- 1Faculty of Environment, University of Northern British Columbia, Prince George, BC, Canada
- 2British Columbia Ministry of Water, Land, and Resource Stewardship, Prince George, BC, Canada
In forestry, glyphosate-based herbicide (GBH) is applied to some logged areas to remove deciduous and herbaceous vegetation competing with commercial coniferous trees. Glyphosate has the potential to form insoluble complexes with metal ions, altering the bioavailability of metals in soil and water. The aim of this study was to determine if GBH influenced the concentrations of available nutrients in forest plants. We sampled willow (Salix sp.), red osier dogwood (Cornus sericea), and fireweed (Chamaenerion angustifolium) from forests up to 12 years after GBH applications. Across the three focal species, only Mn consistently increased with GBH treatment. Significant positive correlations between nutrient and glyphosate concentrations within plant tissues were found for Mn and Zn, while negative correlations were found for Ba, Ca, Mg, and Ni. B, Ca, Mn, and species were significant predictors of GBH treatment. A greater number of significant differences between controls and treated samples were noted in nutrients of fireweed and red osier dogwood at year one post-treatment, while willow showed greater differences between controls and treated samples at year 12 post-treatment. The analyses conducted indicate a relationship between GBH treatment and resulting nutrient regimes after GBH applications, yet it is complex and variable. It is clear that there are species specific trends within the dataset. Due to this complexity, more research should be conducted, as many questions remain unanswered. Further research is needed to disentangle both short and long-term impacts of widespread use of GBH products in modern silviculture.
1 Introduction
Glyphosate [N-(phosphonomethyl) glycine] is the most widely used herbicidal compound in the world (Benbrook, 2016), primarily for agricultural, forestry, and invasive weed control (Henderson et al., 2019). A highly effective, non-selective, broad-spectrum herbicide first introduced in 1974, glyphosate is the active ingredient in numerous glyphosate-based herbicide (GBH) formulations, including Roundup®, Vision®, VisionMax®, and GlySil® (Baylis, 2000; Dost, 2003; Thompson and Pitt, 2011). Glyphosate has been studied extensively in the context of agricultural use. Few studies have examined the effects that these herbicides have on the anatomy and physiology of plants remaining in post-application scenarios in forests, even though it is the most prominently used herbicide active ingredient in forestry operations. Upon GBH application, glyphosate is absorbed into plant leaves, stems or roots (Bernards et al., 2005), and is translocated throughout the plant. After cycling through the plant in both xylem and phloem, glyphosate accumulates in the apical meristems of roots and young leaves (Bernards et al., 2005; Machado et al., 2009; Fadin et al., 2018).
In forestry, aerial herbicide applications are used most (Thompson et al., 2012), causing uneven applications to understory plants (Lloyd, 1990). Incomplete or variable coverage in applications causes sublethal doses of GBH and understory plant survival (Székács and Darvas, 2012; Cederlund, 2017; Fadin et al., 2018). These plants may exhibit deformities, suppressed growth and other negative effects, even though the concentration reaching non-targeted plants in this situation is typically low (Fadin et al., 2018; Timms and Wood, 2020; Golt and Wood, 2021). Glyphosate may persist in perennial plant tissues for an extended duration of time (Roy et al., 1989; Mamy et al., 2016), only recently shown to extend to a year or more (Wood, 2019; Edge et al., 2021; Botten et al., 2021). The exact duration of residue persistence is unknown for plants in forested environments of British Columbia (BC), Canada; however, glyphosate residue was found in 2% of plant root tissues (e.g., fireweed root) up to 12 years after GBH application (Botten et al., 2021), which raises the question if GBH-treated cutblocks could put foraging wildlife at greater risk from cumulative sublethal exposure.
In addition to its herbicidal action, glyphosate is a recognized chelating agent, forming strong, insoluble complexes on its amine, carboxylate, and phosphonate groups with transition-metal and other divalent and trivalent metal ions, including calcium (Ca), copper (Cu), iron (Fe), magnesium (Mg), manganese (Mn) and zinc (Zn) (Subramaniam and Hoggard, 1988; Eker et al., 2006; Cakmak et al., 2009; Jayasumana et al., 2014).
The bioavailability, biological uptake, and thus the acute toxicity of some heavy metals, including silver (Ag), cadmium (Cd), chromium (Cr), Cu, Ni, lead (Pb) and Zn, can be reduced through formation of insoluble metal complexes with glyphosate in water (Tsui et al., 2005). There is also some concern that glyphosate ions present in soil may prevent the adsorption and retention of trace and/or toxic metals by soil particles, even causing desorption from soils, resulting in increased mobility in the soil environment as complexes enter the aqueous phase in soil. Application of GBH to soils increases the mobilization of a range of elements, including aluminum (Al), arsenic (As), Cd, Cu, P, Pb, Ni, silicon (Si) and Zn (Barrett and McBride, 2006; Wang et al., 2006; Divisekara et al., 2018).
Glyphosate-metal complexes have varying degrees of solubility. Sundaram and Sundaram (1997) determined that the solubility products (Ksp) of 1:1 complexes (one glyphosate molecule to one metal ion) of glyphosate with Mg2+, Ca2+, Mn2+, Zn2+, Cu2+ and Fe3+ decreased in the order of Mg ≍ Ca > Mn > Zn > Cu > Fe in distilled water, with lower solubilities in soil. This means that less-soluble complexes with a lower Ksp., especially Fe, will have a greater likelihood of remaining complexed.
Modeling of glyphosate chelation in soils suggests that divalent cations generally form more stable complexes with glyphosate than do trivalent cations, with the following overall stability order taking into account different molecular coordination geometries of both 1:1 and 2:1 (two glyphosate to one metal ion) complexes: Zn2+ > Cu2+ > cobalt (Co)3+ > Fe3+ > Cr3+ > Al3+ > Ca2+ > Mg2+ (Caetano et al., 2012). Stability constants indicate decreasing stability for the following glyphosate-metal complexes: Cu2+ > Zn2+ > Ni2+ > Co2+ > Cd2+ > Fe3+ > Mn2+ > Ca2+ ≍ Mg2+ (Madsen et al., 1978; Motekaitis and Martell, 1985).
While some metal elements are not essential (e.g., Co, Al) and others are toxic (e.g., Pb, As), many are essential nutrients to plants and animals, and their reduced bioavailability due to complexation with glyphosate may result in nutritional deficiencies. Aside from complexing with metal nutrients in the soil and water, glyphosate stored in plant tissues could immobilize certain essential nutritive metals within the plants themselves (Eker et al., 2006; Cakmak et al., 2009). This in turn can affect the availability of nutrients for animals consuming plants treated with, and retaining, sublethal doses of GBH (Mertens et al., 2018).
The purpose of this research was to examine the hypothesis that GBH application will affect metal nutrient concentrations in perennial forest plant tissues. The rationale for this hypothesis lays in the facts that: (a) glyphosate and its residues exhibit persistence in soil and plant tissues after application (Botten et al., 2021); (b) glyphosate is a chelating agent, known to form insoluble complexes with metal ions in soil and in water (Eker et al., 2006; Cakmak et al., 2009), and; (c) short term studies (up to 40 days) have reported reduced concentrations of some plant nutrients after sublethal GBH application (Cakmak et al., 2009). Long term nutrient depletion in perennial plants could have negative repercussions for plant health.
Our specific objective was to determine if persistence (from 1 year up to 12 years) of glyphosate in selected perennial forest plant tissues influenced the concentration of plant mineral nutrients. We contextualized our study in operationally managed forests of British Columbia (BC) to gain understanding outside of agriculture and controlled experimental settings. We predicted that, due to interaction of metal ions with glyphosate (such as in mechanisms like chelation), treated samples would contain reduced concentrations of nutrients 1 year after treatment, but that this effect would diminish over time in concordance with decreasing glyphosate levels in plant tissues.
2 Methods
2.1 Study area
This research project was conducted in forest cutblocks in the Omineca and Peace regions of BC, Canada (Figure 1), within the Sub-Boreal Spruce (SBS) and Boreal White and Black Spruce (BWBS) Biogeoclimatic Ecosystem Classification (BEC) zones. The SBS BEC zone dominates BC’s central interior and features a continental climate with seasonal extremes of temperature: severe, snowy winters, moderate annual precipitation, and relatively warm, moist, and short summers (Meidinger and Pojar, 1991). The BWBS zone extends from the coastal BC mountains to the Alberta border and exists largely north of 54 degrees North latitude. As its name suggests, the BWBS comprises upland boreal forests and muskegs, with a continental climate influenced by both arctic and polar air masses. Winters are long and cold, and growing seasons are short in the BWBS zone (DeLong et al., 2011).
