygous genomes. Without improvements in these methods, even the most advanced genome-editing tools may struggle to deliver consistent results (Song et al., 2019). Recent advancements, including nanoparticle-mediated delivery systems (Cunningham et al., 2018) and CRISPR-associated technologies (including CRISPR-Cas9 ribonucleoprotein delivery, CRISPR-Cas12a for multiplexed editing, and CRISPR-Cas13 for RNA targeting; discussed previously), might show promise in enhancing transformation efficiency and target specificity. These emerging approaches are expected to address existing limitations, paving the way for consistent and reliable results in genome editing of complex tree genomes.
Given the long life cycles of trees, ensuring the stability of CRISPR-edited traits over time is essential. Combining CRISPR/Cas9 with epigenome editing can help enhance precision and maintain the durability of desired traits (Jogam et al., 2022). Long-term field trials and multi-generational studies are critical to evaluate the stability of these modifications and their broader ecological impacts. Comprehensive assessments of how genetic changes influence soil health, biodiversity, and ecosystem services are necessary to avoid unintended consequences and ensure sustainability.
By addressing these interconnected challenges (ranging from genome complexity and regeneration barriers to trait stability and ecological evaluation), it might be possible to develop super trees that are both effective and sustainable. Integrating the genetic diversity of native trees with advanced genome-editing tools and optimized protocols offers a promising path forward. Such a systematic approach will ensure that genetic modifications contribute to forestry innovation while protecting and preserving forest ecosystems.
3.2 Artificial intelligence: a promising aid but not a panacea
Artificial intelligence (AI) offers a powerful means of improving the precision of gene-editing processes. By analyzing vast amounts of genomic data, AI can help identify potential off-target effects and refine the editing process (Lin and Wong, 2018; Cheng et al., 2023; Sherkatghanad et al., 2023). AI could also simulate how genetic modifications will interact with the environment, enabling scientists to predict the ecological impact of modified trees. This predictive power could lead to safer and more informed decisions when introducing genetically engineered trees into natural ecosystems (Chen L. et al., 2023; Holzinger et al., 2023; Callaway, 2024). However, AI is not a magic bullet. The complexity of tree genetics and ecosystems means that AI’s predictions will always be limited by the quality and breadth of the data available. While AI has shown potential in predicting phenotypic traits in woody plants, its accuracy varies significantly across species and traits. For example, a study on almond germplasm demonstrated moderate success using the Random Forest algorithm, achieving a correlation of 0.727 ± 0.020 and an R2 of 0.511 ± 0.025 for predicting shelling fraction. This approach also integrated SHAP (SHapley Additive exPlanations) values, which highlighted key genomic regions and identified genes potentially linked to seed development, emphasizing the dual benefits of prediction and interpretability (Novielli et al., 2024). However, similar applications in forest trees, such as predicting disease resistance and wood quality, are still in their early stages. A critical limitation in advancing AI-driven predictions is the lack of robust genomic and phenotypic datasets for training models. AI’s effectiveness depends on the quality of its models, which may fail to capture the full complexity of ecological interactions, further underscoring the challenges of applying AI in forestry research.
While AI holds promise, it should be viewed as a tool to support, not replace, careful scientific experimentation and ethical consideration. AI can certainly speed up the development of super trees by improving precision, but it cannot eliminate the need for careful field trials and environmental monitoring.
3.3 Ecological, ethical, and regulatory considerations
The introduction of genetically modified trees into natural ecosystems raises serious ecological concerns. Trees are long-lived, and their impact on the environment may only become apparent decades after release. In addition to the risk of disrupting biodiversity, genetically modified trees could affect other ecosystem functions, such as pollination, nutrient cycling, and water regulation (Johnson and Kirby, 2004). The long-term effects of these modifications on local flora and fauna remain largely unknown, and this uncertainty complicates the regulatory approval process. Unlike annual crops like maize and soybeans, which are cultivated in controlled environments, genetically modified trees will likely be planted in natural habitats, where their impact on the ecosystem will be less predictable (Jacobs et al., 2023; James, 2024).
