- 1College of Geographical Sciences, Harbin Normal University, Harbin, China
- 2Heilongjiang Wuyiling Wetland Ecosystem National Observation and Research Station, Yichun, China
- 3Wuyiling Wetland Ecosystem Observation and Research Station of Heilongjiang Province, Yichun, China
Introduction: To investigate the differences in the response of radial growth of larch (Larix gmelinii) at varying altitude gradients to climatic factors, this study established tree-ring width chronologies of Larix gmelinii at altitudes of 900 m, 1,120 m and 1,300 m based dendroclimatology.
Methods: We also employed R packages including “dplR,” “static” and “moving” to determine the correlation between larch at different altitudes and climatic factors and to explore the sensitivity and stability between radial growth and the climate of larch at different altitudes in the northern part of the Greater Khingan Mountains Range in Northeast China.
Results: The results revealed the radial growth of larch in high altitude areas to exhibit a downward trend due to climate warming, while the growth of trees in intermediate and low altitude areas showed an upward trend. The growth–climate relationship exhibited a significant negative correlation between radial growth in low altitude larch (900 m) and temperatures in the current winter (February and March) and growing season (July, October of the current year, and October of the previous year) (p < 0.05). Moreover, high altitude larch (1,300 m) showed a significant negative correlation with temperature in January, March, and October of the current year (p < 0.05). However, in intermediate altitude areas (1,120 m), the radial growth of trees was significantly positively correlated with the temperature of the growing season (May, June and August). Precipitation in April was observed to promote the radial growth of low-altitude larch. Moving correlation analysis revealed that the inhibitory effect of low temperatures in winter and high temperatures in the growing season on the radial growth of larch at three altitudes gradually strengthened, transitioning from significant negative/positive correlations to significant positive/negative correlations along the altitudinal gradient.
Discussion: Larch trees in high and low altitudes exhibited an increased sensitivity to winter and spring precipitation, while in the intermediate region, the inhibitory effect of growing season precipitation on larch radial growth continued to intensify. The results of the study have an important reference value for the in-depth understanding of the growth dynamics of Larix gmelinii natural forests in the northern part of the Greater Khingan Mountains under climate change.
1 Introduction
Climate warming is a prominent global environmental issue that poses a significant threat to people’s lives and the quality of the ecological environment, and has led to substantial changes in global terrestrial ecosystems (Tollefson, 2018). The Intergovernmental Panel on Climate Change (IPCC) Sixth Assessment Report has emphasized that high-latitude regions are particularly sensitive to this climate change (IPCC, 2021). Forest ecosystems, being a vital component of terrestrial ecosystems, provide energy and ecological services that are also severely disrupted by climate change. To adapt to global climate warming, the growth distribution of numerous plants is currently undergoing alterations in terms of altitude or latitude (Kueppers et al., 2017), and the suitability for the long-established survival of numerous species is continuously changing. Climate warming has not only resulted in an increased occurrence of extreme weather events worldwide, such as severe droughts, heatwaves, heavy snowfalls, and frost damage (Kitudom et al., 2022), but it has also prompted trees to migrate to higher altitudes and latitudes (Morgan et al., 2014), thereby greatly impacting tree growth in high-latitude forest areas. Previous research has indicated that rises in temperatures have induced changes in species distribution and land cover types in high-latitude forest regions (Ustin and Xiao, 2001; Tchebakova et al., 2010). More specifically, the increase in temperature has led to a northward or uphill shift of forest lines and a reduction in tree species seed production, ultimately affecting forest composition and structure (Soja et al., 2007; Kharuk et al., 2009). In fact, numerous studies, both at the regional and global scale, have demonstrated the influence of global warming on woody plants (Park Williams et al., 2013). Tree rings can be employed as a precise tool to accurately record the annual impact of climatic factors on tree growth, revealing the impact of past and recent climate change on trees, as well as the response trends of forests to future climate change (Tessier et al., 1997). Therefore, tree rings can be used as a historical tool to reveal long-term change trends in forest ecosystems, further assess the impact of climate warming on tree growth and its dynamic changes, and predict potential alterations in forests through feedback mechanisms with climate elements under future global changes (Fritts, 2012).
The study of tree rings at different altitudes is of great significance for understanding the impact of climate change, ecosystem evolution and the resource management of tree radial growth (Chhin et al., 2008). The relationship between radial growth and climate in the Douglas fir (Pseudotsuga menziesii) mountain areas (Zhang and Hebda, 2004), revealing that precipitation during the growing season influenced growth in both high and low-altitude regions, while temperature played varying roles at different altitudes. The study pointed out that the role of tree-ring studies in revealing a distinct “threshold” in the temperature response in alpine regions (Wilmking et al., 2004). In particular, the tree-ring width of white spruce along the Alaska Mountain alpine forest line has been reported to exhibit a positive correlation with temperature within a certain temperature range, however, beyond a specific “threshold,” this correlation becomes negative. Studies based in the Alpine Alps also reveal an increased sensitivity of Norway spruce (Picea abies) to precipitation during the 20th century, while its sensitivity to temperature is observed to decrease. Taking Mont Saint-M, France, as the study area, Vila et al. (2008) showed that different tree species exhibited contrasting responses to temperature increases. More specifically, Aleppo pine (Pinus halepensis) and Scots pine (Pinus sylvestris) displayed divergent reactions to elevated temperatures, while higher temperatures promoted radial growth in Aleppo pine across various elevations. In addition, Scots pine in higher altitudes showed a decelerated trend in radial growth in response to warming temperatures. For each specific tree species, the distribution of limiting factors along the altitudinal gradient has undergone changes, shifting from moisture stress in lower altitudes to cold limitations in higher altitudes (Salzer et al., 2009), particularly in temperate and northern forest regions.
The Greater Khingan Mountains in China is one of the most intact subarctic forests globally, as well as the largest larch nature reserve and the most extensive continuous habitat in China. As a consequence, current research regarding the relationship between Dahurian larch growth and climate predominantly focuses on this region and high-altitude areas (Serre, 1978). The Dahurian larch stands as a pivotal species within the forest ecosystems of Northeast Asia, with its southernmost distribution extending to the Greater Khingan Mountains region in northern China. The presence of larch in China consistently hugs the southeastern fringes of the Eurasian continent. As temperature decreases gradually with ascending altitude, the Dahurian larch has expanded toward the high-altitude regions in Northeastern China (Leng et al., 2008). However, in the context of global warming, the ecological system of the Dahurian larch is threatened by permafrost degradation, leading to a northward shift of its southern boundary (Wei et al., 2011). Zhang et al. (2016) utilized a network of larch tree-ring width chronologies from various regions within the northeastern permafrost area to investigate the impact of rapid warming on tree growth, and discovered that increasing the depth of permafrost thaw benefits the growth of trees in high-latitude permafrost regions. Li et al. (2021) conducted a study on the spatial heterogeneity of tree growth across the Daxing’anling region of Inner Mongolia in response to climate warming. The authors found a significant positive correlation between Dahurian larch and spring temperatures, while a significant negative correlation was observed with summer temperatures, and the larch showed strong resilience to drought. Chen et al. (2023) investigated the response of Dahurian larch trees at different altitudes and under varying competitive intensities in the Greater Kingan Mountains to climate. The results revealed key climatic and environmental variables influencing Dahurian larch growth in this region, and the models predicted a gradual reduction in larch growth with future increases in temperature. The aforementioned studies provide valuable insights into the response patterns of northern forest ecosystems, highlighting the intricate roles of temperature and precipitation in shaping these ecosystems. Thus, it is crucial to understand the role of the altitudinal effect as a key geographical feature in growth–climate response patterns and future species distributions. Based on this, we can utilize variations along altitudinal gradients to indicate the evolution of climate change over specific time periods (Change, 2013).
In this study, we set up three tree-ring width chronologies at altitudes of 900, 1,120 and 1,300 m, and conducted dendroclimatic analysis on Larix gmelinii in the northern region of the Greater Khingan Mountains in China. Based on the research question “divergent climate responses in radial growth of Larix gmelinii along different altitudinal gradients in Northeastern China,” we aim to: (1) investigate the growth trends of L. gmelinii on the northwestern slope of the Greater Khingan Mountains under climate warming; (2) explore the distinct growth patterns of L. gmelinii at different altitudes under the influence of climate gradients, and identify the primary climatic constraints affecting growth at various elevations; and (3) analyze the dynamic variations in the radial growth of L. gmelinii at different elevations. The findings of this study provide valuable references into the future dynamics and development trends of forest growth, as well as the distribution ranges of forest tree species in the context of global warming.
2 Materials and methods
2.1 Study area
The study area is located on the northwestern slope of the Greater Khingan Mountains in northeast China (Figure 1) (51° 51′ 02″-52° 30′ 52” N, 121° 05′ 44″-122° 47′ 05″ E). The sampling sites are situated within the Beian Forest Farm on the northern bank of Mangui Town, Hulunbuir City, Inner Mongolia Autonomous Region. The area is characterized by a middle-low mountains terrain, with altitudes ranging from 509 to 1,409 m above sea level, a high latitude, and permafrost that gradually transitions from the discontinuous permafrost zone in the northwest of the Greater Khingan Mountains to the continuous permafrost zone in the southeast (Guo et al., 2015). The study area is mainly influenced by the cold temperate continental monsoon climate, with simultaneous rainy and warm periods, and a generally low temperature and humid climate. From 1960 to 2020, the mean annual temperature was −4.7°C, with the highest temperature occurring in July (24.0°C) and the lowest in January (−36.9°C). The mean annual temperature in this area has exhibited a constantly increasing trend (Figure 2). The mean annual precipitation within the study area is 438 mm, with the concentration primarily in June, July, and August, totaling 289 mm, and the maximum mean precipitation in July, accounting for 26.5% of the total annual precipitation (Figure 2). The forest coverage in this area stands at an impressive 95.84%, with natural forests constituting over 95%. The predominant soil type is brown coniferous forest soil, and the existing tree species are mainly Larix gmelinii, Pinus sylvestris var. mogolica, Betula platyphylla and Picea koraiensis.