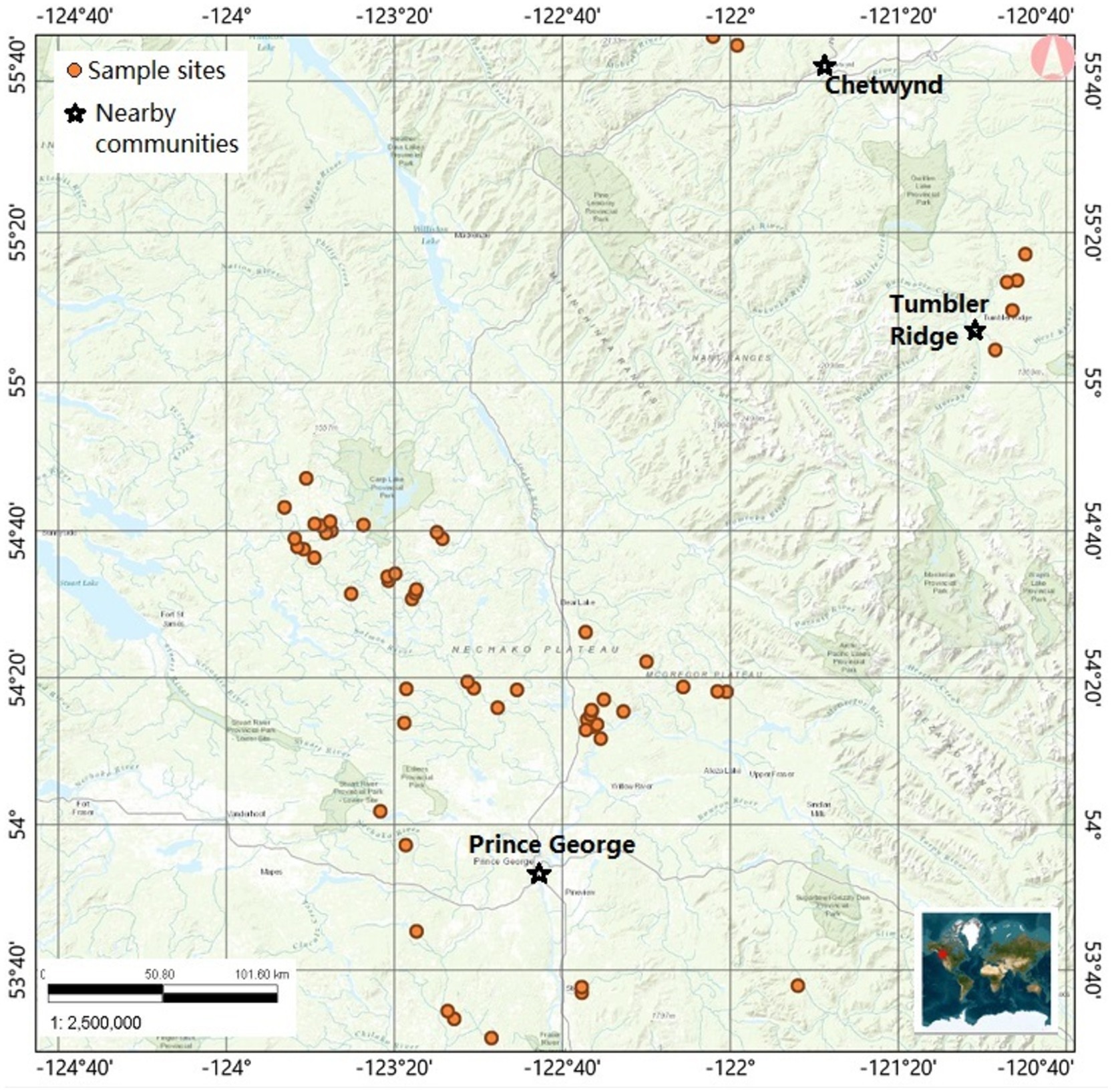
Figure 1. Map of sampled sites, where plants were collected for analysis of nutrients. Glyphosate based herbicides were applied in some of these locations. Samples were obtained from forested cutblocks treated with glyphosate-based herbicides, and from nearby untreated control blocks.
2.2 Experimental design and sampling
Shoot samples were collected from three species of plants chosen primarily for their importance to the diet of moose (Alces alces), a prominent herbivore of cultural importance in North America (Renecker and Schwartz, 1998), and due to their prevalence across the northern BC landscape: Chamaenerion angustifolium (L.) Scop. (fireweed), Cornus sericea L., syn. C. stolonifera (red-osier dogwood), and Salix species (representatives of the willow genus). Fireweed is an herbaceous perennial with rhizome-like roots and 0.5–3 m tall stems and is especially common in disturbed areas and open forests (MacKinnon et al., 1999). Fireweed is consumed by wildlife, including both moose (Broderick, 1990) and bears (Ursus sp.) (Ciarniello, 2018). Red-osier dogwood is a stoloniferous shrub 1–4 m tall, growing in moist soils (MacKinnon et al., 1999). Its shoots are an extremely important winter food source for moose (Zach et al., 2011), and the berries are an important food for many songbirds and black bears (Noyce and Garshelis, 2011; Benson and Chamberlain, 2006). Finally, willow is a 0.5–5 m tall shrub or small tree common at low to moderate elevations throughout northern BC, growing in upland forests, lowland thickets, riparian zones, swamps, muskeg, and on disturbed sites (MacKinnon et al., 1999). Willow species readily hybridize, and therefore genus was used as the defining level for taxonomic identification. Willow is a staple diet item for moose (Shipley et al., 1998; Rea et al., 2015) and other herbivores (MacKinnon et al., 1999).
Shoot samples were all collected from operationally treated forestry cutblocks. Cutblocks are large openings created by the forest industry, where mature trees have been harvested and replaced by new seedlings. In order to remove competition pressure from the newly growing crop trees, these cutblocks are often treated with GBH within the first 10 years of growth. Most shoot samples were collected within cutblocks over a 10-day period from July 5 to 15, 2018 where VisionMax® (Canadian registration no. 27736 under the Pest Control Products Act), a GBH, was aerially applied at rates of 3.3–4.0 L ha−1 (resulting in concentrations of 1.78–2.16 kg a.i. ha−1). Note that the dose of the treatments did not vary experimentally, the dose was determined by the forest industry, and we visited these cutblocks in years after they were sprayed with GBH to measure the effects of treatments on plant nutrients. Treatments identified for this experiment (sprayed areas) all received the same concentration of herbicide, but were measured at different intervals post-treatment, as follows:
• T1: cutblocks were treated 1 year prior to sampling (treated in 2017),
• T2: cutblocks were treated 3 years prior to sampling (treated in 2015),
• T3: cutblocks were treated 6 years prior to sampling (treated in 2012), and.
• T4: cutblocks were treated 12 years prior to sample collection (treated in 2006).
Additional samples were collected in 2021 (one-year post-treatment) as part of a different study, from cutblocks sprayed in 2020 with 6.0 L/ha of GBH GlySil®, Canadian registration no. 29009 under the Pest Control Products Act (resulting concentration of application was 2.13 kg a.i. ha-1). These additional samples were also collected in July and were collected in the same manner as those previously collected. The additional samples were added to the T1 dataset obtained in 2018 to create a more robust dataset.
Within each cutblock only one composite plant sample of each species was collected. Separate and corresponding control samples were collected from cutblocks of the same age (trees were harvested in the same year) that were never treated with herbicide. A total of 59 cutblocks were sampled, 10 of these contained control and sprayed (treated) areas; 12 blocks were untreated controls, and 37 were GBH treated cutblocks.
To create each composite plant sample from within each cutblock, a systematic random plot vector sampling design was used. Five plots were placed 100 m apart. The first plot was placed at least 20 m from the cutblock boundary (or treatment boundary in the case of GBH treated cutblocks), and each subsequent plot was 100 m apart. The direction of the next plot in the vector was systematically determined based on the shape of the cutblock/sprayed area to avoid shaded edges of the cutblock boundaries. Within each plot, one to three individuals of each of the targeted species were collected. Plants collected from all plots within a cutblock were combined to form one composite sample containing tissues from a minimum of five individual plants of the same species from the cutblock. It should be noted that not all species were found within each plot, or within each cutblock, and therefore our sampling numbers are not even across the species and intervals (Table 1). Plants were randomly selected within the plots, and where possible, plants with obvious signs of poor health (other than symptoms of glyphosate stress) were avoided. All sampling plots were flat (< 4% slope), ranged in elevation from 760 to 890 m asl, and were a minimum of 30 m from the edge of the treatment zone. The outer 20 cm length of willow and dogwood shoot tips were clipped and bagged, that portion that would be theoretically consumed by browsing moose, while the entire above-ground portions of fireweed plants were collected. Plant samples were frozen in sealed plastic bags until processed. Since plant age can affect plant nutrient content (Spaeth et al., 2002), vegetation age was taken as zero at the time of logging. All sampling sites were treated 4–6 years after logging. Control sites were chosen to match the age of the vegetation in treated sites.