Ethical considerations are particularly pertinent in this context. Public apprehension surrounding genetically modified organisms (GMOs) remains significant, especially when applied to trees, which serve as critical environmental components and are often regarded as shared public resources. It is essential to differentiate GMOs from genome-edited organisms. Among genome editing techniques, those categorized as Site Directed Nuclease 1 (SDN-1) are not considered GMOs under the Cartagena Protocol. Public perception of both GMOs and genome-edited trees will be pivotal in shaping the development and societal acceptance of “super trees, “particularly in the face of climate change (Brennan et al., 2023; Berseth et al., 2024). Transparent research, robust risk assessments, and clear communication with the public will be necessary to gain the support needed for the widespread adoption of genetically engineered trees. In this regard, DNA-free genome editing using the CRISPR/Cas9 system provides an innovative and potentially more acceptable solution. This approach employs pre-assembled CRISPR-Cas9 ribonucleoproteins to edit genomes without incorporating foreign DNA, addressing public concerns about transgenic modifications (Metje-Sprink et al., 2019). Successfully applied in crops like rice (Lu et al., 2021), and wheat (Poddar et al., 2023), these methods enhance agronomic traits and stress tolerance while aligning with regulatory frameworks distinguishing them from conventional GMOs. In crops such as rice and wheat, the probability of obtaining null segregants (plants that no longer carry the CRISPR components in subsequent generations) is high, making this strategy particularly appealing for developing genome-edited next-generation crops. However, this approach has not been extensively focused on forest trees. Despite the shared biological mechanisms and advanced delivery techniques that suggest feasibility, challenges such as longer generation times, higher genetic complexity, and less-established delivery systems in trees may contribute to this gap. Addressing these barriers could unlock the potential of DNA-free genome editing to sustainably develop climate-resilient forests.
3.4 The path forward: integration with traditional breeding methods
Despite the many challenges, the potential for super trees remains promising. Advances in gene-editing technologies, such as base editing and prime editing, offer more precision and fewer off-target effects than traditional CRISPR/Cas9 (Molla et al., 2021; Hua et al., 2022; Chen and Liu, 2023; Li et al., 2023). These tools could eventually make it easier to develop trees with multiple beneficial traits, including drought resistance, faster growth, and pest tolerance. However, gene editing should not replace traditional breeding methods but rather complement them. Traditional breeding techniques, such as marker-assisted selection (MAS), can help preserve genetic diversity, which is crucial for ensuring the long-term resilience of forest ecosystems (Mohammad et al., 2024). By combining genetic engineering with traditional methods, researchers can create trees that are both resilient and diverse, ensuring they can thrive in a changing climate.
Moreover, genome edited trees could support agroecological practices by reducing the need for harmful chemicals and improving ecosystem health. For example, pest-resistant super trees could reduce the reliance on chemical pesticides, while carbon-sequestering trees could help mitigate climate change. However, integrating genetically modified trees into agroecological systems requires careful planning. It is essential to ensure that these trees support biodiversity and maintain the ecosystem services that forests provide, rather than disrupting them.
4 Conclusion: the long road ahead
The development of super trees is an ambitious and exciting goal, brimming with potential. Advances in gene-editing tools, synthetic biology, and AI offer promising solutions to technical challenges, while ecological, ethical, and regulatory considerations will shape their responsible implementation. Super trees could become pivotal in combating climate change and securing sustainable forestry, but realizing this vision demands collaboration among scientists, policymakers, and the public. With ongoing research and a balanced approach to risks and benefits, these innovations could transform forestry for a resilient future. Yet, caution is vital, as the journey ahead is intricate, requiring time, innovation, and careful stewardship to turn this bold concept into reality.
Author contributions
MS: Conceptualization, Formal analysis, Investigation, Methodology, Software, Visualization, Writing – original draft, Writing – review & editing. SM: Supervision, Writing – review & editing. RB: Funding acquisition, Validation, Writing – review & editing. LS: Funding acquisition, Writing – review & editing. KŠ: Funding acquisition, Writing – review & editing.
Funding
The author(s) declare that financial support was received for the research, authorship, and/or publication of this article. This work was funded by the Faculty of Economics and Management, Czech University of Life Sciences in Prague, grant number 2022B0004.
Conflict of interest
The authors declare that the research was conducted in the absence of any commercial or financial relationships that could be construed as a potential conflict of interest.
Generative AI statement
The authors declare that no Gen AI was used in the creation of this manuscript.
Publisher’s note
All claims expressed in this article are solely those of the authors and do not necessarily represent those of their affiliated organizations, or those of the publisher, the editors and the reviewers. Any product that may be evaluated in this article, or claim that may be made by its manufacturer, is not guaranteed or endorsed by the publisher.