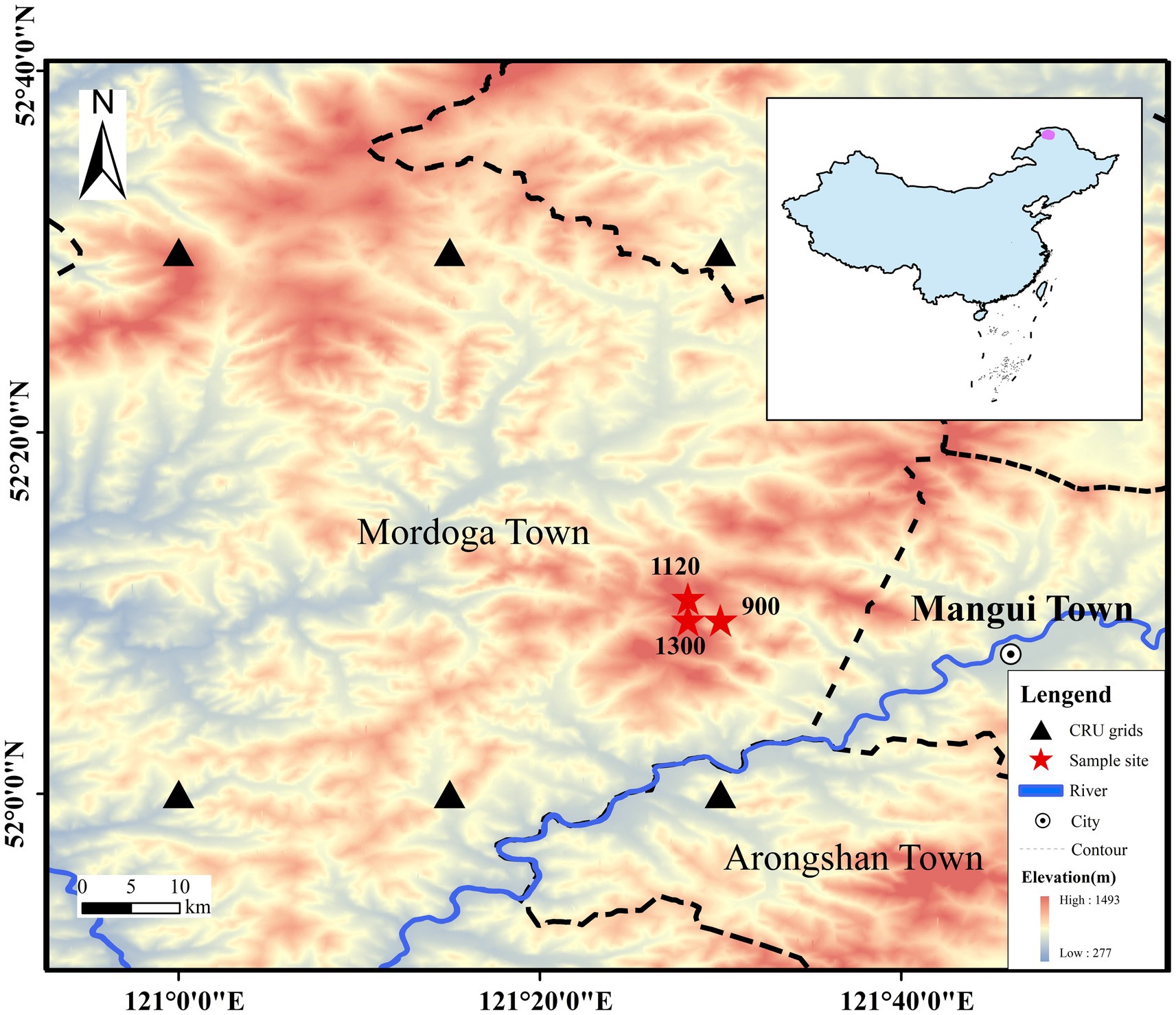
Figure 1. Distribution of sample sites. Inset map shows the location of the study area (shaded in purple) within China.
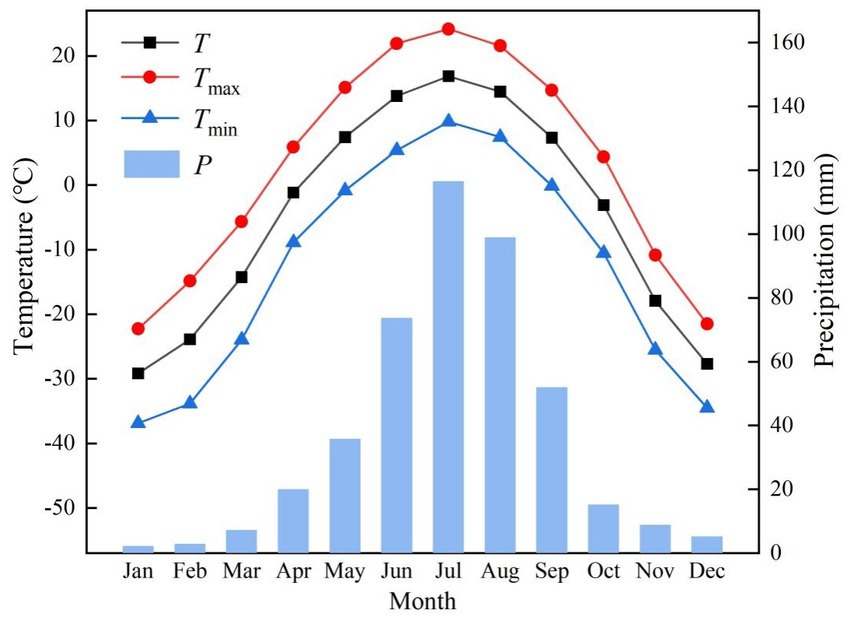
Figure 2. Mean values in monthly and meteorological factors from 1960 to 2020 in the study area. T, mean temperature; Tmax, mean maximum temperature; Tmin, mean minimum temperature; P, precipitation.
2.2 Tree-ring sample collection and chronology establishment
Based on the principles of dendrochronology (Stokes and Smiley, 1968), and considering the elevation, topography, and distribution characteristics of L. gmelinii in the study area, sampling was conducted in July 2022. Three sampling sites were established at elevations of 1,300, 1,120 and 900 m. Due to the relatively small response of dominant trees to non-climatic factors and their better signal-to-noise ratio, we selected healthy dominant trees with larger breast diameters in undisturbed areas within each sampling site. Using an increment borer, we extracted two coarse cores (with an inner diameter of 10.00 mm) from each selected tree at breast height (1.3 m above the ground) along two different directions (perpendicular and parallel to the slope). One fine core (with an inner diameter of 5.00 mm) was then collected in at a random direction and numbered. A minimum of 20 larch trees were collected from each sampling site. Table 1 presents details of the site information.

Table 1. Information for the sampling sites of Larix gmelinii at tree elevations in the northern Greater Khingan Mountains.
The prepared, dried, fixed, sanded, and polished tree core samples were cross-dated under a microscope and measured using a LINTAB6 tree-ring width measuring system (Rinntech, Heidelberg, Germany) with a 0.01 mm accuracy. The cross-dating of the measured tree-ring width data was conducted using PAST5. This was followed by a quality check of the measurement sequences and dating results using COFECHA (Holmes, 1983). Samples with low correlation coefficients were re-examined, and poor-quality sequences were removed to ensure the reliability of the dating results. Finally, the negative exponential function in the ARSTAN chronology development program (Cook, 1985) was employed to simulate growth trends, and the standard chronology (STD), residual chronology (RES) and autoregressive chronology (ARS) of three larch tree-ring widths at different altitudes were established. We calculated the average sensitivity (MS), signal-to-noise ratio (SNR), sample population explanatory amount (EPS) and first-order autocorrelation coefficient, among other parameters, revealing the STD to contain high-quality high-frequency information. Therefore, it was adopted from the subsequent analysis.
2.3 Meteorological data
The growth season for L. gmelinii typically lasts around 165 days (Hoff et al., 2015), from early May to mid-to-late October. The growth of trees is not only influenced by the climate of the current year but also by that of the previous year, that is, climate has a “lag effect” on tree growth (Cabral-Alemán et al., 2022). Therefore, climate factors from September of the previous year to October of the current year for the period ranging from 1960 to 2020 were selected for this study. Meteorological data were obtained from the Royal Netherlands Meteorological Institute (http://climexp.knmi.nl). Climate grid data of 0.5° × 0.5° CRU (4.05) within the ranges of 52.00°-52.50° N and 121.00°-121.50° E were employed as the background of climate change in this study area. The CRU database consists of the difference of regional weather stations. In the areas with a small number of weather stations, the CRU data are non-uniform. Therefore, the accuracy of CRU climate data is verified by using the observation data of Genhe (50.47° N, 121.31° E), Mohe (52.58° N, 122.31° E) and Huzhong (52.02° N, 123.34° E) weather stations and the correlation between the average observation data of the three stations and CRU climate data. The results showed that there was a significant correlation between CRU grid data and climate station data (p < 0.01). The width of tree rings formed during the growth process is influenced by a combination of climate factors from both the current year and the pre-growing season, particularly temperature and precipitation, and consequently play a crucial role in shaping tree growth and ring structure (He et al., 2017). Therefore, climate factors from 1960 to 2020, including monthly mean temperature (T), monthly mean maximum temperature (Tmax), monthly mean minimum temperature (Tmin), and precipitation (P), were selected for the subsequent analysis.