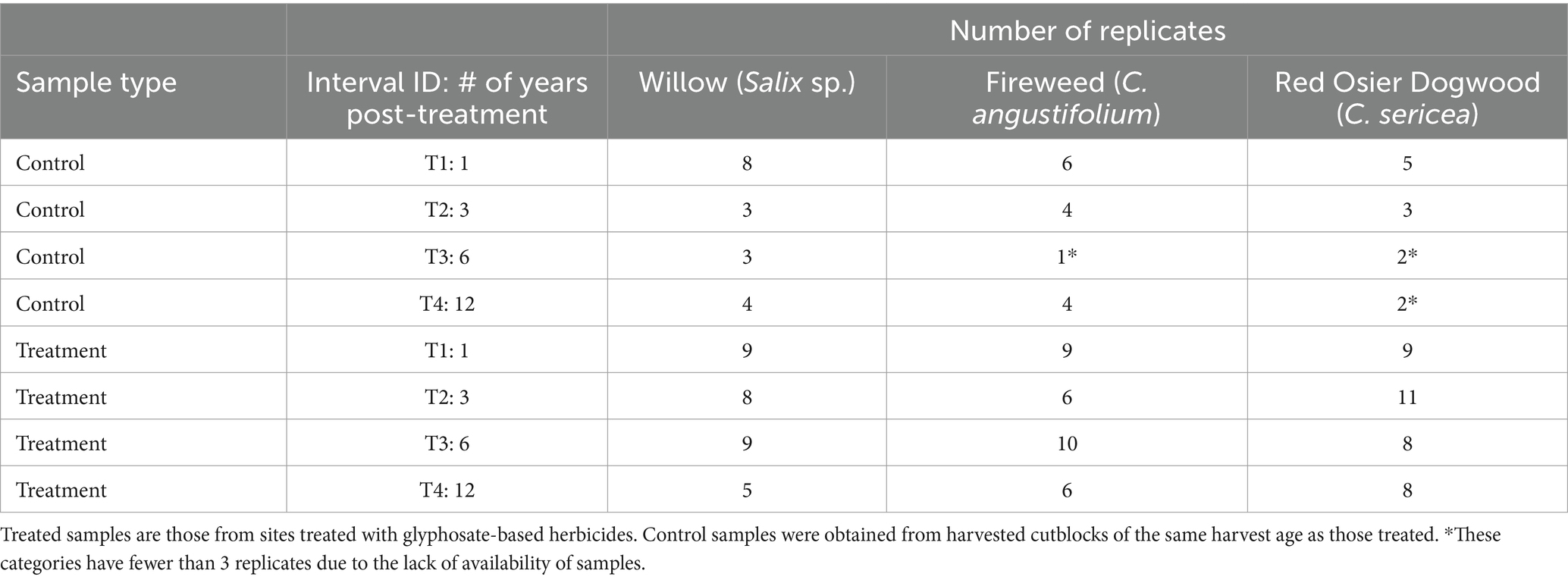
Table 1. Composite plant samples collected and analyzed by species and interval, from areas of Northern British Columbia, Canada.
2.3 Sample processing and laboratory analysis
A total of 143 composite plant samples were prepared and analyzed, 45 controls and 98 GBH treated samples (Table 1). Sample portions analyzed for residue content were individually washed with a minimum of three rinses to remove all traces of soil, oven dried at 80°C and ground to a powder (0.8–0.5 mm particle size). Grinders were blown clean with forced air between samples of the same treatment and species from the same interval and washed with soap and water and dried between samples of different exposure (sprayed or control), species or intervals. Ground samples were sent to the University of Guelph Agriculture and Food Laboratory for analysis of glyphosate and aminomethyl phosphonic acid (AMPA), one of glyphosate’s primary metabolite products, using high performance liquid chromatography – tandem mass spectrometry (HPLC-MS/MS).
Full elemental analysis of plant nutrients was conducted by the BC Ministry of Environment and Climate Change Strategy’s Analytical Chemistry Services Laboratory (BC MoE) or by the Northern Analytical Chemistry Lab at the University of Northern BC (NALS). Samples were washed, freeze-dried at −80°C, and ground to pass a 1 mm screen. Elemental nutrient concentrations were determined at BC MoE using closed vessel microwave digestion HNO3 and HCl digestion for inductively coupled plasma mass spectrometry (ICP-MS), using the U.S. Environmental Protection Agency’s EPA 3051A and 6020A reference methods. Samples tested at NALS were analyzed for elemental nutrient concentrations using inductively coupled plasma optical emission spectroscopy (ICP-OES). We statistically analyzed B, Ba, Ca, Cu, Fe, Mg, Mn, Na, Ni, and Zn for differences in mean, distribution and variation across treatments.
2.4 Statistical analysis
The detection limit for residue data was 0.008 μg g−1, and the quantification limit was 0.03 μg g−1. Where the presence of glyphosate was detected by HPLC-MS, but at less than these amounts, “< MDL” = less than the Minimum Detection Limit and “< MQL” = less than the Minimum Quantification Limit categories were assigned. To include these qualitative results as detected numeric quantities in the analyses, “not detected” was given a value of zero, “< MDL” values were taken as 0.004 μg g−1 (median between 0.000 and 0.008) and “< MQL” values were taken as 0.019 μg g−1 (median between 0.008 and 0.03).
Data were normalized for visual comparisons between variables at the same scale. Histograms, Shapiro–Wilk tests and skewness/kurtosis tests were used to test glyphosate, AMPA, and nutrient data (individually) for normality, which demonstrated in most cases that the data were not normally distributed and skewed to the left. Therefore, only nonparametric statistical tests were used, including independent sample Mann–Whitney and Median tests for comparison between groups of data. GBH-treated and control samples were compared over all combinations of species and intervals (T1-T4).
Binary logistic generalized linear models with a binomial probability distribution and a logit link function were used to predict treatment (control or GBH sprayed), where the procedure modeled 1 (GBH sprayed) as the response and 0 (control) as the reference category. Each predictor variable was tested independently in the model for its significance in predicting treatment following the method suggested by Ranganathan et al. (2017). Following this, the possible predictor variables that were continuous were tested using a Spearman’s rho correlation analysis. Variables that were significantly correlated to one another were not added to any model together, rather the variable that was most significant in predicting treatment was used. Variables that were significant in predicting GBH treatment individually were added one at a time to the model to observe their impact on model fit. After the significant and unrelated continuous predictor variables were identified, categorical factors were added as predictors to the model, one at a time. AIC and BIC (AIC, Akaike’s Information Criterion; BIC, Bayesian Information Criterion), likelihood ratio chi-square value, and p-values for each predictor variable were assessed to determine the best model.
Correlation analyses were conducted to determine the relationship between variation in nutrient concentrations and variation in glyphosate concentrations, both continuous data sets listed by sample number, using Spearman’s rank correlation. The concentration of each nutrient was correlated to the concentration of glyphosate and AMPA in that sample, to determine if the variation across sample nutrients matched that of glyphosate or AMPA. All statistical analyses were conducted with IBM SPSS Statistical Software version 28.0, and α of 0.05 was used to assess significance throughout.
3 Results
3.1 Glyphosate and AMPA concentrations in plant tissues
Glyphosate was detected in 24 of the 27 T1 samples (88.9%) (Figure 2). Only 5 of these 27 samples were below the MQL; concentrations across all T1 samples ranged from not detected to 1.10 μg g-1, with a mean of 0.20 μg g-1. Fireweed shoots contained the lowest concentrations of glyphosate (highest detected amount was a sample containing 0.070 μg g−1). Where T1 dogwood shoots contained concentrations of glyphosate, these ranged from 0.12 to 0.89 μg g−1, and where T1 willow shoots contained glyphosate residues, concentrations ranged between 0.063 and 1.10 μg g−1 (Figure 2). Ten samples also contained traces of AMPA residues; all of which were in the T1 interval and 6/10 of which were willow samples. The general pattern of AMPA residue presence mimics that of glyphosate residue, with the highest amounts found in willow shoots, then red osier dogwood, and lowest amounts found in treated fireweed shoots. Nine out of 25 T2 samples (36%) contained glyphosate, and six out of 27 T3 samples (22%) contained glyphosate (fireweed and willow only). Glyphosate was not detected in any T4 samples. Six of the 45 control samples tested for residues contained detectable glyphosate. This could be result of cross-contamination during sample preparation and analysis despite our best efforts to control contamination between samples. However, these detections could also have resulted from overspray of treatment areas into control portions of cutblocks. Of all the treated samples, 39 out of 98 (39.8%) contained levels of glyphosate above 0.03 μg g−1.