References
Abdallah, N. A., Elsharawy, H., Abulela, H. A., Thilmony, R., Abdelhadi, A. A., and Elarabi, N. I. (2025). Multiplex CRISPR/Cas 9-mediated genome editing to address drought tolerance in wheat. GM Crops Food 16, 1–17. doi: 10.1080/21645698.2022.2120313
Abdelrahman, M., Wei, Z., Rohila, J. S., and Zhao, K. (2021). Multiplex genome-editing Technologies for Revolutionizing Plant Biology and Crop Improvement. Front. Plant Sci. 12:721203. doi: 10.3389/fpls.2021.721203
An, Y., Geng, Y., Yao, J., Fu, C., Lu, M., Wang, C., et al. (2020). Efficient genome editing in Populus using CRISPR/Cas 12a. Front. Plant Sci. 11:593938. doi: 10.3389/fpls.2020.593938
Armario Najera, V., Twyman, R. M., Christou, P., and Zhu, C. (2019). Applications of multiplex genome editing in higher plants. Curr. Opin. Biotechnol. 59, 93–102. doi: 10.1016/j.copbio.2019.02.015
Bahariah, B., Masani, M. Y. A., Fizree, M. P. M. A. A., Rasid, O. A., and Parveez, G. K. A. (2023). Multiplex CRISPR/Cas 9 gene-editing platform in oil palm targeting mutations in EgFAD2 and EgPAT genes. J. Genet. Eng. Biotechnol. 21:3. doi: 10.1186/s43141-022-00459-5
Bandyopadhyay, A., Kancharla, N., Javalkote, V. S., Dasgupta, S., and Brutnell, T. P. (2020). CRISPR-Cas 12a (Cpf1): a versatile tool in the plant genome editing tool box for agricultural advancement. Front. Plant Sci. 11:584151. doi: 10.3389/fpls.2020.584151
Berseth, V., Taylor, J., Hutchen, J., Nguyen, V., Schott, S., and Klenk, N. (2024). Constructing the public in public perceptions research: a case study of forest genomics. Public Underst. Sci. 33, 483–503. doi: 10.1177/09636625231210453
Bewg, W. P., Harding, S. A., Engle, N. L., Vaidya, B. N., Zhou, R., Reeves, J., et al. (2022). Multiplex knockout of trichome-regulating MYB duplicates in hybrid poplar using a single gRNA. Plant Physiol. 189, 516–526. doi: 10.1093/plphys/kiac128
Brennan, A. N., Ma, Z., and Jacobs, D. F. (2023). Perceptions of land managers towards using hybrid and genetically modified trees. New For. 54, 605–636. doi: 10.1007/s11056-021-09895-6
Bruegmann, T., Fendel, A., Zahn, V., and Fladung, M. (2024). “Genome editing in Forest trees,” In A roadmap for plant genome editing, eds. A. Ricroch, D. Eriksson, D. Miladinović, J. Sweet, K. LaereVan, and E. Woźniak-Gientka (Cham: Springer Nature Switzerland), 347–372.
Callaway, E. (2024). “Chat GPT for CRISPR” creates new gene-editing tools. Nature 629:272. doi: 10.1038/d41586-024-01243-w
Cantarello, E., Jacobsen, J. B., Lloret, F., and Lindner, M. (2024). Shaping and enhancing resilient forests for a resilient society. Ambio 53, 1095–1108. doi: 10.1007/s13280-024-02006-7
Cao, H. X., Vu, G. T. H., and Gailing, O. (2022). From genome sequencing to CRISPR-based genome editing for climate-resilient Forest trees. Int. J. Mol. Sci. 23:966. doi: 10.3390/ijms23020966
Cao, J., Xiao, Q., and Yan, Q. (2018). The multiplexed CRISPR targeting platforms. Drug Discov. Today Technol. 28, 53–61. doi: 10.1016/j.ddtec.2018.01.001
Charles, K. M., and Stehlik, I. (2021). Assisted species migration and hybridization to conserve cold-adapted plants under climate change. Conserv. Biol. 35, 559–566. doi: 10.1111/cobi.13583
Charrier, A., Vergne, E., Dousset, N., Richer, A., Petiteau, A., and Chevreau, E. (2019). Efficient targeted mutagenesis in apple and first time edition of pear using the CRISPR-Cas 9 system. Front. Plant Sci. 10:40. doi: 10.3389/fpls.2019.00040
Chen, L., Chen, Z., Zhang, Y., Liu, Y., Osman, A. I., Farghali, M., et al. (2023). Artificial intelligence-based solutions for climate change: a review. Environ. Chem. Lett. 21, 2525–2557. doi: 10.1007/s10311-023-01617-y
Chen, P. J., and Liu, D. R. (2023). Prime editing for precise and highly versatile genome manipulation. Nat. Rev. Genet. 24, 161–177. doi: 10.1038/s41576-022-00541-1