2.4 Data analysis
The R (R Core Team) packages “dplR” and “treeclim,” and the functions “TRADER,” “dcc,” “static” and “moving” were employed to compute the correlations and moving correlation coefficients between the standardized tree ring width chronologies and climate factors for September of the previous year to October of the current year from 1960 to 2020. A sliding window of 31 years was determined using Dendroclim 2002, and graphical representations were generated using the “graphics” and “ggplot2” R packages. We also used the univariate linear regression function in the software SPSS25 to calculate the growth trend of larch at different altitudes over the past 30 years. Mapping was performed in Arcmap 10.8 (Esri).
3 Results
3.1 Chronology characteristics
For the standard chronology of larch at the three different elevations, the time span ranged from 121 to 277 years (Table 2; Figure 3), the common interval was 1905–2022, and the intermediate altitude sample plot had the longest chronology time span of 277 years (1745–2022). As the main objective of this study is to investigate the impact of altitude gradients on radial growth and the response of radial growth to climate, we used an approximate 60-year chronology, spanning from 1960 to 2020, matching the length of climate records (Figure 4). The minimum replication threshold of the chronology was determined using sub-sample signal strength (SSS). In this study, tree-ring width chronologies with SSS > 0.85 were selected as reliable intervals to ensure both sequence reliability and maximum length. The standard deviation (SD) indicates the magnitude of climate signals contained in the tree-ring width chronologies. Compared to the middle and lower altitude regions, higher altitude areas exhibit a reduced amount of climate information, yet tree growth demonstrates a higher average sensitivity (MS) and preserves a higher level of climate information (SNR). The first-order autocorrelation coefficient (1st AC) reveals that the growth of L. gmelinii at all three altitudes is greatly influenced by the previous year’s climate. The mean within-tree correlation coefficient indicates a high degree of consistency in inter-annual growth variations among different trees, with all values of EPS exceeding 0.85 (Table 2). This indicates high-quality chronologies that effectively represent the overall characteristics of L. gmelinii growth at various altitudes within the study area. In the three distinct altitude-based L. gmelinii tree-ring chronologies, high-altitude growth has exhibited a declining trend with a negative growth slope over the past three decades, while intermediate and low-altitude growth has shown an upward trend. Note that these growth trends are not statistically significant (Figure 4).
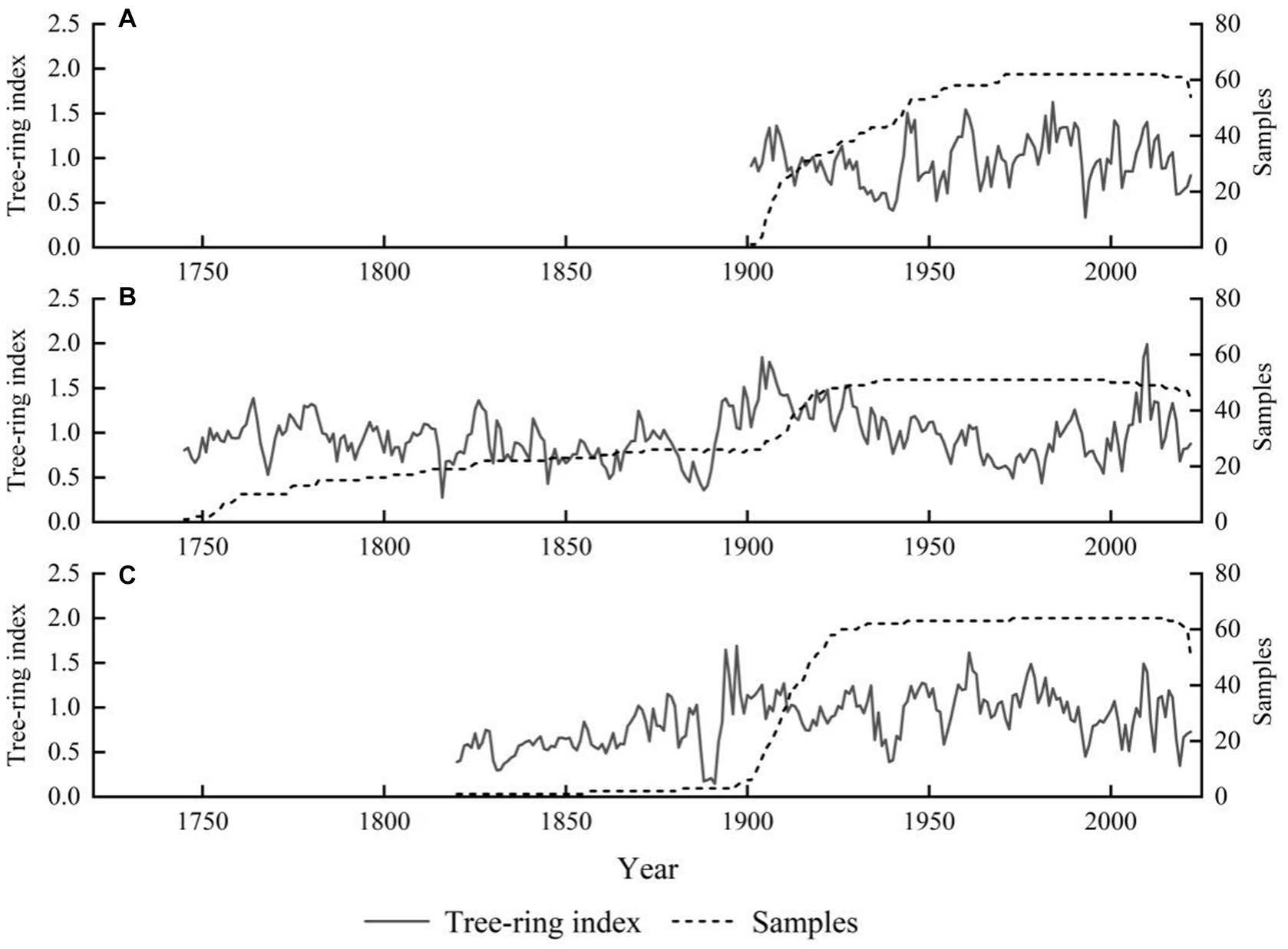
Figure 3. Standardized chronology of the tree-ring width of Larix gmelinii at three altitudes in the northern Greater Khingan Mountains. (A), High altitude: 1300 m; (B), Intermediate altitude: 1120 m; (C), Low altitude: 900 m.
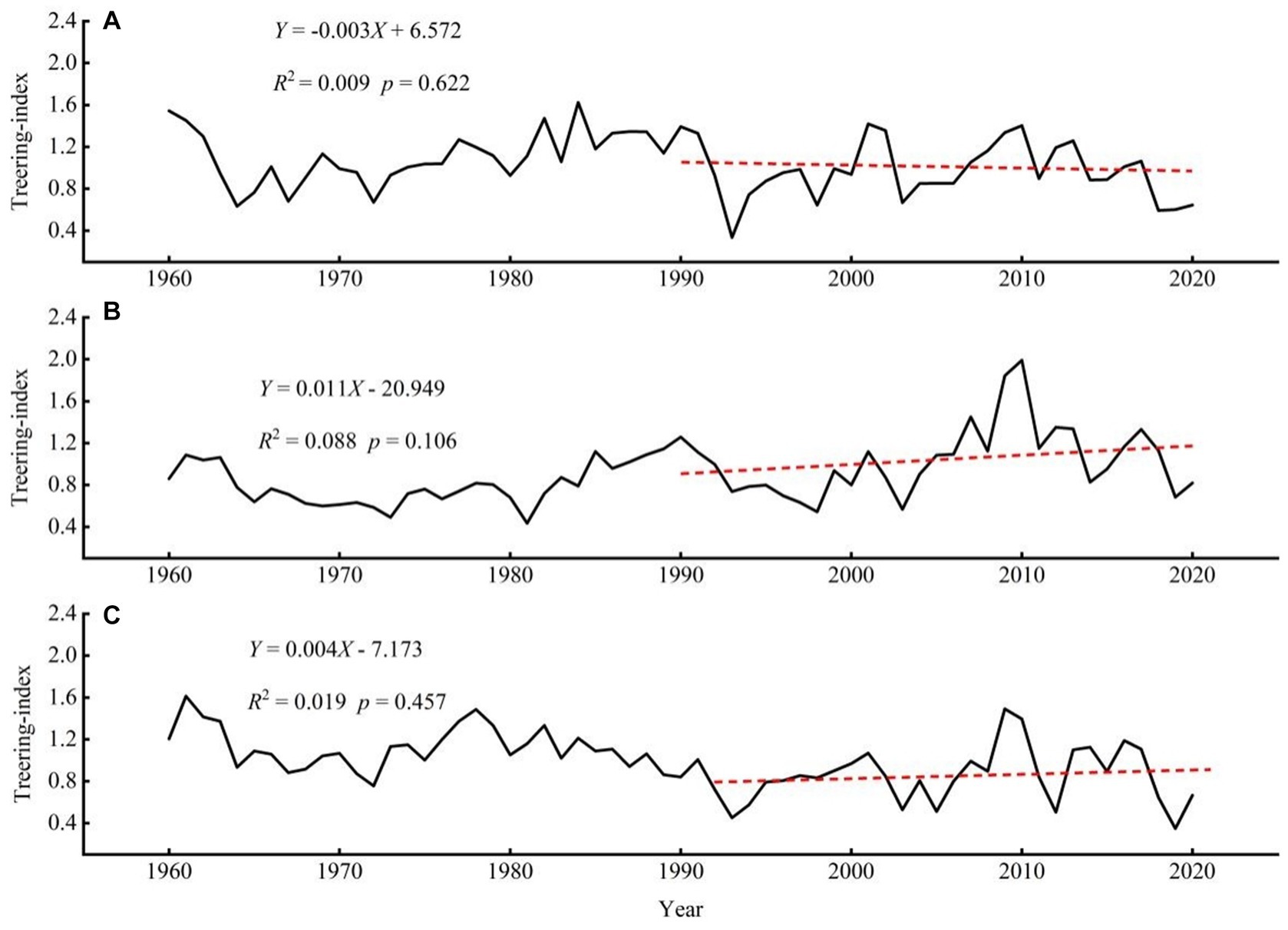
Figure 4. Chronologies of Larix gmelinii at three altitudes from 1960 to 2020. Red line denotes the growth trend during the latest 30 years. (A), High altitude: 1300 m; (B), Intermediate altitude: 1120 m; (C), Low altitude: 900 m.