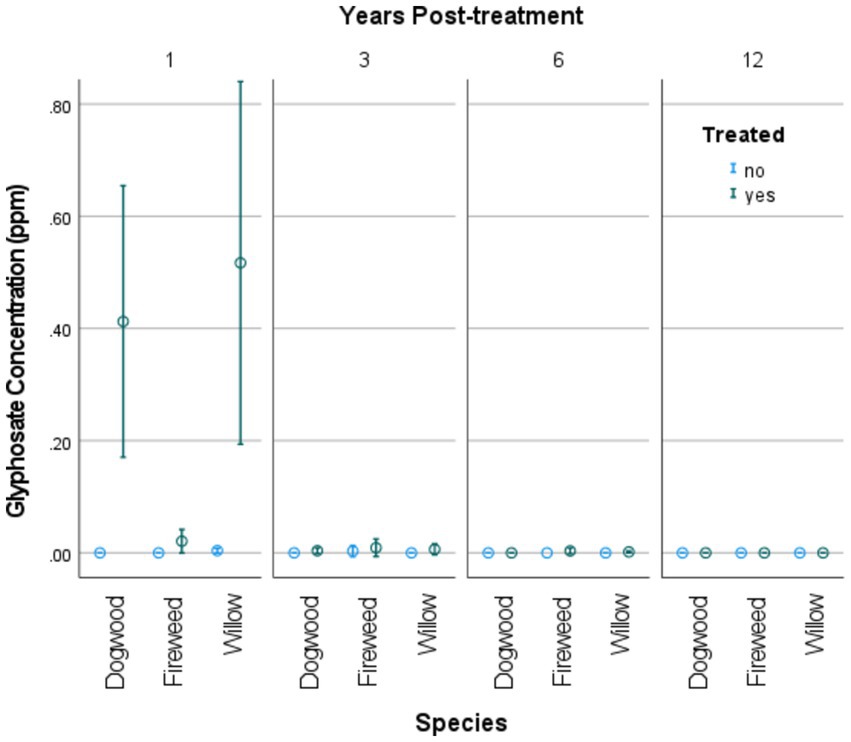
Figure 2. Concentration of glyphosate residues found in plant shoot tissues of red osier dogwood (Cornus stolonifera) = Dogwood, willow (Salix sp.), and fireweed (Chamaenerion angustifolium) shoots, at intervals post-treatment (with 95% CI). Treatments were with glyphosate-based herbicides in operational forestry cutblocks of northern BC, Canada.
3.2 Plant nutrient concentrations after GBH exposure
Out of the 10 nutrients analyzed, Ca and Na were the only nutrients to show no significant differences in median and distribution between control and GBH sprayed groups across all categories (Table 2). Significant differences between sprayed and control groups were most pronounced in T1 (year 1 post-treatment) for red osier dogwood and fireweed (with most nutrients flagged as significantly different in both median and distribution), and in T4 (year 12) for willow. The only similarities across species were in T1 and T4 (Years 1 and 12 post-treatment). Both Cu and Zn were significantly different in medians and distributions in T1 dogwood samples (Cu: median p = 0.021; Mann–Whitney p = 0.004, Zn: median p = 0.021; Mann–Whitney p < 0.001) and fireweed samples (Cu: median p = 0.007; Mann–Whitney p = 0.026, Zn: median p = 0.007; Mann–Whitney p = 0.002). Boron was significantly different in medians and distributions in T4 fireweed samples (median p = 0.048; Mann–Whitney p = 0.019) and willow samples (median p = 0.048; Mann–Whitney p = 0.032). Cu, Mn and B most frequently showed significant differences; these nutrients showed at least one difference between control and GBH treated in all species. Significant differences were shown in Mn in T1 fireweed samples (median p = 0.007; Mann–Whitney p < 0.001), in Zn in T4 fireweed samples (median p = 0.048; Mann–Whitney p = 0.010), in Mg in T3 willow samples (median p = 0.045; Mann–Whitney p = 0.018), and in Mn in T4 willow samples (median p = 0.048; Mann–Whitney p = 0.032) (Table 2).
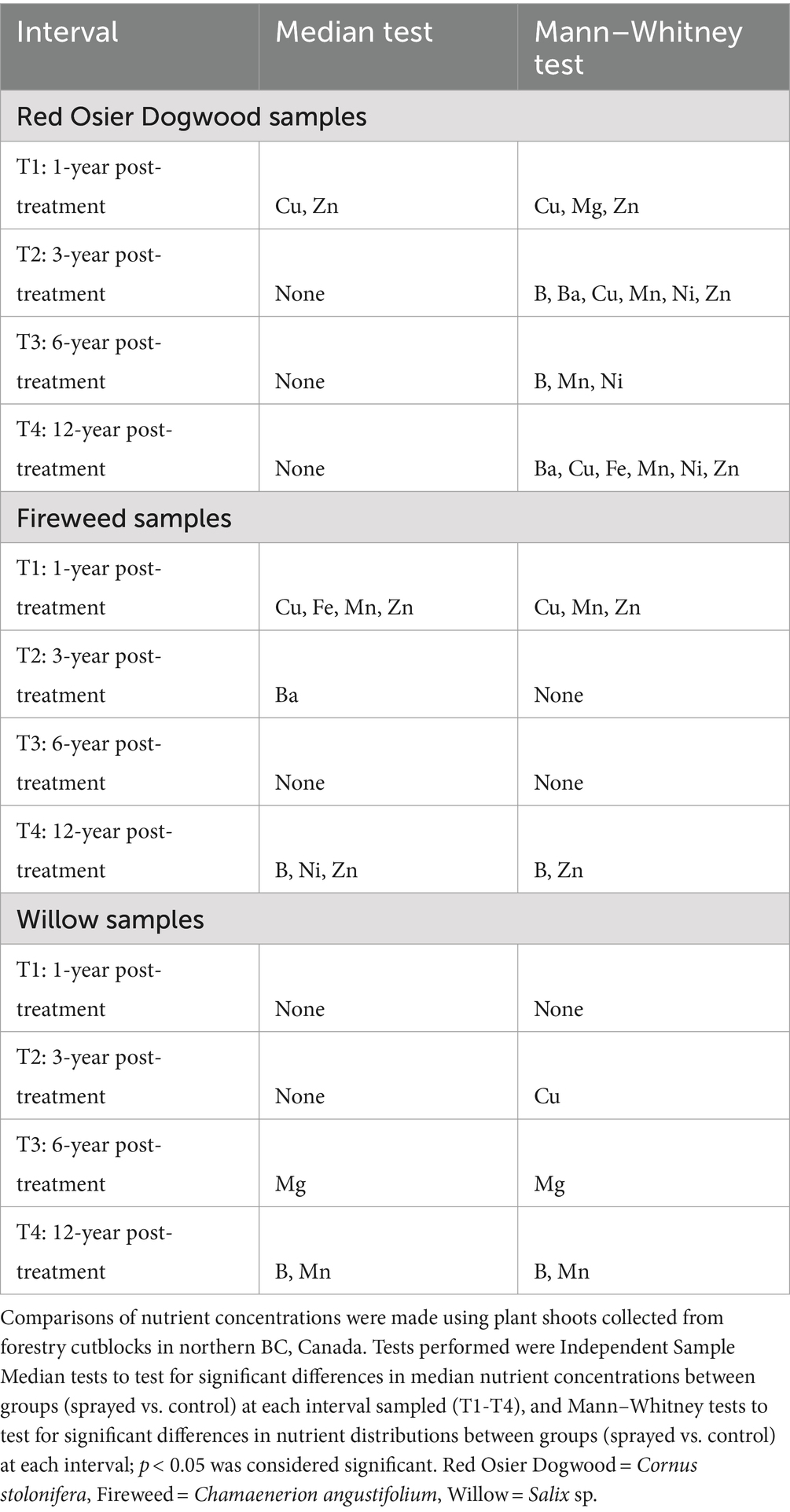
Table 2. Nutrients with significant differences between cutblocks that were “sprayed” (treated with glyphosate-based herbicides) or “control” (not treated).
In every case where a nutrient was found to be significantly different in both median and distribution between controls and GBH treated samples (Table 2), the nutrients were shown to increase with the application of GBH (T1 trends shown in Figure 3); except for T3 in willow, where Mg decreased with treatment. We observe in Figure 3 that T1, in dogwood B, Cu, Mn, Na, and Zn increase with treatment, while Ca, Fe, Mg, and Ni decrease with treatment. In fireweed we observe that Cu, Mn, and Zn increase with treatment, while Fe and Na decrease; and in willow we observe that Mn increases with treatment and Fe, Na and Ni decrease with treatment. Mn is the only nutrient that consistently increases with GBH treatment in T1, and Fe is the only nutrient that consistently decreased with treatment in T1 over all species. In most cases there was less variability in the GBH treated samples nutrient concentrations, when compared to the control samples (Figure 3).