Chen, Y.-H., Sharma, S., Bewg, W. P., Xue, L.-J., Gizelbach, C. R., and Tsai, C.-J. (2023). Multiplex editing of the Nucleoredoxin 1 tandem Array in poplar: from small Indels to translocations and complex inversions. CRISPR J. 6, 339–349. doi: 10.1089/crispr.2022.0096
Cheng, X., Li, Z., Shan, R., Li, Z., Wang, S., Zhao, W., et al. (2023). Modeling CRISPR-Cas13d on-target and off-target effects using machine learning approaches. Nat. Commun. 14:752. doi: 10.1038/s41467-023-36316-3
Cortés, A. J., Restrepo-Montoya, M., and Bedoya-Canas, L. E. (2020). Modern strategies to assess and breed Forest tree adaptation to changing climate. Front. Plant Sci. 11:583323. doi: 10.3389/fpls.2020.583323
Cunningham, F. J., Goh, N. S., Demirer, G. S., Matos, J. L., and Landry, M. P. (2018). Nanoparticle-mediated delivery towards advancing plant genetic engineering. Trends Biotechnol. 36, 882–897. doi: 10.1016/j.tibtech.2018.03.009
Doust, A. N., Lukens, L., Olsen, K. M., Mauro-Herrera, M., Meyer, A., and Rogers, K. (2014). Beyond the single gene: how epistasis and gene-by-environment effects influence crop domestication. Proc. Natl. Acad. Sci. 111, 6178–6183. doi: 10.1073/pnas.1308940110
Fan, D., Liu, T., Li, C., Jiao, B., Li, S., Hou, Y., et al. (2015). Efficient CRISPR/Cas9-mediated targeted mutagenesis in Populus in the first generation. Sci. Rep. 5, 12217. doi: 10.1038/srep12217
Giri, C. C., Shyamkumar, B., and Anjaneyulu, C. (2004). Progress in tissue culture, genetic transformation and applications of biotechnology to trees: an overview. Trees 18, 115–135. doi: 10.1007/s00468-003-0287-6
Gkazi, S. A. (2019). Quantifying CRISPR off-target effects. Emerg. Top Life Sci. 3, 327–334. doi: 10.1042/ETLS20180146
Guo, C., Ma, X., Gao, F., and Guo, Y. (2023). Off-target effects in CRISPR/Cas9 gene editing. Front. Bioeng. Biotechnol. 11:1143157. doi: 10.3389/fbioe.2023.1143157
Gurel, F., Wu, Y., Pan, C., Cheng, Y., Li, G., Zhang, T., et al. (2023). On- and off-target analyses of CRISPR-Cas12b genome editing Systems in Rice. CRISPR J. 6, 62–74. doi: 10.1089/crispr.2022.0072
Hassan, M. M., Zhang, Y., Yuan, G., De, K., Chen, J.-G., Muchero, W., et al. (2021). Construct design for CRISPR/Cas-based genome editing in plants. Trends Plant Sci. 26, 1133–1152. doi: 10.1016/j.tplants.2021.06.015
Healy, T. M., Brennan, R. S., Whitehead, A., and Schulte, P. M. (2018). Tolerance traits related to climate change resilience are independent and polygenic. Glob. Chang. Biol. 24, 5348–5360. doi: 10.1111/gcb.14386
Hillary, V. E., and Ceasar, S. A. (2023). A review on the mechanism and applications of CRISPR/Cas9/Cas12/Cas13/Cas14 proteins utilized for genome engineering. Mol. Biotechnol, 65, 311–325. doi: 10.1007/s12033-022-00567-0
Holzinger, A., Keiblinger, K., Holub, P., Zatloukal, K., and Müller, H. (2023). AI for life: trends in artificial intelligence for biotechnology. New Biotechnol. 74, 16–24. doi: 10.1016/j.nbt.2023.02.001
Hua, K., Han, P., and Zhu, J.-K. (2022). Improvement of base editors and prime editors advances precision genome engineering in plants. Plant Physiol. 188, 1795–1810. doi: 10.1093/plphys/kiab591
Jacobs, D. F., Dumroese, R. K., Brennan, A. N., Campbell, F. T., Conrad, A. O., Delborne, J. A., et al. (2023). Reintroduction of at-risk forest tree species using biotechnology depends on regulatory policy, informed by science and with public support. New For. 54, 587–604. doi: 10.1007/s11056-023-09980-y
James, D. (2024). “Impact assessment of genetically engineered trees: an overview on risk assessment and management” in Biotechnological approaches for sustaining Forest trees and their products. eds. D. Thomas T, M. K. Razdan, and A. Kumar (Singapore: Springer Nature Singapore), 425–462.