3.2 Correlation between radial growth and climate factors at different altitudes
During 1960–2020, the response of Larix gmelinii tree-ring width variations to climate factors exhibited both similarities and differences across the three distinct altitudes. Comparative analysis revealed temperature to be the primary climatic factor influencing radial growth in the region. Moreover, all three altitudes of Larix gmelinii were positively correlated with precipitation (Figure 5). Notably, only low-altitude Larix gmelinii had a significant positive correlation with precipitation in April of the current year (r = 0.252, p < 0.05). The correlation analysis with temperature factors (mean, mean maximum and mean minimum temperature) (Figure 5) indicated the radial growth of Larix gmelinii at different altitudes to exhibit varying responses to temperature. Among them, the response of low-altitude Larix gmelinii to temperature showed an overall negative correlation, with significant negative correlations (p < 0.05) with the monthly mean temperatures of February, March, July, and October of the current year (r = −0.291, r = −0.335, r = −0.293 and r = −0.467, respectively), as well as with October (r = −0.397) of the previous year. Furthermore, significant negative correlations and above (p < 0.05 for February, March, and October; p < 0.01 for July) were observed with the mean maximum temperatures in February, March, and October of the current year, as well as with that of October of the previous year (r = −0.276, r = −0.289, r = −0.393 and r = −0.363, respectively). In addition, highly significant negative correlations (p < 0.01) were observed with the mean minimum temperature of October for both the previous and current year (r = −0.482 and r = −0.336, respectively), and significant negative correlations (p < 0.05) with those of February, March, June and July (r = −0.287, r = −0.289, r = −0.263 and r = −0.289, respectively) for the current year. For the intermediate altitude Larix gmelinii, the overall response to temperature was positive. In particular, highly significant positive correlations (p < 0.01) were observed with the mean temperatures of May and August (r = 0.421 and r = 0.335, respectively), the mean maximum temperature in May (r = 0.354), and the mean minimum temperature in June (r = 0.406 and r = 0.464, respectively), all of which in the current year. Moreover, the Larix gmelinii at this altitude exhibited significant positive correlations (p < 0.05) with the mean temperature in June (r = 0.288) and mean minimum temperature in August (r = 0.320) of the current year. In contrast, high-altitude Larix gmelinii demonstrated an overall negative response to temperature. More specifically, significant negative correlations (p < 0.05) were observed with the mean temperatures (r = −0.251, r = −0.270 and r = −0.283, respectively), mean maximum temperatures (r = −0.260, r = −0.262 and r = −0.266, respectively), and mean minimum temperatures (r = −0.235, r = −0.275 and r = −0.259, respectively) of January, March, and October in the current year.
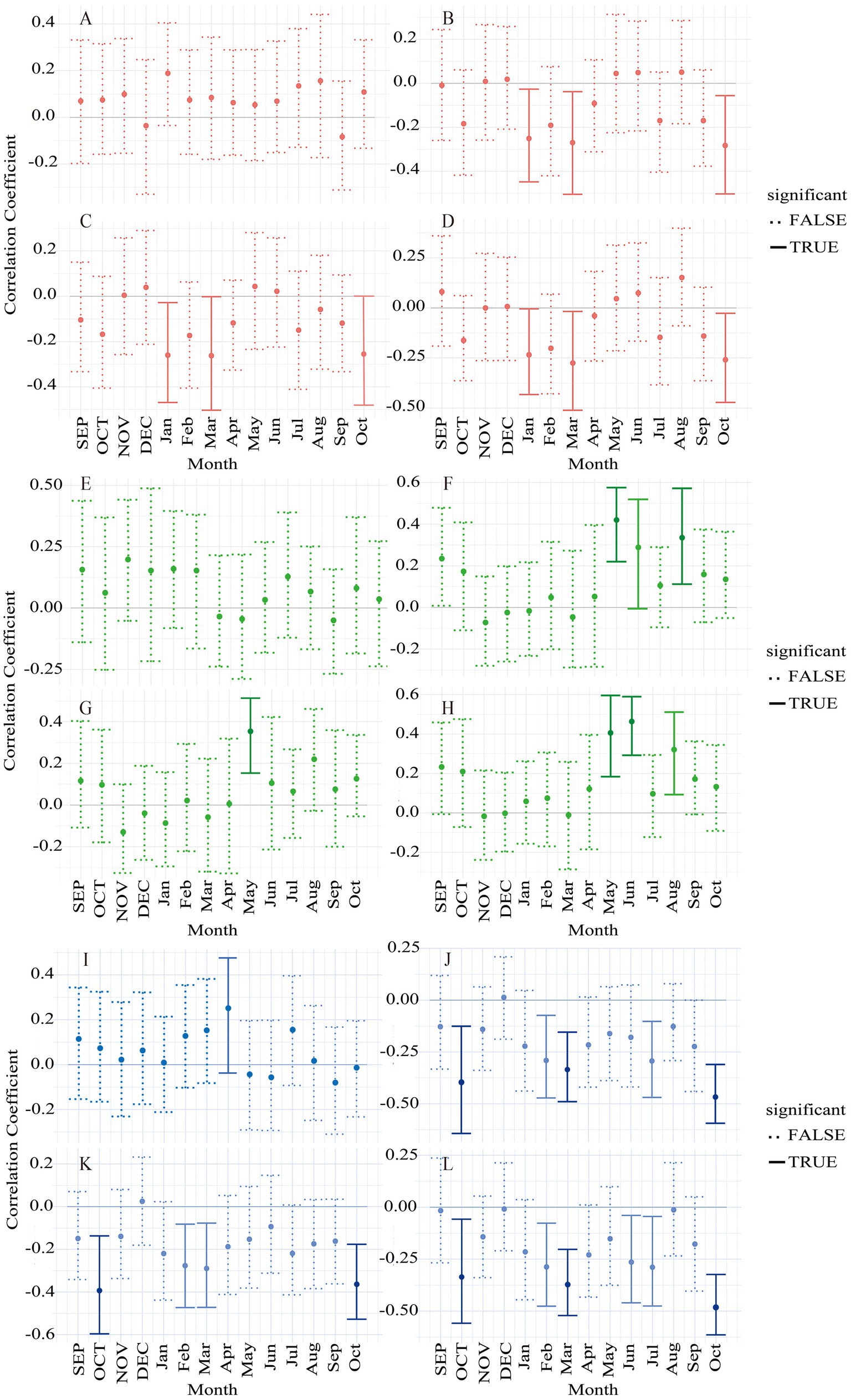
Figure 5. Correlation between the standardized chronology of tree-ring width and the monthly climate factors of Larix gmelinii at three elevations in the northern part of the Great Khingan Mountains. (A–D) represent precipitation, mean temperature, mean maximum temperature and mean minimum temperature, respectively, as do (E–L). Red, green and blue charts denote high-, intermediate and low altitude Larix gmelinii, respectively. Light tones for p < 0.05 and dark tones for p < 0.01. Capital letters indicate previous year months; lowercase: current year months. The analysis was performed with a sample size of n = 61.
3.3 Dynamics of radial growth and climate factors at different altitudes
The moving correlation analysis reveals variations in the responses of Larix gmelinii at different altitudes to radial growth and climatic factors (Figure 6). As the climate warms, there is a general upward trend in the sensitivity of tree-ring width to hydrothermal factors, resulting in periods where correlation coefficients convert between positive and negative values. In terms of precipitation, distinct variations in response to precipitation factors are observed among Larix gmelinii at different altitudes. For low-altitude Larix gmelinii, correlations with precipitation were significantly negative (p < 0.05) only during July in the years from 1961 to 1992, gradually transitioning from a negative to positive response. Intermediate altitude Larix gmelinii exhibited a significant positive correlation with precipitation in June of the current year, which diminished over time, eventually turning into a negative response. High-altitude Larix gmelinii displayed a significant negative correlation with December precipitation of the previous year that gradually weakened with time, while unstable correlations were observed with June and August of the current year. These findings illustrate the varying and complex responses of Larix gmelinii at different altitudes to precipitation, with numerous shifts from negative to positive correlations as well as weakening responses over time.