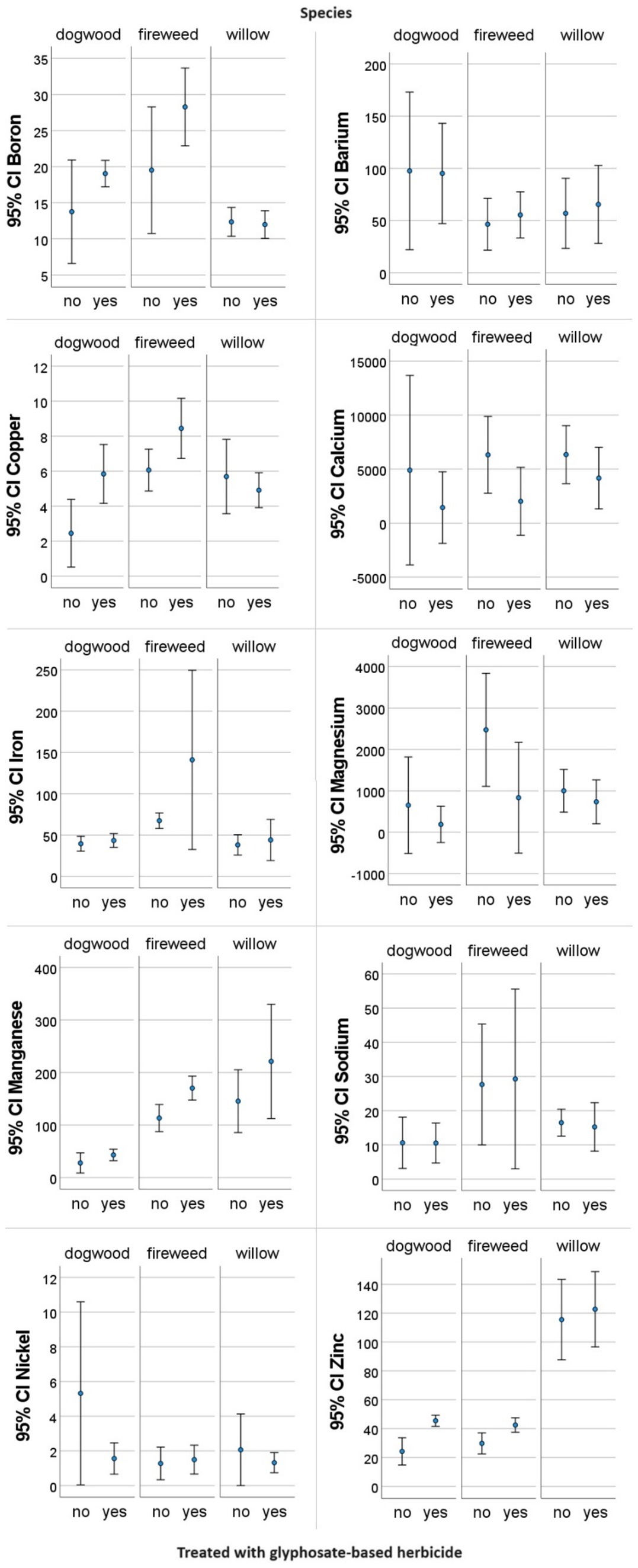
Figure 3. Nutrient concentrations measured in plant shoot tissues of three forest species: dogwood (Cornus sericea), fireweed (Chamaenerion angustifolium), and willow (Salix sp.), in cutblocks sprayed with glyphosate-based herbicide (yes) one-year after treatment (T1) and control/untreated areas (no) of the same stage of maturity in northern British Columbia, Canada. Confidence Intervals (CI) are provided for each of the mean data points illustrated. Note: scales on individual nutrient graphs are different from one another.
Out of nutrients analyzed, B, Ca, Mg, and Ni were individually significant in predicting GBH sprayed areas (Table 3). B was added to the model first as the most significant individual predictor of the presence of spray. The only nutrients that were not significantly correlated to B were Ca, Zn, and Mn. These were added to the model individually, however Zn and Mn were correlated to one another (rho = 0.550, p < 0.001), so the most significant of the two in the model (Mn) was kept in the final iteration. Species was added as a significant factor; interval was insignificant in the model when added (Table 3). All other variables were highly related to the response variable (presence or absence of GBH spray treatment) and it would have been illogical to include them as predictors because they were dependent on the treatment itself and not applicable to the controls (ie. herbicide formula, glyphosate residue concentration, and AMPA residue concentration). For example, it would be irrelevant to try to predict if an area was treated if one already knew what herbicide formula was applied. The model that was found to be the best fit for predicting GBH treatment was based on predictor variables B, Ca, Mn, and Species (model #8 in Table 3). This model had the best combination of overall significance, AIC and BIC values, and each of the independent variables was significant. The final model expression, following methods by Ranganathan et al. (2017), for the best fit model was:
Loge (odds of GBH Treatment (control or GBH sprayed)) = −3.92924 + (0.32606 × B) + (0.00025 × Ca) + (0.27956 × dogwood) – (1.96930 × fireweed) + (0.00730 × Mn).
For model verification, treatment categories (spray or control) were compared to the modeled outcome for model #8 (Table 3) and visually compared with a linear trend line. The predicted values were significantly correlated to the observed (rho = 0.559, p < 0.001; r2 = 0.392) indicating that the four predictor variables were able to capture approximately 39% of the variation in treatment occurrence.
3.3 Correlations between glyphosate concentrations and plant nutrients
Statistically significant positive correlations were found between glyphosate concentration and each of Mn and Zn when GBH exposure, all species, and intervals (T1-T4) were considered. Significant negative correlations were found between glyphosate concentration and Ba, Ca, Mg, and Ni (Table 4).
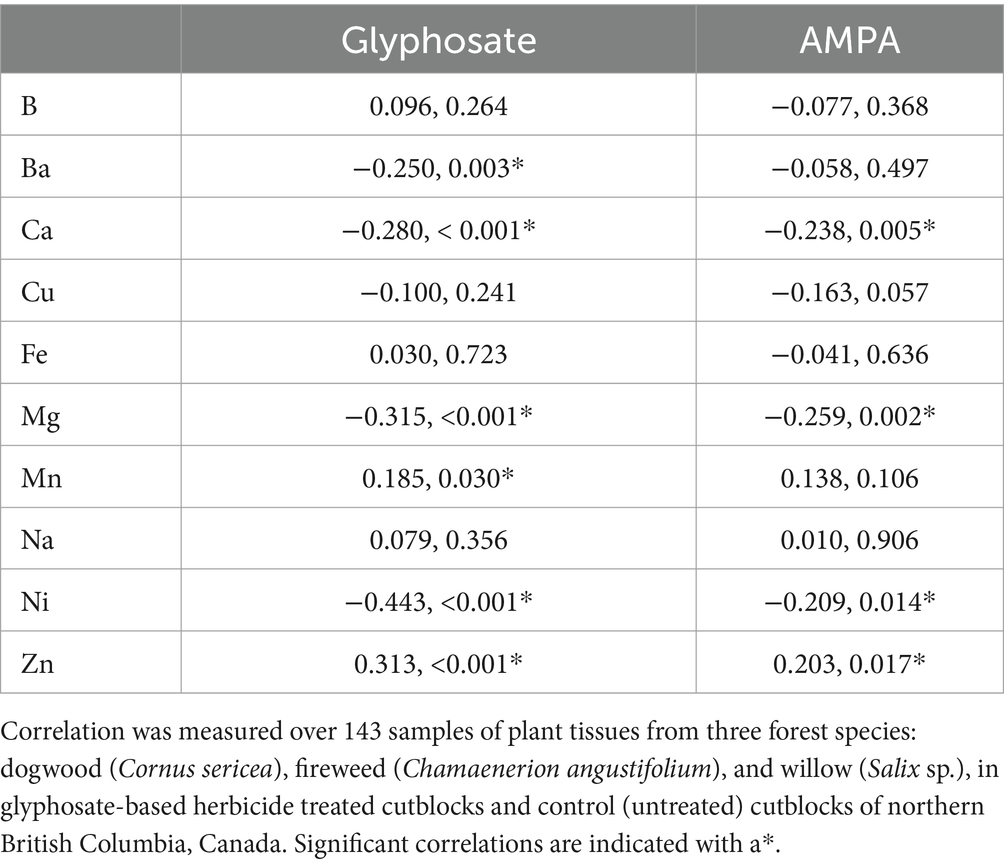
Table 4. Spearman’s correlation coefficients, and p-values, for the relationships between the variation in the concentration of each nutrient listed and the variation in glyphosate residue and AMPA concentrations.
When considering glyphosate chelation, it should be noted that in most cases in T1, the plant tissue concentration of glyphosate was much lower in magnitude than the concentration of the nutrient being considered.