Jia, J., Zhou, J., Shi, W., Cao, X., Luo, J., Polle, A., et al. (2017). Comparative transcriptomic analysis reveals the roles of overlapping heat−/drought-responsive genes in poplars exposed to high temperature and drought. Sci. Rep. 7:43215. doi: 10.1038/srep43215
Jogam, P., Sandhya, D., Alok, A., Peddaboina, V., Allini, V. R., and Zhang, B. (2022). A review on CRISPR/Cas-based epigenetic regulation in plants. Int. J. Biol. Macromol. 219, 1261–1271. doi: 10.1016/j.ijbiomac.2022.08.182
Johnson, B., and Kirby, K. (2004). “Potential impacts of genetically modified trees on biodiversity of forestry plantations: a global perspective” in The bioengineered forest (pp. 190–207). (Routledge). Available at:https://www.taylorfrancis.com/chapters/edit/10.4324/9781936331352-14/potential-impacts-genetically-modified-trees-biodiversity-forestry-plantations-brian-johnson-keith-kirby
Kavuri, N. R., Ramasamy, M., Qi, Y., and Mandadi, K. (2022). Applications of CRISPR/Cas13-based RNA editing in plants. Cells 11:2665. doi: 10.3390/cells11172665
Keenan, R. J. (2015). Climate change impacts and adaptation in forest management: a review. Ann. For. Sci. 72, 145–167. doi: 10.1007/s13595-014-0446-5
Kijowska-Oberc, J., Staszak, A. M., Kamiński, J., and Ratajczak, E. (2020). Adaptation of Forest trees to rapidly changing climate. Forests 11:123. doi: 10.3390/f11020123
Li, J., Zhang, C., He, Y., Li, S., Yan, L., Li, Y., et al. (2023). Plant base editing and prime editing: the current status and future perspectives. J. Integr. Plant Biol. 65, 444–467. doi: 10.1111/jipb.13425
Lin, J., and Wong, K.-C. (2018). Off-target predictions in CRISPR-Cas9 gene editing using deep learning. Bioinformatics 34, i656–i663. doi: 10.1093/bioinformatics/bty554
Lu, Y., Wang, J., Chen, B., Mo, S., Lian, L., Luo, Y., et al. (2021). A donor-DNA-free CRISPR/Cas-based approach to gene knock-up in rice. Nat. Plants 7, 1445–1452. doi: 10.1038/s41477-021-01019-4
Ma, X., and Liu, Y.-G. (2016). CRISPR/Cas9-based multiplex genome editing in monocot and dicot plants. Curr. Protoc. Mol. Biol. 115:31.6.1-31. 6.21. doi: 10.1002/cpmb.10
Mahas, A., Aman, R., and Mahfouz, M. (2019). CRISPR-Cas13d mediates robust RNA virus interference in plants. Genome Biol. 20:263. doi: 10.1186/s13059-019-1881-2
Malhi, Y., Franklin, J., Seddon, N., Solan, M., Turner, M. G., Field, C. B., et al. (2020). Climate change and ecosystems: threats, opportunities and solutions. Philos. Trans. R. Soc. B 375:20190104. doi: 10.1098/rstb.2019.0104
McCarty, N. S., Graham, A. E., Studená, L., and Ledesma-Amaro, R. (2020). Multiplexed CRISPR technologies for gene editing and transcriptional regulation. Nat. Commun. 11:1281. doi: 10.1038/s41467-020-15053-x
McDowell, N. G., Sapes, G., Pivovaroff, A., Adams, H. D., Allen, C. D., Anderegg, W. R. L., et al. (2022). Mechanisms of woody-plant mortality under rising drought, CO2 and vapour pressure deficit. Nat. Rev. Earth Environ. 3, 294–308. doi: 10.1038/s43017-022-00272-1
Metje-Sprink, J., Menz, J., Modrzejewski, D., and Sprink, T. (2019). DNA-free genome editing: past, present and future. Front. Plant Sci. 9:1957. doi: 10.3389/fpls.2018.01957
Min, T., Hwarari, D., Li, D., Movahedi, A., and Yang, L. (2022). CRISPR-based genome editing and its applications in Woody plants. Int. J. Mol. Sci. 23:10175. doi: 10.3390/ijms231710175
Mohammad, N., Dahayat, A., and Agrahari, H. (2024). “Molecular markers in the management and improvement of Forest genetic resources” in Biotechnological approaches for sustaining Forest trees and their products. eds. D. Thomas T, M. K. Razdan, and A. Kumar (Singapore: Springer Nature Singapore), 181–198.