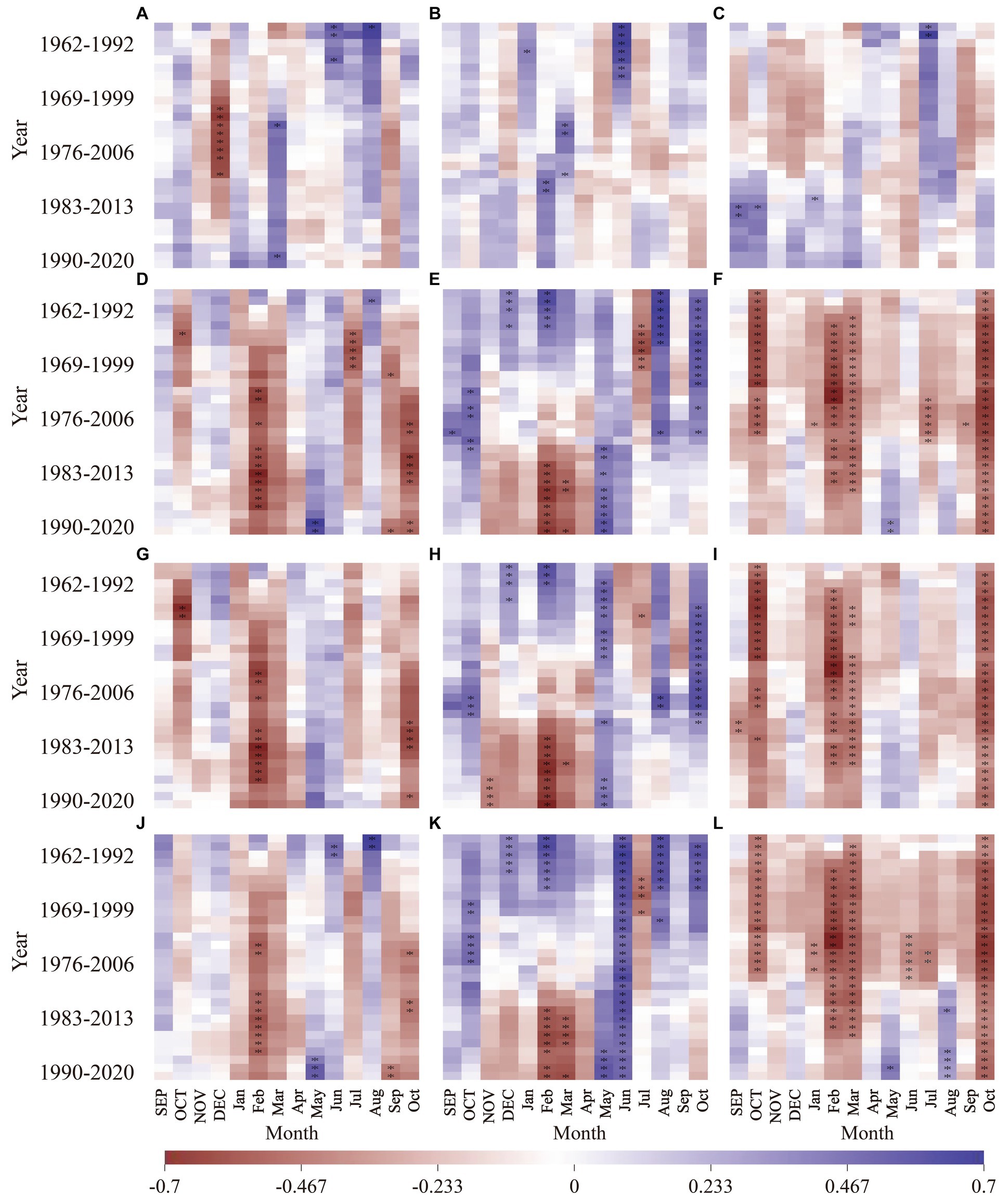
Figure 6. Moving correlation analysis between the standardized chronology of tree-ring width and monthly climate factors of Larix gmelinii at three elevations in the northern part of Greater Khingan Mountains. (A–C), Precipitation; (D–F), Mean temperature; (G–I), Mean maximum temperature; (J–L), Mean minimum temperature. The first, second and third columns indicate high, intermediate and low altitude, respectively. p < 0.05. Capital letters: previous year months; lowercase: current year months.
Figure 6 reveals both similarities and differences in the response of the three Larix gmelinii chronologies at different altitudes to temperature variations during the period from 1960 to 2020. At low altitude, the sensitivity to temperature, including the previous year’s October, the current year’s February, March, and October mean temperatures, as well as mean maximum and minimum temperatures, progressively increased to a significant level. In addition, a significant negative correlation with the average temperature in July and the average minimum temperatures in June and July of the current year emerged in 1974, and subsequently gradually diminished. The intermediate altitude Larix gmelinii exhibit a significantly weakened positive correlation with the average temperature, mean maximum temperature, and mean minimum temperature for the months of December of the previous year and February, August, and October of the current year. Notably, a significant negative response emerged gradually with temperature of February in the current year, while a significant positive response was observed gradually with the mean and mean maximum temperatures of May in the current year. Furthermore, there was a consistently significant positive relationship with the average minimum temperature in June that remained stable throughout the study period. In contrast, high-altitude Larix gmelinii radial growth exhibited a gradual transition from a non-significant negative correlation to a significant negative correlation (p < 0.05) with average temperatures in the current year for February, July, and October. Similarly, the mean maximum and minimum temperatures presented significant negative correlations in February and October of the current year. However, a gradually shift to a significant positive correlation (p < 0.01) was observed for the mean minimum temperature in May.
4 Discussion
4.1 Radial growth characteristics of larch at different altitudes
Extensive surveys and data analysis reveal that tree-ring width in high-altitude forests is often limited by temperature during the cold season, while growth during the warm season faces fewer restrictions. Simultaneously, the growth of low-altitude trees depends on the facilitation of water availability, resulting in a gradually increasing connection between tree-ring width and precipitation (Harsch et al., 2009). However, due to the complex and variable climate patterns in the study area, as well as local environmental disturbances, differences in tree growth among different altitudes are common (Sidor et al., 2015; Matías et al., 2017). In extremely cold regions, temperature is considered the primary factor limiting tree growth, and temperature fluctuations are directly reflected in variations in annual ring width (Hartl-Meier et al., 2014). The geographical distribution of forests is shifting toward higher altitudes as the climate warms, particularly for trees at the southern boundaries of boreal forests, making them more susceptible to drought stress induced by climate warming. As altitude increases, changes in temperature and adjustments in precipitation patterns will impact the growth rate and tree-ring width of these trees (Moritz and Agudo, 2013).
This study reveals significant differences in the radial growth trends of Larix distributed at various altitudes within the study areas over the past 30 years: larch trees at high altitudes exhibit a negative growth trend, while that of trees at middle and low altitudes is positive (Figure 3). Terrestrial vegetation responds to changes in weather and climate, and such responses are influenced by physiological, population, and ecosystem functions. The distribution of plant species, growth status, and ecological community evolution are constrained by these functions, and this relationship changes over time (Bonan, 2015). Therefore, changes in climate and weather can directly impact plant phenology (e.g., growth rates, bud development, and flowering) and can also have indirect effects by influencing forest structure and dynamics (Loehle and LeBlanc, 1996). Such growth trends may gradually form the alpine tree line within the study area when temperatures exceed a certain threshold. This phenomenon considered to be primarily influenced by temperature, as temperatures decrease with increasing altitude. When temperatures fall below a certain value, trees cease to grow, forming an alpine forest line (Oberhuber, 2004). However, studies reveals that tree growth in the Daxing’anling region is also influenced by moisture availability, with water scarcity around the tree line affecting tree growth. Even in regions where temperatures and moisture are suitable for tree growth, negative growth trends are observed in trees. This is attributed to the strong competitiveness of other species in the forest line shrub, which occupy the majority of the soil and water resources, resulting in the seed germination of forest tree species (Tranquillini, 2012). It is worth noting that the annual mean temperature varies by approximately 3°C between high and low altitudes. Therefore, this altitude-related temperature difference masks the effects related to latitude in Larix growth (Kosaka and Xie, 2013). Furthermore, the tree line is highly sensitive to environmental changes at forest boundaries, particularly in the study region, where even small fluctuations in temperature and moisture evaporation can affect the growth of trees at the tree line (Billings, 1969). This suggests that temperature constraints dominate over other environmental factors (e.g., topography and biological communities) in the radial growth of Larix, as reflected in the analysis results of Larix’s response to climate. Based on the response of high-altitude tree line trees to climate, combined with climate change and ecological disturbance factors, we can predict the growth trends of forests under temperature changes. The differential response of Larix radial growth to climate reveals distinct growth patterns of trees at different altitudes in the study area. More specifically, high altitudes are subject to low-temperature constraints, which consequently slows down tree growth under cold conditions. Conversely, as altitude decreases, the degree of tree growth response to precipitation changes becomes more pronounced.
4.2 Differences in the climate response of radial growth of larch at different altitudes
This study reveals a positive correlation between radial growth and precipitation in three different altitude Larix species within the study area. This relationship may be attributed to Larix’s preference for light and relative drought tolerance, primarily observed in well-drained and moist but not excessively wet soil environments (Rehfeldt and Jaquish, 2010). With rising temperatures, increased solar radiation and surface evaporation create drier habitats with shallow and nutrient-poor soil conditions, which increases demand for moisture in tree growth (Hirano et al., 2017). Previous research has shown that the growth initiation time and maximum growth rate duration of Pinus densata, a high-altitude pine species in the Baishuijiang Mountains, are also regulated by soil moisture availability (Sun et al., 2021). Our results reveal low-altitude Larix to exhibit a significant positive correlation with precipitation in April of the current year, while no significant relationship is observed between intermediate and high altitude Larix and precipitation. This altitude-based difference in the relationship between Larix radial growth and precipitation may reflect variations in water utilization and tree environmental adaptability in different altitude regions.
For low-altitude Larix in the northern part of the Greater Khingan Mountains, if there is no water stress during the previous year’s growing season, the water reserve from the previous year can support early-season radial growth in the current year, with winter snow providing moisture for the next growing season (Hessburg et al., 2019). April marks the beginning of spring and is typically accompanied by increased precipitation, while rising temperatures induce a hydrothermal phenomenon (Cai et al., 2020). Ground temperatures gradually recover (monthly averages of −11.3°C, −1.1°C and 7.4°C in March, April and May, respectively) and the thawing of permafrost commences, allowing for sufficient soil moisture to stimulate tree growth. During the early part of the growing season, trees exhibit heightened sensitivity to effective water supply due to enhanced physiological activity (Vaganov et al., 1999), and as temperatures rise and daylight hours increase during this period, increased precipitation can provide the necessary moisture for trees, promoting their physiological activity and metabolism leading to a positive growth response. Moreover, in low-altitude areas, permafrost degradation leads to decreased soil moisture and pre-rainy season water shortages. Therefore, radial growth in low-altitude Larix relies more on April precipitation than other months (Kolář et al., 2017).