4 Discussion
4.1 Glyphosate and AMPA concentrations in plant tissues
Glyphosate residue is present in plants for years after applications in our study region (Botten et al., 2021). GBH treatments alter vegetation complexes, creating changes in nutrient cycling and water availability. The ecological consequences of a drastic change to vegetation, such as that which is experienced after GBH treatment, include changes to soil water availability and temperature, altered electrical conductivity and ion flow, and exposure to increased solar radiation for surviving plants—all of which have been clearly documented in forest harvesting studies (Likens et al., 1970; Lousier, 1990). Each of these environmental changes can influence nutrient availability (Likens et al., 1970; Grand et al., 2014) and uptake, as well as plant stress responses, which likely lead to changes in how nutrients are used and stored by plants (Kozlowski and Pallardy, 2002; Marschner, 1974; Costa et al., 2024). Therefore, we deduce that changes to nutrients from GBH treatments are likely, but complex and difficult to pattern across space, time, and species. Reduced plant diversity, such as that associated with conifer release, has been linked with altered nutrient ratios and increased variation of nutrient composition in plants (Abbas et al., 2013).
Possible inherent differences exist between forestry cutblocks targeted for herbicide applications, and those left untreated (which we used as controls). Generally, we expect blocks targeted for treatment to exhibit soil nutrient and moisture regimes more conducive to plentiful shrub and forb growth. On the other hand, factors such as historic site preparation, the timing and species of crop trees planted, and the predisposition of the license holder to use GBH will also have influenced the distribution of treatment and controls available for study. These potential sources of confounding variation could not be separated in our analyses. The benefit of studying the effects of GBH application in an operational context is that it reflects what we experience in the real world of forestry in BC. Future studies will benefit from a more formal experimental design.
4.2 Plant nutrient concentrations after GBH exposure
Our results support the hypothesis that GBH treatments influence the concentrations of metal nutrients in perennial forest plant tissues, albeit in ways that do not show a straightforward pattern. The complexity in studying nutrients as they relate to GBH within plant tissues has also been noted in other studies (Werner et al., 2022) and is due to the highly responsive and plastic nature of nutrient availability, uptake, movement, interaction with plant chemicals and use/role in physiological processes at any given time (Mertens et al., 2018). Likely, this is why a higher success in teasing apart trends in nutrients within plant tissues has been obtained in controlled greenhouse or lab studies such as those presented by Bernards et al. (2005), Barrett and McBride (2006), or Cakmak et al. (2009).
In our field study, most nutrients showed significant differences between control and treated samples in at least one interval out of the four intervals studied (T1–T4), and when we modeled treatment based on nutrients, we found that B, Ca, Mg, Mn, and Ni were significant predictor variables. Furthermore, six nutrients showed significant correlations between glyphosate residue and nutrient concentrations across the whole dataset. These relationships indicate that GBH treatments are likely having an impact on nutrient concentrations.
Nutrients interact with glyphosate in unique ways. Duke et al., 2012 reviewed the literature on effects of glyphosate applications on plant nutrients and reported that reductions and impediments in Ca, Mn, Mg, and Fe within plant tissues were most common. Some of this is consistent with our findings, although the results reviewed in Duke et al. (2012) are limited to agricultural plants which may not be comparable to native boreal plants; we noted that species was a prominent factor in modeling nutrients in our study. Glyphosate-induced changes in certain plant nutrients can also indirectly affect other nutrients. For example, reduced Ni caused by glyphosate has been reported to affect nitrogen (N2) fixation in plants, since Ni is essential for N2-fixing microorganisms (Zobiole et al., 2010), while high Ni in plants may reduce Fe and Mn concentrations (Hewitt and Smith, 1975).
We see a strong effect of species in predicting most nutrient levels, which is not surprising. Each focal species is genetically unique, with separate phenology and perennation strategies that have different requirements for building and maintaining tissues. Fireweed is an herbaceous perennial, while dogwood and willow are woody shrubs, each with different root structures, rooting depth, and absorption capabilities (Cataldo and Wildung, 1978). Furthermore, mycorrhizal symbionts unique to each species which influence plant nutrient access and uptake, may also be variously affected by glyphosate (Zaller et al., 2018). Wood (2019) demonstrated differences in persistence of glyphosate across plants with different life strategies, and we observe the same trend. Physical damage to plants surviving GBH treatment, including loss of foliage, malformed regrowth, and associated physiological responses such as reduced photosynthesis and increased oxidative stress, may also alter nutrient levels (Gomes et al., 2014; Timms and Wood, 2020).
Our hypothesis was only partly correct: nutrients do change with GBH treatments in many cases, but this effect does not necessarily diminish with time. Nutrients measured in T1 (year one post-treatment) showed the largest number of significant differences in median and distribution between control and sprayed groups in fireweed and dogwood, but the opposite was true for willow. Data for willow suggested that nutrient concentrations in their tissues may continue to be altered over a longer period. Willow is often used for remediation purposes due to its great ability to take up and store metals (Landberg and Greger, 2022; Licinio et al., 2022) and it has also been shown to remove glyphosate from soils (Gomes et al., 2016). It is possible that willows are accumulating nutrients at a different rate, when compared to other species, accounting for differences between sprayed and control areas at year 12 post-treatment (T4). Werner et al. (2022) found that there was also a longer-term trend in changes to digestible protein and initial increases in the availability of organic nitrogen in these spaces. It is possible that there is a short-term nutrient flux that is evident one-year post-treatment, owing to reduced competition and an increased supply of decaying material (evidenced by the increase in nutrients found in treated areas at that time), and then a longer-term effect caused by the changes to overall vegetation structure and soils.
4.3 Correlations between glyphosate concentrations and plant nutrients
Considering that there was a large difference in magnitude between glyphosate and metal ion concentrations found in this study, as is often the case (Duke et al., 2012), it does not seem feasible that glyphosate chelation within plant tissues could have been solely responsible for the significant changes observed in nutrient concentrations aside from Ni and possibly Zn, both of which were present in relatively low concentrations. It was impossible to determine whether metal nutrients were chelated in soil, in plants, or not at all. The digestion stage of the analysis of metal nutrients separated metal ions from all organic compounds, including glyphosate, to determine total metal content (USEPA, 1994); metals that were complexed with glyphosate were therefore detected as present in the sample, regardless of whether they were biologically available to plants in their complexed form. The capacity for chelation by glyphosate is limited by the number of metal ions that can bind to each glyphosate molecule. Although the glyphosate molecule has three functional groups to which metal ions may bind, calculations of stability constants of glyphosate-metal complexes generally assume either a 1:1 or 2:1 glyphosate:metal ratio (Caetano et al., 2012). Although this study lacks an analysis of soil nutrient concentrations, Mertens et al. (2018) came to the same conclusion regarding the likelihood of a significant direct long-term effect of glyphosate on metal bioavailability in soils. Whether or not chelation with glyphosate had a significant effect, various other factors most certainly influenced the changes observed in plant nutrient concentrations, which is why we see only low correlations between plant nutrient concentrations and glyphosate residue concentrations.
5 Conclusion
GBH treatment resulted in significant but not necessarily consistent or predictable alterations to nutrient availability in the plant species studied. Species was a large factor influencing nutrient concentrations. Treatment did not impact all nutrients, and this varied by species. Higher glyphosate concentrations were correlated with higher concentrations of Mn across all samples and treatments. Fireweed and dogwood seemed to show greater changes in nutrients due to treatment in the shorter term (year one: T1), whereas willow showed greater changes over the long-term (year 12: T4), perhaps related to its ability to uptake and store metal nutrients. In most cases, at year one post-treatment (T1), nutrients were shown to increase with the treatment. Some nutrients were significant in predicting the presence of GBH spray treatments, providing further evidence of a relationship between nutrient variability and applications of GBH.
Within the scope of this study, it is not possible to determine the precise cause(s) of changes in plant nutrients in GBH treated areas. Altered nutrient bioavailability due to chelation by glyphosate is one possible explanation for small changes to nutrient levels but is unlikely to be the main cause of the nutrient fluctuations we observed, simply due to the low concentrations of residue present in plants. Multiple other factors, some resulting from GBH application, and others because of differences in species response, likely affect plant nutrient concentrations. These include changes to plant environmental exposure, plant perennation type, rooting depth, root absorption, and existing mycorrhizal associations.
Data availability statement
The raw data supporting the conclusions of this article will be made available by the authors, without undue reservation.
Author contributions
LW: Conceptualization, Formal analysis, Funding acquisition, Methodology, Project administration, Resources, Supervision, Writing – review & editing. NB: Data curation, Formal analysis, Investigation, Methodology, Writing – original draft. AF: Resources, Supervision, Validation, Writing – review & editing. JW: Investigation, Methodology, Resources, Supervision, Validation, Writing – review & editing.