Molla, K. A., Sretenovic, S., Bansal, K. C., and Qi, Y. (2021). Precise plant genome editing using base editors and prime editors. Nat. Plants 7, 1166–1187. doi: 10.1038/s41477-021-00991-1
Neale, D. B., and Kremer, A. (2011). Forest tree genomics: growing resources and applications. Nat. Rev. Genet. 12, 111–122. doi: 10.1038/nrg2931
Novielli, P., Romano, D., Pavan, S., Losciale, P., Stellacci, A. M., Diacono, D., et al. (2024). Explainable artificial intelligence for genotype-to-phenotype prediction in plant breeding: a case study with a dataset from an almond germplasm collection. Front. Plant Sci. 15:1434229. doi: 10.3389/fpls.2024.1434229
Pak, S., Li, C., Pak, S., and Li, C. (2022). Progress and challenges in applying CRISPR/Cas techniques to the genome editing of trees. For. Res. (Fayettev) 2:6. doi: 10.48130/FR-2022-0006
Poddar, S., Tanaka, J., Running, K. L. D., Kariyawasam, G. K., Faris, J. D., Friesen, T. L., et al. (2023). Optimization of highly efficient exogenous-DNA-free Cas9-ribonucleoprotein mediated gene editing in disease susceptibility loci in wheat (Triticum aestivum L.). Front. Plant Sci. 13:1084700. doi: 10.3389/fpls.2022.1084700
Poovaiah, C., Phillips, L., Geddes, B., Reeves, C., Sorieul, M., and Thorlby, G. (2021). Genome editing with CRISPR/Cas9 in Pinus radiata (D. Don). BMC Plant Biol. 21:363. doi: 10.1186/s12870-021-03143-x
Prajapati, R., and Tyagi, K. (2024). “CRISPR/Cas-mediated multiplex genome editing in plants and applications” in Applications of genome engineering in plants. Ed. Santosh Kumar Upadhyay (Wiley), 20–39. Available at: https://onlinelibrary.wiley.com/doi/abs/10.1002/9781394183913.ch2
Ribeiro, J. A., Varanda, C. M. R., Materatski, P., Campos, M. D., Patanita, M., Albuquerque, A., et al. (2024). “CRISPR/Cas13 for the control of plant viruses” in CRISPR and plant functional genomics (CRC Press). Available at: https://www.taylorfrancis.com/chapters/edit/10.1201/9781003387060-16/crispr-cas13-control-plant-viruses-joana-ribeiro-carla-varanda-patrick-materatski-maria-doroteia-campos-mariana-patanita-andr%C3%A9-albuquerque-nicol%C3%A1s-garrido-tom%C3%A1s-monteiro-filipa-santos-maria-ros%C3%A1rio-f%C3%A9lix
Senthilnathan, R., Ilangovan, I., Kunale, M., Easwaran, N., Ramamoorthy, S., Veeramuthu, A., et al. (2023). An update on CRISPR-Cas12 as a versatile tool in genome editing. Mol. Biol. Rep. 50, 2865–2881. doi: 10.1007/s11033-023-08239-1
Sharma, V. K., Marla, S., Zheng, W., Mishra, D., Huang, J., Zhang, W., et al. (2022). CRISPR guides induce gene silencing in plants in the absence of Cas. Genome Biol. 23:6. doi: 10.1186/s13059-021-02586-7
Sherkatghanad, Z., Abdar, M., Charlier, J., and Makarenkov, V. (2023). Using traditional machine learning and deep learning methods for on- and off-target prediction in CRISPR/Cas9: a review. Brief. Bioinform. 24:bbad131. doi: 10.1093/bib/bbad131
Singh, A., Sharan, S., Chakraborty, A., Roy, A., and Singh, I. K. (2024). Transgenic poplar for resistance against pest and pathogen attack in forests: an overview. Front. For. Glob. Change 7:1490562. doi: 10.3389/ffgc.2024.1490562
Singh, J., Sharma, D., Brar, G. S., Sandhu, K. S., Wani, S. H., Kashyap, R., et al. (2022). CRISPR/Cas tool designs for multiplex genome editing and its applications in developing biotic and abiotic stress-resistant crop plants. Mol. Biol. Rep. 49, 11443–11467. doi: 10.1007/s11033-022-07741-2