For intermediate and high altitude Larix, the environmental water supply and water utilization differ from those in low-altitude areas, and the growth conditions are more severe, for example, low temperature, higher evaporation rates, and soil moisture limitations. In arid conditions under climate warming, enduring a long dry season combined with insufficient spring moisture and reduced humidity can result in a water deficit for trees, limiting their springtime growth (Ren et al., 2018). Under such scenarios, trees rely more on factors such as temperature and soil nutrients to regulate their growth activities, and changes in precipitation may be masked by other factors. For example, the melting of spring snow and permafrost great contributes to the soil moisture content in the upper layers (Zhang et al., 2018), providing additional moisture to the trees. In addition, summer precipitation can lead to soil oversaturation, affecting tree root respiration, and further reducing the correlation between radial growth and precipitation (Yang et al., 2018). Therefore, although increased spring precipitation can provide the necessary moisture for tree growth, it is not the primary influencing factor, and thus the response of intermediate and high altitude Larix to precipitation variations is not as pronounced as in low-altitude areas. In summary, the significant positive correlation between low-altitude Larix and April precipitation in the Greater Khingan Mountains is attributed to the provision of adequate moisture by spring precipitation for tree growth, which consequently promotes growth activities and metabolism, leading to a positive response. While, the absence of a significant relationship between middle and high-altitude Larix and precipitation is a result of interactions with other environmental factors, such as permafrost degradation, distribution patterns, soil moisture, and solar radiation.
The radial growth of low-altitude Larix is negatively correlated with monthly mean temperatures (in February, March, July, and October of the current year and October of the previous year), monthly mean maximum temperatures (in October of the previous and current years), and monthly mean minimum temperatures (in October of the previous year and in February, March, June, July, and October of the current year) (Figure 5). These significant negative correlations principally occur during the winter and growing seasons, with highly significant negative correlations largely observed during the winter months. This indicates that low temperatures during the winter and high temperatures during the growing season inhibit the growth of low-altitude trees. In the study area located in the Greater Xing’an Mountains, cold winter temperatures may induce cold stress in trees, damaging cell membrane structures and protein activities, and harming the woody part of the trees. Low temperatures also cause the freezing of moisture in the soil, preventing root absorption of water and nutrients. Moreover, excessive snowfall can shorten or delay the growing season (Kirdyanov et al., 2003). In recent decades, climate warming has led to rapid and widespread discontinuous permafrost cracking and degradation in low-altitude stands (Bockheim et al., 2013). Changes in water resources caused by the degradation of permafrost have a significant impact on tree growth and available water supply, subjecting forests to unprecedented drought stress and forcing them to adapt to greater environmental changes (Helbig et al., 2016). Along with excessively high temperatures during the growing season, soil moisture evaporation accelerates, increasing water loss. This subsequently induces a marked rise in the evaporation and vapor pressure deficits. Even the occurrence of abundant precipitation cannot prevent the formation of narrow rings in trees. In addition, inadequate precipitation in degraded permafrost areas leads to net losses in forests and restrictions on tree growth (Linares and Tíscar, 2011). Zhang et al. (2008) demonstrated that the response of tree growth to climate exhibits an altitudinal trend, with the negative impact of temperature increasing with decreasing altitude. This is attributed to the drought stress induced by warming, as well as the corresponding reduced net photosynthesis, increased soil moisture evaporation, and plant transpiration, all of which affect carbohydrate synthesis in trees, resulting in slow growth (Huang et al., 2010). Therefore, excessively high temperatures during the growing season are negatively correlated with the radial growth of low-altitude Larix.
Intermediate altitude Larix generally exhibits a positive correlation with temperature, exhibiting significant positive correlations with mean temperatures, mean maximum temperatures, and mean minimum temperatures during the growing season. Previous studies report the most vigorous tree growth to occur during the current-year growing season, and an increase in temperature at the beginning of the growing season benefits trees by extending the duration of their growth season, while enhanced photosynthesis in plants simultaneously promotes radial growth in trees (Akkemik, 2000; Chen et al., 2017). Intermediate altitude Larix is located in the transition zone between discontinuous and continuous permafrost, and warming provides trees with more heat and favorable conditions for photosynthesis (Goodine et al., 2008). The ground temperature is restored, and the winter snow can retain the water content of the topsoil when spring arrives, effectively achieving relative “cold insulation,” “heat preservation” and “nutrient storage,” which can promote the early-season growth of trees (Kirdyanov et al., 2003). Jyske et al. (2014) found that warm winters stimulate tree growth in northern forests, aligning with the overall findings of the radial growth of middle-altitude Larix in the current study.
On the other hand, high-altitude Larix exhibits a significant negative correlation with the average temperature and average minimum temperatures in January, March, and October, as well as the average maximum temperatures in January and March. This is consistent with the results of Zhao et al. (2019), and suggests that cold winters (freezing or snow cover loss) and high temperature in summer limit the growth of Larix (Case and Peterson, 2007). In high-altitude ecosystems, trees generally have a relatively short growing season and plants growing above the snow cover are exposed to cold winter temperatures, which can be limiting and stressful by low temperatures. Thick snow cover may also damage branches or buds, leading to reduced growth in the following year (Chapin III et al., 2008). The combination of a short and cold growing season is unfavorable for radial growth in trees, resulting in narrower growth rings (Gindl et al., 2000). Furthermore, in the high-altitude regions of the Greater Khingan Mountains, low temperatures during the winter and spring delay the onset of the growing season, putting trees in a dormant state (Lo et al., 2010). During the growing season, high temperatures and drought conditions cause trees to absorb a large amount of nutrients for growth, leading to significant nutrient and organic matter loss during allocation from the crown to the roots. This situation can contribute to the formation of narrow growth rings. In this study, the correlation between radial growth in high-altitude larch and temperature is not highly significant. This is because interannual climate variability and factors at the stand level, such as interspecies competition, stand density, microtopography, soil nutrients, and carbon allocation, collectively influence the radial growth of larch in high-altitude areas. Research has shown that different soil types and nutrient levels can impact a plant’s nutrient uptake and utilization (Havranek and Tranquillini, 1995). In addition, tree species composition and stand density play vital roles in forest growth, determining competitive relationships in various species, photosynthetic efficiency, and the overall structure and function of ecosystems. The negative correlation between radial growth in high-altitude larch and temperature indirectly suggests that larch prefer a moist environment, with its growth primarily influenced by soil moisture content. In October, which coincides with the end of the rainy season, soil moisture content and air humidity are relatively high. During this time, the response to precipitation is not very pronounced, and the average temperature becomes the primary influencing factor. A high average temperature accelerates the evaporation of water from both the soil and the air, which is unfavorable for tree growth activities. In contrast, the temperature in March has a negative impact on radial tree growth. This negative response may be linked to the premature temperature increase in the region, which is not conducive to the subsequent growth of trees.
4.3 Dynamic response of radial growth of larch at different altitudes to climate change
The sliding correlation analysis between the standard tree-ring chronologies of larch at different altitudes and precipitation and temperature (Figure 5) reveals the presence of varying degrees of dynamic response relationships between larch radial growth and climate factors at different altitudes. In low-altitude regions, the response of tree radial growth to temperature maintains a stable negative correlation, particularly during the previous and current winter and early spring months, while the response to precipitation gradually shifts toward a positive correlation. With ongoing climate warming, the increase in winter temperatures may lead to reduced snow cover and shallower soil freezing depths. This reduction in snow cover and frozen soil diminishes the protective insulation around tree roots, exposing them to harsher external conditions. This enhances the low temperature stress experienced by trees during the winter, limiting their physiological activities during this period and consequently resulting in a stable negative correlation during the winter and early spring months. At the same time, the water released by melting snow is similar to rainfall due to the slow and long-lasting melting process and the water supply provided by snowfall has a beneficial effect on the growth of trees. When moisture is insufficient in the late growth period, snowmelt can provide compensatory moisture (Zhang et al., 2019). The correlation between intermediate altitude larch with winter and August temperatures gradually diminishes, while correlations with temperatures in May and June gradually become significantly positive. Moreover, the response of larch at this altitude to precipitation in June shifts from positive to negative. This trend indicates that during spring, when the tree cambium layer becomes active, enhanced photosynthesis occurs, and warmer weather provides the energy and necessary temperature range for growth. However, this positive correlation weakens over time, particularly in February of the same year, possibly due to early warming conditions not being conductive to subsequent tree growth. In the Daxing’anling region, the rainy season can influence water competition among trees and induce soil waterlogging, which can inhibit root respiration (Baltzer et al., 2014). Winter precipitation at high altitudes in the Greater Khingan Mountains represents a dynamic and complex process, the continuous thawing of permafrost over several years ensures adequate soil moisture. Snow cover not only supplies moisture and protection for tree growth but also has the potential to influence the timing of growth events.
5 Conclusion
The growth trends of Larix gmelinii in the northern part of the Greater Khingan Mountains and their response to climate indicate the radial growth–climate relationship to be influenced by the elevation gradient. The larches at three different altitudes exhibit varying radial growth–climate patterns, and thus the tree radial growth–climate relationship is elevation-specific. Comparisons revealed that precipitation correlated with the radial growth of larch at lower altitudes, with increasing elevation, the growth pattern gradually shifts to be temperature-dependent, and differences are observed in key month. When the temperature exceeds a certain threshold, high-altitude larch (1,300 m) may gradually form alpine forest lines in the study area, which can accelerate the natural succession of larch. With the ongoing climate warming, larch trees in the low-altitude area (900 m) exhibited a slow growth trend over the past 30 years. Their growth of larch at this altitude is primarily influenced positively by precipitation, while the inhibitory effect of temperature on growth is also gradually increasing. In the mid-altitude area (1,120 m), larch trees exhibited a rapid growth trend that was mainly driven by the promoting effect of temperature, and their sensitivity to temperature increased gradually during the growing season.
Larix is the dominant tree species in the Greater Khingan Mountains, with a wide distribution and complex ecological environment. Future research can increase research density by conducting dendrochronological studies across various altitudes and for multiple tree species. This can provide further insights into the dynamic changes of trees in the context of global climate warming and their impact on ecosystem stability.