Funding
The author(s) declare that financial support was received for the research, authorship, and/or publication of this article. This research was funded through the Natural Sciences and Engineering Research Council of Canada, with support from West Fraser Mills Chetwynd Forest Industries and BC Timber Sales. Further funding was provided by Habitat Conservation Trust Foundation. In kind support was provided by British Columbia Ministry of Forests, who assisted in sample collection.
Acknowledgments
Authors would like to acknowledge BC Timber Sales and West Fraser Chetwynd Forest Industries for their support of this research.
Conflict of interest
The authors declare that the research was conducted in the absence of any commercial or financial relationships that could be construed as a potential conflict of interest.
Publisher’s note
All claims expressed in this article are solely those of the authors and do not necessarily represent those of their affiliated organizations, or those of the publisher, the editors and the reviewers. Any product that may be evaluated in this article, or claim that may be made by its manufacturer, is not guaranteed or endorsed by the publisher.
References
Abbas, M., Ebeling, A., Oelmann, Y., Ptacnik, R., Roscher, C., Weigelt, A., et al. (2013). Biodiversity effects on plant stoichiometry. PLoS One 8:e58179. doi: 10.1371/journal.pone.0058179
Barrett, K. A., and McBride, M. B. (2006). Trace element mobilization in soils by glyphosate. Soil Sci. Soc. Am. J. 70, 1882–1888. doi: 10.2136/sssaj2005.0415
Baylis, A. D. (2000). Why glyphosate is a global herbicide: strengths, weaknesses and prospects. Pest Manag. Sci. 56, 299–308. doi: 10.1002/(SICI)1526-4998(200004)56:4<299::AID-PS144>3.0.CO;2-K
Benbrook, C. M. (2016). Trends in glyphosate herbicide use in the United States and globally. Environ. Sci. Eur. 28:3. doi: 10.1186/s12302-016-0070-0
Benson, J. F., and Chamberlain, M. J. (2006). Food habits of Louisiana black bears (Ursus americanus luteolus) in two subpopulations of the Tensas River basin. Am. Midl. Nat. 156, 118–127. doi: 10.1674/0003-0031(2006)156[118:FHOLBB]2.0.CO;2
Bernards, M. L., Thelen, K. D., Penner, D., Muthukumaran, R. B., and McCracken, J. L. (2005). Glyphosate interaction with manganese in tank mixtures and its effect on glyphosate absorption and translocation. Weed Sci. 53, 787–794. doi: 10.1614/WS-05-043R.1
Botten, N., Wood, L. J., and Werner, J. R. (2021). Glyphosate remains in forest plant tissues for a decade or more. For. Ecol. Manag. 493:119259. doi: 10.1016/J.FORECO.2021.119259
Broderick, D. H. (1990). The biology of Canadian weeds.: 93. Epilobium angustifolium L. (Onagraceae). Can. J. Plant Sci. 70, 247–259. doi: 10.4141/cjps90-027
Caetano, M. S., Ramalho, T. C., Botrel, D. F., da Cunha, E. F. F., and de Mello, W. C. (2012). Understanding the inactivation process of organophosphorus herbicides: a DFT study of glyphosate metallic complexes with Zn2+, Ca2+, Mg2+, Cu2+, Co3+, Fe3+, Cr3+, and Al3+. Int. J. Quantum Chem. 112, 2752–2762. doi: 10.1002/qua.23222
Cakmak, I., Yazici, A., Tutus, Y., and Ozturk, L. (2009). Glyphosate reduced seed and leaf concentrations of calcium, manganese, magnesium, and iron in non-glyphosate resistant soybean. Eur. J. Agron. 31, 114–119. doi: 10.1016/J.EJA.2009.07.001
Cataldo, D. A., and Wildung, R. E. (1978). Soil and plant factors influencing the accumulation of heavy metals by plants. Environ. Health Perspect. 27, 149–159. doi: 10.1289/ehp.7827149
Cederlund, H. (2017). Effects of spray drift of glyphosate on nontarget terrestrial plants—a critical review. Environ. Toxicol. Chem. 36, 2879–2886. doi: 10.1002/etc.3925
Ciarniello, L. (2018). A review of food security for grizzly bears in British Columbia. Technical report. Vancouver, BC: The Grizzly Bear Foundation.
Costa, M. G., Prado, R., Palaretti, L. F., de Souza, P., and Júnior, J. (2024). The effect of abiotic stresses on plant C:N:P homeostasis and their mitigation by silicon. Crop J. 11, 2214–5141. doi: 10.1016/j.cj.2023.11.012
DeLong, C., Banner, A., MacKenzie, W. H., Rogers, B. J., and Kaytor, B. (2011). A field guide to ecosystem identification for the boreal white and black spruce zone of British Columbia. Vancouver, BC: Province of British Columbia Forest Science Program.
Divisekara, T., Navaratne, A. N., and Abeysekara, A. S. K. (2018). Impact of a commercial glyphosate formulation on adsorption of cd(II) and Pb(II) ions on paddy soil. Chemosphere 198, 334–341. doi: 10.1016/j.chemosphere.2018.01.155
Dost, F. N. (2003). Toxicology and potential health risk of chemicals that may be encountered by workers using forest vegetation management options: Part IV, risk to workers using glyphosate formulations. Victoria: Forest Practices Branch, BC Ministry of Forests.
Duke, S. O., Lydon, J., Koskinen, W. C., Moorman, T. B., Chaney, R. L., and Hammerschmidt, R. (2012). Glyphosate effects on plant mineral nutrition, crop rhizosphere microbiota, and plant disease in glyphosate-resistant crops. J. Agric. Food Chem. 60, 10375–10397. doi: 10.1021/jf302436u
Edge, C. B., Ramadoss, M., Brown, M. I., Heartz, S., Thompson, D., and Ritter, L. (2021). The persistence of glyphosate in vegetation one year after application. Forests 12:601. doi: 10.3390/F12050601
Eker, S., Ozturk, L., Yazici, A., Erenoglu, B., Romheld, V., and Cakmak, I. (2006). Foliar-applied glyphosate substantially reduced uptake and transport of iron and manganese in sunflower (Helianthus annuus L.) plants. J. Agric. Food Chem. 54, 10019–10025. doi: 10.1021/JF0625196
Fadin, D. A., Tornisielo, V. L., Barroso, A. A. M., Ramos, S., Dos Reis, F. C., Florencia, F. M., et al. (2018). Effects of the herbicide glyphosate on non-target plant native species from Chaco forest (Argentina). Ecotoxicol. Environ. Saf. 144, 360–368. doi: 10.1016/j.ecoenv.2017.06.049
Golt, A. R., and Wood, L. J. (2021). Glyphosate-based herbicides alter the reproductive morphology of Rosa acicularis (prickly rose). Front. Plant Sci. 12:698202. doi: 10.3389/fpls.2021.698202
Gomes, M. P., le Manac’h, S. G., Moingt, M., Smedbol, E., Paquet, S., Labrecque, M., et al. (2016). Impact of phosphate on glyphosate uptake and toxicity in willow. J. Hazard. Mater. 304, 269–279. doi: 10.1016/j.jhazmat.2015.10.043
Gomes, M. P., Smedbol, E., Chalifour, A., Hénault-Ethier, L., Labrecque, M., Lepage, L., et al. (2014). Alteration of plant physiology by glyphosate and its by-product aminomethylphosphonic acid: an overview. J. Exp. Bot. 65, 4691–4703. doi: 10.1093/jxb/eru269
Grand, S., Hudson, R., and Lavkulich, L. M. (2014). Effects of forest harvest on soil nutrients and labile ions in Podzols of southwestern Canada: mean and dispersion effects, CATENA 122: 18-26. ISSN 122, 18–26. doi: 10.1016/j.catena.2014.06.004
Henderson, A. M., Gervais, J. A., Luukinen, B., Buhl, K., Stone, D., Strid, A., et al. (2019). Glyphosate technical fact sheet. Corvallis, OR: National Pesticide Information Center, Oregon State University Extension Services.
Jayasumana, C., Gunatilake, S., and Senanayake, P. (2014). Glyphosate, hard water and nephrotoxic metals: are they the culprits behind the epidemic of chronic kidney disease of unknown etiology in Sri Lanka? Int. J. Environ. Res. Public Health 11, 2125–2147. doi: 10.3390/IJERPH110202125
Kozlowski, T. T., and Pallardy, S. G. (2002). Acclimation and adaptive responses of woody plants to environmental stresses. Bot. Rev. 68, 270–334. doi: 10.1663/0006-8101(2002)068[0270:AAAROW]2.0.CO;2
Landberg, T., and Greger, M. (2022). Phytoremediation using willow in industrial contaminated soil. Sustain. For. 14:8449. doi: 10.3390/su14148449
Licinio, A., Laur, J., Pitre, F. E., and Labrecque, M. (2022). Willow and herbaceous species’ phytoremediation potential in Zn-contaminated farm field soil in eastern Québec, Canada: a greenhouse feasibility study. Plan. Theory 12:167. doi: 10.3390/plants12010167
Likens, G. E., Bormann, F. H., Johnson, N. M., Fisher, D. W., and Pierce, R. S. (1970). Effects of forest cutting and herbicide treatment on nutrient budgets in the Hubbard brook watershed-ecosystem. Ecol. Monogr. 40, 23–47. doi: 10.2307/1942440
Lloyd, R. (1990). Herbicide effects on moose browse in northern British Columbia. FRDA memo no. 161. Smithers, BC: BC Ministry of Forests.