Song, G., Prieto, H., and Orbovic, V. (2019). Agrobacterium-mediated transformation of tree fruit crops: methods, Progress, and challenges. Front. Plant Sci. 10:226. doi: 10.3389/fpls.2019.00226
Sulis, D. B., Jiang, X., Yang, C., Marques, B. M., Matthews, M. L., Miller, Z., et al. (2023). Multiplex CRISPR editing of wood for sustainable fiber production. Science 381, 216–221. doi: 10.1126/science.add4514
Tran, M. T., Son, G. H., Song, Y. J., Nguyen, N. T., Park, S., Thach, T. V., et al. (2023). CRISPR-Cas9-based precise engineering of SlHyPRP1 protein towards multi-stress tolerance in tomato. Front. Plant Sci. 14:1186932. doi: 10.3389/fpls.2023.1186932
Wolabu, T. W., Mahmood, K., Chen, F., Torres-Jerez, I., Udvardi, M., Tadege, M., et al. (2024). Mutating alfalfa COUMARATE 3-HYDROXYLASE using multiplex CRISPR/Cas9 leads to reduced lignin deposition and improved forage quality. Front. Plant Sci. 15:1363182. doi: 10.3389/fpls.2024.1363182
Wolter, F., Schindele, P., and Puchta, H. (2019). Plant breeding at the speed of light: the power of CRISPR/Cas to generate directed genetic diversity at multiple sites. BMC Plant Biol. 19:176. doi: 10.1186/s12870-019-1775-1
Xu, W., and Prescott, C. E. (2024). Can assisted migration mitigate climate-change impacts on forests? For. Ecol. Manag. 556:121738. doi: 10.1016/j.foreco.2024.121738
Yan, F., Wang, W., and Zhang, J. (2019). CRISPR-Cas12 and Cas13: the lesser known siblings of CRISPR-Cas9. Cell Biol. Toxicol. 35, 489–492. doi: 10.1007/s10565-019-09489-1
Yao, Q., Shen, R., Shao, Y., Tian, Y., Han, P., Zhang, X., et al. (2024). Efficient and multiplex gene upregulation in plants through CRISPR-Cas-mediated knockin of enhancers. Mol. Plant 17, 1472–1483. doi: 10.1016/j.molp.2024.07.009
Yu, Y., Pan, Z., Wang, X., Bian, X., Wang, W., Liang, Q., et al. (2022). Targeting of SPCSV-RNase3 via CRISPR-Cas13 confers resistance against sweet potato virus disease. Mol. Plant Pathol. 23, 104–117. doi: 10.1111/mpp.13146
Keywords: climate change, forest resilience, multiplex genome editing, polygenic trait engineering, sustainable forestry
Citation: Sen MK, Mondal SK, Bharati R, Severova L and Šrédl K (2025) Multiplex genome editing for climate-resilient woody plants. Front. For. Glob. Change. 8:1542459. doi: 10.3389/ffgc.2025.1542459
Edited by:
Milica Zlatkovic, University of Novi Sad, SerbiaReviewed by:
Hafiz Muhammad Ahmad, Government College University, Faisalabad, PakistanAi Nagamine, University of Tsukuba, Japan
Faiza Shafique Khan, Chinese Academy of Tropical Agricultural Sciences, China
Copyright © 2025 Sen, Mondal, Bharati, Severova and Šrédl. This is an open-access article distributed under the terms of the Creative Commons Attribution License (CC BY). The use, distribution or reproduction in other forums is permitted, provided the original author(s) and the copyright owner(s) are credited and that the original publication in this journal is cited, in accordance with accepted academic practice. No use, distribution or reproduction is permitted which does not comply with these terms.
*Correspondence: Madhab Kumar Sen, c2VubUBhZi5jenUuY3o=