Data availability statement
The data that support the findings of this study are not openly available and are available from the corresponding author upon reasonable request.
Author contributions
TL: Conceptualization, Data curation, Investigation, Methodology, Resources, Software, Writing – original draft, Writing – review & editing. ZW: Investigation, Methodology, Software, Writing – review & editing. DZ: Data curation, Funding acquisition, Investigation, Methodology, Resources, Software, Supervision, Writing – review & editing. XL: Writing – review & editing. XW: Investigation, Writing – review & editing.
Funding
The author(s) declare that financial support was received for the research, authorship, and/or publication of this article. This research was supported by the National Natural Science Foundation of China (No. 41671064) and the Natural Science Foundation of Heilongjiang Province of China (No. TD2023D005).
Conflict of interest
The authors declare that the research was conducted in the absence of any commercial or financial relationships that could be construed as a potential conflict of interest.
Publisher’s note
All claims expressed in this article are solely those of the authors and do not necessarily represent those of their affiliated organizations, or those of the publisher, the editors and the reviewers. Any product that may be evaluated in this article, or claim that may be made by its manufacturer, is not guaranteed or endorsed by the publisher.
References
Akkemik, Ü. (2000). Dendroclimatology of umbrella pine (Pinus pinea L.) in Istanbul, Turkey. Tree-Ring Bulletin 56, 17–20.
Baltzer, J. L., Veness, T., Chasmer, L. E., Sniderhan, A. E., and Quinton, W. L. (2014). Forests on thawing permafrost: fragmentation, edge effects, and net forest loss. Glob. Chang. Biol. 20, 824–834. doi: 10.1111/gcb.12349
Billings, W. D. (1969). Vegetational pattern near alpine timberline as affected by fire-snowdrift interactions. Vegetatio 19, 192–207. doi: 10.1007/bf00259010
Bockheim, J., Vieira, G., Ramos, M., López-Martínez, J., Serrano, E., Guglielmin, M., et al. (2013). Climate warming and permafrost dynamics in the Antarctic peninsula region. Glob. Planet. Chang. 100, 215–223. doi: 10.1016/j.gloplacha.2012.10.018
Cabral-Alemán, C., Villanueva-Díaz, J., Quiñonez-Barraza, G., and Gómez-Guerrero, A. (2022). Resilience of Pinus durangensis Martínez in extreme drought periods: vertical and horizontal response of tree rings. Atmosphere-Basel 14:43. doi: 10.3390/atmos14010043
Cai, Q., Liu, Y., Fang, C., Zhang, H., Song, H., Li, Q., et al. (2020). Ground surface temperature reconstruction for the Jinggangshan Mountains: interpreting the hydro-thermal coupling pattern in southeastern China. Quaternary Sci Rev. 249:106591. doi: 10.1016/j.quascirev.2020.106591
Case, M. J., and Peterson, D. L. (2007). Growth-climate relations of lodgepole pine in the North cascades National Park, Washington. Northwest Sci. 81, 62–75. doi: 10.3955/0029-344X-81.1.62
Chapin, F. S. III, Randerson, J. T., McGuire, A. D., Foley, J. A., and Field, C. B. (2008). Changing feedbacks in the climate-biosphere system. Front. Ecol. Environ. 6, 313–320. doi: 10.1890/080005
Chen, L., Huang, J. G., Stadt, K. J., Comeau, P. G., Zhai, L., Dawson, A., et al. (2017). Drought explains variation in the radial growth of white spruce in western Canada. Agric. For. Meteorol. 233, 133–142. doi: 10.1016/j.agrformet.2016.11.012
Chen, R., Liu, J., Zhang, W., and Wang, F. (2023). Effects of climate on the radial growth of Larix gmelinii under different competition intensities in the greater Khingan mountains. Appl Ecol Env Res. 21, 2075–2089. doi: 10.15666/aeer/2103_20752089
Chhin, S., Hogg, E. T., Lieffers, V. J., and Huang, S. (2008). Potential effects of climate change on the growth of lodgepole pine across diameter size classes and ecological regions. Forest Ecol. Manag. 256, 1692–1703. doi: 10.1016/j.foreco.2008.02.046
Cook, E. R. (1985). A time series analysis approach to tree ring standardization. Ph.D. Thesis,. Tucson, AZ, USA: University of Arizona Tucson.
Gindl, W., Grabner, M., and Wimmer, R. (2000). The influence of temperature on latewood lignin content in treeline Norway spruce compared with maximum density and ring width. Trees 14, 409–414. doi: 10.1007/s004680000057
Goodine, G. K., Lavigne, M. B., and Krasowski, M. J. (2008). Springtime resumption of photosynthesis in balsam fir (Abies balsamea). Tree Physiol. 28, 1069–1076. doi: 10.1093/treephys/28.7.1069
Guo, Y. D., Song, C. C., Wan, Z. M., Lu, Y. Z., Qiao, T. H., Tan, W. W., et al. (2015). Dynamics of dissolved organic carbon release from a permafrost wetland catchment in Northeast China. J. Hydrol. 531, 919–928. doi: 10.1016/j.jhydrol.2015.10.008
Harsch, M. A., Hulme, P. E., McGlone, M. S., and Duncan, R. P. (2009). Are treelines advancing? A global meta-analysis of treeline response to climate warming. Ecol. Lett. 12, 1040–1049. doi: 10.1111/j.1461-0248.2009.01355.x
Hartl-Meier, C., Dittmar, C., Zang, C., and Rothe, A. (2014). Mountain forest growth response to climate change in the northern limestone Alps. Trees 28, 819–829. doi: 10.1007/s00468-014-0994-1
Havranek, W. M., and Tranquillini, W. (1995). Physiological processes during winter dormancy and their ecological significance//ecophysiology of coniferous forests. Cambridge, Massachusetts, USA: Academic Press, 95–124.
He, M., Shishov, V., Kaparova, N., Yang, B., Bräuning, A., and Grießinger, J. (2017). Process-based modeling of tree-ring formation and its relationships with climate on the Tibetan plateau. Dendrochronologia 42, 31–41. doi: 10.1016/j.dendro.2017.01.002
Helbig, M., Wischnewski, K., Kljun, N., Chasmer, L. E., Quinton, W. L., Detto, M., et al. (2016). Regional atmospheric cooling and wetting effect of permafrost thaw-induced boreal forest loss. Glob. Chang. Biol. 22, 4048–4066. doi: 10.1111/gcb.13348
Hessburg, P. F., Miller, C. L., Parks, S. A., Povak, N. A., Taylor, A. H., Higuera, P. E., et al. (2019). Climate, environment, and disturbance history govern resilience of western North American forests. Front. Ecol. Evol. 7:239. doi: 10.3389/fevo.2019.00239
Hirano, T., Suzuki, K., and Hirata, R. (2017). Energy balance and evapotranspiration changes in a larch forest caused by severe disturbance during an early secondary succession. Agric. For. Meteorol. 232, 457–468. doi: 10.1016/j.agrformet.2016.10.003
Hoff, U., Biskaborn, B. K., Dirksen, V. G., Dirksen, O., Kuhn, G., Meyer, H., et al. (2015). Holocene environment of Central Kamchatka, Russia: implications from a multi-proxy record of two-yurts Lake. Glob. Planet. Chang. 134, 101–117. doi: 10.1016/j.gloplacha.2015.07.011
Holmes, R. L. (1983). Computer-assisted quality control in tree-ring dating and measurement; tree-ring society: Tucson. USA: AZ.