Lousier, J. D. (1990). Impacts of Forest harvesting and regeneration on Forest sites. Smithers, BC: BC Ministry of Forests Land Management Report.
Machado, A. F. L., Ferreira, L. R., Santos, L. D. T., Santos, J. B., Ferreira, F. A., and Viana, R. G. (2009). Absorption, translocation and radicular glyphosate exudation in Eucalyptus sp. Clones. Planta Daninha 27, 549–554. doi: 10.1590/S0100-83582009000300016
MacKinnon, A., Pojar, J., and Coupe, R. (1999). Plants of northern British Columbia: Expanded. 2nd Edn. Edmonton, AB: Lone Pine Publishing.
Madsen, H. E., Christensen, H. H., Gottlieb-Petersen, C., Andresen, A. F., Smidsrod, O. A., Pontchour, C., et al. (1978). Stability constants of copper(II), zinc, manganese(II), calcium, and magnesium complexes of N-(Phosphonomethyl)glycine (glyphosate). Acta Chem. Scand. 32, 79–83. doi: 10.3891/ACTA.CHEM.SCAND.32A-0079
Mamy, L., Barriuso, E., and Gabrielle, B. (2016). Glyphosate fate in soils when arriving in plant residues. Chemosphere 154, 425–433. doi: 10.1016/j.chemosphere.2016.03.104
Marschner, H. (1974). Mechanisms of regulation of mineral nutrition in higher plants. Royal Soc. 12, 99–109.
Meidinger, D. V., and Pojar, J. (1991). Ecosystems of British Columbia, special report series 6. Victoria, BC: Research Branch, Ministry of Forests.
Mertens, M., Höss, S., Neumann, G., Afzal, J., and Reichenbecher, W. (2018). Glyphosate, a chelating agent—relevant for ecological risk assessment? Environ. Sci. Pollut. Res. 25, 5298–5317. doi: 10.1007/S11356-017-1080-1
Motekaitis, R. J., and Martell, A. E. (1985). Metal chelate formation by N-phosphonomethylglycine and related ligands. J. Coord. Chem. 14, 139–149. doi: 10.1080/00958978508073900
Noyce, K. V., and Garshelis, D. L. (2011). Seasonal migrations of black bears (Ursus americanus): causes and consequences. Behav. Ecol. Sociobiol. 65, 823–835. doi: 10.1007/s00265-010-1086-x
Ranganathan, P., Pramesh, C. S., and Aggarwal, R. (2017). Common pitfalls in statistical analysis: logistic regression. Perspect. Clin. Res. 8, 148–151. doi: 10.4103/picr.PICR_87_17
Rea, R., Hjeljord, O., and Gillingham, M. (2015). Factors influencing the use of willow and birch by moose in winter. Eur J Wildl Res. 61, 231–239. doi: 10.1007/s10344-014-0891-3
Renecker, L. A., and Schwartz, C. G. (1998). “Food habits and feeding behaviour” in Chapter 13 in ecology and Management of the North American Moose. ed. A. W. Franzmann (Washington, DC: Smithsonian Institution Press), 414–419.
Roy, D. N., Konar, S. K., Banerjee, S., Charles, D. A., Thompson, D. G., and Prasad, R. (1989). Uptake and persistence of the herbicide glyphosate (vision®) in fruit of wild blueberry and red raspberry. Can. J. For. Res. 19, 842–847. doi: 10.1139/x89-128
Shipley, L. A., Blomquist, S., and Danell, K. (1998). Diet choices made by free-ranging moose in northern Sweden in relation to plant distribution, chemistry, and morphology. Can. J. Zool. 76, 1722–1733. doi: 10.1139/z98-110
Spaeth, D. F., Bowyer, R. T., Stephenson, T. R., Barboza, P. S., and Van Ballenberghe, V. (2002). Nutritional quality of willows for moose: effects of twig age and diameter. Alces. 38, 143–154.
Subramaniam, V., and Hoggard, P. E. (1988). Metal complexes of glyphosate. J. Agric. Food Chem. 36, 1326–1329. doi: 10.1021/jf00084a050
Sundaram, A., and Sundaram, K. M. S. (1997). Solubility products of six metal:glyphosate complexes in water and forestry soils, and their influence on glyphosate toxicity to plants. J. Environ. Sci. Health B 32, 583–598. doi: 10.1080/03601239709373104
Székács, A., and Darvas, B. (2012). “Forty years with glyphosate” in Herbicides - properties, synthesis and control of weeds. ed. M. N. Hasaneen (Rijeka: BoD–Books on Demand), 247–284.
Thompson, D., Leach, J., Noel, M., and Odsen, S. (2012). Aerial forest herbicide application: comparative assessment of risk mitigation strategies in Canada. For. Chron. 88, 176–184. doi: 10.5558/tfc2012-034
Thompson, D. G., and Pitt, D. G. (2011). Frequently asked questions (FAQs) on the use of herbicides in Canadian forestry. Marie, ON: Canadian Forest Service, Great Lakes forestry Centre.
Timms, K. P., and Wood, L. J. (2020). Sublethal glyphosate disrupts photosynthetic efficiency and leaf morphology in fruit-producing plants, red raspberry (Rubus idaeus) and highbush cranberry (Viburnum edule). Global Ecol. Conserv. 24:e01319. doi: 10.1016/j.gecco.2020.e01319
Tsui, M. T. K., Wang, W.-X., and Chu, L. M. (2005). Influence of glyphosate and its formulation (roundup®) on the toxicity and bioavailability of metals to Ceriodaphnia dubia. Environ. Pollut. 138, 59–68. doi: 10.1016/j.envpol.2005.02.018
USEPA (1994). Method 200.2, revision 2.8: sample preparation procedure for Spectrochemical determination of Total recoverable elements. Available at: https://www.epa.gov/sites/default/files/2015-08/documents/method_200-2_rev_2-8_1994.pdf (Accessed October 10, 2019).
Wang, Y.-J., Zhou, D.-M., Sun, R.-J., Cang, L., and Hao, X.-Z. (2006). Cosorption of zinc and glyphosate on two soils with different characteristics. J. Hazard. Mater. 137, 76–82. doi: 10.1016/j.jhazmat.2006.02.032
Werner, J. R., Wood, L. J., Botten, N., and Fredeen, A. (2022). Potential lasting impacts of industrial herbicides on ungulate nutrition. Can. J. For. Res. 52, 1098–1109. doi: 10.1139/cjfr-2021-0283
Wood, L. J. (2019). The presence of glyphosate in forest plants with different life strategies one year after application. Can. J. For. Res. 49, 586–594. doi: 10.1139/cjfr-2018-0331
Zach, R., Crichton, V. F. J., Stewart, J. M., and Mayoh, K. R. (2011). Early winter food habits of Manitoba moose as determined by three rumen analysis methods. Can. J. Zool. 60, 1300–1304. doi: 10.1139/z82-175
Zaller, J. G., Cantelmo, C., Dos Santos, G., Muther, S., Gruber, E., Pallua, P., et al. (2018). Herbicides in vineyards reduce grapevine root mycorrhization and alter soil microorganisms and the nutrient composition in grapevine roots, leaves, xylem sap and grape juice. Environ. Sci. Pollut. Res. 25, 23215–23226. doi: 10.1007/S11356-018-2422-3
Keywords: glyphosate, glyphosate persistence, plant nutrients, sublethal herbicide, forest management
Citation: Wood LJ, Botten N, Fredeen AL and Werner JR (2024) Glyphosate-based herbicide contributes to nutrient variability in forest plants. Front. For. Glob. Change. 7:1463454. doi: 10.3389/ffgc.2024.1463454
Edited by:
Manoj Kumar Jhariya, Sant Gahira Guru Vishwavidyalaya, IndiaReviewed by:
Abhishek Raj, Rajendra Agricultural University, IndiaArnab Banerjee, Sarguja University, India
Copyright © 2024 Wood, Botten, Fredeen and Werner. This is an open-access article distributed under the terms of the Creative Commons Attribution License (CC BY). The use, distribution or reproduction in other forums is permitted, provided the original author(s) and the copyright owner(s) are credited and that the original publication in this journal is cited, in accordance with accepted academic practice. No use, distribution or reproduction is permitted which does not comply with these terms.
*Correspondence: Lisa J. Wood, bGlzYS53b29kQHVuYmMuY2E=