Huang, J., Tardif, J. C., Bergeron, Y., Denneler, B., Berninger, F., and Girardin, M. P. (2010). Radial growth response of four dominant boreal tree species to climate along a latitudinal gradient in the eastern Canadian boreal forest. Glob. Chang. Biol. 16, 711–731. doi: 10.1111/j.1365-2486.2009.01990.x
Jyske, T., Mäkinen, H., Kalliokoski, T., and Nöjd, P. (2014). Intra-annual tracheid production of Norway spruce and scots pine across a latitudinal gradient in Finland. Agric. For. Meteorol. 194, 241–254. doi: 10.1016/j.agrformet.2014.04.015
Kharuk, V. I., Ranson, K. J., Im, S. T., and Dvinskaya, M. L. (2009). Response of Pinus sibirica and Larix sibirica to climate change in southern Siberian alpine forest-tundra ecotone. Scandi J Forest Res. 24, 130–139. doi: 10.1080/02827580902845823
Kirdyanov, A., Hughes, M., Vaganov, E., Schweingruber, F., and Silkin, P. (2003). The importance of early summer temperature and date of snow melt for tree growth in the Siberian subarctic. Trees 17, 61–69. doi: 10.1007/s00468-002-0209-z
Kitudom, N., Fauset, S., Zhou, Y., Fan, Z., Li, M., He, M., et al. (2022). Thermal safety margins of plant leaves across biomes under a heatwave. Sci. Total Environ. 806:150416. doi: 10.1016/j.scitotenv.2021.150416
Kolář, T., Čermák, P., Trnka, M., Žid, T., and Rybníček, M. (2017). Temporal changes in the climate sensitivity of Norway spruce and European beech along an elevation gradient in Central Europe. Agric. For. Meteorol. 239, 24–33. doi: 10.1016/j.agrformet.2017.02.028
Kosaka, Y., and Xie, S. P. (2013). Recent global-warming hiatus tied to equatorial Pacific surface cooling. Nature 501, 403–407. doi: 10.1038/nature12534
Kueppers, L. M., Conlisk, E., Castanha, C., Moyes, A. B., Germino, M. J., de Valpine, P., et al. (2017). Warming and provenance limit tree recruitment across and beyond the elevation range of subalpine forest. Global Change Bio. 23, 2383–2395. doi: 10.1111/gcb.13561
Leng, W., He, H. S., Bu, R., Dai, L., Hu, Y., and Wang, X. (2008). Predicting the distributions of suitable habitat for three larch species under climate warming in northeastern China. Forest Ecol Manag. 254, 420–428. doi: 10.1016/j.foreco.2007.08.031
Li, W., Jiang, Y., Dong, M., Du, E., Wu, F., Zhao, S., et al. (2021). Species-specific growth-climate responses of Dahurian larch (Larix gmelinii) and Mongolian pine (Pinus sylvestris var. mongolica) in the greater Khingan range, Northeast China. Dendrochronologia 65:125803. doi: 10.1016/j.dendro.2020.125803
Linares, J. C., and Tíscar, P. A. (2011). Buffered climate change effects in a Mediterranean pine species: range limit implications from a tree-ring study. Oecologia 167, 847–859. doi: 10.1007/s00442-011-2012-2
Lo, Y. H., Blanco, J. A., Seely, B., Welham, C., and Kimmins, J. H. (2010). Relationships between climate and tree radial growth in interior British Columbia, Canada. Forest Ecol Manag. 259, 932–942. doi: 10.1016/j.foreco.2009.11.033
Loehle, C., and LeBlanc, D. (1996). Model-based assessments of climate change effects on forests: a critical review. Ecol. Model. 90, 1–31. doi: 10.1016/0304-3800(96)83709-4
Matías, L., Linares, J. C., Sánchez-Miranda, Á., and Jump, A. S. (2017). Contrasting growth forecasts across the geographical range of scots pine due to altitudinal and latitudinal differences in climatic sensitivity. Glob. Chang. Biol. 23, 4106–4116. doi: 10.1111/gcb.13627
Morgan, C., Losey, A., and Trout, L. (2014). Late-Holocene paleoclimate and treeline fluctuation in Wyoming’s Wind River range, USA. The Holocene 24, 209–219. doi: 10.1177/0959683613516817
Moritz, C., and Agudo, R. (2013). The future of species under climate change: resilience or decline? Science 341, 504–508. doi: 10.1126/science.1237190
Oberhuber, W. (2004). Influence of climate on radial growth of Pinus cembra within the alpine timberline ecotone. Tree Physiol. 24, 291–301. doi: 10.1093/treephys/24.3.291
Park Williams, A., Allen, C. D., Macalady, A. K., Griffin, D., Woodhouse, C. A., Meko, D. M., et al. (2013). Temperature as a potent driver of regional forest drought stress and tree mortality. Nat. Clim. Chang. 3, 292–297. doi: 10.1038/nclimate1693
Rehfeldt, G. E., and Jaquish, B. C. (2010). Ecological impacts and management strategies for western larch in the face of climate-change. Mitig. Adapt. Strat. Gl. 15, 283–306. doi: 10.1007/s11027-010-9217-2
Ren, P., Rossi, S., Camarero, J. J., Ellison, A. M., Liang, E., and Peñuelas, J. (2018). Critical temperature and precipitation thresholds for the onset of xylogenesis of Juniperus przewalskii in a semi-arid area of the north-eastern Tibetan plateau. Ann Bot-Landon 121, 617–624. doi: 10.1093/aob/mcx188
Salzer, M. W., Hughes, M. K., Bunn, A. G., and Kipfmueller, K. F. (2009). Recent unprecedented tree-ring growth in bristlecone pine at the highest elevations and possible causes. P Natl A Sci. 106, 20348–20353. doi: 10.1073/pnas.0903029106
Serre, F. (1978). The dendroclimatological value of the European larch (Larix decidua mill.) in the French maritime Alps. Tree-Ring Bull. 38, 25–34.
Sidor, C. G., Popa, I., and Vlad, R. (2015). Different tree-ring responses of Norway spruce to air temperature across an altitudinal gradient in the eastern Carpathians (Romania). Trees 29, 985–997. doi: 10.1007/s00468-015-1178-3
Soja, A. J., Tchebakova, N. M., French, N. H., Flannigan, M. D., Shugart, H. H., Stocks, B. J., et al. (2007). Climate-induced boreal forest change: predictions versus current observations. Glob. Planet. Chang. 56, 274–296. doi: 10.1016/j.gloplacha.2006.07.028
Stokes, M., and Smiley, T. (1968). An introduction to tree-ring dating. Tucson: University of Arizona Press.
Sun, M., Li, J., Cao, R., Tian, K., Zhang, W., Yin, D., et al. (2021). Climate-growth relations of Abies georgei along an altitudinal gradient in Haba Snow Mountain, Southwestern China. Forests 9:1569. doi: 10.3390/f9100606
Tchebakova, N. M., Rehfeldt, G. E., and Parfenova, E. I. (2010). From vegetation zones to climatypes: effects of climate warming on Siberian ecosystems. Permafrost Ecosyst. 427–446. doi: 10.1007/978-1-4020-9693-8_22
Tessier, L., Guibal, F., and Schweingruber, F. H. (1997). Research strategies in dendroecology and dendroclimatology in mountain environments. Climatic Change High Elevation Sites. 267–285. doi: 10.1007/978-94-015-8905-5_14
Tollefson, J. (2018). IPCC says limiting global warming to 1.5 [degrees] C will require drastic action. Nature 562, 172–174.1. doi: 10.1038/d41586-018-06876-2
Tranquillini, W. (2012). Physiological ecology of the alpine timberline: Tree existence at high altitudes with special reference to the European Alps. Geneva, Switzerland: Springer Science & Business Media.
Ustin, S. L., and Xiao, Q. F. (2001). Mapping successional boreal forests in interior Central Alaska. Int. J. Remote Sens. 22, 1779–1797. doi: 10.1080/01431160118269
Vaganov, E. A., Hughes, M. K., Kirdyanov, A. V., Schweingruber, F. H., and Silkin, P. P. (1999). Influence of snowfall and melt timing on tree growth in subarctic Eurasia. Nature 400, 149–151. doi: 10.1038/22087
Vila, B., Vennetier, M., Ripert, C., Chandioux, O., Liang, E., Guibal, F., et al. (2008). Has global change induced opposite trends in radial growth of Pinus sylvestris and Pinus halepensis at their bioclimatic limit? The example of the Sainte-Baume forest (south-East France). Ann. For. Sci. 65:709. doi: 10.1051/forest:2008048
Wei, Z., Jin, H., Zhang, J., Yu, S., Han, X., Ji, Y., et al. (2011). Prediction of permafrost changes in northeastern China under a changing climate. Sci. China Earth Sci. 54, 924–935. doi: 10.1007/s11430-010-4109-6
Wilmking, M., Juday, G. P., Barber, V. A., and Zald, H. S. (2004). Recent climate warming forces contrasting growth responses of white spruce at treeline in Alaska through temperature thresholds. Glob. Chang. Biol. 10, 1724–1736. doi: 10.1111/j.1365-2486.2004.00826.x
Yang, R. Q., Fan, Z. X., Li, Z. S., and Wen, Q. Z. (2018). Radial growth of Pinus yunnanensis at different elevations and their responses to climate factors in the Yulong Snow Mountain, Northwest Yunnan. China. Acta. Ecol. Sin. 38, 8983–8991. doi: 10.5846/stxb201805311214
Zhang, X., Bai, X., Chang, Y., and Chen, Z. (2016). Increased sensitivity of Dahurian larch radial growth to summer temperature with the rapid warming in Northeast China. Trees 30, 1799–1806. doi: 10.1007/s00468-016-1413-6
Zhang, Q. B., and Hebda, R. J. (2004). Variation in radial growth patterns of Pseudotsuga menziesii on the central coast of British Columbia, Canada. Can. J. For. Res. 34, 1946–1954. doi: 10.1139/x04-078
Zhang, Y., Ishikawa, M., Ohata, T., and Oyunbaatar, D. (2008). Sublimation from thin snow cover at the edge of the Eurasian cryosphere in Mongolia. Hydrol. Process. 22, 3564–3575. doi: 10.1002/hyp.6960
Zhang, X., Liu, X., Zhang, Q., Zeng, X., Xu, G., Wu, G., et al. (2018). Species-specific tree growth and intrinsic water-use efficiency of Dahurian larch (Larix gmelinii) and Mongolian pine (Pinus sylvestris var. mongolica) growing in a boreal permafrost region of the greater Hinggan Mountains, Northeastern China. Agr. For. Meteorol. 248, 145–155. doi: 10.1016/j.agrformet.2017.09.013
Zhang, X., Manzanedo, R. D., D'Orangeville, L., Rademacher, T. T., Li, J., Bai, X., et al. (2019). Snowmelt and early to mid-growing season water availability augment tree growth during rapid warming in southern Asian boreal forests. Glob. Chang. Biol. 25, 3462–3471. doi: 10.1111/gcb.14749
Keywords: Greater Khingan Mountains, Larix gmelinii , tree-ring width, radial growth, climate response
Citation: Luo T, Wang Z, Zhang D, Li X and Wang X (2024) Response of radial growth of Dahurian larch (Larix gmelinii) to climate factors at different altitudes in the northern part of the Greater Khingan Mountains. Front. For. Glob. Change. 7:1434773. doi: 10.3389/ffgc.2024.1434773
Edited by:
Aldo Rafael Martínez Sifuentes, National Institute of Forestry and Agricultural Research (INIFAP), MexicoReviewed by:
Ulises Manzanilla Quiñones, Michoacana University of San Nicolás de Hidalgo, MexicoClaudia Cecilia Astudillo Sánchez, Universidad Autónoma de Tamaulipas, Mexico
Copyright © 2024 Luo, Wang, Zhang, Li and Wang. This is an open-access article distributed under the terms of the Creative Commons Attribution License (CC BY). The use, distribution or reproduction in other forums is permitted, provided the original author(s) and the copyright owner(s) are credited and that the original publication in this journal is cited, in accordance with accepted academic practice. No use, distribution or reproduction is permitted which does not comply with these terms.
*Correspondence: Dongyou Zhang, emhhbmdkeUBocmJudS5lZHUuY24=